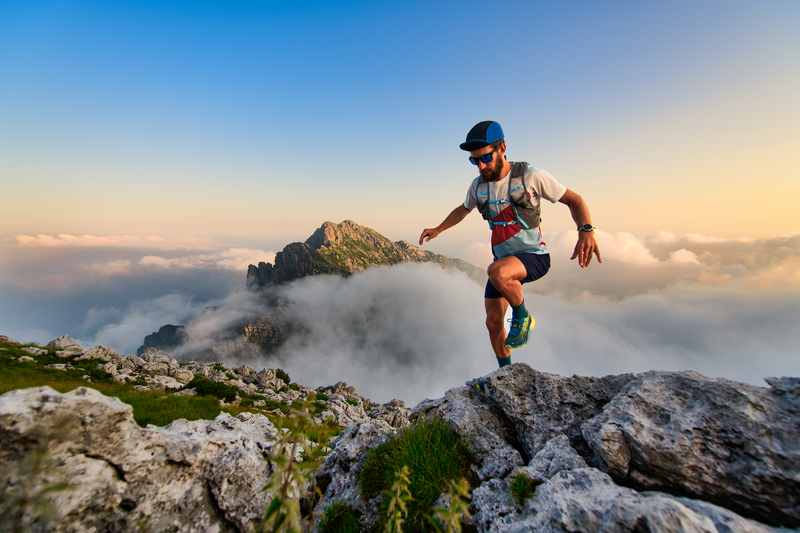
95% of researchers rate our articles as excellent or good
Learn more about the work of our research integrity team to safeguard the quality of each article we publish.
Find out more
REVIEW article
Front. Built Environ. , 25 July 2024
Sec. Geotechnical Engineering
Volume 10 - 2024 | https://doi.org/10.3389/fbuil.2024.1396542
The stabilization and application of expansive geomaterials are critical in geotechnical engineering. These naturally expansive materials exhibit complex hydro-chemo-mechanical properties because they undergo volumetric changes in response to variations in moisture content and/or temperature. The characteristic shrink-swell behavior of these materials makes their use problematic and plays a substantial role in influencing the stability of geo-infrastructure applications. However, there is a lack of comprehensive knowledge of the mechanisms and factors impacting their behavior to ensure mechanical integrity in natural and built infrastructure and geo-engineering projects. This work provides a comprehensive review of the intrinsic and extrinsic factors contributing to the shrink-swell behavior and expansion mechanisms of frost-heaving and natural-expansive geomaterials, such as expansive clays and sulfate minerals. We reviewed and synthesized peer-reviewed published works in various databases and academic repositories in the last 100 years. The influence of shrink-swell behavior of these geomaterials and the critical role they play in engineering infrastructure were highlighted, explicitly focusing on their involvement in geotechnical-related hazards, such as the freeze-thaw cycle, and the damage and sulfate-attack of geo-infrastructure. We analyzed the interactions between clay minerals, especially how bentonite enhances grout stability and acts as a buffer material in high-level nuclear waste repositories. The findings indicate that water interaction with geomaterials and concrete can cause about a 10% volume expansion when frozen. Also, the exposure of fractured rocks to low (≤0°C) and high (>0°C) temperatures can greatly change rock deformation and strength. Finally, gypsum interacting with water can theoretically increase in volume by 62% to form ice crystals. This forward-leading review presents the advantages, disadvantages, and unresolved issues of expansive natural geotechnical materials that improve the resiliency and sustainability of geological infrastructure.
Natural expansive geomaterials are essential to geotechnical engineering due to their unique properties during the construction and reinforcement of surface, near-surface, and underground infrastructure. These natural expansive materials exhibit complex hydro-chemo-mechanical properties, and their use poses a threat because they undergo volumetric changes in response to variations in moisture content and/or temperature.
These geomaterials often exhibit a freeze-thaw behavior, and their expansion and shrinkage mechanism can be detrimental to geo-infrastructure. Geo-infrastructure refers to infrastructures built on or around geomaterials (rocks and soils) on the surface, near-surface, and deep underground environments that support human activities. The inherent volume change characteristics of naturally expansive materials can lead to cracking and deformations (i.e., failures), which play a substantial role in influencing the stability and geotechnical-related hazards, especially in slopes (Qi and Vanapalli, 2015), underground tunnels (Schullera and Schweiger, 2002), structures (Liu et al., 2016), wellbore (Zhang et al., 1999), and in underground mining (Feng et al., 2022). The problematic results associated with these materials have led researchers to address innovative approaches to mitigate geomaterials' freeze-thaw cycles and shrink-swell behavior, especially in cold regions in our dynamic, fast-paced age. The typical contributing factors that result in the volume change of these materials are the variations in temperature, moisture content, pressure, and chemical reaction, and the details are: (i) the soil and rock masses undergo freeze-thaw cycles because of temperature variations, and these cycles cause phase transitions between water and ice, as the volume of ice is greater than that of water, which may induce the volume expansion; and (ii) some natural soils and rocks contains mineral constituents that could cause volume expansion owing to water absorption and this are the clay minerals and sulfate minerals.
Generally, frost-heaving behavior in geomaterials is one of the leading causes of damage to geo-infrastructure in cold climates, especially in the winter season (Huang et al., 2020). The freeze-thaw settlement of structures on soft clay significantly impacts the geotechnical characteristics of soft soils in cold regions (Konrad and Morgenstern, 1980; Barker and Thomas, 2013). Also, the seasonal and alternating temperatures can cause freeze-thaw cycles in soil and rocks, disturbing engineered underground infrastructure and resulting in potential hazards. Frost heaving varies based on humidity and soil conditions, which can result in non-uniform deformation of railway and highway subgrade constructed on permafrost (Chen Y. et al., 2020). Geo-infrastructure projects built in freeze-thaw geomaterials are one of the most prevalent challenges in the world (Matsuoka, 2001). mentioned that rocks can uptake water during slow freezing, and thus, for frost damage, high initial water content is unnecessary. Consequently, the implications of frost heaving on geotechnical practices in cold regions have been investigated by several researchers. Michaud and Dyke, (2008) discussed the mechanism of bedrock frost heave in permafrost regions, emphasizing the potential threat it poses to engineering design stability.
Shi et al. (2020) analyze the influence of soil frost heaving on the internal force and displacement of foundation pit supporting structures. Jiang et al. (2023) focused on the frost-heaving characteristics of hydraulic tunnel wall rock in cold regions, emphasizing the spatial distribution and variation of frozen areas and frost-heaving forces. Low temperatures, especially below-freezing points, significantly affect surrounding rock stability in rock engineering projects. Prolonged exposure to negative temperature and freezing conditions alters the deformation and intensity of fractured rocks (Wang and Zhou, 2018). As a result, in the study of rock tunneling in cold regions, the occurrence and expansion of the frost-heaving temperature have been addressed to prevent and reduce frost damage. Studies have shown that the temperature at the entrance section of tunnels is significantly affected by the colder air both inside and outside the tunnel during the cold season, consequently making it the main area susceptible to freezing damage (Yu et al., 2019; Zhou et al., 2021). Zhang et al. (2022) reported that the decrease in temperature in the tunnel causes the freezing of water, and the volume expansion damages the tunnel structure further, causing other forms of freezing damage. This highlights the critical role of low temperatures in driving freezing damage. Furthermore, freezing damage to tunnel foundations encompasses various aspects, including failures in the drainage system, snowmelt and ice formation on roadcut surfaces, foundation seepage, and icing issues (Li et al., 2022).
Natural expansive geomaterials cause many problems in geo-engineering and vary significantly in volume; thus, they expand as they absorb water and shrink as they evaporate (Pooni et al., 2019). Natural expansive geomaterials (expansive soils) are common worldwide, covering about 33% of Sudan, 20% of Indonesia and India, 12% of Syria, and approximately 6% of China (Jalal et al., 2020), and their existence severely slows down geotechnical projects and causes long-term stability problems (Aziz et al., 2015). Several researchers have discussed the inter-particle swelling and intercrystalline expansion mechanism of expansive soils and pointed out that the economic losses due to the alternating shrinkage and expansion behavior of these materials outweigh the damage caused by natural disasters (Jones and Holtz, 1973). Basma et al. (1996) found that cyclic swelling and shrinkage of expansive clays can result in changes in their expansive behavior and microstructure. Lajurkar et al. (2013) highlighted the damaging effects of alternate swelling and shrinkage on structures built on expansive soils. Phanikumar and Singla, (2016) discussed the problems posed by expansive soils and explored the efficiency of fiber reinforcement in reducing swelling and shrinkage. Muthukumar and Shukla (2019) explained that the swelling decreased slightly with an increase in fiber content, while shrinkage significantly decreased with the addition of fibers.
Comparatively, sulfates cover a significant portion of the Earth’s surface, making them a crucial area of interest for researchers due to their significance in both geological and environmental contexts. The diverse nature of sulphates and their widespread distribution demands thorough investigation to understand their implications and effects on geo-systems. Joanna, (2012) highlighted that sulphates cover a significant portion of the Earth’s surface, including coastal salt lakes, sabkhas, and salt lakes in different regions. Tarragona, (2014) discussed the expansion mechanisms of sulphated rocks and soils, emphasizing the role of gypsum precipitation in discontinuities, which can lead to swelling strains and geo-structural damage. Maio et al. (2014) investigated the natural occurrence of sulphates in groundwater, suggesting that gypsum formations associated with specific carbonate rocks are the predominant source of sulphates in a particular area. Samborska et al. (2013) studied Triassic carbonate aquifers in Upper Silesia, Poland, and found that sulphate sources included sulphide weathering and gypsum dissolution. Fontboté et al. (2017) focused on sulfide minerals in hydrothermal deposits, which play a crucial role in concentrating metals and triggering the deposition of valuable metals through the precipitation of less economically significant sulfides. These studies represent a diverse range of investigations into sulphates and shed light on their geological significance, expansion mechanisms and natural occurrence on earth.
The behavior of expansive soils, sulfate attack, and freeze-thaw cycles can influence the volumetric expansion of cement structures. Over time, the shrinkage and expansion of expansive soil can cause foundation movement, cracking, and structural damage (Holtz et al., 2011). Sulfates can penetrate concrete and react with calcium hydroxide and hydrated calcium aluminate to form expanding compounds that can cause cracking, spalling and loss of structural integrity (Al-Dulaijan et al., 2003; Mamun and Bindiganavile, 2011; Min et al., 2019; Othman et al., 2020; Zhang et al., 2021; Tian et al., 2023). Freeze and thaw cycles can create internal stresses within the concrete and lead to structural damage and failure (Valenza and Scherer, 2007; Dabas et al., 2021).
The objective of this study is to comprehensively review the expansion behavior and mechanisms of natural expansive materials and frost-heaving behavior in geo-infrastructure. Further, this study will provide an innovative review of: i) the relationship between several potential underlying factors, including moisture content, temperature, and pressure contributing to the volume expansion and shrinkage variations of natural expansive materials; ii) consequences of material expansion and shrinkage for mitigating these challenges in geotechnical engineering, and iii) ongoing efforts to enhance the resilience and sustainability of geo-engineering practices in the face of variable natural expansive material behavior. The rest of the paper is organized as follows: i) Section 2 introduces the swelling-shrinkage behavior of expansive natural materials, and Section 3 covers swelling-shrinkage mechanisms of natural expansive materials; ii) Section 4 focuses on the impact of freeze-thaw cycles and sulfate exposure on the durability of natural and built infrastructure, and Section 5 presents the significance and implications of natural expansive materials in geotechnical engineering applications, and iii) lastly, these sections will be followed by the conclusion section and future research direction section.
Many types of soils and rock masses show frost-heaving behavior during the freeze-thaw cycles because of temperature variations when they contain water. Generally, frost heaving occurs in the northern hemisphere (cold regions) countries like America, Canada, Russia, and the Nordic regions e.g., Denmark, Finland, Iceland, Norway, Sweden, and Greenland (Brown et al., 1998; Prince et al., 2018) (Figure 1A), and its influence is a problem of national concern since it delays many engineering projects (Taivainen, 1963). The effect of frost heaving causes a lot of damage to geo-structures, such as pipelines (Oswell, 2011), subgrades (Wu et al., 2018), foundations, tunnels, etc.
Figure 1. Freezing and thawing in geomaterials in the Northern Hemisphere: (A) Northern Hemisphere land cover [tundra (blue), forest (green), open land (yellow), and water/ice (white); Red dots mark weather stations] [modified after (Prince et al., 2018)]; (B) Permafrost distribution in the Nordic area based on Circum-Arctic Permafrost Map [modified after (Brown et al., 1998)].
The prevalence of seasonal freezing and thawing in the northern hemisphere has far-reaching implications with more than half of the northern hemisphere’s land area (Figure 1A) (Prince et al., 2018). Within this vast region, a significant portion, precisely one-quarter as well as 17% of the Earth’s exposed land surface is characterized by permafrost, where the ground remains frozen at or below 0°C for a minimum of two consecutive years (Christiansen et al., 2010; Gruber, 2012; Biskaborn et al., 2019). In China, there is an extensive distribution of frozen soil in the northern climates like Tibet, Xinjiang, and Qinghai, where the temperature is low (below 0°C), large frozen depths, and long durations in the soil throughout two-thirds of the time in a year (Liu et al., 2017). It is reported that engineers working at underground tunnels in these areas in China often observe ice in fractured rock mass at low temperatures, which sometimes causes the fissure volume to expand, resulting in underground instability (Wang et al., 2016b). Permafrost in the Nordic region can be found in lowland areas with marine sediments, palsas, and peat plateaus, and in many mountainous regions at temperatures near 0.8°C (Figure 1B), making it highly responsive to climate variations (Christiansen et al., 2010).
In locations such as Svalbard, northeast Greenland, and the highest mountainous zones throughout the Nordic area, permafrost is slightly colder but still just a few degrees above freezing (Christiansen et al., 2010). Notably, in Finland, the penetration of frost into glacial soils exhibits distinct regional patterns; thus, in the southern part of the country, frost reaches depths of approximately 1.22 m–1.83 m (4–6 ft), while in the northern part, this freezing phenomenon extends much deeper, ranging from 2.13 m to 3.05 m (7–10 ft) which accounts for more frost occurrence in the northern climate of Finland (Taivainen, 1963). As a result, boundary markers often shift from their designated positions due to the upward movement of rocks resulting from the volumetric expansion of ice beneath road beds (Taivainen, 1963).
Extensive research on frost-heaving has been conducted since the early 1990s till date. Taber (1930) and Taber (1929) explored the frost-heaving mechanisms such as the growth of ice lenses in soil. Mu and Ladanyi, (1987) developed models that integrated stress-strain behavior, heat, and mass transport to estimate frost heave. Matsuoka (1990) focused on measuring frost-heaving strains in rocks, observing the influence of surface area on freezing expansion (Huang et al., 2020). Dagli et al. (2018) investigated the role of suction in water migration to the frost front. Wang and Zhou, (2018) emphasized how jointed rock mass properties can affect frost-heaving pressure due to phase changes. Osokin et al. (2000), Rekstad et al. (2013), and Liu et al. (2017) highlighted the importance of freezing and negative temperatures in soil, leading to volume expansion and negative pressure formation in frozen areas.
Frost heaving is primarily affected by factors such as temperature and pressure, and the details of each factor are expressed below. However, it is important to recognize that the study of this phenomenon requires a comprehensive understanding of multiple contributing factors (Lu et al., 2021). The change in volume resulting from the freezing of water and the subsequent melting of ice serves as the fundamental trigger for soil deformation within a freezing-thawing environment. Periodic frozen soil is susceptible to temperature variation (Niu et al., 2017; Niu et al., 2017; Lin et al., 2018), and the cyclic pattern of freezing and thawing of soil significantly affects soil strength. Bouyoucos (1920) studied soil physics and chemistry and discovered that water in the soil freezes at multiple temperatures, not just one. Huixin et al. (2012) pointed out that in a closed system, as the freezing temperature rises, the amount of frost heave and the ratio of frost heave relative to the soil mass water content and dry density also increase. Liu et al. (2017) identified frost heave in coarse-grained soils when specific combinations of clay content (the mass fraction of the particle with a diameter less than 0.075 mm), initial moisture, and temperature occurred in seasonal frozen regions.
In rock mass, as the freezing temperature decreases, the unfrozen water film thickness between the rock and the ice decreases, leading to an increase in disjoining pressure (De Gennes, 1985; Rempel et al., 2001; Huang et al., 2020). Wang and Zhou (2018) explained that under freezing conditions and low temperatures, prolonged rock exposure can lead to significant changes in the deformation and strength characteristics of the fractured rock mass. Penner (1959) stated that structures and roadways built on materials highly susceptible to frost, along with their associated expansion, could heave up to 0.61 m. Brimblecombe et al. (2010) stated that the count of freeze-thaw cycles can be determined based on the assumption that rocks freeze solely when the mean daily temperature drops below −3°C, and thawing only occurs when the mean daily temperature rises above +1°C. Recently, a novel technique has been developed that utilizes meteorological data to analyze the daily maximum and minimum temperatures. This method entails monitoring and quantifying freeze-thaw cycles, which are defined by temperature fluctuations either from above freezing to below 0°C or from below 0°C to above freezing (Al-Omari et al., 2015). Table 1 provides a comprehensive overview of the geographic distribution of frost-heaving geomaterials and addresses their implications from a geotechnical engineering perspective.
Freezing can generate high pressure when water is constrained to prevent expansion. This phenomenon can also affect the physical and mechanical properties of rocks. Taber, (1929) and Taber, (1930) mentioned that under atmospheric pressure, when a definite amount of water is cooled, it freezes at 0°C with an expansion in volume at about 10%. Also, in soils, Beskow, (1991) stated that an increase in load pressure leads to soil consolidation, resulting in the squeezing out of water. When the pressure decreases, the soil tends to expand and under these conditions, there is potential for the soil to suck the water required for the volume increase (Black and Hardenberg, 1991). Tarefder and Ahmad (2015) reported that when water enters the road surface, under the intense action of the wheel load, it causes a higher pressure concentration at the weak joint between the aggregate and the asphalt binder, which accelerates the damage process and leads to permanent deformation.
Based on the analysis made, it is recognized that freeze-thaw cycles (temperature variations) and pressure control rock heave and settlement in the foundation when little or no water-ice phase change is involved. Also, the volumetric expansion of frozen water (ice) and the melting of pore ice cause heave in wet geomaterials, which in turn affect the concrete structure and underground space engineering.
Various types of natural soil and rock masses exhibit a volume expansion behavior. The natural expansive minerals can be divided into two types: i) clay minerals, mainly montmorillonite, illite, or kaolin. Montmorillonite clay will expand significantly as the volume increases, while illite and kaolin clay will have limited expansion. The typical expansive geomaterials which contain montmorillonite are mud rock and bentonite, etc. ii) The other one is the sulphate minerals such as gypsum, anhydrite, calcium thenardite, and anhydrous thenardite. These two types of expansive minerals expand because of the reaction with water.
Expansive clay minerals are prevalent geomaterials that exist in various regions worldwide, including both humid and arid/semi-arid regions, with their primary mineral constituent being dispersed layered silicate (Cherif et al., 2018). They are heterogeneous in nature and their chemical composition depends on other elements, not only the swelling minerals. The expansion behavior of clay minerals can cause significant damage to geotechnical engineering projects. According to research, the damages associated with expansive clays exceed the average annual damages from floods, hurricanes, earthquakes, and tornados (Jones and Holtz, 1973; Chen, 1975; Simmons, 1991; Jones and Jefferson, 2012; Hyndman and Hyndman, 2014). Bouassida et al. (2022) stated that cracked foundations, pavements, floors, and basement walls represent common forms of damage induced by expansive clay minerals. Chen (1975) stated that many floor slabs constructed in an expansive clay area crack and sometimes heave due to improper infrastructure design. Also, there have been reported cases where swelling pressure generated by expansive clay caused lateral deflections of basement walls in foundations (Chen, 1975; Elarabi, 2010)
Experimental research in soil mechanics suggests that moisture content, volume increase, swelling pressure, expansion time, surface area, mineral composition and pore morphology are the factors that contribute to the extensive shrink-swell behavior of most expansive clays. The volume of expansive clay minerals tends to increase as they absorb additional water, which is observed in approximately 90% of clays (Chen, 1975; Jones and Jefferson, 2012). When the moisture content of the clay changes, the swelling pressure will increase and cause volume expansion both in the vertical and horizontal directions. Holtz et al. (2011) experimented on the shrink/swell behavior of expansive clay minerals and observed the following: i) the process of swelling and shrinkage are not entirely reversible, ii) shrinkage induces cracks that upon re-wetting, do not completely close which result in a slight expansion or bulking of the soil, and also allow enhanced access to water for the swelling process. Zhang et al. (2018) studied the expansion characteristics of clay minerals within diverse layers of weathered crust elution-deposited ore bodies and revealed that clay minerals in the humus layer exhibited the highest tendency for swelling. Despite the high swelling potential, if the moisture content of the clay remains unchanged, there will be no volume change; and structures founded on clays with constant moisture content will not be subjected to movement caused by heaving Jones and Jefferson (2012).
Several test results suggest that the swelling pressure increases with respect to decreasing initial water content. However, swelling pressure increases with increasing initial dry density at a controlled initial water content. These trends can either be linear or exponential, according to different research studies (Komine, 2004; Rao et al., 2004; Tu and Vanapalli, 2016). Meanwhile, Tu and Vanapalli (2016) reported that the swelling pressure increase can be attributed to the increased interaction between clay particles due to closer packing. Further, the swelling pressure of clay minerals is primarily affected by their average specific surface area. A larger surface area leads to greater surface forces, which causes significant changes in volume and swelling pressure (Huang et al., 2019; Keskin et al., 2023). Moreover, the duration of expansion and temperature are key factors in determining the linear swelling ratio and initial expansion rate of clay minerals. As the temperature increases, the linear swelling ratio tends to rise which results in a higher initial expansion rate (Chen et al., 2020). Therefore, the swelling of clay minerals is likely to increase with temperature.
On the contrary, it has been recognized that mineralogical composition is an influential factor since different clay minerals have different microstructures in nature (Madsen and Müller-Vonmoos, 1989; Azam, 2003). This variation in microstructure affects the physical and chemical properties of the clay (kaolinite, illite, and montmorillonite), such as its plasticity index, shrink-swell potential, and ion exchange capacity (Shan et al., 2021). Shan et al. (2021) investigated the impact of clay mineral composition on the dynamic properties and structure of artificial marine clay. It was found that marine clays with a high content of montmorillonite showed increased plasticity index (PI) and Atterberg limits. This increase was attributed to the tendency of montmorillonite to more readily adsorb strong and loosely bound water (stern layer) on its surfaces when in a plastic state. Accordingly, understanding the specific mineralogical composition is essential for predicting and managing the behavior of clay in various engineering applications such as soil stabilization and contaminant containment.
Pore morphology substantially influences the swelling behavior of clay minerals (Sarman et al., 1994). experimented on the pore morphology of clay minerals and concluded that the swelling phase did not only relate to the clay mineral type, but also to the pore morphology. It was found that samples with large pore volumes combined with a high percentage of small-sized pores exhibited high swelling potential. The swelling characteristics of various bentonites with different montmorillonite contents with expansion development time, volume expansion rate, water content, and restricted pressure are summarized in Table 2.
Sulfate minerals are abundant and cover about 25% of the constituent of Earth materials and are also found on other planets (Blatt et al., 1980; Ford and Williams, 2007). They occur mainly in the form of gypsum
The formation of ettringite [Ca6Al2(SO4)3(OH)12·26H2O] in soils, is a type of sulfate attack that occurs due to the reaction between sulfate, calcium, and alumina-bearing stages in the presence of water (Figure 2) (Ehwailat et al., 2022). This phenomenon is commonly observed in cement and soils treated with lime, especially in an environment enriched with sulfate (Rajasekaran, 2005; Ehwailat et al., 2022). When calcium and aluminate react with sulfate anions, ettringite can swell up to 250% when form completely and produce insoluble ettringite with increased porosity as the reaction progresses (Zhang et al., 2018; Akula and Little, 2020). According to Ouhadi and Yong (2008), for soil stabilization, the lower solubility of aluminum compounds in a clay fraction is an important source of ettringite formation.
Figure 2. Schematic of ettringite formation: (A) ettringite’s chemical structure, (B) columnar formation of ettringite, (B) existence of aluminum and calcium polyhedral [modified after (Ehwailat et al., 2022)].
The expansion behavior of sulfated rock formations is more significant than clay or marl rocks when involved in near-surface and underground tunnel excavation (Alonso, 2012; Tarragona, 2014). Structural damage attributed to severe heave and settlement in sulfated natural formations has often been associated with tunneling and bridge abutments when periodically exposed to wetting (Yılmaz, 2001; Alonso, 2012). In open discontinuities in sulphated rock formations, gypsum, upon contact with water molecules at a molecular level, expands and undergoes a volume increase of about 62% creating ice crystals (Wittke, 2006; Alonso, 2012). This percentage volume increase causes the crystals to fill the discontinuities, thereby resulting in heaving/expansion behavior in rocks (Figure 3).
Figure 3. Gypsum crystal growth: (A) expansion of gypsum crystal within a rock vein, (B) gypsum crystals after opening with hands [modified after (Tarragona, 2014)]; and (C) Gigantic gypsum crystals in Naica’s Crystal Cave (Chihuahua, Mexico) [modified after (Van Driessche et al., 2019)].
Gypsum is susceptible to rapid dissolution upon contact with water; as a result, its availability causes the spontaneous collapse of individual caverns and migration of voids, eventually leading to subsidence of the overlying ground surface (Yılmaz, 2001). When gypsum acts as a cementing agent, the dissolution of the cement can result in the breakdown of the soil structure (Abduljauwad and Al-Amoudi, 1995; Yılmaz, 2001). The leaching of gypsum and anhydrite creates cavities in the subsoil, which may induce the collapse of light structures without prerequisite warning (Yılmaz, 2001; Azam, 2007).
Zanbak and Arthur (1986) discussed the mechanism of hydration expansion and deformation of anhydrite rock, and the study showed that the molar volume of anhydrite increases after water absorption, and the volume expansion could be 62.6%. Madsen and Müller-Vonmoos, (1989) carried out theoretical and experimental studies on the microscopic scale from the perspective of mineralogy on the interaction between clay particles, anhydrite, and gypsum crystal. Kaiser (1975) studied the growth and expansion of ettringite and anhydrite crystals in solution, and believed that with the change of thermal equilibrium and hydrodynamics, the anhydrite expansion curve showed a logarithmic curve growth form. Barnhoorn et al. (2005) used the residual strain scanning method to study the texture characteristics of anhydrite rocks in the Swiss Bibiani Islands, which is of great significance for underground engineering works, such as mining and tunnels.
Rauh and Thuro (2007) performed powdery swelling tests, thin slice analysis, electron microscope scanning analysis, x-ray diffraction, and specific surface area analysis on gypsum rocks in three different places, and reported that swelling is related to the crystallinity of CaSO4, the larger the crystal size, the smaller the expansion capacity. Azam (2007) studied the geological and engineering issues involved in the expansion and compression of the anhydrite-gypsum transition along the Arabian Gulf coast, some key experimental stages of anhydrite have evaluated their swellability using volume morphological changes, and the gypsum compression index and rebound index were determined. Alonso (2012) conducted softening and swelling experiments of clay-containing anhydrite rocks under the effect of water evaporation and studied the effect of sulphate concentration in solution on swelling.
The analysis suggests that when the sulphate minerals absorb water, the moisture content in gypsum increases, which causes swelling and when the moisture content decreases, it shrinks, leading to structural cracks in civil engineering and other construction works.
The expansion mechanism of frost-heaving soil arises from the interaction between three frost actions: water supply, frost-susceptible soil composition, and temperature as the primary factors (Taber, 1930; Penner, 1959; Black and Hardenberg, 1991; Guthrie et al., 2007; Liu et al., 2017). Heaves are more likely to happen in soil with unique textures, such as loam, silt, and clay, which are moisture-retaining.
Freeze-thaw cycles are common in soils due to fluctuating temperatures. When soil layers freeze, pore water movement is transferred from unfrozen areas to negative regions, which leads to the expansion of the soil’s volume and the development of negative pressure (Beskow, 1991; Black and Hardenberg, 1991; Osokin et al., 2000). In these cold regions, pore water crystallizes and forms ice in the pores, sometimes forming ice lenses (Konrad and Morgentern, 1980; Kozlowski and Nartowska, 2013; Schreiber, 2014). This phenomenon can lead to significant frosts and a decrease in the engineering properties of the soil. In cold climates, the cyclic freezing and thawing processes strongly influence the durability and performance of geo-infrastructures (Tian et al., 2019). The physical and mechanical properties of soils, including expansive soils, change significantly due to freeze-thaw cycles (Yang et al., 2021). Freezing and thawing cycles result in a decrease in the soil’s bulk density and penetration resistance (Unger, 1991). The freeze-thaw process induces uneven stresses within the soil, which create cracks, fractures, and joints in most clay soils, leading to a significant increase in permeability (Eigenbrod, 1996; Fouli et al., 2013; Aksakal et al., 2021). However, coarser soils show a slight change in permeability (Eigenbrod, 1996). As shown in Figure 4A (Schreiber, 2014), when the ice expands within the frozen ground, it induces a soil volume increase of 9%, as proposed by the pore water pressure hypothesis. With high soil moisture content, the surrounding regions will continually provide moisture to the frozen area through capillary action.
Figure 4. Frost heave mechanism in susceptible soils: (A) In-situ freezing, (B) ice crystals’ expansion, and (C) continuous heaving of ice crystals in soils. Modified after (Schreiber, 2014).
This process subsequently contributes to additional ice volume expansion, ultimately leading to frost heaving at the base of the soil. (Guthrie et al., 2007; Liu et al., 2017). The expansion of the frozen water (ice lens) within the soil will exert upward pressure from the penetration limit and induce deformation, which in turn lifts the in-situ frozen soil (Figure 4B) (Schreiber, 2014; Wang and Zhou, 2018). As the temperature gradually decreases over an extended period at a point where the water supply to the lens stops, the frost front drops down until it encounters the saturated soil and creates another ice lens, which creates consecutive volume expansion within the soil (Figure 5) (Schreiber, 2014). The process can continue and cause vulnerability to the infrastructures in the affected area. Consequently, (Alonso et al., 1987; Alonso et al., 1990; Alonso et al., 1999), Adem and Vanapalli (2015), and Lu et al. (2018) and reported the expansion mechanism in expansive soil during freezing at different degrees of saturation in porous materials. Figures 5A,B represent particle types and arrangements in unfrozen expanded clay at higher and lower saturations, respectively.
Figure 5. Schematic diagram of expansive soil freezing showing freezing expansion from (A) lower saturation to (C) higher saturation; and freezing shrinkage from (B) higher saturation to (D) lower saturation. Modified after (Lu et al., 2018).
Clay particles form aggregates that control macropore space, while elementary clay particles regulate the micropore distribution within the aggregates, creating dual porosity. When soil with higher saturation freezes (Figure 5C), the pores between aggregates expand due to the growth of ice lenses, resulting in the compression and rearrangement of soil aggregates. Low-temperature suction forces the water within the aggregate out, which causes drying-induced shrinkage (Lu et al., 2018). This compensatory shrinkage is insufficient to balance the expansion of the growing ice, resulting in an overall increase in soil volume. In contrast, under lower saturation conditions (Figure 5D), the volume expansion of water between the aggregate and ice is contained by the air present in the pores.
In rock mechanics, the presence of joints and fissures significantly affects the geological stability of a rock mass, especially in fractured rock mass, where they play a pivotal role. As the strength of rock is impacted by these discontinuities, the properties of jointed rock are particularly susceptible to the influence of ice within these joints during freezing temperatures (Wang and Zhou, 2018). The frost heave of a rock mass is subject to various factors: temperature, rock frost susceptibility, surrounding rock grade, porosity, and external water supply conditions (Wang et al., 2016a). Joint water in its free state condenses by undergoing a phase change to ice due to negative temperature, exhibiting an expansion coefficient of 9% (Wang et al., 2016a). The water freezing within rock joints initiates a volumetric expansion of the joint filling. This expansion is constrained by the surrounding rock mass, leading to an increase in frost-heaving pressure as a result of the phase transition. If this pressure exceeds the rock mass strength, the jointed rock will fracture and break apart as shown in Figure 6. Given a homogeneous rock medium, the frost heaving ratio is the relationship between volume increment and the original rock volume. This ratio is affected by the 9% volume phase transition process, influencing the rate of frost heave as shown in Eq. 1 (Wang et al., 2016a):
where
Figure 6. Conceptual volume expansion of ice in a fractured rock mass (A) freeze-thaw cracking process of fractured rock mass [modified after (Chang et al., 2022)], (B) Frost wedging causing the detachment of blocks from the bedrock [modified after (Geocache, 2023)].
Additionally, when a rock is exposed to negative temperatures and freezing conditions for a long time, the deformation and intensity properties of the fractured rock mass change greatly. Such occurrences pose threats to constructions such as tunnels, shafts, storage caves, and other infrastructure (Luo et al., 2015; Wang and Zhou, 2018)
In contrast, the above analysis shows that the frost heaving in geomaterials does not promote water flow, causing high hydraulic conductivity. As geomaterials freeze, their porosity decreases and restricts capillary flow. This phenomenon can prevent water ingress from forming ice lenses that cause volume expansion of the geomaterials, resulting in an uplift at the surface. On the contrary, the freeze-thaw process of these natural expansive materials makes it difficult to control water movement in the underground support environment.
The expansion of the interlayer space in clay minerals is due to the hydration energy forces associated with the interaction of the particles (Karpiński and Szkodo, 2015). Clay mineral expansion (montmorillonite) is generally considered to be mainly intergranular and lattice expansion (Figure 7).
Figure 7. Schematic illustration of expansion mechanism of montmorillonite minerals: (A) Intergranular expansion, (B) Lattice expansion. Modified from (Tuller and Or, 2003).
The intergranular expansion is mainly caused by the absorption of water in the aqueous medium by the surface of the clay particles under the effect of electrostatic attraction, which results in the expansion of the soil or rock due to the increase of the thickness of the combined water film (Figure 7A). The expansion of the crystal layer (lattice) occurs when there is a change in the expansive mineral under the action of water or in a humid environment. Water enters the mineral either by becoming an inherent part of the mineral composition or by occupying the spaces within its crystal lattice structure (Tuller and Or, 2003), which causes the mineral volume to expand significantly (Figure 7B). This phenomenon makes it difficult to control water movement in foundations and underground support environments.
The mechanism that leads to the expansion of sulphate minerals is primarily attributed to the evaporation-based and gypsum
The evaporation-based mechanism requires a boundary that interacts with an atmosphere of lower relative humidity than the water potential in the soil or rock. Alternatively, the rate of evaporation and the gypsum solubility also affect this mechanism. The evaporation rate controls moisture removal, while the limited solubility of gypsum restricts the amount that can be dissolved. Nonetheless, the extent of precipitation is constrained by the combined effects of low solubility and controlled evaporation rates. The limited precipitation primarily occurs on or near the evaporation surface, rather than within the volume of the geomaterial and, therefore, initiates a small mass of precipitated gypsum (Tarragona, 2014; Butscher et al., 2015).
Gypsum precipitation through an aqueous solution with anhydrite can lead to larger volumes of gypsum formation, posing greater engineering risks compared to evaporation-based mechanisms (Alonso, 2012; Tarragona, 2014; Butscher et al., 2015). For instance, since natural calcium sulfate-rich water is supersaturated and anhydrite has higher solubility compared to gypsum at temperatures below 56°C, the water in contact with anhydritic claystone at the active layer will dissolve anhydrite and subsequently lead to the precipitation of gypsum (Kontrec et al., 2002; Alonso, 2012; Tarragona, 2014). Afterward, the water becomes supersaturated with respect to gypsum, which causes the excess dissolved calcium sulphate to precipitate as gypsum crystals.
In practical terms, the excess hydrated gypsum can be transported in an aqueous solution or precipitate within available voids in rocks. This action can exert pressure on the rock mass and push it apart, while potentially triggering the expansion of discontinuities and inducing swelling strains (Figure 8) (Alonso, 2012; Tarragona, 2014; Butscher et al., 2015). As presented in Figure 8, the analysis shows that hydrated gypsum can be transported in an aqueous solution and fill facture spaces in the rock mass, making it difficult to control water movement in the geo-infrastructural environment.
Figure 8. Conceptual model for gypsum precipitation. Source: (Tarragona, 2014).
The primary mechanism of freeze-thaw damage in cemented structures is described by both the hydraulic pressure theory and the osmotic pressure theory, and both mechanisms are attributed to concrete deterioration (Powers, 1949; Powers, 1975; Hudec, 1991; Pigeon et al., 1996; Valenza and Scherer, 2007; Zeng et al., 2010; Dabas et al., 2021; Guo et al., 2022).
According to Powers and Darcy’s law, the hydraulic pressure theory explains the effects of low temperatures on concrete (Powers, 1949; Powers, 1975). When concrete is exposed to low temperatures, the outer layer of the concrete freezes first. This freezing causes the liquid water within the concrete to migrate through capillary pores due to the volume differences between ice and liquid water. Precisely, ice occupies a greater volume than liquid water, creating a differential pressure that drives the migration (Valenza and Scherer, 2007; Dabas et al., 2021; Guo et al., 2022). As the temperature continues to drop, the volume of ice within the concrete increases. This increase in ice volume compresses the remaining liquid water, generating significant compressive stress in the pores of the concrete. Simultaneously, ice expansion induces tensile stress in the concrete matrix (Guo et al., 2022) (Figure 9A). If the tensile stress exceeds the ultimate tensile strength of the concrete, microcracks begin to generate (Zeng et al., 2010). These microcracks generated can compromise the structural integrity of the concrete which can lead to long-term durability issues. In spite of the knowledge addressed by the hydraulic pressure theory, it does not fully explain certain phenomena. For instance, it does not account for the expansion observed in ordinary concrete during freezing or the behavior of non-expansive liquids when they freeze (Rønning, 2001; Yu et al., 2017).
Figure 9. Hydrostatic pressure and osmotic pressure mode: (A) Hydrostatic pressure principle (Zeng et al., 2010), (B) Osmotic pressure model. Modified after (Sun et al., 2019; Guo et al., 2022).
Studies have discussed the osmotic pressure theory, which involves specific salt ions in concrete (Powers, 1949; Powers et al., 1953; Powers, 1975; Hudec, 1991; Valenza and Scherer, 2007). According to this theory, the complex pore structure of concrete results in varied migration rates of ions and water which leads to the development of osmotic pressure and interior damage. When unfrozen water transitions to ice, osmotic pressure is generated because the vapor pressure of liquid water becomes higher than that of ice (Figure 9B). This pressure is directly related to the concentration of the solution; as the concentration increases, so does the osmotic pressure. Conversely, the amount of ice formation has an inverse relationship with osmotic pressure, with the pressure value being highest at a specific concentration of the solution (Washburn, 1921; Guo et al., 2022). Basically, osmotic pressure theory emphasizes that the migration and distribution of salt ions and water within the porous structure of concrete can generate significant internal pressure during freeze-thaw cycles. This can cause damage such as microcracks, especially in areas where the solution concentration is at maximum coupling values (Figure 9B).
To mitigate cracking in concrete due to freeze-thaw cycles, an effective method will be to reduce hydrostatic pressure by decreasing the spacing between pores. This may be accomplished through the use of air-entraining agents, which introduce tiny air bubbles into the concrete mix. These air bubbles create additional space in the concrete that can accommodate the expanding ice, thereby reducing the overall pressure and preventing damage. For instance, in regular concrete, the pressure exerted by the freezing water causes the concrete to expand in volume. Nevertheless, in air-entrained concrete, the presence of micro-pores created by the air-entraining agents allows the ice to form within these spaces, leading to a contraction rather than expansion (Sun and Scherer, 2010).
Concrete deterioration occurs due to sulfate ions reacting with hydrated cement composites in pore solution, leading to expansion and corrosion in geo-infrastructure (Figure 10), and this reaction forms expansion phases like gypsum and ettringite, as well as corrosion types such as calcium alumina, magnesium sulfate complex, and carbon-sulfur calcium silica stone corrosion (Al-Dulaijan et al., 2003; Mamun and Bindiganavile, 2011; Min et al., 2019; Othman et al., 2020; Zhang et al., 2021; Tian et al., 2023). Sulfate attack in concrete is affected by the availability of sulfate ions, moisture content, temperature fluctuations, and exposure duration.
Figure 10. Sulfate attack in cemented structures: (A) Macro cracking due to sodium sulfate attack leading to boundary movement [modified after (Yin et al., 2022)], (B) Experimental analysis of sulfate attack [modified after (Liu et al., 2022)].
Basically, sulfate attack in concrete involves physical and chemical reactions (Chen et al., 2020). In the physical reaction, sulfate ions penetrate concrete pores, causing crystallization-induced expansion, leading to internal cracking, which can generate tensile stress and lead to progressive loss of mechanical strength (Tulliani et al., 2002; Oualit and Jauberthie, 2019; Brekailo et al., 2023). The deterioration process originates from two main sources: internal and external attacks. The internal attack results from sulfate within the concrete itself, while external sources include various environmental factors like soils, groundwater, transport fluids, river water, seawater, coastal areas, and acid rain (Neville, 2004; Zhao et al., 2020).
Water serves as a carrier that allows sulfate ions to infiltrate the concrete matrix over time. This infiltration is further heightened by wetting and drying cycles, which can occur naturally or as a result of environmental conditions such as temperature and water table fluctuations. At higher temperatures, water travels at a faster rate along with sulfate ions, which accelerates the chemical reaction between the sulfate and the hydration products of the cement (Ikumi and Segura, 2019; Chen et al., 2020; Zhu et al., 2023). This acceleration causes swellable compounds to form more quickly and increases the rate of deterioration. This reaction results in the formation of expansive compounds such as gypsum and ettringite (Ikumi and Segura, 2019; Chen et al., 2020). These compounds exert pressure within the concrete matrix, causing internal cracking, expansion, and ultimately deterioration of the concrete structure. However, areas with warmer climates may experience a more rapid progression of sulfate attack than colder environments where temperatures are lower and reactions proceed more slowly.
During sulfate attack, particularly assuming sodium sulfate
Gypsum formation
Ettringite formation
The reaction process of these compounds causes concrete expansion, and microcrack formation, and facilitates the entry of harmful ions, accelerating concrete damage.
Frost heaving, a critical geotechnical concern in cold regions, causes structures built on geomaterials to uplift due to freezing temperatures. It alters soil and rock properties by impacting load-bearing capacity, weakening their strength integrity. This poses a serious threat to both surface and underground geo-infrastructures like tunnels, foundations, pipelines, roads, etc., and, however, necessitates a profound understanding of accurate design and mitigation for geotechnical considerations and engineering practices. Table 3 provides a comprehensive summary of the freeze-thaw cycle effects on expansion materials, highlighting their factors and implications in geotechnical engineering.
Table 3. Summary of freeze-thaw effects of natural expansive material and their implications in geotechnical engineering.
Further, in cold weather, especially during negative temperatures, the expansion of ice within fractures acts like a stabilizing agent that binds the fractured rock mass together and enhances its stability as well as any structure adjacent to or within it. This phenomenon is often referred to as frost wedging. In the context of underground openings such as tunnels or mines, the presence of ice filling the fractures can provide additional support and reinforcement to the surrounding rock mass. It helps maintain the structural integrity of the openings and minimize the risk of collapses or instability. In frost-susceptible soils, natural rubber latex (NRL) can significantly improve durability against the adverse effects of wetting and drying (w-d) cycles in cement-stabilized soil, particularly when subjected to cyclic tensile loads (Udomchai et al., 2021; Hoy et al., 2023). The NRL forms films that infiltrate and fill the pores and microcracks within the soil-cement matrix. This infiltration not only strengthens the bond between soil particles and cement but also enhances the overall durability of the geo-infrastructure. By preventing the propagation of microcracks and reducing permeability, these NRL films provide a robust barrier against freeze-thaw cycles (Udomchai et al., 2021; Hoy et al., 2023). It is important to note that this stability enhancement is particularly significant during cold seasons when freezing occurs. However, during warmer seasons or when the ice melts, rock mass maintenance and monitoring are crucial to ensure continued stability.
Natural expansive geomaterials with high shrink-swell potential play a crucial role in geotechnical engineering. These materials exhibit unique properties that can significantly affect infrastructure stability and environmental concerns. Therefore, this section discusses the significance and implications of clay minerals and sulfate minerals in geotechnical engineering, drawing upon relevant research and findings.
Clays have complex structural properties, including their small-sized expansive surface areas, which control the solid soil fraction’s reactivity, act as water reservoirs in soils, and maintain plant-friendly moisture levels in hot and dry environmental conditions (Villiéras et al., 1997). Clay minerals are widely used in geotechnical engineering as impermeable barriers and underground seals due to their low hydraulic conductivity, which effectively retains fluids and prevents the rapid convective transport of various leachates from waste disposal sites (Villiéras et al., 1997; Met and Akgun, 2015). However, swelling clays pose risks in geotechnical engineering projects due to stability concerns (Villiéras et al., 1997). Understanding the properties of clay minerals and their relationship and interaction with water is critical to characterizing clay material applications. One effective approach to addressing these issues is the chemical grouting method, which involves injecting materials such as lime, cement, and silica fume into the soil. This method has been extensively studied and has been shown to improve soil stability, reduce permeability, and enhance load-bearing capacity, making the soil more suitable for supporting structures (Zhao et al., 2014; Puppala and Pedarla, 2017; Al-Gharbawi et al., 2022; Dharini et al., 2023).
Al-Gharbawi et al. (2022) investigated the swell and contraction behavior of expansive soils, primarily composed of montmorillonite, in response to water content changes. The study focused on using 5%, 7%, and 9% lime, cement, and silica fume to stabilize soils. The results showed that stabilization reduced free swell and swelling pressure by about 65% and 76%, respectively. Additionally, grouting with silica fume improved the bearing capacity of footings by 64%–82% for soils treated with 5% and 9% silica fume, respectively. Dharini et al. (2023) investigated the treatment of expansive clay soils using hydrated lime powder and sodium silicate. The study identified 10% lime as the optimal content based on standard proctor and unconfined compressive strength tests. Different proportions of sodium silicate (2%, 4%, 6%, 8%, 10%) were examined while maintaining the optimal lime content. The results from California Bearing Ratio (CBR) tests indicated that soil treated with lime exhibited a CBR value 5.2 times higher than untreated soil, and soil treated with both lime and sodium silicate showed a CBR value of 7.86 times higher than untreated soil. While undisturbed clay barriers have historically shown higher performance in containing chemical waste, identifying thick natural barriers is not always feasible (Met and Akgun, 2015). As a result, compacted clay liners have become essential in municipal and hazardous waste landfill lining systems (Met and Akgun, 2015).
In addition to the use of clays as soil barriers, several clay minerals have useful applications in geo-infrastructure. Bentonite is particularly known for its low permeability, which makes it ideal for certain geotechnical applications. For instance, it is used for its high cation-exchange ability, allowing effective binding and retaining ions making it useful for soil stabilization and geo-infrastructure projects (Klik et al., 2022). When bentonite absorbs water, it hydrates and swells, forming a stable gel structure that enhances its adsorption capacity. The resulting swelling pressure creates a dense structure that fills capillary pores and effectively seals fractures, cracks, and void spaces, thus preventing the migration of radioactive materials over long periods (Komine, 2004; Liu et al., 2019; Klik et al., 2022). Bentonite enhances grout waterproofing and strength, prevents bleeding, and forms a low-permeability hardened material purposely for sealing groundwater inflow and reinforcing fractured rock (Peila et al., 2011; Jinpeng et al., 2018; Benyounes, 2019; Zhou et al., 2020; Abdalqader et al., 2023). Aside from these traditional applications, bentonite have been used as buffer materials in high-level nuclear waste repositories to prevent radioactive waste from leaching into groundwater, and potential escape into the environment (Swedish Nuclear Fuel and Waste Management, 1992; Johnson et al., 1994; JNCDI, 1999; Ito, 2006; Sellin and Leupin, 2013; Tripathy et al., 2015; Liu et al., 2019).
Ensuring consistent quality of bentonite clay is critical, but challenging due to natural variations in its composition. Variations in mineral content, particle size and moisture content can affect its swelling characteristics and performance. This requires strict quality control measures, including periodic testing and characterization of bentonite batches, to ensure consistency and reliability in engineering applications (Kiviranta and Kumpulainen, 2011; Svensson et al., 2017; Magnus et al., 2020). Under changing environmental conditions, bentonite buffer can undergo physical and chemical changes over an extended period that can affect its performance as a sealant or barrier material, especially in geological repositories. Factors such as chemical interactions with surrounding materials, changes in temperature (high temperatures) and humidity, specific pH and salinity conditions, and microbial activity can reduce its performance. In nuclear and geotechnical engineering, long-term durability is an important issue, specifically for applications such as nuclear waste containment, where materials need to maintain their integrity for thousands of years (Kolstad et al., 2004; Laine and Karttunen, 2010; Mazzieri et al., 2017). Table 4 summarizes the geotechnical applications of bentonite, highlighting its high cation exchange capacity and low permeability which make it effective for soil stabilization and geo-infrastructure projects.
Gypsum is extensively used in geotechnical engineering for diverse purposes such as embankment and subgrade backfill material, cement additive, building material, treatment agent for expansive soils, and soft soil reinforcement (Shen et al., 2012; Rashad, 2017; Jiang et al., 2018; Rosales et al., 2020; Pu et al., 2021). Gypsum plays a crucial role in cement manufacturing by preventing or slowing down the rapid setting of cement particles, a phenomenon known as flash setting (Aakriti et al., 2023). Its application as an additive material to stabilize expansive soils (clay soils) has gained significant attention due to its widespread availability, cost-effectiveness, minimal carbon emissions, and its ability to improve water resistance (Toksöz Hozatlıoğlu Yılmaz, 2021; Ma et al., 2022).
Relatively, recycled gypsum’s positive impact on soil stabilization is evident through its influence on various engineering and environmental factors, including compaction characteristics, consistency, strength, deformation, compression, shear, expansion properties, and long-term durability in the past decades (Pu et al., 2021). However, the number of comprehensive studies exploring the efficiency of gypsum in soil stabilization remains relatively limited, with notable contributions from researchers (Yilmaz and Civelekoglu, 2009; Kiliç et al., 2015). In contrast, combining gypsum with other materials offers a wide range of applications in the construction industry. For instance, fly ash-lime-gypsum bricks are used as a substitute for traditional clay bricks in construction, and their application contributes to soil conservation, pollution reduction, and an increase in the consumption of fly ash and gypsum (Jayasudha and Niranjan, 2014).
Table 5 provides a summary of various natural expansive materials, highlighting how their expansion behavior can affect geo-infrastructure. The analysis indicates that clay mineral expansion, influenced by factors such as moisture content and volume increase, leads to shrink-swell behavior and can cause significant damage to foundations. Sulfate mineral expansion results in structural heave in tunnels and bridges. Additionally, sulfate attack in cemented structures causes concrete expansion and deterioration through reactions with hydration products like gypsum and ettringite.
This paper reviews the intrinsic and extrinsic contributing factors and significance of natural expansive materials and their impact on geo-infrastructures. Additionally, this work emphasizes the critical role these materials play in engineering practices, with a particular focus on their involvement in geotechnical-related hazards, such as freeze-thaw cycles, damage, and sulfate attack in underground cemented structures, as well as their applications in geotechnical engineering. The following deductions were made:
i. Frost heaving is prevalent in the Northern Hemisphere, Arctic regions, temperate zones, and high-altitude areas. In these geographical locations, when a significant amount of water within the geomaterials and concrete structures freezes, they can expand by approximately 10% of their original volume. This expansion can enlarge fractures and void spaces in geomaterials and concrete structures during the thawing process, which can contribute to structural instability in these regions.
ii. The freeze-thaw cycle is influenced by variations in temperature and pressure. In rock mechanics, prolonged exposure of rocks to low temperatures (≤0°C) and high temperatures (>0°C) can lead to significant changes in the deformation and strength characteristics of fractured rock masses. Additionally, when pressure decreases, soil tends to expand, while increasing pressure causes soil to shrink. Therefore, it is recognized that freeze-thaw cycles make it difficult to control settlement in geo-infrastructure and rock heave in underground space engineering.
iii. Expansive clay minerals (notably montmorillonite) are widespread in both humid and arid regions which pose significant geotechnical hazards, surpassing damages caused by natural disasters. The expansion mechanism of clay minerals, driven by hydration energy and water absorption into interlayer spaces and crystal lattices is complex which makes it difficult to control water movement in underground excavation and foundation engineering.
iv. Bentonite is being explored as a buffer material in high-level nuclear waste repositories for storage due to its unique swelling and sealing properties to provide a safer containment and isolation of radioactive wastes over extended periods in deep underground environments. Also, bentonite provides a reliable substitute for cementitious materials in geo-infrastructure and can enhance grouted rock stability, waterproofing, and rock strength, in addition to contributing to soil stabilization.
v. Sulfate minerals, particularly gypsum, interact with water, resulting in a theoretical 62% volume increase while transforming into ice crystals. This transformation process can fill rock discontinuities. Moreover, the formation of these ice crystals exerts pressure on the surrounding rock, causing heaving and potentially leading to geo-infrastructural damage.
vi. Sulfate attack in concrete structures and geo-infrastructure involves a chemical reaction between sulfate ions and hydrated compounds, leading to expansion, corrosion, and the formation of gypsum and ettringite phases. The expansion of sulfate minerals is influenced by evaporation-based precipitation and anhydrite-aqueous solution mechanisms, making it crucial to assess risks and implement mitigation strategies for geological and engineered structures.
This review highlights several key areas for future research, particularly in understanding the frost-heaving behavior in rocks, clay minerals, sulfate minerals, and concrete structures, as well as the shrink-swell behavior and expansive mechanisms in geomaterials within geotechnical engineering. Extensive studies have explored frost-heaving mechanisms, considering factors such as ice lens growth, stress-strain behavior, temperature, pressure, and load conditions. Critical elements in this complex process include moisture content, temperature variations, and cyclic freezing-thawing patterns. However, to achieve effective engineering design practices, key research gaps related to expansive materials should be prioritized in future studies:
i. Frost heaving in tunneling and rock engineering is a significant concern due to its impact on rock mechanical integrity, driven by pressure changes during freezing and thawing. However, the mechanisms of frost heaving in deep underground rock openings remain poorly understood and warrant further investigation.
ii. There is a knowledge gap regarding how the expansion, shrinkage, and chemical reactions of sulfate minerals in geomaterials and cemented infrastructures can induce significant reinforcements under varied loading and environmental conditions. The influence of expansive natural materials on geo-infrastructures can be profound, often resulting in foundation cracks, structural tilting, and compromised integrity. Repairing damage caused by expansive natural materials can be costly and time-consuming, necessitating proper site assessment and engineering solutions.
iii. Expansive soils also impact the environment, causing soil erosion, sedimentation in water bodies, and altered groundwater flow patterns. To mitigate these effects, geotechnical engineers should implement strategies such as moisture control, proper drainage, moisture barriers, and foundation designs that accommodate soil movement by using experimental, numerical modeling, and field investigations.
iv. Ongoing research using emerging technologies to better understand natural expansive materials and their interactions with water, which cause shrink/swell behavior should be investigated through advanced soil and rock testing methods, predictive models, numerical modeling, and microstructural characterization. These approaches will enable geotechnical engineers to predict material behavior, identify internal mechanisms, and develop strategies to minimize the impact on geo-infrastructure, resulting in safer and more resilient construction.
v. Strict measures must be tailored to ensure that building codes and regulations, which include comprehensive guidelines for construction in regions susceptible to expansive soils and freeze-thaw cycles, are strictly adhered to. These measures are crucial for safeguarding the safety and structural stability of buildings and infrastructure.
FO: Conceptualization, Formal Analysis, Methodology, Writing–original draft, Writing–review and editing. OK: Project administration, Supervision, Validation, Writing–review and editing.
The author(s) declare that no financial support was received for the research, authorship, and/or publication of this article.
The authors declare that the research was conducted in the absence of any commercial or financial relationships that could be construed as a potential conflict of interest.
All claims expressed in this article are solely those of the authors and do not necessarily represent those of their affiliated organizations, or those of the publisher, the editors and the reviewers. Any product that may be evaluated in this article, or claim that may be made by its manufacturer, is not guaranteed or endorsed by the publisher.
Aakriti, , Maiti, S., Jain, N., and Malik, J. (2023). A comprehensive review of flue gas desulphurized gypsum: production, properties, and applications. Constr. Build. Mater. 393, 131918. doi:10.1016/j.conbuildmat.2023.131918
Abdalqader, A., Fayyad, T., and Sonebi, M. (2023). Optimization of fresh properties, rheological parameters and mechanical performances of grouts containing bentonite. Mater. Today Proc. doi:10.1016/j.matpr.2023.07.194
Abduljauwad, S. N., and Al-Amoudi, O. S. B. (1995). Geotechnical behaviour of saline sabkha soils. Géotechnique 45 (3), 425–445. doi:10.1680/geot.1995.45.3.425
Adem, H. H., and Vanapalli, S. K. (2015). Review of methods for predicting in situ volume change movement of expansive soil over time. J. Rock Mech. Geotechnical Engineering/Journal Rock Mech. Geotechnical Eng. 7 (1), 73–86. doi:10.1016/j.jrmge.2014.11.002
Akhmetiev, M. A. (2015). High-latitude regions of Siberia and Northeast Russia in the Paleogene: stratigraphy, flora, climate, coal accumulation. Stratigr. Geol. Correl. 23 (4), 421–435. doi:10.1134/s0869593815040024
Aksakal, E. L., Angin, I., and Sari, S. (2021). Effects of freeze-thaw cycles on consistency limits of soils amended with diatomite. Soil & Tillage Res. 213, 105144. doi:10.1016/j.still.2021.105144
Akula, P., and Little, D. N. (2020). Coupled thermodynamic and experimental approach to evaluate ettringite formation in a soil stabilized with fluidized bed ash by-product: a case study. Transp. Geotech. 23, 100352. doi:10.1016/j.trgeo.2020.100352
Al-Dulaijan, S. U., Maslehuddin, M., Al-Zahrani, M. M., Sharif, A. M., Shameem, M., and Ibrahim, M. (2003). Sulfate resistance of plain and blended cements exposed to varying concentrations of sodium sulfate. Cem. Concr. Compos. 25 (4-5), 429–437. doi:10.1016/s0958-9465(02)00083-5
Al-Gharbawi, A. S. A., Najemalden, A. M., and Fattah, M. Y. (2022). Expansive soil stabilization with lime, cement, and silica fume. Appl. Sci. 13 (1), 436. doi:10.3390/app13010436
Al-Omari, A., Beck, K., Brunetaud, X., Török, Á., and Al-Mukhtar, M. (2015). Critical degree of saturation: a control factor of freeze–thaw damage of porous limestones at Castle of Chambord, France. Eng. Geol. 185, 71–80. doi:10.1016/j.enggeo.2014.11.018
Alonso, E., Vaunat, J., and Gens, A. (1999). Modelling the mechanical behaviour of expansive clays. Eng. Geol. 54 (1–2), 173–183. doi:10.1016/s0013-7952(99)00079-4
Alonso, E. E. (2012). “Crystal growth and geotechnics,” in Paper presented at the arrigo croce lecture (Rome, Italy).
Alonso, E. E., Gens, A., and Hight, D. W. (1987). “Special problem soils,” in Proceedings of the 9th European conference on soil mechanics and foundation engineering (Dublin), 1087e146.
Alonso, E. E., Gens, A., and Josa, A. (1990). A constitutive model for partially saturated soils. Géotechnique/Geotechnique 40 (3), 405–430. doi:10.1680/geot.1990.40.3.405
Al-samawi, M., and Zhu, J. (2020). Evaluation of the corrosion effects on the performance of composite bridge based on cellular automata and finite element method. Struct. Infrastructure Eng. 18 (5), 630–652. doi:10.1080/15732479.2020.1860095
Al-samawi, M., Zhu, J., and Rong, W. (2023). Application of 3D cellular automata-based analysis to chloride diffusion process in concrete bridges. Structures 47, 500–519. doi:10.1016/j.istruc.2022.11.071
Auer, I., Matulla, C., Böhm, R., Ungersböck, M., Maugeri, M., Nanni, T., et al. (2005). Sensitivity of frost occurrence to temperature variability in the European Alps. Int. J. Climatol. 25 (13), 1749–1766. doi:10.1002/joc.1217
Azam, S. (2003). Influence of mineralogy on swelling and consolidation of soils in eastern Saudi Arabia. Can. Geotechnical J. 40 (5), 964–975. doi:10.1139/t03-047
Azam, S. (2007). Study on the geological and engineering aspects of anhydrite/gypsum transition in the Arabian Gulf coastal deposits. Bull. Eng. Geol. Environ. 66 (2), 177–185. doi:10.1007/s10064-006-0053-2
Aziz, M., Saleem, M., and Irfan, M. (2015). Engineering behavior of expansive soils treated with rice husk ash. Geomechanics Eng. 8 (2), 173–186. doi:10.12989/gae.2015.8.2.173
Baladi, G., and Rajaei, P. (2015). “Predictive modeling of freezing and thawing of frost-susceptible soils,” in Michigan state university. Dept. Of civil and environmental engineering.
Barker, J., and Thomas, H. (2013). Geotechnical engineering in cold regions. ISCORD ISCORD Plan. Sustain. Cold Regions. doi:10.1061/9780784412978.020
Barnhoorn, A., Bystricky, M., Kunze, K., Burlini, L., and Burg, J.-P. (2005). Strain localisation in bimineralic rocks: experimental deformation of synthetic calcite–anhydrite aggregates. Earth Planet. Sci. Lett. 240 (3-4), 748–763. doi:10.1016/j.epsl.2005.09.014
Basma, A. A., Al-Homoud, A. S., Husein Malkawi, A. I., and Al-Bashabsheh, M. A. (1996). Swelling-shrinkage behavior of natural expansive clays. Appl. Clay Sci. 11 (2-4), 211–227. doi:10.1016/s0169-1317(96)00009-9
Benyounes, K. (2019). Rheological behavior of cement-based grout with Algerian bentonite. SN Appl. Sciences/SN Appl. Sci. 1 (9), 1037. doi:10.1007/s42452-019-1089-9
Beskow, G. (1991). Soil freezing and frost heaving with special application to roads and railroad. Cold Regions Res. Eng., 37–157.
Bird, T. (2017). Nordic action on climate change. Copenhagen, Denmark: Nordic Council of Ministers. doi:10.6027/anp2017-766
Biskaborn, B. K., Smith, S. L., Noetzli, J., Matthes, H., Vieira, G., Streletskiy, D. A., et al. (2019). Permafrost is warming at a global scale. Nat. Commun. 10 (1), 264. doi:10.1038/s41467-018-08240-4
Black, P. B., and Hardenberg, M. J. (1991). Historical perspectives in frost heave research. The early works of S. Taber and G. Beskow. Hanover, NH, United States: Defense Technical Information Center, 1–167.
Blatt, H., Middleton, G. V., and Murray, R. C. (1980). Origin of sedimentary rocks. 2nd Ed. New Jersey: Prentice-Hall, 634.
Bouassida, M., Manigniavy, S. A., Azaiez, D., and Bouassida, Y. (2022). New approach for characterization and mitigation of the swelling phenomenon. Front. Built Environ. 8. doi:10.3389/fbuil.2022.836277
Bouyoucos, G. (1920). Degree of temperature to which soils can be cooled without freezing. J. Agric. Res. 20 (4), 267–269. doi:10.1175/1520-0493(1920)48%3C718a:dottws%3E2.0.co;2
Brekailo, F., Pereira, E., and Medeiros-Junior, R. A. (2023). Migration test as an accelerated methodology in concrete for evaluating sulfate attack by Na2SO4 and MgSO4. Constr. Build. Mater. 388, 131681. doi:10.1016/j.conbuildmat.2023.131681
Brimblecombe, P., Grossi, C. M., and Harris, I. (2010). Climate change critical to cultural heritage. Surviv. Sustain., 195–205. doi:10.1007/978-3-540-95991-5_20
Brown, J., Ferrians, O. J., Heginbottom, J. A., and Melnikov, E. S. (1998). “Circum-Arctic map of permafrost and ground-ice conditions,” in National snow and ice data center/world data center for glaciology digital media (Boulder, CO: U.S. Geological Survey). doi:10.3133/cp45
Butscher, C., Mutschler, T., and Blum, P. (2015). Swelling of clay-sulfate rocks: a review of processes and controls. Rock Mech. Rock Eng. 49 (4), 1533–1549. doi:10.1007/s00603-015-0827-6
Chang, Z., Zhang, W., Zhao, G., Dong, F., and Geng, X. (2022). Aging stability analysis of slope considering cumulative effect of freeze–thaw damage—a case study. Minerals 12 (5), 598. doi:10.3390/min12050598
Chen, F. H. (1975). “Foundations on expansive soils,” in Internet archive (Amsterdam; New York: Elsevier Scientific Pub. Co). Available at: https://archive.org/details/foundationsonexp0000chen.
Chen, Y., Liu, P., and Yu, Z. (2020a). Study on degradation of macro performances and micro structure of concrete attacked by sulfate under artificial simulated environment. Constr. Build. Mater. 260, 119951. doi:10.1016/j.conbuildmat.2020.119951
Chen, Z., Zhang, Z., Liu, D., Chi, X.-W., Chen, W., and Chi, R. (2020b). Swelling of clay minerals during the leaching process of weathered crust elution-deposited rare earth ores by magnesium salts. Powder Technol. 367, 889–900. doi:10.1016/j.powtec.2020.04.008
Cherif, M. M., Amal, M., and Ramdane, B. (2018). Effect of swelling mineral on geotechnical characteristics of clay soil. MATEC Web Conf. 149, 02067. doi:10.1051/matecconf/201814902067
Christiansen, H. H., Etzelmüller, B., Isaksen, K., Juliussen, H., Farbrot, H., Humlum, O., et al. (2010). The thermal state of permafrost in the nordic area during the international polar year 2007-2009. Permafr. Periglac. Process. 21 (2), 156–181. doi:10.1002/ppp.687
Dabas, M., Martín-Pérez, B., and Husham, A. (2021). Combined effects of freeze-thaw and corrosion on performance of RC structures: state-of-the-art review. J. Perform. Constr. Facil. 35 (5). doi:10.1061/(asce)cf.1943-5509.0001637
Dagli, D., Zeinali, A., Gren, P., and Laue, J. (2018). Image analyses of frost heave mechanisms based on freezing tests with free access to water. Cold Regions Sci. Technol. 146, 187–198. doi:10.1016/j.coldregions.2017.10.019
DeBeer, C. M., Wheater, H. S., Pomeroy, J. W., Barr, A. G., Baltzer, J. L., Johnstone, J. F., et al. (2021). Summary and synthesis of Changing Cold Regions Network (CCRN) research in the interior of western Canada – Part 2: future change in cryosphere, vegetation, and hydrology. Hydrology Earth Syst. Sci. 25 (4), 1849–1882. doi:10.5194/hess-25-1849-2021
De Gennes, P. G. (1985). Wetting: statics and dynamics. Rev. Mod. Phys. 57 (3), 827–863. doi:10.1103/revmodphys.57.827
Denny, C. S. (1951). Pleistocene frost action near the border of the Wisconsin drift in Pennsylvania. Available at: https://kb.osu.edu/bitstream/handle/1811/3830/1/V51N03_116.pdf.
Dharini, V., Balamaheswari, M., and Presentia, A. N. (2023). Enhancing the strength of expansive clayey soil using lime as soil stabilizing agent along with sodium silicate as grouting chemical. Mater. Today Proc. doi:10.1016/j.matpr.2023.05.156
Dimri, A. P., and Dash, S. K. (2011). Wintertime climatic trends in the western Himalayas. Clim. Change 111 (3-4), 775–800. doi:10.1007/s10584-011-0201-y
Donald, L. J., and Hansen, K. L. (1974). The effects of frost-heaving on objects in soils. Plains Anthropol. 19 (64), 81–98. doi:10.1080/2052546.1974.11908691
Ehwailat, K. I. A., Ismail, M. a. M., and Ezreig, A. M. A. (2022). Ettringite Formation and stabilization methods of sulfate-bearing soil: a state-of-the-art review. Indian Geotechnical J. 52 (4), 927–941. doi:10.1007/s40098-022-00619-x
Eigenbrod, K. D. (1996). Effects of cyclic freezing and thawing on volume changes and permeabilities of soft fine-gained soils. Can. Geotechnical J. 33 (4), 529–537. doi:10.1139/t96-079-301
Elarabi, H. (2010). “Damage mechanism of expansive soils,” in Proceeding of the 2nd international conference on geotechnical engineering ICGE’10 (Tunisia), 125–131.
Feng, F., Chen, S., Zhao, X., Li, D., Wang, X., and Cui, J. (2022). Effects of external dynamic disturbances and structural plane on rock fracturing around deep underground cavern. Int. J. Coal Sci. Technology/International J. Coal Sci. Technol. 9 (1), 15. doi:10.1007/s40789-022-00487-z
Fontboté, L., Kouzmanov, K., Chiaradia, M., and Pokrovski, G. S. (2017). Sulfide minerals in hydrothermal deposits. Elements 13 (2), 97–103. doi:10.2113/gselements.13.2.97
Ford, D., and Williams, P. (2007). Karst hydrogeology and geomorphology. John Wiley & Sons Ltd. doi:10.1002/9781118684986
Fouli, Y., Cade-Menun, B. J., and Cutforth, H. W. (2013). Freeze–thaw cycles and soil water content effects on infiltration rate of three Saskatchewan soils. Can. J. Soil Science/Canadian J. Soil Sci. 93 (4), 485–496. doi:10.4141/cjss2012-060
Geocache (2023). Geocaching - the official global GPS cache hunt site. Available at: https://www.geocaching.com/geocache/GC43QP8 (Accessed June 8, 2024).
Gruber, S. (2012). Derivation and analysis of a high-resolution estimate of global permafrost zonation. Cryosphere 6 (1), 221–233. doi:10.5194/tc-6-221-2012
Guo, J., Sun, W., Xu, Y., Lin, W., and Jing, W. (2022). Damage mechanism and modeling of concrete in freeze–thaw cycles: a review. Buildings 12 (9), 1317. doi:10.3390/buildings12091317
Guthrie, W. S., Lay, R. D., and Birdsall, A. J. (2007). Effect of reduced cement contents on frost heave of silty soil: laboratory testing and numerical modeling. Washington, DC, USA: Transportation Research Board 86th Annual Meeting Transportation Research Board, Transportation Research Board.
Hauer, F. R., Baron, J. S., Campbell, D. H., Fausch, K. D., Hostetler, S. W., Leavesley, G. H., et al. (1997). Assessment of climate change and freshwater ecosystems of the rocky mountains, USA and Canada. Hydrol. Process. 11 (8), 903–924. doi:10.1002/(sici)1099-1085(19970630)11:8<903::aid-hyp511>3.0.co;2-7
Holtz, R. D., Kovacs, W. D., and Sheahan, T. C. (2011). An introduction to geotechnical engineering. 2nd Edition. London, England: Pearson, 853.
Hoy, M., Tran, N. Q., Suddeepong, A., Horpibulsuk, S., Buritatum, A., Yaowarat, T., et al. (2023). Wetting-drying durability performance of cement-stabilized recycled materials and lateritic soil using natural rubber latex. Constr. Build. Mater. 403, 133108. doi:10.1016/j.conbuildmat.2023.133108
Huang, C., Wang, X., Zhou, H., and Liang, Y. (2019). Factors affecting the swelling-compression characteristics of clays in yichang, China. Adv. Civ. Eng. 2019, 1–13. doi:10.1155/2019/6568208
Huang, S., Ye, Y., Cui, X., Cheng, A., and Li, G. (2020). Theoretical and experimental study of the frost heaving characteristics of the saturated sandstone under low temperature. Cold Regions Sci. Technol. 174, 103036. doi:10.1016/j.coldregions.2020.103036
Hudec, P. (1991). “Freezing or osmosis as deterioration mechanism of concrete and aggregate,” in Proc., 2nd Canadian/Japanese workshop on low temperature effects on concrete, 1–2.
Huixin, Z., Zhiqin, W., and Zhaoyu, L. (2012). Experimental study on frost heave of silty clay in seasonally frost soil regions. Procedia Eng. 28, 282–286. doi:10.1016/j.proeng.2012.01.720
Hyndman, D. W., and Hyndman, D. W. (2014). “Natural hazards & disasters,” in Google books (California: Brooks/Cole, Cengage Learning).
Ikumi, T., and Segura, I. (2019). Numerical assessment of external sulfate attack in concrete structures. A review. Cem. Concr. Res. 121, 91–105. doi:10.1016/j.cemconres.2019.04.010
Isarin, R. F. B. (1997). Permafrost distribution and temperatures in europe during the younger dryas. Permafr. Periglac. Process. 8 (3), 313–333. doi:10.1002/(sici)1099-1530(199709)8:3<313::aid-ppp255>3.0.co;2-e
Ito, H. (2006). Compaction properties of granular bentonites. Appl. Clay Sci. 31 (1–2), 47–55. doi:10.1016/j.clay.2005.08.005
Ives, J. D., and Fahey, B. D. (1971). Permafrost Occurrence in the Front Range, Colorado Rocky Mountains, U.S.A. J. Glaciol. 10 (58), 105–111. doi:10.3189/s0022143000013034
Jaesche, P., Veit, H., and Huwe, B. (2003). Snow cover and soil moisture controls on solifluction in an area of seasonal frost, eastern Alps. Permafr. Periglac. Process. 14 (4), 399–410. doi:10.1002/ppp.471
Jalal, F. E., Xu, Y., Jamhiri, B., and Memon, S. A. (2020). On the Recent Trends in Expansive Soil Stabilization Using Calcium-Based Stabilizer Materials (CSMs): A Comprehensive Review. Adv. Mater. Sci. Eng. 2020, 1–23. doi:10.1155/2020/1510969
Jayasudha, R. K., and Niranjan, P. S. (2014). “Properties of FaL-G hollow masonry blocks,” in International journal of research in engineering and Technology, 415–420. Available at: https://ijret.org/volumes/2014v03/i01/IJRET20140301072.pdf.
Jiang, G., Wu, A., Wang, Y., and Lan, W. (2018). Low cost and high efficiency utilization of hemihydrate phosphogypsum: Used as binder to prepare filling material. Constr. Build. Mater. 167, 263–270. doi:10.1016/j.conbuildmat.2018.02.022
Jiang, H., Zhai, D., Shi, K., and Xiang, P. (2023). Analysis of Bearing Safety and Influencing Factors of Supporting Structures of Hydraulic Tunnels in Cold Regions Based on Frost Heave. Buildings 13 (2), 544. doi:10.3390/buildings13020544
Jinpeng, Z., Limin, L., Futao, Z., and Junzhi, C. (2018). Development and application of new composite grouting material for sealing groundwater inflow and reinforcing wall rock in deep mine. Sci. Rep. 8 (1), 5642. doi:10.1038/s41598-018-23995-y
JNCDI (1999). H12: Project to Establish the Scientific and Technical Basis for HLW Disposal in Japan. Supporting Report 2, Repository Design and Engineering Technology. JNC TN1410 2000-003, D38–D42. Available at: https://jopss.jaea.go.jp/pdfdata/JNC-TN1410-2000-003.pdf.
Joanna, J. (2012). Crystallization, Alternation and Recrystallization of Sulphates Advances in Crystallization Processes. InTech. doi:10.5772/37409
Johnson, L., Tait, J., Shoesmith, D., Crosthwaite, J., and Gray, M. (1994). The disposal of Canada’s nuclear fuel waste: engineered barriers alternatives. Ottawa, Ont: Atomic Energy of Canada Limited. Available at: https://inis.iaea.org/collection/NCLCollectionStore/_Public/43/103/43103480.pdf?r=1.
Jones, D. E., and Holtz, W. G. (1973). Expansive soils—The hidden disaster. Civ. Eng. 43 (8), 49–51. Available at: https://trid.trb.org/view/133235.
Jones, L. D., and Jefferson, I. (2012). Expansive soils. Nora.nerc.ac.UK; ICE manual of geotechnical engineering. New York, NY, United States: ICE Publishing, 413–441. Available at: https://nora.nerc.ac.uk/id/eprint/17002/.
Kaiser, W. (1975). Behaviour of anhydrite after addition of water. Bull. Eng. Geol. Environ. 14 (1), 68–69. doi:10.1007/bf02634732
Karpiński, B., and Szkodo, M. (2015). Clay Minerals – Mineralogy and Phenomenon of Clay Swelling in Oil & Gas Industry. Adv. Mater. Sci. 15 (1), 37–55. doi:10.1515/adms-2015-0006
Kassam, A. H. (1981). Climate, Soil and Land Resources in North Africa and West Asia. Plant Soil 58 (1/3), 1–29. doi:10.1007/978-94-015-0861-2_1
Keskin, İ., Salimi, M., Ateyşen, E. Ö., Kahraman, S., and Vakili, A. H. (2023). Comparative Study of Swelling Pressure in Expansive Soils considering Different Initial Water Contents and BOFS Stabilization. Adv. Civ. Eng. 2023, 1–11. doi:10.1155/2023/4823843
Kiliç, R., Küçükali, Ö., and Ulamiş, K. (2015). Stabilization of high plasticity clay with lime and gypsum (Ankara, Turkey). Bull. Eng. Geol. Environ. 75 (2), 735–744. doi:10.1007/s10064-015-0757-2
Kiviranta, L., and Kumpulainen, S. (2011). Quality control and characterization of bentonite materials. Working Report 2011-84.
Klik, B., Holatko, J., Jaskulska, I., Gusiatin, M. Z., Hammerschmiedt, T., Brtnicky, M., et al. (2022). Bentonite as a Functional Material Enhancing Phytostabilization of Post-Industrial Contaminated Soils with Heavy Metals. Materials 15 (23), 8331. doi:10.3390/ma15238331
Kolstad, D. C., Benson, C. H., and Edil, T. B. (2004). Hydraulic Conductivity and Swell of Nonprehydrated Geosynthetic Clay Liners Permeated with Multispecies Inorganic Solutions. J. Geotechnical Geoenvironmental Eng. 130 (12), 1236–1249. doi:10.1061/(asce)1090-0241(2004)130:12(1236
Komine, H. (2004). Simplified evaluation for swelling characteristics of bentonites. Eng. Geol. 71 (3-4), 265–279. doi:10.1016/s0013-7952(03)00140-6
Komine, H., and Ogata, N. (1999). Experimental Study on Swelling Characteristics of Sand-Bentonite Mixture for Nuclear Waste Disposal. Soils Found. 39 (2), 83–97. doi:10.3208/sandf.39.2_83
Konrad, J.-M., and Morgenstern, N. R. (1980). A mechanistic theory of ice lens formation in fine-grained soils. Can. Geotech. J. 17 (4), 473–486. doi:10.1139/t80-056
Kontrec, J., Kralj, D., and Brečević, L. (2002). Transformation of anhydrous calcium sulphate into calcium sulphate dihydrate in aqueous solutions. J. Cryst. Growth 240 (1-2), 203–211. doi:10.1016/s0022-0248(02)00858-8
Kozlowski, T., and Nartowska, E. (2013). Unfrozen water content in representative bentonites of different origin subjected to cyclic freezing and thawing. Vadose Zone J. 12 (1), 1–11. doi:10.2136/vzj2012.0057
Kumari, N., and Mohan, C. (2021). Basics of clay minerals and their characteristic properties. London, United Kingdom: IntechOpen. Available at: https://www.intechopen.com/chapters/76780.
Laine, H., and Karttunen, P. (2010). “Long-term stability of bentonite,” in A literature review (Posiva: Working Report 2010-53).
Lajurkar, S., Khandeshwar, S. R., Dhoble, R. M., and Bade, R. G. (2013). Experimental Study on Shrink-Swell Behaviour of Expansive Soil. Int. J. Innovative Res. Sci. Eng. Technol. 2 (6), 2085–2090.
Li, S., Shen, Y., Dong, J., Ma, W., Lv, Y., Ren, S., et al. (2022). Freezing Damage to Tunnels in Cold Regions and Weights of Influencing Factors. Sustainability 14 (21), 14637. doi:10.3390/su142114637
Lin, Z., Niu, F., Li, X., Li, A., Liu, M., Luo, J., et al. (2018). Characteristics and controlling factors of frost heave in high-speed railway subgrade, Northwest China. Cold Regions Sci. Technol. 153, 33–44. doi:10.1016/j.coldregions.2018.05.001
Liu, D., Cao, K., Tang, Y., Zhong, A., Jian, Y., Gong, C., et al. (2022). Ultrasonic and X-CT measurement methods for concrete deterioration of segmental lining under wetting-drying cycles and sulfate attack. Measurement 204, 111983. doi:10.1016/j.measurement.2022.111983
Liu, F., Shen, S.-L., Hou, D.-W., Arulrajah, A., and Horpibulsuk, S. (2016). Enhancing behavior of large volume underground concrete structure using expansive agents. Constr. Build. Mater. 114, 49–55. doi:10.1016/j.conbuildmat.2016.03.075
Liu, F., Tang, R., Ma, W., and Yuan, X. (2024). Analysis on frost resistance and pore structure of phase change concrete modified by Nano-SiO2 under freeze-thaw cycles. Measurement 230, 114524. doi:10.1016/j.measurement.2024.114524
Liu, J., Cen, G., and Chen, Y. (2017). Study on frost heaving characteristics of gravel soil pavement structures of airports in Alpine regions. RSC Adv. 7 (40), 24633–24642. doi:10.1039/C7RA02151H
Liu, X., Liao, J., Song, H., Yang, Y., Guan, C., and Zhang, Z. (2019). A Biochar-Based route for environmentally friendly controlled release of nitrogen: Urea-Loaded biochar and bentonite composite. Sci. Rep. 9 (1), 9548. doi:10.1038/s41598-019-46065-3
Loranger, B. (2020). Laboratory investigation of frost susceptibility of crushed rock aggregates and field assessment of frost heave and frost depth. Available at: https://hdl.handle.net/11250/2686021.
Lu, X., Zhang, F., Qin, W., Zheng, H., and Feng, D. (2021). Experimental investigation on frost heave characteristics of saturated clay soil under different stress levels and temperature gradients. Cold Regions Sci. Technol. 192, 103379. doi:10.1016/j.coldregions.2021.103379
Lu, Y., Liu, S., Alonso, E., Wang, L., Xu, L., and Li, Z. (2018). Volume changes and mechanical degradation of a compacted expansive soil under freeze-thaw cycles. Cold Regions Sci. Technol. 157, 206–214. doi:10.1016/j.coldregions.2018.10.008
Luo, X., Jiang, N., Fan, X., Mei, N., and Luo, H. (2015). Effects of freeze–thaw on the determination and application of parameters of slope rock mass in cold regions. Cold Regions Sci. Technol. 110, 32–37. doi:10.1016/j.coldregions.2014.11.002
Ma, C., Chen, G., Shi, J., Zhou, H., Ren, W., and Du, Y. (2022). Improvement mechanism of water resistance and volume stability of magnesium oxychloride cement: A comparison study on the influences of various gypsum. Sci. Total Environ. 829, 154546. doi:10.1016/j.scitotenv.2022.154546
Madsen, F. T., and Müller-Vonmoos, M. (1989). The swelling behaviour of clays. Appl. Clay Sci. 4 (2), 143–156. doi:10.1016/0169-1317(89)90005-7
Magnus, K., Lars-Erik, J., and Peter, E. (2020). Strategy, adaptive design and quality control of bentonite materials for a KBS-3 repository. Technical Report TR-20-03.
Maio, M. D., Lodi, L. P., and Suozzi, E. (2014). Natural occurrence of sulphates in ground and surface waters in aosta plain (Italy). Springer EBooks, 1361–1364. doi:10.1007/978-3-319-09048-1_260
Mamun, M., and Bindiganavile, V. (2011). Sulphate resistance of fibre reinforced cement-based foams. Constr. Build. Mater. 25 (8), 3427–3442. doi:10.1016/j.conbuildmat.2011.03.034
Marchand, J., Odler, I., and Skalny, J. P. (2001). Sulfate attack on concrete. Boca Raton, FL, United States: CRC Press. doi:10.4324/9780203301623
Masaki, Y. (2019). Future risk of frost on apple trees in Japan. Clim. Change 159 (3), 407–422. doi:10.1007/s10584-019-02610-7
Matsuoka, N. (1990). Mechanisms of rock breakdown by frost action: An experimental approach. Cold Regions Sci. Technol. 17 (3), 253–270. doi:10.1016/s0165-232x(05)80005-9
Matsuoka, N. (2001). Microgelivation versus macrogelivation: towards bridging the gap between laboratory and field frost weathering. Permafr. Periglac. Process. 12 (3), 299–313. doi:10.1002/ppp.393
Mazzieri, F., Di Emidio, G., and Pasqualini, E. (2017). Effect of wet-and-dry ageing in seawater on the swelling properties and hydraulic conductivity of two amended bentonites. Appl. Clay Sci. 142, 40–51. doi:10.1016/j.clay.2016.10.031
Met, İ., and Akgün, H. (2015). Geotechnical evaluation of Ankara clay as a compacted clay liner. Environ. Earth Sci. 74 (4), 2991–3006. doi:10.1007/s12665-015-4330-x
Michaud, Y., and Dyke, L. (2008). Mechanism of bedrock frost heave in permafrost regions. Semantic Sch.
Min, H., Sui, L., Xing, F., Tian, H., and Zhou, Y. (2019). An effective transport model of sulfate attack in concrete. Constr. Build. Mater. 216, 365–378. doi:10.1016/j.conbuildmat.2019.04.218
Mu, S., and Ladanyi, B. (1987). Modelling of coupled heat, moisture and stress field in freezing soil. Cold Regions Sci. Technol. 14 (3), 237–246. doi:10.1016/0165-232x(87)90016-4
Müllauer, W., Beddoe, R. E., and Heinz, D. (2013). Sulfate attack expansion mechanisms. Cem. Concr. Res. 52, 208–215. doi:10.1016/j.cemconres.2013.07.005
Muthukumar, M., and Shukla, S. K. (2019). Influence of fibres on volume change attributes of expansive soil blended with lime. Proc. Institution Civ. Eng. - Ground Improv. 172 (1), 37–43. doi:10.1680/jgrim.17.00075
Naumov, A., Sidorova, D., and Goncharov, R. (2020). “Farming on Arctic margins: models of agricultural development in northern regions of Russia, Europe and North America,” in Regional science policy & practice. doi:10.1111/rsp3.12273
Neville, A. (2004). The confused world of sulfate attack on concrete. Cem. Concr. Res. 34 (8), 1275–1296. doi:10.1016/j.cemconres.2004.04.004
Niu, F., Li, A., Luo, J., Lin, Z., Yin, G., Liu, M.-H., et al. (2017). Soil moisture, ground temperatures, and deformation of a high-speed railway embankment in Northeast China. Cold Reg. Sci. Technol. 133, 7–14. doi:10.1016/j.coldregions.2016.10.007
Niu, F., Zheng, H., and Li, A. (2020). The study of frost heave mechanism of high-speed railway foundation by field-monitored data and indoor verification experiment. Acta Geotech. 15 (3), 581–593. doi:10.1007/s11440-018-0740-8
Osokin, N. I., Samoylov, R. S., Sosnovskiy, A. V., Sokratov, S., and Zhidkov, V. A. (2000). Model of the influence of snow cover on soil freezing. Ann. Glaciol. 31, 417–421. doi:10.3189/172756400781820282
Oswell, J. M. (2011). Pipelines in permafrost: geotechnical issues and lessons 12010 R.M. Hardy Address, 63rd Canadian Geotechnical Conference. Can. Geotechnical J. 48 (9), 1412–1431. doi:10.1139/t11-045
Othman, R., Muthusamy, K., Duraisamy, Y., Sulaiman, M. A., Jaya, R. P., Abdul, A., et al. (2020). Evaluation of the sulphate resistance of foamed concrete containing processed spent bleaching earth. Eur. J. Environ. Civ. Eng. 26 (8), 3632–3647. doi:10.1080/19648189.2020.1809526
Oualit, M., and Jauberthie, R. (2019). Corrosion of concrete pipes in a sewer environment – two case studies. WEJ/Water Environ. J. 34 (4), 600–610. doi:10.1111/wej.12488
Ouhadi, V. R., and Yong, R. N. (2008). Ettringite formation and behaviour in clayey soils. Appl. Clay Sci. 42 (1–2), 258–265. doi:10.1016/j.clay.2008.01.009
Panin, G. N., Solomonova, I. V., and Vyruchalkina, T.Yu. (2009). Climatic trends in the middle and high latitudes of the Northern Hemisphere. Water Resour. 36 (6), 718–730. doi:10.1134/s0097807809060116
Peila, D., Borio, L., and Pelizza, S. (2011). The behaviour of a two-component backfilling grout used in a Tunnel-Boring Machine. ACTA GEOTECH. SLOV. 1, 5–15. Available at: http://porto.polito.it/2435575/1/2011-AGS-backfilling.pdf.
Penner, E. (1959). in The mechanism of frost heaving in soils. Editor N. R. C. Ottawa (Ottawa, Canada: Division of Building Research), 1–13.
Phanikumar, B. R., and Singla, R. (2016). Swell-consolidation characteristics of fibre-reinforced expansive soils. Soils Found. 56 (1), 138–143. doi:10.1016/j.sandf.2016.01.011
Pigeon, M., Marchand, J., and Pleau, R. (1996). Frost resistant concrete. Constr. Build. Mater. 10 (5), 339–348. doi:10.1016/0950-0618(95)00067-4
Pooni, J., Giustozzi, F., Robert, D., Setunge, S., and O’Donnell, B. (2019). Durability of enzyme stabilized expansive soil in road pavements subjected to moisture degradation. Transp. Geotech. 21, 100255. doi:10.1016/j.trgeo.2019.100255
Powers, T. (1949). The air requirement of frost-resistant concrete. Proc. Highw. Res. Board, 184–211.
Powers, T., Helmuth, R., and Research, J. (1953). “Theory of Volume Changes in Hardened Port-land-Cement Paste During Freezing,” in Proceedings of the thirty-second annual meeting of the highway research board (Washington, DC, USA), 285–297.
Powers, T. C. (1975). Freezing effects in concrete, 47. Special Publication. American Concrete Institute, 1–12.
Prince, M., Roy, A., Brucker, L., Royer, A., Kim, Y., and Zhao, T. (2018). Northern Hemisphere surface freeze–thaw product from Aquarius L-band radiometers. Earth Syst. Sci. Data 10 (4), 2055–2067. doi:10.5194/essd-10-2055-2018
Pu, S., Zhu, Z., and Huo, W. (2021). Evaluation of engineering properties and environmental effect of recycled gypsum stabilized soil in geotechnical engineering: A comprehensive review. Resour. Conservation Recycl. 174, 105780. doi:10.1016/j.resconrec.2021.105780
Puppala, A. J., and Pedarla, A. (2017). Innovative ground improvement techniques for expansive soils. Innov. Infrastruct. Solutions 2 (1), 24. doi:10.1007/s41062-017-0079-2
Qi, S., and Vanapalli, S. K. (2015). Hydro-mechanical coupling effect on surficial layer stability of unsaturated expansive soil slopes. Comput. Geotechnics 70, 68–82. doi:10.1016/j.compgeo.2015.07.006
Rajasekaran, G. (2005). Sulphate attack and ettringite formation in the lime and cement stabilized marine clays. Ocean. Eng. 32 (8–9), 1133–1159. doi:10.1016/j.oceaneng.2004.08.012
Rao, A. S., Phanikumar, B. R., and Sharma, R. S. (2004). Prediction of swelling characteristics of remoulded and compacted expansive soils using free swell index. Q. J. Eng. Geol. Hydrogeology 37 (3), 217–226. doi:10.1144/1470-9236/03-052
Rashad, A. M. (2017). Phosphogypsum as a construction material. J. Clean. Prod. 166, 732–743. doi:10.1016/j.jclepro.2017.08.049
Rauh, F., and Thuro, K. (2007). “Investigations On the Swelling Behavior of Pure Anhydrites,” in Paper presented at the 1st Canada - U.S. Rock mechanics symposium (Vancouver, Canada).
Rekstad, L. C., Wasmuth, H. H., Ystgaard, B., Stornes, T., and Seternes, A. (2013). Topical negative-pressure therapy for small bowel leakage in a frozen abdomen. J. Trauma Acute Care Surg. 75 (3), 487–491. doi:10.1097/ta.0b013e3182995e6d
Rempel, A. W., Wettlaufer, J. S., and Worster, M. G. (2001). Interfacial Premelting and the Thermomolecular Force: Thermodynamic Buoyancy. Phys. Rev. Lett. 87 (8), 088501. doi:10.1103/physrevlett.87.088501
Rønning, T. (2001). Freeze-thaw resistance of concrete: effect of: curing conditions, moisture exchange and materials. Trondheim, Norway: The Norwegian Institute of Technology. Ph.D. Thesis Thesis.
Rosales, J., Pérez, S. M., Cabrera, M., Gázquez, M. J., Bolivar, J. P., de Brito, J., et al. (2020). Treated phosphogypsum as an alternative set regulator and mineral addition in cement production. J. Clean. Prod. 244, 118752. doi:10.1016/j.jclepro.2019.118752
Samborska, K., Halas, S., and Bottrell, S. H. (2013). Sources and impact of sulphate on groundwaters of Triassic carbonate aquifers, Upper Silesia, Poland. J. Hydrology 486, 136–150. doi:10.1016/j.jhydrol.2013.01.017
Sarman, R., Shakoor, A., and Palmer, D. F. (1994). A multiple regression approach to predict swelling in mudrocks. Environ. Eng. Geoscience xxxi (1), 107–121. doi:10.2113/gseegeosci.xxxi.1.107
Savi, S., Delunel, R., and Schlunegger, F. (2015). Efficiency of frost-cracking processes through space and time: An example from the eastern Italian Alps. Geomorphology 232, 248–260. doi:10.1016/j.geomorph.2015.01.009
Schreiber, A. (2014). Seeding Into Frost Heaves: Leveraging a Natural Soil Disturbance Event. Permaculture Res. Inst. Available at: https://www.permaculturenews.org/2014/06/14/seeding-frost-heaves-leveraging-natural-soil-disturbance-event/#google_vignette (Accessed June 14, 2024).
Schuller, H., and Schweiger, H. F. (2002). Application of a Multilaminate Model to simulation of shear band formation in NATM-tunnelling. Comput. Geotechnics 29 (7), 501–524. doi:10.1016/s0266-352x(02)00013-7
Sellin, P., and Leupin, O. X. (2013). The use of clay as an Engineered Barrier in Radioactive-Waste Management – A review. Clays Clay Minerals 61 (6), 477–498. doi:10.1346/ccmn.2013.0610601
Shan, Y., Wang, X., Cui, J., Mo, H., and Li, Y. (2021). Effects of Clay Mineral Composition on the Dynamic Properties and Fabric of Artificial Marine Clay. J. Mar. Sci. Eng. 9 (11), 1216. doi:10.3390/jmse9111216
Sharifi, N. P., Chen, S., You, Z., Van Dam, T., and Gilbertson, C. (2019). A review on the best practices in concrete pavement design and materials in wet-freeze climates similar to Michigan. J. Traffic Transp. Eng. Engl. Ed. 6 (3), 245–255. doi:10.1016/j.jtte.2018.12.003
Shen, W., Gan, G., Dong, R., Chen, H., Tan, Y., and Zhou, M. (2012). Utilization of solidified phosphogypsum as Portland cement retarder. J. Material Cycles Waste Manag. 14 (3), 228–233. doi:10.1007/s10163-012-0065-x
Shi, D., Li, Y., Gao, X., Li, S., and Xiang, T. (2020). Analysis of internal force and displacement of foundation pit pile anchor supporting structure based on soil frost heaving. IOP Conf. Ser. Earth Environ. Sci. 580 (1), 012028. doi:10.1088/1755-1315/580/1/012028
Simons, K. B. (1991). Limitations of Residential Structures on Expansive Soils. J. Perform. Constr. Facil. 5 (4), 258–270. doi:10.1061/(asce)0887-3828(1991)5:4(258)
Stewart, R. E., Szeto, K. K., Bonsal, B., Hanesiak, J., Bohdan, K., Li, Y., et al. (2019). Summary and synthesis of Changing Cold Regions Network (CCRN) research in the interior of western Canada – Part 1: Projected climate and meteorology. Hydrology Earth Syst. Sci. 23 (8), 3437–3455. doi:10.5194/hess-23-3437-2019
Sun, M., Xin, D., and Zou, C. (2019). Damage evolution and plasticity development of concrete materials subjected to freeze-thaw during the load process. Mech. Mater. (Print) 139, 103192. doi:10.1016/j.mechmat.2019.103192
Sun, Z., and Scherer, G. W. (2010). Effect of air voids on salt scaling and internal freezing. Cem. Concr. Res. 40 (2), 260–270. doi:10.1016/j.cemconres.2009.09.027
Svensson, D., Lundgren, C., Johannesson, L.-E., and Norrfors, K. (2017). Developing strategies for acquisition and control of bentonite for a high level radioactive waste repository. Solna, Sweden: TR, 16–14.
Swedish Nuclear Fuel and Waste Management (1992). “SKB91 final disposal of spent nuclear fuel,” in Importance of the bedrock for safety (Solna, Sweden: SKB Technical Report), 92–20.
Taivainen, O. A. (1963). Preventive measures to reduce frost action on highways in Finland. Oulu, Finland: Faculty of Technology, University of Oulu, 33. Available at: https://trid.trb.org/View/127919.
Tarefder, R. A., and Ahmad, M. (2015). Evaluation of pore structure and its influence on permeability and moisture damage in asphalt concrete. Int. J. Pavement Eng. 18 (3), 274–283. doi:10.1080/10298436.2015.1065995
Tarragona, R. A. (2014). Expansion mechanisms in sulphated rocks and soils. Consorc. Serv. Univ. Cataluña. PhD Thesis.
Thiry, M., Milnes, A. R., and Mohamed, B. B. (2014). Pleistocene cold climate groundwater silicification, Jbel Ghassoul region, Missour Basin, Morocco. J. Geol. Soc. 172 (1), 125–137. doi:10.1144/jgs2014-033
Tian, B., and Cohen, M. D. (2000). Does gypsum formation during sulfate attack on concrete lead to expansion? Cem. Concr. Res. 30 (1), 117–123. doi:10.1016/s0008-8846(99)00211-2
Tian, H., Wei, C., and Tan, L. (2019). Effect of freezing-thawing cycles on the microstructure of soils: A two-dimensional NMR relaxation analysis. Cold Regions Sci. Technol. 158, 106–116. doi:10.1016/j.coldregions.2018.11.014
Tian, W., Jin, L., Fan, T., Li, Q., Liu, W., Zhou, Q., et al. (2023). A multi-phase numerical simulation method for the changing process of expansion products on concrete under sulfate attack. Case Stud. Constr. Mater. 19, e02458. doi:10.1016/j.cscm.2023.e02458
Toksöz Hozatlıoğlu, D., and Yılmaz, I. (2021). Shallow mixing and column performances of lime, fly ash and gypsum on the stabilization of swelling soils. Eng. Geol. 280, 105931. doi:10.1016/j.enggeo.2020.105931
Tripathy, S., Bag, R., and Thomas, H. R. (2015). “Enhanced Isothermal Effect on Swelling Pressure of Compacted MX80 Bentonite,” in Engineering geology for society and territory - volume 6. Editor G. Lollino (Cham: Springer). doi:10.1007/978-3-319-09060-3_96
Tu, H., and Vanapalli, S. K. (2016). Prediction of the variation of swelling pressure and one-dimensional heave of expansive soils with respect to suction using the soil-water retention curve as a tool. Can. Geotechnical J. 53 (8), 1213–1234. doi:10.1139/cgj-2015-0222
Tuller, M., and Or, D. (2003). Hydraulic functions for swelling soils: pore scale considerations. J. Hydrology 272 (1-4), 50–71. doi:10.1016/s0022-1694(02)00254-8
Tulliani, J.-M., Montanaro, L., Negro, A., and Collepardi, M. (2002). Sulfate attack of concrete building foundations induced by sewage waters. Cem. Concr. Res. 32 (6), 843–849. doi:10.1016/s0008-8846(01)00752-9
Tuukka, P., Ganzei, K. S., Lappalainen, H. K., Ksenia, T., Makkonen, R., Räisänen, J., et al. (2020). Research agenda for the Russian Far East and utilization of multi-platform comprehensive environmental observations. Int. J. Digital Earth 14 (3), 311–337. doi:10.1080/17538947.2020.1826589
Udomchai, A., Buritatum, A., Suddeepong, A., Hoy, M., Horpibulsuk, S., Arulrajah, A., et al. (2021). Evaluation of durability against wetting and drying cycles of cement-natural rubber latex stabilised unpaved road under cyclic tensile loading. Int. J. Pavement Eng. 23 (12), 4442–4453. doi:10.1080/10298436.2021.1950719
Unger, P. W. (1991). Overwinter changes in physical properties of No-Tillage soil. Soil Sci. Soc. Am. J. 55 (3), 778–782. doi:10.2136/sssaj1991.03615995005500030024x
Vaitkus, A., Gražulytė, J., Skrodenis, E., and Kravcovas, I. (2016). Design of Frost Resistant Pavement Structure Based on Road Weather Stations (RWSs) Data. Sustainability 8 (12), 1328. doi:10.3390/su8121328
Valenza, J. A., and Scherer, G. W. (2007). A review of salt scaling: II. Mechanisms. Cem. Concr. Res. 37 (7), 1022–1034. doi:10.1016/j.cemconres.2007.03.003
Van Driessche, A. E. S., Stawski, T. M., and Kellermeier, M. (2019). Calcium sulfate precipitation pathways in natural and engineered environments. Chem. Geol. 530, 119274. doi:10.1016/j.chemgeo.2019.119274
Villiéras, F., Michot, L., Cases, J., Berend, I., Bardot, F., François, M., et al. (1997). “Chapter 11. Static and dynamic studies of the energetic surface heterogeneity of clay minerals,” in Studies in surface science and catalysis, 573–623. doi:10.1016/s0167-2991(97)80074-2
Wang, D., Li, Q., and Zhang, W. (2016). The research of frost heaving damage caused by fracture extension length under the freezing conditions. Int. J. Sci. 3 (2), 125–130.
Wang, L., Wu, Z., He, H., Wang, F., Du, H., and Zong, S. (2017). Changes in start, end, and length of frost-free season across Northeast China. Int. J. Climatol. 37, 271–283. doi:10.1002/joc.5002
Wang, P., and Zhou, G. (2018). Frost-heaving pressure in geotechnical engineering materials during freezing process. Int. J. Min. Sci. Technol. 28 (2), 287–296. doi:10.1016/j.ijmst.2017.06.003
Wang, Q., Liu, J., Zhu, X., and Liu, Z. (2016). The experiment study of frost heave characteristics and gray correlation analysis of graded crushed rock. Cold Regions Sci. Technol. 126, 44–50. doi:10.1016/j.coldregions.2016.03.003
Wang, Z., Sigdel, P., and Hu, L. (2019). Chemo-Mechanical Interactions in the Ettringite Induced Expansion of Sulfate-Bearing Soils. Geosciences 9 (9), 375. doi:10.3390/geosciences9090375
Washburn, E. W. (1921). The Dynamics of Capillary Flow. Phys. Rev. 17 (3), 273–283. doi:10.1103/physrev.17.273
Wittke, M. (2006). Design, construction, supervision and long-term behaviour of tunnels in swelling rock. Abingdon, United States: Taylor & Francis EBooks, 211–216. doi:10.1201/9781439833469.ch29
Wu, X., Niu, F., Lin, Z., Luo, J., Zheng, H., and Shao, Z. (2018). Delamination frost heave in embankment of high speed railway in high altitude and seasonal frozen region. Cold Regions Sci. Technol. 153, 25–32. doi:10.1016/j.coldregions.2018.04.017
Wynn, G. (2006). “Canada and Arctic North America: An Environmental History,” in Google books (New York, NY, United States: Bloomsbury Publishing USA).
Xiao, L., Liu, L., Asseng, S., Xia, Y., Tang, L., Liu, B., et al. (2018). Estimating spring frost and its impact on yield across winter wheat in China. Agric. For. Meteorology 260-261, 154–164. doi:10.1016/j.agrformet.2018.06.006
Yang, Z., Lv, J., Shi, W., Jia, C., Wang, C., Hong, Y., et al. (2021). Experimental study of the freeze thaw characteristics of expansive soil slope models with different initial moisture contents. Sci. Rep. 11 (1), 23177. doi:10.1038/s41598-021-02662-9
Yeon, J. H., and Kim, K.-K. (2018). Potential applications of phase change materials to mitigate freeze-thaw deteriorations in concrete pavement. Constr. Build. Mater. 177, 202–209. doi:10.1016/j.conbuildmat.2018.05.113
Yılmaz, I. (2001). Gypsum/anhydrite: some engineering problems. Bull. Eng. Geol. Environ. 60 (3), 227–230. doi:10.1007/s100640000071
Yilmaz, I., and Civelekoglu, B. (2009). Gypsum: An additive for stabilization of swelling clay soils. Appl. Clay Sci. 44 (1-2), 166–172. doi:10.1016/j.clay.2009.01.020
Yin, G.-J., Shan, Z.-Q., Miao, L., Tang, Y.-J., Zuo, X.-B., and Wen, X.-D. (2022). Finite element analysis on the diffusion-reaction-damage behavior in concrete subjected to sodium sulfate attack. Eng. Fail. Anal. 137, 106278. doi:10.1016/j.engfailanal.2022.106278
Yu, H., Ma, H., and Yan, K. (2017). An equation for determining freeze-thaw fatigue damage in concrete and a model for predicting the service life. Constr. Build. Mater. 137, 104–116. doi:10.1016/j.conbuildmat.2017.01.042
Yu, Q., Zhang, C., Dai, Z., Du, C., Soltanian, M. R., and Soltanian, M. (2019). Impact of Tunnel Temperature Variations on Surrounding Rocks in Cold Regions. Period. Polytech. Civ. Eng. doi:10.3311/ppci.13109
Zanbak, C., and Arthur, R. C. (1986). Geochemical and Engineering Aspects of Anhydrite/Gypsum Phase Transitions. Environ. Eng. Geoscience 23 (4), 419–433. doi:10.2113/gseegeosci.xxiii.4.419
Zeng, Q., Li, K., Fen-Chong, T., and Dangla, P. (2010). “A study of the behaviors of cement-based materials subject to freezing,” in International conference on mechanic automation and control engineering. doi:10.1109/mace.2010.5535987
Zhang, C., Chen, W., Mu, S., Šavija, B., and Liu, Q. (2021). Numerical investigation of external sulfate attack and its effect on chloride binding and diffusion in concrete. Constr. Build. Mater. 285, 122806. doi:10.1016/j.conbuildmat.2021.122806
Zhang, X., Powrie, W., and Harkness, R. M. (1999). Numerical modelling of wellbore behaviour in fractured rock masses. J. Petroleum Sci. Eng. 23 (2), 95–115. doi:10.1016/s0920-4105(99)00010-8
Zhang, Y., Xia, C., Zhou, S., Hu, Y., and Zhang, J. (2022). A new sustainable energy based freeze proof method for drainage system in cold-region tunnels: A case study of Tianshan Shengli Tunnel. Case Stud. Therm. Eng. 34, 102020. doi:10.1016/j.csite.2022.102020
Zhang, Z., He, Z., Zhou, F., Zhong, C., Sun, N., and Chi, R. (2018). Swelling of clay minerals in ammonium leaching of weathered crust elution-deposited rare earth ores. Rare Met. 37 (1), 72–78. doi:10.1007/s12598-017-0977-7
Zhao, G., Shi, M., Guo, M., and Fan, H. (2020). Degradation Mechanism of Concrete Subjected to External Sulfate Attack: Comparison of Different Curing Conditions. Materials 13 (14), 3179. doi:10.3390/ma13143179
Zhao, H., Peila, D., Petry, T. M., and Sun, Y.-Z. (2014). Effects of chemical stabilizers on an expansive clay. KSCE J. Civ. Eng. 18 (4), 1009–1017. doi:10.1007/s12205-013-1014-5
Zhou, X., Ren, X., Ye, X., Tao, L., Zeng, Y., and Liu, X. (2021). Temperature field and anti-freezing system for cold-region tunnels through rock with high geotemperatures. Tunn. Undergr. Space Technol. 111, 103843. doi:10.1016/j.tust.2021.103843
Zhou, Y., Wang, G. H., and Yuan, Y. F. (2020). Basic properties and engineering application of Bentonite-Cement-Water glass grouting. KSCE J. Civ. Eng. 24 (9), 2742–2750. doi:10.1007/s12205-020-1928-7
Zhu, J., Al-samawi, M., Yang, Y., and Najm, A. A.-S. (2023). An approach for simulating and evaluating the effect of sulfate attack on the durability of concrete bridges based on a three-dimensional cellular automata model. Eng. Struct. (Online) 291, 116451. doi:10.1016/j.engstruct.2023.116451
Zuo, X.-B., Sun, W., and Yu, C. (2012). Numerical investigation on expansive volume strain in concrete subjected to sulfate attack. Constr. Build. Mater. 36, 404–410. doi:10.1016/j.conbuildmat.2012.05.020
Keywords: geomaterials, expansive clay, frost-heaving, sulfate minerals, soil improvement, geotechnical engineering
Citation: Oppong F and Kolawole O (2024) Reassessment of natural expansive materials and their impact on freeze-thaw cycles in geotechnical engineering: a review. Front. Built Environ. 10:1396542. doi: 10.3389/fbuil.2024.1396542
Received: 05 March 2024; Accepted: 04 July 2024;
Published: 25 July 2024.
Edited by:
Fei Wang, Tarleton State University, United StatesReviewed by:
Menglim Hoy, Suranaree University of Technology, ThailandCopyright © 2024 Oppong and Kolawole. This is an open-access article distributed under the terms of the Creative Commons Attribution License (CC BY). The use, distribution or reproduction in other forums is permitted, provided the original author(s) and the copyright owner(s) are credited and that the original publication in this journal is cited, in accordance with accepted academic practice. No use, distribution or reproduction is permitted which does not comply with these terms.
*Correspondence: Oladoyin Kolawole, b2xhZG95aW4ua29sYXdvbGVAbmppdC5lZHU=
Disclaimer: All claims expressed in this article are solely those of the authors and do not necessarily represent those of their affiliated organizations, or those of the publisher, the editors and the reviewers. Any product that may be evaluated in this article or claim that may be made by its manufacturer is not guaranteed or endorsed by the publisher.
Research integrity at Frontiers
Learn more about the work of our research integrity team to safeguard the quality of each article we publish.