- 1Faculty of Civil Engineering, University of Applied Sciences Konstanz HTWG, Konstanz, Germany
- 2GemeinWerk Ventures GmbH, München, Germany
- 3Faculty of Architecture, KU Leuven, Ghent, Belgium
This paper introduces a transformative “living” hypothesis in architecture and engineering, proposing a paradigm shift from conventional design to regenerative, ecologically interconnected resilient systems. At the heart of our hypothesis is the integration of earth-bound materials and bioreceptive surfaces through metabolic exchanges that can be directly monitored via bioelectricity using advanced computational models and cooperative governance structures. This innovative approach that links the living world with natural materials and digital computing, aims to foster sustainable urban development that dynamically and meaningfully responds to ecological shifts, thereby enhancing social sustainability and environmental resilience. Founded on an active relationship with Earth Based Materials (EBMs) our work operationalises the foundational link between organic life and inorganic matter, e.g., minerals, to establish a dynamic relationship between building materials, and ecological systems drawing on the foundational metabolisms of microbes. To enable this ambitious synthesis, our work builds upon and diverges from traditional foundations by operationalizing actor-network theory, new materialism, and regenerative design principles through the application of bioelectrical microbes to “living” materials and digital twins. We propose a novel resilience framework that not only advocates for a symbiotic relationship between human habitats and natural ecosystems but also outlines practical pathways for the creation of adaptive, self-organizing built environments that are informed by data collection and metabolic feedback loops. These environments are fundamentally regenerative, dynamic, and environmentally responsive in ways that can be understood and engaged by human engineers and designers, transcending current sustainability and resilience targets through a methodology rooted in interdisciplinary collaboration. We address challenges such as regulatory barriers, lack of standardization, and perceptions of inferiority compared to conventional materials, proposing a new standardization framework adaptable to the unique properties of these materials. Our vision is supported by advanced predictive digital modelling techniques and sensors, including the integration of biofilms that generate action potentials, enabling the development of Digital Twins that respond to metabolic signals to enhance sustainability, biodiversity, and ultimately generate environmentally positive socio-economic outcomes. This paper reviews existing methodologies to establish an overview of state-of-the-art developments and offers a clear, actionable plan and recommendations for the realization of regenerative and resilient systems in urban development. It contributes a unique perspective on sustainable urban development, emphasizing the need for a holistic approach, which integrates the foundational metabolism of microbes, assisted by big biological data and artificial intelligences that act in concert to respect both the environment and the intricate dynamics of living systems.
1 Introduction—redefining the built environment through earth based materials
We are reaching global environmental tripping points, and the imperative for a sustainable and adaptive built environment that repairs and supports our living realm has never been more urgent. Conventional construction methods, characterised by rigidity and a disconnection from ecological systems, are increasingly at odds with the environmental and social imperatives of our times. Our transformative hypothesis establishes an approach for the overall enlivening of our living spaces: embracing the principles of resilient regenerative design (Petrovski et al., 2021; Yang et al., 2021; Soares and Puccinelli, 2023) by integrating the tenets of actor-network theory (Latour, 2007) with a new materialist view (Dolphijn, 2021) that are implemented via microbial metabolisms that invoke applications of novel living technology (Armstrong et al., 2017), biobased and natural materials. By operationalising the dynamic aspects that characterise the living world—such as the balance between growth and decay, metabolic cycles, regenerative processes, adaptive responses, reproduction and evolution, and energy transformation—we envision the convergence of technology and matter to perform useful “work” within architectural and engineering contexts (bioreceptivity, circularity, biocompatible by-products etc.), which can be monitored through dynamic feedback loops to generate positive environmental impacts of our buildings and cities, that ultimately produce downstream effects on human health, wellbeing at an individual and societal level (Table 1). The integration of active, living systems into architectural design, establishes the potential for buildings that actively contribute to ecological processes, promoting sustainability and resilience while fostering a deeper connection between humans and the natural world. In other words, our buildings are becoming increasingly lively (Haraway, 1991).
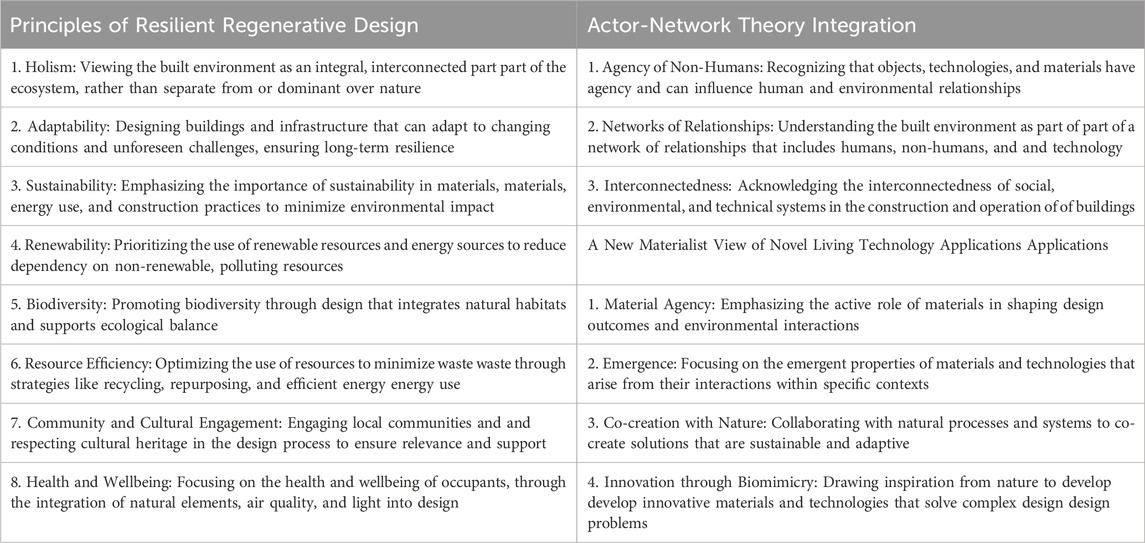
Table 1. Principles of resilient regenerative design (Petrovski, Pauwels, and Gonzalez 2021; Yang 2021; Soares and Puccinelli 2023) and integrating the tenets of actor-network theory with a new materialist view of novel living technology application.
The convergence of these paradigms suggests the built environment that emerges from these synergies is fluid, generative, and life-like, being beneficially interwoven with information streams and local ecosystems (i.e., becoming part of the local natural realm). The medium for information exchange within this context is metabolism, specifically through the transfer of electrons, which is essential for sustaining life processes in biological systems by providing the energy needed for cellular functions and facilitating the interconversion of different forms of energy and matter (Gasteiger et al., 2010). While these vital processes take place in many kinds of biological systems from cellular respiration to photosynthesis, redox reactions in metabolism, electron transport systems and cell signalling, a window of opportunity for visualisation is possible when they become directly accessible through the specific exchanges within electroactive microbes (Potter, 1911). These anaerobes form biofilms that produce action potentials, which can be harvested, providing a direct monitoring technique for otherwise indecipherably complex processes. We envisage that being able to access and even manipulate dynamic “living” processes within biological systems and the eco-systemic infrastructures that support them, enables a vital transformation based on life-promoting metabolism, which can meet the demands of an evolving society. Simultaneously, these metabolic processes can perform bioremediating actions that mitigate urban impacts that currently characterise the Anthropocene, where the consequences of our modern industrialised Western lifestyles have tipped our “living” planet into an emergency condition of distress and pending collapse.
Through a critical examination of historical contexts and approaches and a visionary adoption of interdisciplinary methods, and a more fundamental, interactive relationship with fundamental biological processes that support the base of the biosphere, made accessible through the digital realm, we chart a course towards spaces through a new relationship with Earth Based Materials (EBMs) that form the foundations for a novel selection and choreography of material choices, which promote the natural activities of dynamic, living systems—and are capable of negotiating a symbiotic relationship between the built and natural world.
2 From vision to impact: charting the course of innovative research
2.1 Visionary transformation: reshaping construction through regenerative principles
Our proposed “living” urban environment suggests a significant departure from traditional, static architectural structures governed by hierarchical control systems reminiscent of “machines” (Tzonis and Corbusier, 2002). We propose that via living metabolisms, cities will develop infrastructures that enable our living spaces to evolve into fluid, adaptable, and eco-systemically active environments, shaped by collaborative, empowered agents working in concert. This collective effort is afforded by a dynamic material condition, which is integrated into a broader intelligence comprising “smart” digital systems, and microbes to form new material configurations based on natural substrates that collectively possess qualities reminiscent of life itself. These systems transcend mere structures or “intelligent” (i.e., digital computing-centred) resilience and are directly intertwined with the very fabric of life. This is achieved by leveraging dynamic material exchanges such as metabolism, growth, active decay, to cultivate a profound symbiosis between the built environment and the natural world (Koolhaas et al., 2011; Schalk, 2014; Tamari, 2014), envisaging the built environment as an active participant in a circular, environmentally compatible economy (Kirchherr et al., 2017), where natural metabolism, materials and energy are exchanged in continuous, regenerative cycles that use electron exchange to incorporate renewable materials/nutrients into their life cycles (Figure 1), and are compatible with the regenerative, transformational processes found in nature (Bühler M. et al., 2023). These advancements initiate a dialogue towards a renewed relationship with our depleted planet, establishing fresh protocols of practice and human development centred on the soils and EBMs that support us and on which we base our values, care, and reciprocity, adapting alongside evolving cultural trends and changing patterns of society.
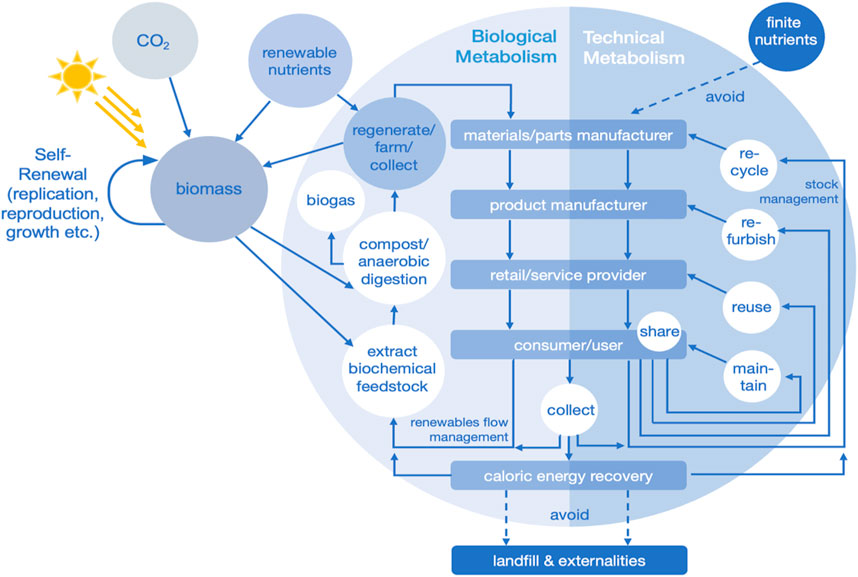
Figure 1. The diagram of circular economy systems, inspired by the butterfly model from the Ellen MacArthur Foundation informed by Braungart et al. (2007), illustrates the dual metabolism concept. Here, biological metabolism thrives on renewable nutrients, while ideally the technical metabolism is ingeniously designed to operate independently of finite resources and avoiding reliance on landfills or creating other externalities.
Combining the principles of architecture, materials science, civil engineering, philosophy, artistic research, and the social sciences, our methodological framework involves interdisciplinary convergence that reinterprets the nature of buildings as having many synergies with “living” organisms that are accessible through the specifics of their metabolism. By incorporating various aspects of living organisms into buildings in a material way, means their capabilities can be engaged to create dynamic and responsive built environments, where the inhabited space possesses some of the sensibilities of entities that we instinctively recognise as “living” e.g., sensitivity, decision-making, change with time. Living organisms offer a wealth of potential contributions, including the ability to metabolise waste, regulate temperature, generate energy, and interact with their surroundings in adaptive ways.
Transitioning from traditional, energy-intensive, centralised, hierarchical operations of machinery to the highly distributed, soft, horizontal exchanges that typify “living” materials and technologies, holds the promise of significantly reducing the carbon footprint associated with our buildings and infrastructure (Chang et al., 2015; Bhina et al., 2023). Our strategy not only aims to decrease the embodied energy needed for manufacturing construction materials but also aims to transform building systems, including utilities, through the implementation of regenerative and bioreceptive design. Such a shift aligns with the United Nations’ Sustainable Development Goals (SDGs) but also promotes practices for human development that actively repair damaged ecosystems, while nourishing and sustaining life (Goubran et al., 2023).
2.2 Earth based materials
This nature-inspired, or biophilic approach, recognises the foundational role of earth in the story of life (Logan, 2007) as the site for creative meetings between animal, plant and mineral and its deep relationship with the evolution of diverse ecosystems, supported by their living soils. In this context, the inherent material properties of earth-based materials (EBMs) such as rammed earth, cob, and adobe, offer a sustainable pathway to constructing buildings that are environmentally friendly, energy-efficient and durable through the implementation of a range of novel, EBM applications, which include building services and bioreceptive surfaces, each of which promote the growth of microorganisms, creatures, and plants in their own way, creating a living interface between the building and the ecosystem.
Earth-based materials encompass a wide range of substances found within or on the Earth’s crust. These materials have been integral to architecture for millennia and are typically derived from geological processes and can vary significantly in composition and properties that include minerals and organic matter in various configurations of textures and particles ranging from silt and clay to sand and gravel. Offering sustainable, environmentally embedded solutions that support circularity and local ecosystems, they are valued not only for their aesthetic appeal but also for their low environmental impact, durability, and ability to create buildings that are integrated with their surroundings. They offer excellent thermal mass and insulation, contributing to energy efficiency and indoor comfort. At the end of their lifespan, earth-based buildings can be returned to the Earth, completing the cycle without leaving a long-term environmental footprint.
The selection of materials and methodologies, as detailed in Table 2, is founded on regenerative design principles, with the objective of enhancing sustainable urban development. This choice is critically informed by Life Cycle Assessment (LCA) steps, emphasizing the reduction of the ecological footprint across the materials’ lifecycle. Special attention is given to unprocessed materials such as rammed earth, which stand out for their lower carbon footprint, attributable to minimal processing and the absence of hydraulic binders. These materials are not only pivotal in decreasing greenhouse gas emissions but also play a significant role in improving the thermal efficiency and aesthetic qualities of the built environment. Data and references from the Bundesinstitut für Bau-, Stadt-und Raumforschung (BBSR) validate the incorporation of these principles within the realm of sustainable construction, highlighting their applicability within Germany’s regulatory environment and their potential for wider adoption. This approach showcases the environmental and practical merits of using naturally abundant, minimally processed materials. By adhering to this methodology, which is supported by exhaustive environmental assessments, the aim is to contribute towards a construction paradigm that is sustainable, resilient, and in sync with ecological systems and sustainability objectives, as illustrated by the strategic selection presented in Table 2.
While they are versatile building materials, EBMs are also fundamentally life-promoting to support “living” materials and technologies that comprise the tissues, flesh and living bodies of the “living” city, each “living” architecture providing its own version of sustainability, breathability, and natural insulation according to its niche and embodying the foundational dynamic relationship between life and minerals (Wei-Haas, 2016).
Although the building details are highly contextualised and locally sourced, the built interventions themselves share general principles for excellent practice being structurally sound, and minimising energy consumption (embodied, building operations) while retaining their capacity for ecological integration, life-promoting actions, and responsiveness to human presence (Table 3).
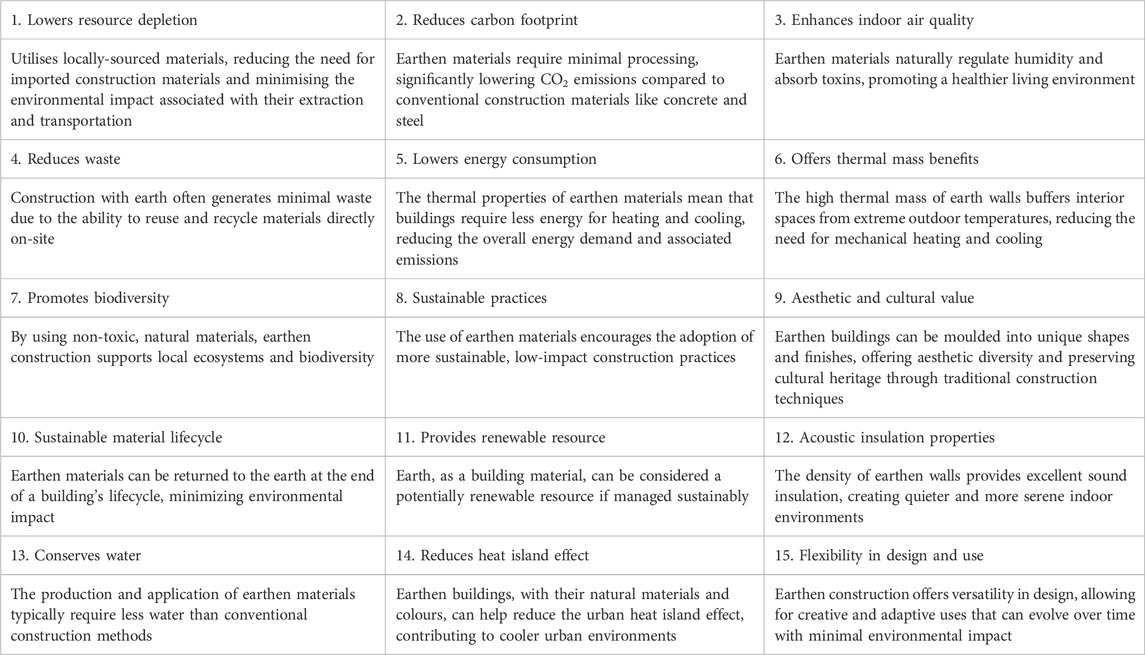
Table 3. 15 reasons to build with earth-based materials (EBMs) as an alternative to conventional materials.
2.3 Microbial technology
While individual microbes—such as fungi, bacteria, viruses, archaea, and protists—are invisible to the naked eye, they become readily observable when they aggregate into structured communities known as biofilms. Although they are largely unwelcome in the built environment and regarded as “biofouling” they, nonetheless, possess remarkable capabilities that shape their interactions with the environment and are often the first organisms to colonise any available surface. At their core, microbes are adept at metabolizing various substances, breaking them down and transforming them into new compounds. This metabolic prowess forms the foundation for their potential as environmental mediators. By leveraging the metabolic activities of microbes, we can initiate transformative processes that remediate pollution, enhance nutrient cycling, and restore ecosystems. For example, certain microbial species are capable of metabolizing pollutants such as hydrocarbons or heavy metals, effectively detoxifying contaminated environments and restoring ecological balance.
Bioreceptive surfaces facilitate the colonisation and growth of living organisms on their surface, particularly microbes which are often the first colonisers. They can occur naturally, such as patinas on rocks, or can be intentionally designed to interact with biological elements. EBMs are highly bioreceptive materials, readily fostering a symbiotic relationship between the built environment and the natural world, being characterised by high surface roughness, high open porosity, high capillary water content, an abrasion pH below 10 (Miller et al., 2012) and minerals, e.g., phosphorus (Jones et al., 2022). Recently, bioreceptive surfaces have been installed to integrate human-made structures with the surrounding microbial ecosystem to promote biodiversity, ecological equilibrium, and sustainability by accommodating and nurturing the natural growth and colonisation of surfaces by environmentally beneficial microbes, followed by small plant species. While many surfaces can be colonised, EBMs in particular naturally leverage their material composition and physical properties to support microbial growth and surface succession through features like rough surfaces, which create microclimates and provide attachment points, as well as porosity and patterns that facilitate water flow (Mustafa et al., 2021). Without intentional design efforts, facades can become unintended hosts to plants like microalgae, mosses, liverworts, and lichens. Although these plants offer considerable environmental advantages, they are often viewed as unwelcome intruders (Elbert et al., 2012). For instance, according to Freeman et al. (1974), mosses have the capacity to capture 1 kg of carbon dioxide for every half square metre, contributing to an annual global reduction of 14 billion tonnes of carbon dioxide and the fixation of 50 million tonnes of nitrogen, as documented by Elbert et al. (2012).
In 2018, the innovative installation “Subculture: Microbial Metrics and the Multi-Species City,” brought to life by David Benjamin (The Living), Kevin Slavin, and Elizabeth Hénaff at the Storefront for Art and Architecture Gallery, showcased an inventive use of wooden panels. These panels, designed with concentric circular patterns (macro shapes), were deployed to capture microbial life—specifically, the urban microbiome—from their immediate surroundings. The collected samples were then analysed through advanced small-scale genetic sequencing techniques (King, 2014), transforming the facade of the building into a dynamic living environment and a functional analytical lab. This novel concept effectively repurposed the entire structure into an “urban metagenomic sensor.” Wood was selected as the material of choice for its bio-receptive properties, attributed to its molecular structure, which includes micro shapes inherent in the wood grain and macro shapes in the geometric design (Storefront, 2023). Although bio-receptive materials do not integrate microbes into their fabric directly, they recognize and harness the vital role these organisms play in enhancing ecosystem services. This approach is especially pertinent for urban areas, where the preservation of natural soils is increasingly compromised, highlighting the importance of integrating nature-based solutions for urban environmental health.
2.4 Functionalising biofilms and bioreceptive surfaces
Although they look slimy and formless to us, at the microscopic level, biofilm architecture is highly complex (Shi and Xu, 2019) and is the preferred mode of existence for >80% of microbes. On attachment to a substratum, these multicellular, surface-associated communities transform into a microcosmic city, being Earth’s first type of formal settlement—and biofilm-forming organisms have been traced back as long as 3.5–3.8 billion years (Mojzis et al., 1996). Biofilms have intricate but highly effective, nutrient transportation, communication, and waste management networks, which play a critical role in nutrient cycling (Shi and Xu, 2019), interspecies electron transfer (Kato et al., 2012) and are common in natural environments like rice-paddy soils (Rosenbaum and Franks, 2014), compost (Dulon et al., 2007), ocean sediments (Mathis et al., 2008) and wastewaters (Logan, 2005). ElectroActive Bacteria (EABs) (Marsili et al., 2010) are a subset of biofilms (Nevin, 2008) comprised of ElectroActive Microbes (EAMs) that use their attachment material for respiration. When mature, attached EAMs cross-link between cells to create an EAB by forming a structurally stable conductive network with nutrient transportation, communication, and waste management networks. Just as urban planners and architects use their knowledge of civil engineering principles and building regulations to build better cities for people, the organisational rules for forming EABs can also be used by scientists and architects to develop nature-based solutions for buildings and urban spaces. The flow of electrons in EABs is complex and the Extracellular Electron Transfer (EET) mechanisms, attachments to the anode surface, electrochemical, biochemical, and physical dynamics of stable EABs have been extensively studied (Baubata et al., 2012). By viewing electroactive microbes as the opportunity to interact with a biobased technological platform via action potentials using electronics and digital technologies to establish fundamental new knowledge for establishing a platform for sustainable interactions and connections with the natural world. Key to generating an accessible interface between these human and more-than-human realms, electrogenic bacteria offer a rich source of inspiration for designing dynamic new materials and interfaces that facilitate interactions between people and nature through mediated digital platforms.
Microbes are parallel environmental processors that do not perform one task at a time but operate multiple metabolic programs simultaneously to serve as powerful agents of bioremediation, converting organic waste materials into valuable resources. Through processes such as composting and anaerobic digestion, microbes break down organic matter, releasing nutrients and generating biogas for energy production. This not only reduces the burden of waste disposal but safeguards soil health and fertility, acting as mediators in nutrient cycling and plant-microbe interactions. Mycorrhizal fungi, for instance, form symbiotic relationships with plant roots, enhancing nutrient uptake and improving soil structure. Similarly, nitrogen-fixing bacteria convert atmospheric nitrogen into a form that plants can utilize, enriching soil fertility and reducing the need for synthetic fertilizers.
By engaging with the metabolic capabilities of microbes, we can design innovative technologies and strategies that promote environmental sustainability and resilience. By harnessing the bioelectric properties of these microorganisms, researchers can create innovative solutions that enable bidirectional communication, dynamic adaptation, and sustainable coexistence between biological and digital ecosystems (Gorby, 2006). From bioremediation to waste valorisation to soil restoration, microbes offer a versatile toolkit for shaping our relationship with nature at the most fundamental level, thereby creating opportunities to address some of the most pressing environmental challenges of our time. These innovations are achieved through the collaboration of chemists, biologists and engineers/roboticists to ensure the structures are deployed appropriately with respect to their material characteristics and readable information exchange. They are also designed to support and enhance the wellbeing of society by producing their solutions locally, which greatly reduces carbon emissions from transport), sources materials in sustainable ways (e.g., cleaning industrial soils to generate EBM blocks, using agricultural waste materials like wood chips for mycelium bio-composites) and by not using Critical Raw Materials (CRMs). The incorporation of advanced computer models and simulations, led by engineers, computer scientists and data analysts, enables the optimization of these materials (for performance, and embodied energy) and designs (via algorithms that generate resource-efficient designs) in real time, which is perceptible using bioelectrodes that respond directly to changes in microbial electroactivity. This digital layer enables a continuous feedback and adaptation loop, ensuring that the human designer and engineer can understand, interrogate, and meaningfully influence the processes underpinning the construction of buildings to different degrees. Such structures are not static entities, but dynamically evolving systems that can adapt to the changing needs of occupants, resource availability, optimise embodied energy use, and benefit environmental performance. In conjunction with these material and computational innovations, it is also possible to extrapolate the insights and incorporate ethnographic principles from gerontology for an ageing population, urban ethnography, the study of different communities, e.g., Jacobs (2016) and integrative design to ensure that the built environment is responsive to the unique needs of more diverse types of people, diverse and inclusive ways of living (although a detailed discussion on the ethnographic impacts of “living architecture” are not proposed this particular paper, it is an ongoing exploration that will be detailed in another publication). This comprehensive, cross-disciplinary approach ensures that our approach to making buildings is bespoke, contextualised to meet local challenges and needs, and is sufficiently flexible to provide comfort, accessibility, high quality aesthetics, and adaptability, improving the overall quality of life for inhabitants.
2.5 Living with microbes
Recently, microorganisms have been incorporated into diverse building materials, surfaces, and systems, which has catalysed the development of innovative prototypes and installations aimed at reducing energy and resource consumption while also supporting and revitalising local ecosystems (Chang et al., 2015; Chang et al., 2015; Joshi et al., 2017; Jain et al., 2021; Salifu et al., 2021; Armstrong, 2023a; Garg et al., 2023; Sarkar et al., 2023). Using microbes as a foundational technology presents a ground-breaking approach to sustainable architecture and urban planning. With their inherent capacity to maintain biosphere health, microorganisms like bacteria and fungi represent an underexplored resource capable of reshaping our environment positively. These microscopic entities have been fundamental to Earth’s ecosystems for billions of years, playing vital roles in preserving ecological equilibrium and resilience. By integrating microbes into construction materials, surfaces, and operational systems, we tap into their ancient abilities to produce new construction materials, purify pollutants, and facilitate circular metabolic processes, such as converting organic waste into nutrient-rich compost and energy-dense molecules.
Through meticulous adjustment of environmental conditions, these innovative systems empower microbes to undertake a whole range of biologically useful tasks, effectively transforming waste into valuable resources. Waste streams are not only detoxified but repurposed as productive systems yielding various materials and energy-efficient solutions. This approach lays the foundation for a circular economy, where resources are regenerated, reused, and valorised, thereby minimising waste and diminishing the need for new raw materials. Such a strategy represents a significant advance in sustainable resource management and aligns with broader environmental objectives by creating an implementable platform that enables efficiency and resilience in both natural and human-made ecosystems. As microbial communities thrive within organic waste systems, such approaches offer scalable solutions that seamlessly integrate with waste management protocols to generate eco-friendly materials like mycelium-based composites using agricultural waste materials like wood chips (Bio, 2024) or innovative building services such as PeePower® (which makes energy for LED lighting services from urine). These applications form part of a comprehensive suite of microbial technologies and design approaches aimed at shaping the future of eco-conscious construction. Such innovations provide a versatile toolbox for architects and engineers to facilitate the design and construction of buildings that synergise with and actively contribute to natural processes. While the examples highlighted here only represent the beginnings of more “gardened’ ways of processing wastes, they also underscore the pioneering advancements in circular design and engineering occurring at the intersection of scientific inquiry and architectural ingenuity, where state-of-the-art microbial technology plays a central role in the conception, implementation, and operation of environmentally friendly buildings.
2.6 Microbial building materials transforming sustainable construction
Microbial building materials (Jones et al., 2022), epitomise a transformative approach to sustainable construction towards more adaptable, resilient, and sustainable building practices. By integrating synthetic biology with architectural design, the functions of microbes within the material matrices can be designed, holding the promise of revolutionising how structures are conceptualised, constructed, and interact with their surroundings. Introducing the possibility of cellular engineering into the processes of building design and engineering, establishes the groundwork for a future where buildings can self-repair, dynamically respond to environmental changes, and minimise their ecological footprint according to human design.
This innovative application of microbial technology not only reduces the environmental impact of our built environment but also improves the ecological wellbeing and vitality of human communities, ecosystems, and the planet (Timmis et al., 2019), enabling a paradigmatic change toward construction practices that are not merely sustainable but regenerative, paving the way for structures that actively promote the restoration of their adjacent ecosystems (Armstrong, 2023a). Adopting these biologically integrated solutions can progress us toward a future where buildings and infrastructure not only work along with nature but actively engage in its mechanisms, enabling a more robust and life-affirming connection between human progress and the biosphere.
Microbial Fuel Cells (MFCs) mimic the structure of a chemical battery by consisting of an anode and a cathode (Rabaey and Verstraete, 2005). Unlike traditional batteries that use inert solutions, MFCs utilise anaerobic biofilms developed on the anode, separated from the cathode by a proton-exchange membrane. This membrane is commonly a porous EBM like terracotta, which is an excellent material for promoting biofilm formation and is a prime example of how EBMs derived from natural clay deposits can be transformed through advanced technological applications as well as traditional craftsmanship into a versatile and enduring medium capable of acquiring new functions. Energy production in MFCs occurs as the biofilm digests an organic “feedstock,” such as urine, greywater, blackwater, or various forms of liquid organic waste. This process leads to the bacteria at the anode decomposing the feedstock and releasing electrons. These electrons are then transported to the cathode compartment through a conductive material, creating an electrical current that can power electronic devices. Simultaneously, protons move across the membrane to the cathode, where they combine with oxygen to produce water. MFCs also play a crucial role in nutrient recovery, producing biofertilizer from stabilised sludge rich in nitrogen and phosphate. They effectively treat wastewater and eliminate pathogens, mirroring the processes of natural soil biofilms. MFCs excel beyond conventional utilities by multitasking resources simultaneously, functioning as parallel processing units.
Recent advancements in MFC technology have focused on optimising various parameters to boost performance, including selective use of microbes, application of genetic and molecular engineering techniques, improvement of electrode materials, and innovative membrane designs. Over the past few years, MFCs have been explored in field trials for wastewater treatment, notably in projects like the development of PeePower® urinals by Ioannis Ieropoulos at the University of Southampton. These urinals, supported by The Bill and Melinda Gates Foundation and Oxfam under the Reinvent the Toilet Challenge, aim to provide essential services in refugee camps and schools. They offer basic nighttime lighting for safety and support water and sanitation needs (Walter et al., 2018). Additionally, PeePower® urinals have been tested in Western settings, such as the Glastonbury festival, where attendees contributed their urine to power a variety of services, including phone charging, gaming, and powering festival screens, showcasing the versatile application of MFC technology in both developing and developed contexts.
Household bioreactors can often present interpretive challenges for the average user. To bridge this gap, the “Active Living Infrastructure: Controlled Environment,” or ALICE, has been developed with a user-friendly digital interface. This interface simplifies the data from Microbial Fuel Cells (MFCs) into easily understandable insights (ALICE, 2019). ALICE employs engaging animations named “Mobes,” which creatively represent biofilm activity, demonstrating the system’s capability to produce 200 mW/L from urine (You et al., 2022). Moreover, ALICE has gained recognition as an art installation, featured at the Victoria and Albert Museum during the Digital Design Weekend in September 2021 and at the Electromagnetic Field festival in Eastnor in June 2022. As an embodiment of a novel bio-digital platform, ALICE marries microbial and artificial intelligences with both biological and technical systems. This innovative approach offers an architectural solution that effectively conveys to inhabitants the performance of their domestic waste streams in an engaging and comprehensible manner.
Microbial Fuel Cells (MFCs) have been integrated into a variety of mixed bioreactor systems, enhancing the treatment, production, and recovery of microbial products in a sustainable, closed-loop system for residential use (Armstrong et al., 2017). The Living Architecture initiative has successfully shown how different types of bioreactors, including photobioreactors, MFCs, and synthetic bioreactors equipped with engineered microbes, can transform nutrients from household liquid waste into both electrical energy (4–5 mW/unit) and biomass. While the energy yield of this setup is relatively low, it paves the way for the proliferation of low-powered Direct Current (DC) electronic systems. This represents a viable alternative to the high-powered (230V) Alternating Current (AC) infrastructure that dominates contemporary settings. Additionally, it fosters the broader acceptance and use of low-power electronic appliances in homes, promoting energy efficiency and sustainability.
Further advances in converging different bioreactors are being developed with the integration of MFCs and hydroponics. The Microbial Hydroponics (Mi-Hy) project pioneers a cutting-edge circular platform and Hydroponic-Bioelectrical System (BES), which is specifically designed for urban agriculture, seamlessly merging Carbon (C), Nitrogen (N), and Phosphorus (P) metabolism in plants with microbial metabolism. This innovative system combines Microbial Fuel Cell (MFC) technology, which generates electricity from biochemical reactions involving electrons, with hydroponics, a soilless plant cultivation method aimed at optimising CO2 and N transformation (initially for animal feed production) but also valorising the use of organic wastes within urban environments, specifically at the community level. The microbes in Mi-Hy’s electrodes primarily utilise carbon compounds derived from plant root exudates (e.g., sugars, organic acids, amino acids), which are synthesised using atmospheric CO2 captured through photosynthesis. Nitrogen utilised by the microbial fuel cell (MFC) or microbial electrolysis cell (MEC) is sourced from wastewater, which is simultaneously purified by the MFC, removing phosphorus, chemicals, pathogens, and other contaminants. In Mi-Hy, the MFC biofilm acts as a surrogate rhizosphere for plant roots, enhancing nitrogen utilisation by enabling symbiotic relationships between fungi and bacteria to improve nutrient absorption from the environment. This nitrogen optimization, involving processes like chlorophyll and amino acid synthesis, eradicates the need for chemical nitrogen sources (e.g., artificial fertilisers) mitigating costs associated with nitrogen removal, which typically accounts for 10% of wastewater treatment expenses, while converting carbon (gaseous carbon dioxide) into biomass, in ways that can recycle flue gas, or emissions from HVAC systems. Mi-Hy’s processes result in reduced CO2 emissions, electricity production, food cultivation, and the generation of valuable biomolecules, all validated through laboratory testing. In this context, hydroponics serves as a tertiary treatment following wastewater treatment plants, performing similar functions while consuming carbon and inorganic nutrients (such as nitrogen, phosphorus, and sulphur). Additionally, hydroponics aids in bioremediation by addressing various contaminants, including chemicals, pharmaceuticals, and hormones, depending on the microbial composition. Overall, Mi-Hy functions as a fundamentally circular system, relying solely on atmospheric CO2, light, and household wastewater streams as the foundational inputs, enabling the design and engineering of complex ecosystems within building operations as part of the regenerative construction toolset.
The Knowledge-driven Hub for Advancements in Traditional Building Applications (“KHATA”) project is an EIC Pathfinder Challenges proposal for the Architecture, Engineering and Construction digitalisation for a novel triad of design, fabrication, and materials call (EIC, 2023) which goes far beyond state of the art in bioreceptive design by providing reliable alternatives to concrete in an extended range of Earth Based Material (EBM) applications in non-load bearing contexts. It aims to revolutionise the European Architecture, Engineering, and Construction (AEC) sector by proposing a novel combination of Computational Design (subcategories: Parametrics, Biomimicry, Digital Twin, AI); Digitalised Fabrication (subcategories: 3D printing, Additive Manufacturing, Industrial Production, (Rahman et al., 2022)) and Materials (subcategories: Natural Materials, Bio-based, Metamaterials, Engineered Materials Metamaterials, Discrete Blocks). State of the art in bioreceptivity is mostly limited to concrete (Elbert et al., 2012) as a way of reducing the carbon footprint of the material by supporting the natural growth of cryptogamic covers on its surface. These rudimentary soils consist of mosses, microorganisms, and small, rootless plants known as bryophytes. They offer promising substitutes for conventional green walls, eliminating the need for costly infrastructure (Mahrous et al., 2022). The species are not modelled for the Digital Twin, but the surface area related to pattern and curing/drying of EBM links the building block and material. Bioreceptivity is both part of the natural characteristic of a material and can also be achieved by parametrically generated textures to channel water and provide shelter (Stohl et al., 2023) for colonising building surfaces with cryptogamic covers (Mustafa et al., 2021). KHATA extends the unit design of EBMs to extend to non-load bearing, external-facing surfaces by using bioreceptive principles to examine their effect on water flow and surface roughness (D’Orazio et al., 2014) to maximise the curing (consolidation) rate, minimise erosion and foster long-term resilience and carbon-neutrality of the built structure. KHATA, therefore, uses bioreceptivity for the first time to examine its impact on surface erosion as part of the assessment of surface performance in EBMs. Cryptogamic covers may also provide a soft protective cover for EBMs buffering them against erosion, which must be balanced with the degree of water penetration, porosity, and flow on the surface to optimise cryptogamic cover growth, with the erosive forces of water via surface channelling. Establishing the parameters to achieve this balance is KHATA’s breakthrough and will be evaluated using a range of organic surface stabilising treatments, e.g., alginate. The overall EBM unit structure and surface performance will be digitally modelled to determine the overall unit design based on geometric and site-specific attributes such as orientation, light incidence, and local climate while complying with use requirements, aesthetic considerations, and economic constraints. Future renditions will incorporate electroactive microbial communities, potentially on the underside of the panels, as they can metabolism without light and their interactions recorded as direct digital signals. KHATA proposes to achieve this model by developing an Open-source Multiphysics, multi-scale Modelling and Simulation Framework for earth-based material. It generates an Informed Digital Twin as a real-time representation of EBM-based buildings driven by physical insights, advanced numerical modelling, and use of Internet of Things (IoT) sensors embedded within the building (respectively: structure). Feedback to the Informed Digital Twin and its modelling components provide a nuanced understanding of intricate hydraulic processes and mechanical behaviour characteristic for (but not restricted to) EBM-structures. The Twin enables non-specialist use of the heterogeneous EBMs, whose material properties are considered regarding 1) composition/texture, 2) drying dynamics dependent on local environmental conditions, and 3) the relative position within the built structure, establishing a valuable tool for monitoring and maintenance of earth-based structures towards a basic Digital Twin demonstrator and enabling correlations to be made with the bioreceptive surfaces. The broader aim is to develop digital tools to enable low-threshold access to the technology which enables widespread uptake in the ACE sector by using versatile concepts for knowledge exchange and information management, programming interfaces (APIs), and intuitive, low-threshold human-machine-interfaces (HMIs). Advanced rendering and interactive visualisation mechanisms are combined with the investigation of generic business concepts to ensure efficient uptake and scalability, seamless integration with existing digital assets in AEC, and so promote usage by architects, engineers, public authorities, craftsmen, and the citizen science community. This innovation is integrated through a WikiHouse.cc model for construction (Priavolou and Niaros, 2019), not only ensuring effortless access for SMEs and other ACE sector actors, but also serving as a dynamic platform to investigate user behaviour, to enable scenario planning as well as predictive analytics adding value to the community and strengthening the business case for its adoption towards the digital transition and its rollout across Europe.
By combining various microbial processes, with surface topologies, material substrates, and their dynamic environmental conditions it is possible to create hybrid materials and building solutions that surpass the individual capabilities of their components becoming more like a garden, than a building skin or structure. Such a combinatorial process not only applies to geometries, surfaces and structures but also extends to “living” material blends, which will increasingly incorporate living electrogenic microbes that will train predictive modelling systems to enable their performance to be dynamically modelled and enable their broader incorporation into the built environment.
2.7 Living architecture: a hypothesis for adaptive and self-organising built environments
Our vision for the urban environment builds upon the conceptual underpinnings provided by Cedric Price (Price and Hardingham, 1984), who envisaged adaptable and interactive spaces. We are also inspired by the practical advancements realised by Achim Menges (Menges, 2012; Reichert et al., 2015) through the integration of computational design and robotic fabrication. Together, these figures exemplify a bridge from the philosophical exploration of space adaptability to the technological actualization of these concepts through an agentised material perspective, thereby fostering spaces that are not only flexible but also symbiotically integrated with their occupants and the surrounding ecosystem–as living skins. Through this synthesis, we aim to transcend conventional architectural paradigms, creating a built environment that provides windows of opportunity to observe aspects of the responsive, resilient, sustainable, materialisable, biological and technological networks that are deeply entwined with contemporary human lifestyles.
By applying advanced biological insights (biotechnology, metabolic design, microbiology, etc.) in concert with smart embodied systems (e.g., metabolic processes, embedded sensors with digital feedback), agentised materials (e.g., titanium dioxide and other catalytic surfaces), we anticipate an architectural revolution where structures can process their environment (metabolism), self-assemble (e.g., unattenuated mycelium bio-composite units growing together into a single monolithic structure), self-repair (bio-concrete generating a calcium carbonate scar to prevent further water entry into the material by virtue of embedded microbial extremophiles that are activated by water) and evolve (bioreceptive surfaces providing microniches for biological succession), establishing resonances with living systems. Applying the principles of bioinspiration, bio-design and intelligent systems, we anticipate the emergence of materials capable of self-organisation, self-repair, and environmental adaptation that exceed the level of complexity possible using robotically-controlled bricks. Rather, our operations take place as a choreography of material exchanges at the molecular scale (Figure 2), which redefines the essence of construction and maintenance. Buildings can now autonomously adjust to environmental changes persistently and dynamically, leading to an alteration of building performance, programming and modes of occupation, which can ultimately lead to demographic shifts and labour market transformations. This synthesis of dynamic material programming, optimised design and alignment with natural processes is underpinned by a natural building ecosystem, such structures and systems become active agents in their own operation and maintenance, fostering a symbiotic relationship between its form and inhabitants. For example, the Living Architecture project (Armstrong et al., 2017), a prototype “living” wastewater treatment system, turns household greywater into cleaned water, biomass and bioelectricity using the powerful microbial metabolism of natural biofilms.
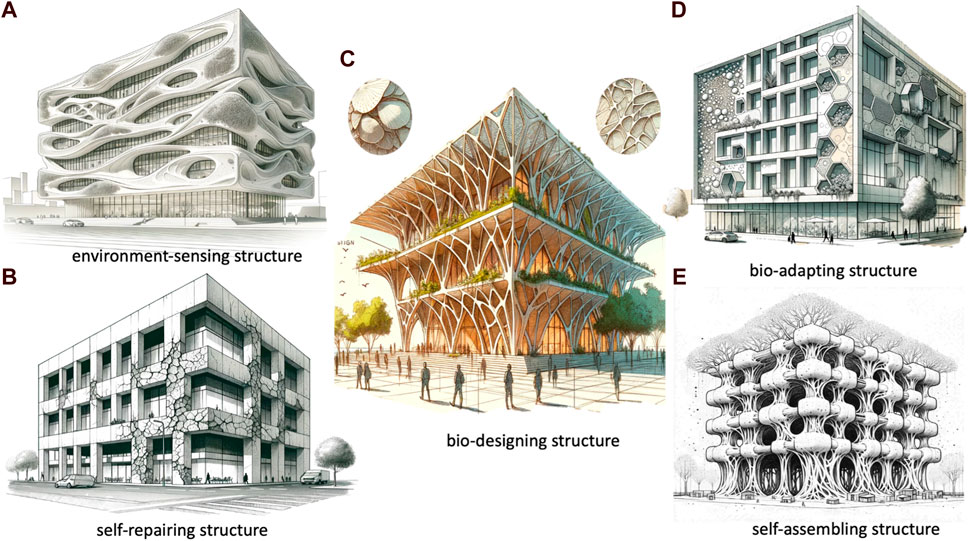
Figure 2. (A) Environment-sensing structure: Smart materials equipped with metabolic processes and digital feedback systems adjust the building’s functionality in real time to optimise energy use and enhance living conditions; (B) self-repairing structure: Bio-concrete that activates microbial extremophiles upon contact with water, triggering a self-repairing process to seal cracks and damage, enhancing the material’s longevity (Sharma et al., 2017); (C) bio-designing structure: Biomimicry and bio-design in creating materials and structures that can self-organise, repair, and adapt, inspired by the efficiency and resilience of natural systems; (D) bio-adapting structure: Buildings can evolve by adopting bioreceptive surfaces that mimic living systems, responding dynamically to changes in the environment and improving over time; (E) self-assembling structure: Materials based on mycelium can grow together autonomously, forming durable, eco-friendly structures without the need for traditional construction methods; illustrations created by the authors using ChatGPT-4/DALL·E 2, 2 February 2024.
Maintenance is reimagined as a continuous, pre-designed process intrinsic to the building’s lifecycle through the water-using daily activities of its inhabitants. Such circular “living” building operations envisage a future where the built environment operates concordantly with natural and human ecosystems, exhibiting inherent resilience, reducing reliance on human labour, and promoting a sustainable cycle of renewal and regeneration—closing the loop between consumption and waste to ensure circular exchanges within our living spaces, while being observable through a digital device. In this context, the built environment emerges as a new actor—a complex, adaptive system characterised by interdependent relationships between its constituents. This hypothesis not only invites a rethinking of architectural design and materials but also calls for a broader consideration of the social theories that inform our understanding of space and place specifically through a reconsideration of relationships, communities of inhabitation (human and non-human) work, and the quality of experiences within our living spaces. By engaging with the existing body of literature and expanding upon it through rigorous experimentation and the production of prototypes, we aim to forge an innovative, compelling narrative that showcases the potential for a truly regenerative and interconnected built environment.
2.8 Transformative impacts: economic and ecological benefits of innovative construction
“Living” architectures and those communities of agencies that comprise “living” cities, also incorporate a form of automation and robotics toward more flexible, decentralised, “softer” technology applications. Embodied intelligence, e.g., the use of smart sensors and adaptive systems for environmental control, and agentised materials, i.e., materials that perform work like self-healing concrete (Jonkers and Schiangen, 2008), self-cleaning glass and electrochromic glass, are key to this convergence making possible assemblages of interactions that form resilient, self-regulating systems capable of revolutionising the industry and yielding economic advantages, such as cutting maintenance costs by up to 30% with self-repairing materials (Shittu et al., 2021; Song et al., 2021) and reducing labour by 20%–25% through smarter design (Turner et al., 2020; Rafsanjani and Nabizadeh, 2023). This shift supports ecological balance by reducing the building’s dependency on fossil fuel, by materials directly performing “work”, e.g., through the production of bioelectricity (Bond and Lovley, 2003) and potentially increases property values by extending infrastructure longevity, and fostering green employment, thereby cultivating an economy that cherishes both human wellbeing and environmental health.
The construction industry stands at a critical juncture in its efforts to address climate change, with a pressing need to reduce its carbon footprint. A transformative strategy to achieve this goal involves leveraging EBMs as alternatives to conventional construction materials. EBMs offer a sustainable alternative to conventional construction resources, significantly reducing the environmental footprint of building projects (Table 2). Sourced locally, these materials minimise transportation emissions and overall carbon output during construction. Their inherent properties, including excellent thermal mass, contribute to energy efficiency by naturally regulating indoor temperatures, thereby reducing reliance on artificial heating and cooling. Furthermore, the biodegradable and recyclable nature of EBMs, alongside their potential for carbon sequestration (as seen in materials like bamboo), and their capacity to promote life (i.e., are regenerative) underscores their role in promoting environmental sustainability. Implementing these materials necessitates a paradigm shift towards sustainable design and construction practices, requiring architects and builders to familiarise themselves with these materials’ unique characteristics through targeted training and education. This approach not only aligns with global sustainability goals but also offers a path toward reducing the construction industry’s carbon footprint, potentially also playing a greater additional role within the cycling of resources within the biosphere (e.g., Nitrogen and Phosphorus cycles).
2.9 Historical context and evolution: paving the way for sustainable architectural practices
Reflecting on the lessons learned from past social and technical efforts (Bloom and Canning, 2004; Montgomery et al., 2013) where unprecedented challenges and opportunities for the built environment, have largely been unsuccessful due to their rigid and isolated approaches, our approach adopts an integrated and adaptive methodology that can be encapsulated by the philosophical insights of Kiesler’s concept of the “Endless House” (Conant and Kiesler, 1966), which regards architecture as organic, fluid spaces that, through perpetual flow, bring the functionality and aesthetics of the environment into the human habitat (Figure 3). We extend and develop this vision through a “living” portfolio of materials, which incorporate mineral and organic substrates, along with electroactive dynamic strategies and interventions to meet the changing demands of an increasingly diverse society, which are creatively enlivened, empowered and enacted at the design phase through the language and imaginaries within ANT and new materialism (Armstrong, 2015). Our theoretical approach incorporates this dynamism via new materialist discourses such as Jane Bennet’s vital materialism (Bennett, 2010; Bennett et al., 2010), Myra Hird who argues for a more nuanced understanding of microbial life acknowledging its role in co-constituting sociability, challenging anthropocentric hierarchies (Hird, 2009; Blute, 2010), Karen Barad who observes the inseparability and mutual constitution of entities through their interactions (Barad, 2007), and Bruno Latour’s Gaian perspectives that can be understood within the broader framework of his actor-network theory (ANT). Emphasising the agency of both human and non-human actors in shaping social and environmental phenomena, Latour regards Gaia is not a transcendent or mystical entity but a material-semiotic network of interactions among diverse entities, including humans, animals, plants, minerals, and ecosystems (Latour, 2017). In this framework, designers and engineers are no longer sole orchestrators of the built environment but collaborators with materials and other actors (plants, microbes, weather) imbued with agency, and capable of autonomous action (Figure 4). In this context, the design process becomes a process of co-construction among diverse communities of inhabitants, including diverse non-human actors such as seeds, bacteria, fungi, erosions, and infestations. This dynamic fabric of the built environment possesses the capacity to perform work, necessitating a reimagining of design challenges to balance human needs with the benefits accrued by participating within an ecosystem fundamentally comprised by non-human actants. In keeping with Carolus Linnaeus’ view of the mineral realm as a distinctive form of life (Linneaus Online, 2024), which is also conducive with contemporary theories concerning life’s origins (Wei-Haas, 2016), our view of this “living” fabric embraces the foundational, creative exchanges between minerals and life which is one of mutual influence and dependency. Minerals provide the physical and chemical framework within which the complex processes leading to the emergence of life can occur, while life, in turn, can modify and interact with minerals in various ways as it evolves and adapts to its environment. Importantly, the relationship between the mineral and organismal realms are considered through the concepts of “bios” and “zoe,” where “bios” signifies a “mode of living” with a fundamental quality of existence, compared with “zoe,” which represents bare life (Agamben, 1998) to highlight different kinds of existence, action and decision-making that occur independently of human observers. “Bios” represents a mode of living characterised by complex interactions, adaptation, and resilience, as seen in living organisms, while “zoe” signifies mere existence or bare life, devoid of the rich dynamics and interdependencies inherent in living systems but is present in a range of activated materials and molecules. In this context, decisions that shape material interactions are distributed through negotiations among multiple bodies, where humans act as diplomatic representatives of our species’ interests, recognising the multiple types and layers of intelligence that are inherent in non-human entities. Time emerges as a critical factor, where human surveillance is inevitably incomplete, which highlights the need for adaptive and responsive design frameworks and multitudinous opportunities to find ways of meaningfully engaging the more-than-human-realm. New materialism furnishes a vocabulary for navigating this Pandora’s Box of potential exchanges, helping us to critically interrogate the agency of matter so that meaningful connections and the spontaneous transformation of matter can be developed through ANT and general systems theory. The juxtaposition of frameworks and disciplines also enables engineering to intersect with unfamiliar territories such as poetic and aesthetic dimensions through disciplinary convergences that materially sustain possibilities on a buildable, experimental platform such as metabolism, fluid dynamics, information and communications technology (ICT), as well as material sciences. While “living” architecture and bio-inspired design are ongoing processes, “living” architecture catalyses a synthesis between design, engineering, and nature, integrating the living realm into creative and construction processes, thus forging a more diplomatic and inclusive approach to architectural practice.
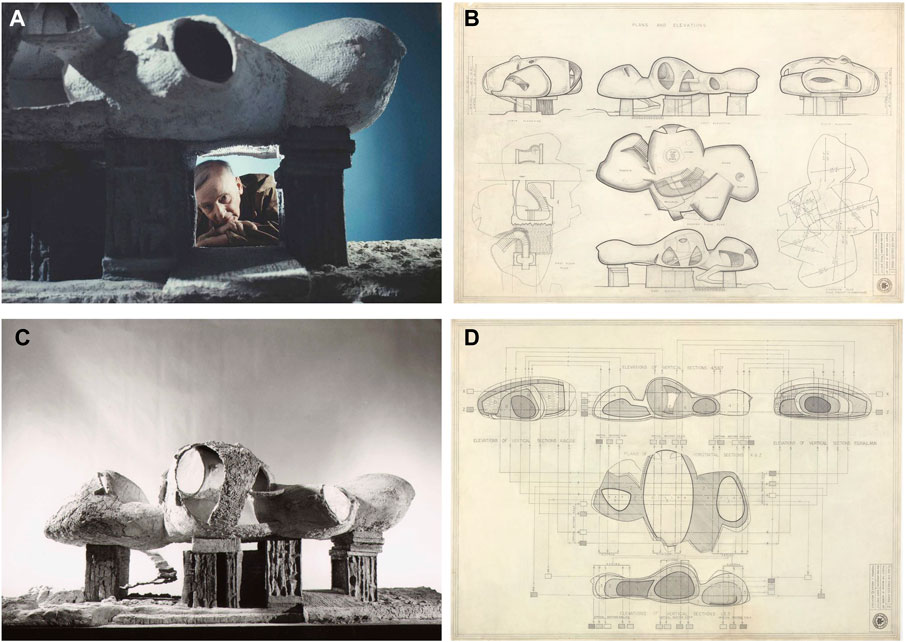
Figure 3. (A) Photography of Friedrick Kiesler and his model of the “Endless House” (1950), (B) sketch with side views and top view, (C) photography of the entire model, (D) various sections; with permission of © 2024 Austrian Frederick and Lillian Kiesler Private Foundation, Vienna.
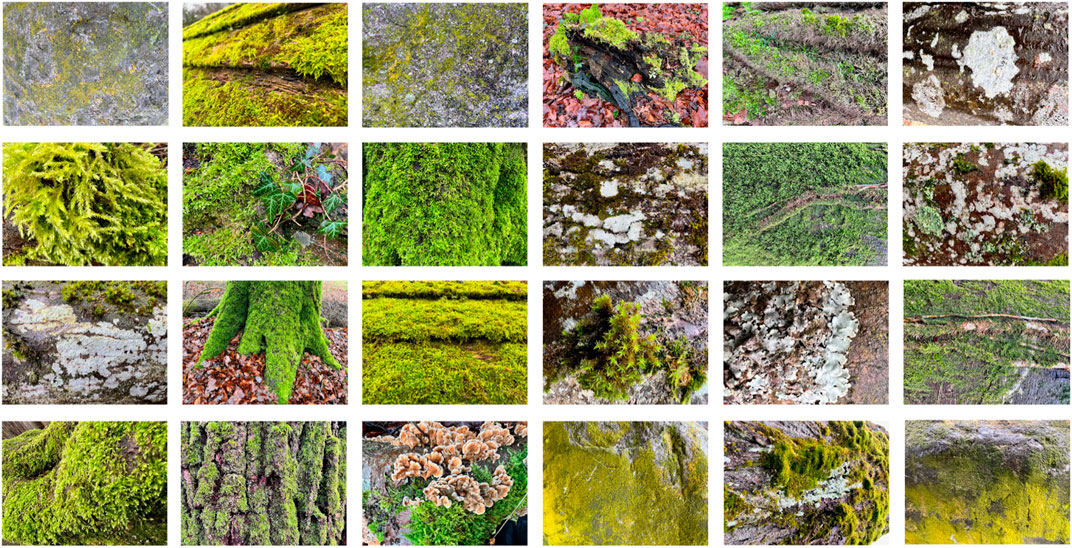
Figure 4. Bioreceptive surfaces in nature consisting of lichens, mosses, ferns, and other cryptogams such as algae and fungi, showcase a remarkable diversity of life forms that thrive in symbiosis and reproduce through spores. These organisms colonise a variety of substrates, from tree bark and stone to soil and water, demonstrating nature’s ability to foster complex ecosystems on seemingly inhospitable surfaces. Through their intricate reproductive strategies and symbiotic relationships, these species contribute to ecosystem stability, nutrient cycling, and the overall health of their environments, illustrating the sophistication of natural bioreceptive surfaces. Photographs Michael Bühler, University Forest Konstanz, 2 February 2024.
The platform and foundational step that enables design and engineering to operate within an agentised, intelligent material system is to conceptualise our living spaces as interconnected metabolic networks of regenerative exchange. These concepts are deeply interrelated, tracing back to the roots of modern principles of material transformation from alchemy in the 18th Century, where the term “metamorphosis”—linked with material change—evolved into “metabolism” (Henderson, 1913). Central to the concept of metabolism, originating from the Greek word for change, was the idea of an animal chemistry or economy, serving as the vital force of zoe, responsible for processing food and eliminating waste (Bing, 1971). By embracing the principles of bios within architecture, we advocate for designs that go beyond mere functionality to actively engage with the vitality and complexity of living systems through feedback loops of valued transactions. Blurring the boundaries between the mineral and organismal realms via zoe and embracing the principles of bios, we strive for a new paradigm that integrates the regenerative capacities of living organisms into the built environment through “living” architecture (Figure 5). This ethos proposes a multi-scalar, borderless architectural ethos that adapts to demographic changes among human and non-human populations, and actively engages them to promote social and environmental sustainability and resilience. By synthesising regenerative design principles with ANT, and the metabolic agency of the material realm, the proposed approach generates design and engineering practices that exceed the traditional logic of spatial constraints and the idea of architecture as a static enclosure. Instead, it proposes a built environment that is responsive, adaptive, intelligent, and constantly negotiating with its occupants, which enables material change over time and thereby can evolve in keeping with ecological dynamics and human wellbeing.
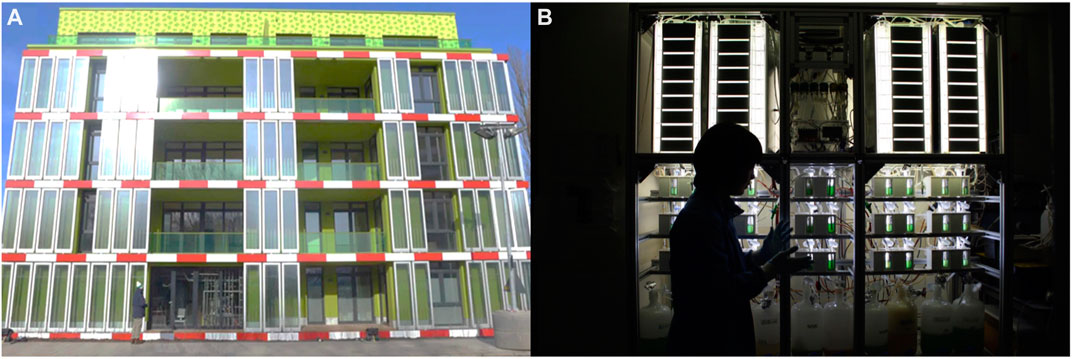
Figure 5. (A) BIQ House (BIQ, 2011), the world’s first building powered by algae, Hamburg, Germany, courtesy of Colt International, Arup (ARUP, 2022), SSC GmbH, 2011. (B) The Living Architecture bioreactor “wall” courtesy of the Living Architecture project, 2019. See a detailed description in Armstrong (2023a).
2.10 A new metaphor for “living” spaces
The concept of the “living” building is no longer well served by Le Corbusier’s mechanistic approach. Consequently, we use the term holobiont as a paradigm for studying the interactions between a host (human inhabitant) and its associated communities of organisms and lively agents that are increasingly shaping our understanding of human interactions with living spaces.
Our contemporary understanding of the relationship between people and microbes extends beyond our ancestral past, or as unwanted agents of disease following insights obtained by metagenomics into the diverse microbial communities inhabiting our personal environments. These studies have revealed the pervasive presence of microbes (“Human Microbiome”) in our bodies (The Human Microbiome Project Consortium, 2012) and living spaces (“The Microbiome of the Built Environment”) (Kembel et al., 2012). Remarkably, approximately half of our body’s cells are bacterial, highlighting the extent of our microbial companionship. Modern medical understanding emphasises the ecological model of the Human Microbiome, recognizing the crucial role of microbes in our overall wellbeing, framing people and their living spaces as holobionts (Guchte et al., 2018). Within these interactive environments, microbial activity influences various aspects of our health, from mood regulation to immune defence and essential nutrient synthesis. It is now clear that microbial colonies are indispensable for our health, as evidenced by the adverse effects of prolonged antibiotic use in disrupting these populations. However, this relationship is complex, as dysfunctional microbial communities can contribute to the onset of various diseases, including cancer, metabolic disorders, allergies, and obesity. Consequently, the Human Microbiome challenges the notion of “pure” human bodies, presenting us instead as “bodies-as-ecosystems,” where an individual person is made up from both human and non-human cells (Sender et al., 2016). This expanded understanding of self, intertwined with the microbial milieu, introduces the concept of the Human Holobiont—a collective entity shaped by the interactions among its constituent agents. By recognising that the holobiont operates as a transactional system, where the wellbeing of all members depends on their ability to coexist, without privileging any single entity, whether human or non-human, a new approach to design, engineering, care, community and the understanding of our role within the construction and maintenance of ecosystems becomes possible with associated new concepts, language and imaginaries.
Since the Human Microbiome and the Microbiome of the Built Environment coexist, microbiology and architecture are no longer separate disciplines. Researchers in the emerging interdisciplinary field of “living” architecture and engineering are now poised to create and engineer new holobiontic structures that are facilitated by EBMs to encourage types of settlement that benefit both microbes and persons. Such possibilities offer new ways of thinking about how we live, our value systems and what kinds of transactions form the basis of societal exchanges. In this context, the terms “oikos” (home) and “eco” (ecology), become intrinsic to our lived environment to alter our understanding towards a more organic and interconnected view of how we live. The concept of holobiont also aligns with the intricate dynamics of living systems and the symbiotic relationships within households. The philosophical implications of integrating biology with architecture provide a catalyst for reimagining the relationship between built environments and the living world that incorporates biological principles, processes, and materials into the creation of products, systems, and environments, integrating living organisms, biological processes. Interdisciplinary approaches that integrate developments in biological sciences, molecular biology, biotechnology, metagenomics, and microbiology will have far-reaching implications for the future of architectural and design innovation, opening up new avenues for the life sciences and applied biosciences within the practice of the built environment. Within this worldview, the concept of an ecological home exceeds the understanding of a living space as a passive structure, envisioning it as an active and semi-living entity that accommodates nonhuman inhabitants, respects its surroundings, and engages in ongoing negotiation for coexistence. In this configuration, all creatures contribute to the circular dynamics of a space, forming through collaborative actions and relationships among its diverse components. The ecological home serves as a dynamic platform for life’s essential activities, requiring first-hand experience to fully comprehend.
Architects of this intricately interconnected space recognise the integral roles played by nonhuman entities such as pets, pests, microbes, and elements from the external environment, which continuously shape every facet of its design. Extending its influence beyond traditional structures, the ecological home embraces contributions from birds, trees, soils, butterflies, and other elements of the surrounding landscape, fostering shifts in our value systems, community dynamics, and power relations. By transcending conventional domestic boundaries and integrating with the broader environment, it offers opportunities that acknowledge our inseparable connection with nature. Where there is life, there is also intelligence. Microorganisms such as slime mould (Zhu et al., 2018) and mycelium networks (Adamatzky et al., 2021) have been used to solve complex problems and challenges within urban contexts, offering tools for responsive and adaptable methods of architectural design and production. Introducing a new metaphor for living based on dynamic systems, material transformation, and collective dynamics, diverse agents contribute to the emergence of a novel domestic commons and macroeconomy (Armstrong, 2023a). In this paradigm, buildings become active participants in the economic landscape, performing tasks as a result of using innovative materials and systems, valuing various forms of labour and recognizing all citizens’ contributions. This perspective involves profound social and ecological dimensions, promoting community engagement, and environmental stewardship, prioritising collective wellbeing and sustainability through an active engagement of the commons. Prioritising shared resources, collaborative decision-making, and equitable distribution of benefits, the ecological home challenges the principles of individual ownership and promotes collective management of resources to meet the needs of present and future generations sustainably. The use of the term holobiont, thereby provides a metaphor for ways of living that adopt a holistic approach to societal organisation, integrating economic, social, and ecological considerations to create resilient, regenerative communities and offering a transformative vision for inhabiting and co-creating our built environment, placing value on the diversity of human activities and the interconnectedness of all life.
3 Recommendations
Support from policymakers and industry leaders is crucial to encouraging the widespread adoption of earth-based materials along with understanding their role and context within a broader bio-inspired range of regenerative solutions for a “living” built environment. Incentives for sustainable construction practices, along with regulations that favour low-carbon materials, can accelerate the transition towards greener building methods.
The business case for sustainability in construction extends beyond environmental stewardship to offer significant economic benefits and competitive advantage (Revell and Blackburn, 2007), where innovative construction methods, include the integration of "softer" technologies and the use of earth-based materials, which contribute to ecological balance and can lead to substantial cost savings as well as increased asset value (Whelan and Fink, 2016). The broader uptake of self-repairing materials and smarter design presents a compelling financial incentive for companies and investors, where the shift towards sustainability can enhance a company’s reputation, attracting consumers and partners who prioritise environmental responsibility. This market differentiation can lead to increased sales, higher profitability, and access to new markets (Dyllick and Hockerts, 2002).
Furthermore, sustainable construction practices offer long-term benefits by future-proofing assets against the increasing regulations aimed at mitigating climate change impacts. Buildings that incorporate sustainable technologies and materials are likely to experience enhanced longevity, reduced operational costs, increased resilience to environmental changes, and offer an attractive nature-inspired aesthetics. This not only ensures compliance with evolving environmental standards but also positions companies as leaders in the green economy. Additionally, sustainable construction supports the creation of green jobs, contributing to a more robust and environmentally conscious economy. By aligning business strategies with sustainability principles, the construction industry can forge a path toward not only mitigating its environmental impact but also securing a sustainable and profitable future.
To substantiate the hypothesis on resilient regenerative design and implement measures for transformative change in the built environment, a comprehensive plan involving multiple stakeholders is essential. This plan should encompass research validation, practical measures, incentive frameworks, and policy recommendations:
1. Research and Validation: (a) Conduct extensive field studies and pilot projects to gather empirical evidence supporting the regenerative design hypothesis. (b) Partner with academic institutions and industry leaders to innovate and test novel living technologies and materials: To enhance the foundation of regenerative design, it is imperative to extend our exploration into rigorous empirical evidence through comprehensive field studies and pilot projects. This involves not only validating the theoretical underpinnings of our hypothesis but also examining the practical applicability and impact of regenerative design principles in diverse settings. Collaborations with academic institutions and industry leaders will serve as a catalyst for innovation, allowing for the development and testing of novel living technologies and materials. By grounding our approach in solid empirical research, we can ensure that regenerative design moves from a promising hypothesis to a proven framework for sustainable development.
2. Implementation Measures: (a) Develop guidelines and standards for regenerative design practices, ensuring they are adaptable to different geographic and socio-economic contexts. (b) Foster public-private partnerships to finance and support regenerative projects: Developing practical guidelines and standards for regenerative design is crucial for its widespread adoption. These guidelines must be adaptable to various geographic and socio-economic contexts, ensuring that regenerative principles can be integrated into a broad spectrum of projects. Additionally, fostering public-private partnerships will be key to financing and supporting regenerative projects. Through these partnerships, resources can be pooled to support the implementation of regenerative design in both public and private sectors, making sustainable development a shared responsibility and opportunity.
3. Incentive Frameworks: (a) Introduce financial incentives, such as tax rebates and grants, for projects that demonstrably adhere to regenerative design principles. (b) Create recognition programs to highlight exemplary projects and practices in resilient regenerative design: The establishment of financial incentives, such as tax rebates and grants, is vital to encourage the adoption of regenerative design principles. These incentives can significantly lower the barrier to entry for projects that aim to incorporate sustainable practices. Additionally, creating recognition programs to highlight and reward exemplary projects and practices in resilient regenerative design will serve to inspire innovation and commitment to sustainability within the industry. Such programs can also provide a platform for sharing best practices and lessons learned, further promoting the adoption of regenerative design.
4. Policy Recommendations: (a) Advocate for the integration of regenerative design principles into urban planning and building codes. (b) Lobby for policies that mandate the use of sustainable materials and technologies in new developments and major renovations: Advocating for the integration of regenerative design principles into urban planning and building codes is essential for ensuring that new developments and major renovations contribute positively to the environment. Lobbying for policies that mandate the use of sustainable materials and technologies will not only reduce the environmental impact of the construction industry but also promote the health and wellbeing of communities. By embedding regenerative design principles into policy, we can create a regulatory environment that supports sustainable development and drives the industry towards more innovative and eco-friendly practices.
By pursuing these recommendations, stakeholders can collaboratively advance the adoption of resilient regenerative design, creating built environments that are sustainable, adaptable, and harmonious with the natural world.
4 Discussion
Our vision responds to emerging demographics characterised by landscapes devastated by anthropogenic ecocide, characterised by significant biodiversity loss and the fragmentation of natural systems due to destructive building practices. Our living architecture and engineering concept can be positioned against the increasingly energy-dense requirements for building construction where the increasing reliance on fossil fuels for energy generation and building operations has had detrimental consequences for the environment, contributing to climate change, pollution, and resource depletion (Calder, 2022). Instead, we reimagine buildings and infrastructures as compatible with and integrated into natural processes, being dynamic complex entities (bodies, structures, systems, fabrics, etc.) like soils, with differing degrees of self-organisation, self-repair, distributed intelligences, low-power energy demands, and environmental adaptation. Accessing the spaces that link the mineral, organic, human and non-human realms have the potential to fundamentally transforming the nature of construction, performance, and maintenance. We envision this dynamic, life-enhancing, and adaptable built environment as being fundamentally regenerative (Armstrong, 2023c, 2023b; Armstrong R. 2023) using the “ecological intelligence” of microbes as building sensors and actuators enabling performances beyond current net-zero targets. Such site-specific, resource availability adjusted infrastructure benefit the local environment in ways that foster social sustainability and environmental resilience - the capacity to anticipate, prepare for, respond to, and recover from environmental and societal shifts (Walker et al., 2004). Our approach for design and engineering these dynamic responsive systems recognises the material transformation encoded in these platforms, and is deeply rooted in actor-network theory (Latour, 2007), new materialism (Dolphijn, 2021), and regenerative design principles (Petrovski et al., 2021; Soares and Puccinelli, 2023). Our methodology integrates the use of earth-bound materials and bioreceptive surfaces (Armstrong, 2023a), assembled under regenerative design principles, agentised via nature-based (or “living”) materiality and augmented by sophisticated computational models and cooperative governance structures (Bühler et al., 2021; Bühler et al., 2022; Bühler M. et al., 2023; Bühler et al., 2023b). Such a synthesis enables the creation of structures that are integrated within green and blue infrastructure (Andersson et al., 2019; Wuit et al., 2023) while also evolving in response to changing ecological dynamics and contributing to overall human wellbeing. Our goal is to develop environments that are not just inclusive and nurturing but also actively promote and enhance life for both humans and nature. The anticipated impact of this paradigm shift is profound, offering significant economic and ecological benefits that are augmented by the development and integration of smart, self-regulating systems. This convergence of matter and intelligence enables humans and nature to (re)negotiate the terms of inhabitation in ways that are attuned to a rapidly transitioning society to prioritise the symbiosis between human and natural ecosystems.
While the environmental benefits of EBMs are clear, architectural and construction challenges such as regulatory barriers, lack of standardisation, and perceptions of inferiority compared to conventional materials need to be addressed. Such standardizations may not be possible in the conventional way with formal benchmarks and targets but, owing to their contextualised nature, comprise tolerances, ranges, and recipes that enable multiple contingencies. The growing demand for sustainable construction presents significant opportunities for innovation, research, and development, specifically using advanced, predictive digital modelling techniques, as the basis of Digital Twins to significantly enhance sustainability, biodiversity, and socio-economic outcomes. These findings align with the broader field’s push towards more microbially-enabled, sustainable urban development practices, including novel biobased materials, bioelectrical systems and services, which presently offer a whole range of approaches to establish next-generation sustainable practices, and demonstrating the tangible benefits of adopting circular economy principles, sharing local knowledge and prototyping the incorporation of living technologies into major builds, e.g., BIQ building (BIQ, 2011).
The evolving paradigm in architecture towards sustainability, regenerative practices, and the integration of earth-based and bio-based materials, offer sustainable options with low embodied energy and carbon footprint, aligning with principles of regenerative architecture and providing new niches for microbial metabolisms that perform ecosystem services. These materials have a long history of use in construction and contribute to a holistic approach that respects the environment but the concepts for design and engineering need updating to embrace a more ecological sensibility and to inspire new imaginaries for architectural practices and to recognise their active contribution to healthier urban environments. As the demand for sustainable building practices grows, there is a need for a range of approaches beyond traditional earth-based materials that build upon their natural, life-promoting materiality and become sites for the construction of new urban microniches. Bio-based solutions, including microbial building materials, mycelium bio-composites (Haneef et al., 2017), and microbial fuel cells, offer innovative platforms that harness biological processes on various surfaces to provide real-time bioelectrical and metabolic data for bio-inspired building operations, paving the way for a more adaptive, resilient, and sustainable built environment (Fairus et al., 2022). To realise the full potential of earth-based materials and bio-based solutions in a next-generation regenerative architecture facilitated by microbial metabolisms, interdisciplinary collaboration is essential. Architects, engineers, biologists, and material scientists can work together to explore synergies between traditional and innovative materials and technologies. Earth-based materials can provide a solid foundation for structures, while bio-based solutions can enhance performance, efficiency, and sustainability. For example, integrating microbial fuel cells into earth-based buildings can enable energy generation from organic waste streams, turning buildings into active participants in the energy landscape, while also performing productive tasks such as bioremediation and biomass creation (Feng et al., 2008). Similarly, incorporating living’ bioelectrical mycelium bio-composites (Sydor et al., 2022) as insulation or structural components can not only enhance thermal performance and reduce environmental impact but could monitor and respond to thermal performance (Robertson et al., 2020). By combining the inherent qualities of earth-based and bio-based materials, architects can create buildings that not only minimise environmental impact but also contribute positively to ecosystem health and human wellbeing.
The synthesis of EBMs, bio-based materials, and nature-inspired building systems, emphasises the complementary nature of earth-based and bio-based materials in the context of regenerative architecture. By embracing both traditional wisdom, cutting-edge innovation, and the deep metabolic knowledge of microbes, architects can design buildings that are not only sustainable but also regenerative, fostering a synergistic relationship between human habitats and the natural world.
Unexpectedly, the economic benefits, including cost savings and job creation, are more pronounced than initially anticipated, suggesting that the economic viability of regenerative designs is perhaps currently underappreciated and undervalued especially from a conventional innovation pathway standpoint that focusses only on the proposed product gains, rather than the additional savings that accompany the breakthrough. This revelation underscores the potential for regenerative design to not only address environmental challenges but also serve as a catalyst for economic innovation.
However, the study faces limitations, including the scalability of pilot projects to larger urban contexts and the need for more longitudinal data to understand the long-term impacts of these designs. Future research should focus on overcoming these challenges, exploring the integration of technology in regenerative design, and further quantifying the economic implications to strengthen the case for widespread adoption.
5 Conclusion
The adoption of EBMs in construction offers a promising route to reducing the industry’s carbon footprint, enhancing sustainability, and combating climate change. By embracing these materials, and developing their potential to support bioremediating and productive metabolic exchanges with microbes, the construction industry can contribute to a livelier, more fertile and sustainable future. The integration of regenerative design principles, actor-network theory, and novel living technologies emerges as a powerful approach to reimagining urban spaces, where the integration of earth-based materials (EBMs) alongside biobased materials and nature-inspired systems represents a promising pathway towards achieving the Triple Transition—digital, green, and societal—across Europe. By combining traditional building practices with the innovative potential of biobased solutions, architects and policymakers can steer the continent towards a more sustainable and regenerative future.
Recommendations for implementing this integration include supporting interdisciplinary collaboration among architects, engineers, biologists, material scientists, and policymakers to enable the development of hybrid approaches that leverage the strengths of both EBMs and biobased materials, maximising sustainability, resilience, and efficiency in construction projects. Additionally, investments in research and development are crucial to advance the understanding and application of biobased materials and nature-based systems in architecture. By embracing the synergy between EBMs, biobased materials, and organic systems, Europe can lead the way in developing exemplary projects that are conducive with the New European Bauhaus triad of beautiful, sustainable and inclusive interventions, while also achieving the Triple Transition while promoting environmental stewardship, social equity, and economic prosperity for current and future generations.
Our findings illustrate significant advancements in sustainability metrics, biodiversity enhancement, and socio-economic benefits, marking a pivotal step forward in sustainable urban development. Future research should focus on scaling these initiatives, addressing long-term impacts, and further refining the integration of innovative technologies with the performance of natural systems. This endeavour not only contributes to the academic discourse but also provides a pragmatic roadmap for practitioners and policymakers aiming to foster resilient, life-affirming built environments.
Data availability statement
The raw data supporting the conclusion of this article will be made available by the authors, without undue reservation.
Author contributions
MB: Conceptualization, Methodology, Visualization, Writing–original draft, Writing–review and editing. PH: Investigation, Resources, Validation, Writing–review and editing. LK: Funding acquisition, Methodology, Resources, Writing–review and editing. RA: Conceptualization, Data curation, Formal Analysis, Funding acquisition, Investigation, Methodology, Resources, Supervision, Validation, Writing–original draft, Writing–review and editing.
Funding
The authors declare that financial support was received for the research, authorship, and/or publication of this article. The hypotheses presented in this work emerged from joint collaborative discussions during the proposal writing phase for an EU project. The article processing charges (APC) were fully funded by the German Science Foundation (DFG), the Baden-Württemberg Ministry of Science, Research and Culture (Ministerium für Wissenschaft, Forschung und Kunst Baden-Württemberg) and the University of Applied Sciences Konstanz (HTWG Hochschule Konstanz Technik, Wirtschaft und Gestaltung). Living Architecture is Funded by the EU Horizon 2020 Future Emerging Technologies Open programme (2016–2019) Grant Agreement 686585 a consortium of six collaborating institutions—Newcastle University, University of Trento, University of the West of England, Spanish National Research Council, Explora Biotech and Liquifer Systems Group. The Active Living Infrastructure: Controlled Environment (ALICE) project is funded by an EU Innovation Award for the development of a bio-digital “brick” prototype, a collaboration between Newcastle University, Translating Nature, and the University of the West of England (2019–2021) under EU Grant Agreement no. 851246. Microbial Hydroponics (Mi-Hy) is funded by an EIC Pathfinder Open award for the development of a next-generation hydroponics system with a prosthetic rhizome, which is a collaboration between KU Leuven (Belgium), the University of Southampton (UK), Sony Computer Science Laboratory (Paris), the Spanish National Research Council (Spain), Biofaction (Austria) and the University of the West of England (UK).
Conflict of interest
Authors MB, PH, and LK were employed by GemeinWerk Ventures GmbH.
The remaining author declares that the research was conducted in the absence of any commercial or financial relationships that could be construed as a potential conflict of interest.
Publisher’s note
All claims expressed in this article are solely those of the authors and do not necessarily represent those of their affiliated organizations, or those of the publisher, the editors and the reviewers. Any product that may be evaluated in this article, or claim that may be made by its manufacturer, is not guaranteed or endorsed by the publisher.
References
Adamatzky, A., Gandia, A., Ayres, P., and Wösten, H. (2021). Adaptive fungal architectures. Available at: https://www.researchgate.net/profile/Phil-Ayres-2/publication/348937077_Adaptive_Fungal_Architectures/links/60181ab3299bf1b33e3dd573/Adaptive-Fungal-Architectures.pdf.
Agamben, G. (1998) Homo sacer: sovereign power and bare life. Redwood City, California, United States: Stanford University Press.
ALICE (2019). Active Living Infrastructure: controlled Environment ALICE. “living” installation that communicates with microbes in real time by monitoring their electricity production so we can “respond” to them by feeding them with our liquid waste. Available at: https://alice-interface.eu.
Andersson, E., Langemeyer, J., Borgström, S., McPhearson, T., Haase, D., Kronenberg, J., et al. 2019. “Enabling green and blue infrastructure to improve contributions to human well-being and equity in urban systems.” , 69, 566, 574. doi:10.1093/biosci/biz058
Armstrong, R. (2015). Innovation and technical transformations in living technology: an entanglement of agentised matter, ANT, and natural computing. Int. J. Actor-Network Theory Technol. Innovation (IJANTTI) 7 (1), 18–42. doi:10.4018/ijantti.2015010103
Armstrong, R. (2023a). Towards the microbial home: an overview of developments in next-generation sustainable architecture. Microb. Biotechnol. 16, 1112–1130. doi:10.1111/1751-7915.14256
Armstrong, R. (2023b). Towards the microbial home: an overview of developments in next-generation sustainable architecture. Microb. Biotechnol. 16 (6), 1112–1130. doi:10.1111/1751-7915.14256
Armstrong, R. (2023c). Introducing regenerative architecture. J. Chin. Archit. Urbanism 0, 1882. doi:10.36922/jcau.1882
Armstrong, R. (2023d). Deanthropocentrising the Microbial Commons. lirias.kuleuven.be. Available at: https://lirias.kuleuven.be/4064397?limo=0.
Armstrong, R., Ferracina, S., Caldwell, G., Ieropoulos, I., Rimbu, G., Adamatzky, A., et al. (2017). Living architecture (LIAR): metabolically engineered building units. Available at: https://eprints.ncl.ac.uk/232922.
ARUP (2022). Solar leaf, Hamburg. Worldwide first façade system to cultivate micro-algae to generate heat and biomass as renew- able energy sources. Available at: https://www.arup.com/projects/solar-leaf.
Babauta, J., Renslow, R., Lewandowski, Z., and Beyenal, H. (2012). Electrochemically active biofilms: facts and fiction. A review. Biofouling 28 (8), 789–812. doi:10.1080/08927014.2012.710324
Barad, K. M. (2007) Meeting the universe halfway: quantum physics and the entanglement of matter and meaning. Durham: Duke University Press.
Bennett, J., Cheah, P., Orlie, M. A., and Grosz, E. (2010). New materialisms: ontology, agency, and politics. Available at: https://books.google.com/books?hl=en\&lr=\&id=UFhBBKKTkMoC\&oi=fnd\&pg=PR7\&dq=new+materialism\&ots=D2WW7-PuTD\&sig=46tXmeruMb9yj2txY37dYcmy3mY.
Bhina, M. R., Wibowo, A. H., Liu, K. Y., Khan, W., and Salim, M. (2023). Microbes in bioconcrete technology: exploring the fundamentals and state-of-the-art findings for advancing civil engineering. J. Chin. 46, 726–736. doi:10.1080/02533839.2023.2238789
BIQ (2011). Smart material houses. Available at: https://www.internationale-bauausstellung-hamburg.de/en/projects/the-building-exhibition-within-the-building-exhibition/smart-material-houses/biq/projekt/biq.html.
Bloom, D. E., and Canning, D. (2004). Global demographic change: dimensions and economic significance. nber.org. Available at: https://www.nber.org/papers/w10817.
Blute, M. (2010). Myra J. Hird. The origins of sociable life: evolution after science studies. New York: palgrave macmillan, 2009, 260pp. $US 85.00 hardcover (978-0-230-20213-9). Can. J. Sociol. 34 (4), 1161–1165. doi:10.29173/cjs6800
Bond, D. R., and Lovley, D. R. (2003). Electricity production by geobacter sulfurreducens attached to electrodes. Appl. Environ. Microbiol. 69 (3), 1548–1555. doi:10.1128/AEM.69.3.1548-1555.2003
Braungart, M., McDonough, W., and Bollinger, A. (2007). Cradle-to-Cradle design: creating healthy emissions – a strategy for eco-effective product and system design. J. Clean. Prod. 15 (13–14), 1337–1348. doi:10.1016/j.jclepro.2006.08.003
Bühler, M., Hollenbach, P., Michalski, A., Meyer, S., Birle, E., Off, R., et al. (2023a). The industrialisation of sustainable construction: a transdisciplinary approach to the large-scale introduction of compacted mineral mixtures (CMMs) into building construction. Sustainability 15, 10677. doi:10.3390/su151310677
Bühler, M. M., Calzada, I., Cane, I., Jelinek, T., Kapoor, A., Mannan, M., et al. (2023b). Unlocking the power of digital commons: data cooperatives as a pathway for data sovereign, innovative and equitable digital communities. Available at: https://www.mdpi.com/2673–6470/3/3/11.
Bühler, M. M., Jelinek, T., and Nübel, K. (2022). Training and preparing tomorrow’s workforce for the fourth industrial revolution. Educ. Sci. 12 (11), 782. doi:10.3390/educsci12110782
Bühler, M. M., Nübel, K., Jelinek, T., Riechert, D., Bauer, T., Schmid, T., et al. (2023c). Data cooperatives as a catalyst for collaboration, data sharing and the digital transformation of the construction sector. Build. (Basel). 13, 442. doi:10.3390/buildings13020442
Bühler, M. M., Sebald, C., Rechid, D., Baier, E., Michalski, A., Rothstein, B., et al. (2021). Application of copernicus data for climate-relevant urban planning using the example of water, heat, and vegetation. Remote Sens. (Basel). 13, 3634. doi:10.3390/rs13183634
Calder, B. (2022) Architecture: from prehistory to climate emergency. London: Pelican: Pelican Books.
Chang, I., Jeon, M., and Cho, G. C. (2015). Application of microbial biopolymers as an alternative construction binder for earth buildings in underdeveloped countries. Int. J. Polym. Sci. 2015, 1–9.
D’Orazio, M., Cursio, G., Graziani, L., Aquilanti, L., Osimani, A., Clementi, F., et al. (2014). Effects of water absorption and surface roughness on the bioreceptivity of ETICS compared to clay bricks. Build. Environ. 77, 20–28. doi:10.1016/j.buildenv.2014.03.018
Dulon, S., Parot, S., Delia, M. L., and Bergel, A. (2007). Electroactive biofilms: new means for electrochemistry. J. Appl. Electrochem. 37, 173–179. doi:10.1007/s10800-006-9250-8
Dyllick, T., and Hockerts, K. (2002). Beyond the business case for corporate sustainability. Bus. Strategy Environ. 11, 130–141. doi:10.1002/BSE.323
EIC (2023). Architecture, engineering and construction digitalisation for a novel triad of design, fabrication, and materials. Available at: https://eic.ec.europa.eu/calls-tenders/architecture-engineering-and-construction-digitalisation-novel-triad-design-fabrication-and_en.
Elbert, W., Bettina, W., Susannah, B., Jörg, S., Burkhard, B., Andreae, M. O., et al. (2012). Contribution of cryptogamic covers to the global cycles of carbon and nitrogen. Nat. Geosci. 5 (7), 459–462. doi:10.1038/ngeo1486
Fairus, M., Bahrin, E. K., Arbaain, E. N. N., and Ramli, N. (2022). Mycelium-based composite: a way forward for renewable material. J. Sustain. Sci. 17, 271–280. doi:10.46754/jssm.2022.01.018
Feng, Y., Xin, W., Logan, B. E., and Lee, He (2008). Brewery wastewater treatment using air-cathode microbial fuel cells. Appl. Microbiol. Biotechnol. 78 (5), 873–880. doi:10.1007/s00253-008-1360-2
Freeman, C., Fenner, N., and Shirsat, A. H. (1974). Peatland geoengineering: an alternative approach to terrestrial carbon sequestration. Phys. Eng. Sci. 370, 4404–4421. doi:10.1098/rsta.2012.0105
Garg, R., Garg, R., and Eddy, N. O. (2023). Microbial induced calcite precipitation for self-healing of concrete: a review. Sustain. Cement-Based Mater. 12, 317–330. doi:10.1080/21650373.2022.2054477
Gasteiger, H. A., Wenbin, Gu, Rohit, M., Mathias, M. F., and Sompalli, B. (2010). Beginning-of-life MEA performance — efficiency loss contributions. Available at: https://api.semanticscholar.org/CorpusID:94572357.
Gorby, Y. A., Yanina, S., McLean, J. S., Rosso, K. M., Moyles, D., Alice Dohnalkova, T., et al. (2006). Electrically conductive bacterial nanowires produced by shewanella oneidensis strain MR-1 and other microorganisms. Proc. Natl. Acad. Sci. 103 (30), 11358–11363. doi:10.1073/pnas.0604517103
Goubran, S., Walker, T., Cucuzzella, C., and Schwartz, T. (2023). Green building standards and the united Nations’ sustainable development goals. J. Environ. 326, 116552. doi:10.1016/j.jenvman.2022.116552
Guchte, V.De, Blottière, H. M., and Doré, J. (2018). Humans as holobionts: implications for prevention and therapy. Microbiome 6 (1), 81. doi:10.1186/s40168-018-0466-8
Haneef, M., Luca, C., Claudio, C., Ilker, S. B., José, A.H.-G., and Athanassiou, A. (2017). Advanced materials from fungal mycelium: fabrication and tuning of physical properties. Sci. Rep. 7 (1), 41292. doi:10.1038/srep41292
Haraway, D. (1991). “A cyborg manifesto: science, technology, and socialist-feminism in the late twentieth Century,” in Simians, cyborgs and women: the reinvention of nature (London, United Kingdom: Routledge).
Henderson, L. J. (1913). “The fitness of the environment, an inquiry into the biological significance of the properties of matter,” in Part Delivered as lectures in the lowell institute (New York: Macmillan). doi:10.5962/bhl.title.21049
Hird, M. J. (2009) The origins of sociable life: evolution after science studies. Online access with purchase: palgrave connect. London, United Kingdom: Palgrave Macmillan.
Jacobs, J. (2016). The economy of cities. Available at: https://books.google.com/books?hl=en\&lr=\&id=OaydDAAAQBAJ\&oi=fnd\&pg=PA3\&dq=%22jane+jacobs%22\&ots=G4uEoUAV3Q\&sig=3ULLYAfygZo3oNsHfCtoXw0bmfA.
Jain, S., Fang, C., and Achal, V. (2021). “A critical review on microbial carbonate precipitation via denitrification process in building materials,” in Bioengineered (Boca Raton, Florida, United States: Taylor & Francis). doi:10.1080/21655979.2021.1979862
Jones, R. J., Delesky, E. A., Cook, S. M., and Cameron, J. C. (2022). “Engineered living materials for construction,” in Engineered living. doi:10.1007/978-3-030-92949-7_7
Jonkers, H. M., and Schlangen, E. (2008). Development of a bacteria-based self healing concrete. Available at: https://www.researchgate.net/profile/Erik-Schlangen/publication/267716612_Development_of_a_bacteria-based_self_healing_concrete/links/54983d0a0cf2519f5a1dda63/Development-of-a-bacteria-based-self-healing-concrete.pdf.
Joshi, S., Goyal, S., Mukherjee, A., and Reddy, M. (2017). Microbial healing of cracks in concrete: a review. J. Ind. Microbiol. Biotechnol. 44, 1511–1525. doi:10.1007/s10295-017-1978-0
Kato, S., Hashimoto, K., and Watanabe, K. (2012). Microbial interspecies electron transfer via electric currents through conductive minerals. Proc. Natl. Acad. Sci. U. S. A. 109, 10042–10046. doi:10.1073/pnas.1117592109
Kembel, S. W., Jones, E., Kline, J., Dale, N., Jason, S., Ann, M. W., et al. (2012). Architectural design influences the diversity and structure of the built environment microbiome. ISME J. 6 (8), 1469–1479. doi:10.1038/ismej.2011.211
King, G. M. (2014). Urban microbiomes and urban ecology: how do microbes in the built environment affect human sustainability in cities? J. Microbiol. 52 (9), 721–728. doi:10.1007/s12275-014-4364-x
Kirchherr, J., Reike, D., and Hekkert, M. (2017). Conceptualizing the circular economy: an analysis of 114 definitions. SSRN J., doi:10.2139/ssrn.3037579
Koolhaas, R., Obrist, H. U., Ota, K., Westcott, J., and Daniell, T. (2011). Project Japan: metabolism talks. Available at: https://www.academia.edu/download/64738174/B01_074_Review2_Rem_Koolhaas.pdf.
Latour, B. (2007). Reassembling the social: an introduction to actor-network-theory. Available at: https://books.google.com/books?hl=en\&lr=\&id=BgJREAAAQBAJ\&oi=fnd\&pg=PR7\&dq=%22actor+network%22+theory\&ots=Z7dy3BAJQh\&sig=d7XCaQgLZ6O_T-ZZEYDv-r0o6Bs.
Linnaeus Online (2024). LINNAEUS ONLINE: the linnaean gardens of uppsala. Available at: https://www.botan.uu.se/learning/linnaeus-online.
Logan, B. E. (2005). Simultaneous wastewater treatment and biological electricity generation. Water Sci. Technol. 52, 31–37. doi:10.2166/wst.2005.0495
Logan, W. B. (2007). Dirt: the ecstatic skin of the earth. WW norton. Available at: https://books.google.de/books?id=bVMsAAAAYAAJ.
Mahrous, R., Giancola, E., Osman, A., Asawa, T., and Mahmoud, H. (2022). Review of key factors that affect the implementation of bio-receptive façades in a hot arid climate: case study north Egypt. Build. Environ. 214, 108920. doi:10.1016/j.buildenv.2022.108920
Marsili, E., Sun, J., and Bond, D. R. (2010). Voltammetry and growth physiology of Geobacter sulfurreducens biofilms as a function of growth stage and imposed electrode potential. Electroanalysis 22, 865–874. doi:10.1002/elan.200800007
Mathis, B. J., Marshall, C. W., Milliken, C. E., Makkar, R. S., Creager, S. E., and May, H. D. (2008). Electricity generation by thermophilic microorganisms from marine sediment. Appl. Microbiol. Biotechnol. 78, 147–155. doi:10.1007/s00253-007-1266-4
Menges, A. (2012). Material computation: higher integration in morphogenetic design. Available at: https://books.google.com/books?hl=en\&lr=\&id=PC7TUfXH4XEC\&oi=fnd\&pg=PA6\&dq=%22achim+menges%22\&ots=3kVS7PVGTF\&sig=9DU7I2vKRoMzvtW9aphncynbv58.
Miller, A. Z., Sanmartín, P., Pereira-Pardo, L., Dionísio, A., Saiz-Jimenez, C., Macedo, M. F., et al. (2012). Bioreceptivity of building stones: a review. Sci. Total Environ. 426, 1–12. doi:10.1016/j.scitotenv.2012.03.026
Mojzsis, S. J., Arrhenius, G., McKeegan, K. D., Harrison, T. M., Nutman, A. P., and Friend, C. R. (1996). Evidence for life on earth before 3,800 million years ago. Nature 384, 55–59. doi:10.1038/384055a0
Montgomery, M. R., Stren, R., Cohen, B., and Reed, H. E. (2013). Cities transformed: demographic change and its implications in the developing world. Available at: https://books.google.com/books?hl=en\&lr=\&id=8dneAQAAQBAJ\&oi=fnd\&pg=PP1\&dq=demographic+changes\&ots=Vc4JpKm1St\&sig=eHNF13L8fTYy1ox_Rf-TYfDp1X4.
Mustafa, , Fahriba, K., Prieto, A., and Ottele, M. (2021). The role of geometry on a self-sustaining bio-receptive concrete panel for facade application. Sustainability 13 (13), 7453. doi:10.3390/su13137453
Nevin, K. P., Richter, H., Covalla, S. F., Johnson, J. P., Woodard, T. L., Orloff, A. L., et al. (2008). Power output and columbic efficiencies from biofilms of geobacter sulfurreducens comparable to mixed community microbial fuel cells. Environ. Microbiol. 10 (10), 2505–2514. doi:10.1111/j.1462-2920.2008.01675.x
Ökobaudat, (2024). Informationsportal nachhaltiges bauen—database of sustainable construction. Available at: https://www.oekobaudat.de.
Petrovski, A., Pauwels, E., and Gonzalez, A. G. (2021). Implementing regenerative design principles: a refurbishment case study of the first regenerative building in Spain. Sustainability 13, 2411. doi:10.3390/SU13042411
Potter, M. C. (1911). Electrical effects accompanying the decomposition of organic compounds. Proc. R. Soc. Lond. Ser. b, Contain. Pap. a Biol. Character 84 (571), 260–276. doi:10.1098/rspb.1911.0073
Priavolou, C., and Niaros, V. (2019). Assessing the openness and conviviality of open source technology: the case of the WikiHouse. Sustain. Multidiscip. Digit. Publ. Inst. 11, 4746. doi:10.3390/su11174746
Price, C., and Hardingham, S. (1984). Cedric Price. Available at: https://www.spatialagency.net/database/price.
Rabaey, K., and Verstraete, W. (2005). Microbial fuel cells: novel biotechnology for energy generation. Trends Biotechnol. 23 (6), 291–298. doi:10.1016/j.tibtech.2005.04.008
Rafsanjani, H. N., and Nabizadeh, A. H. (2023). “Towards digital architecture, engineering, and construction (AEC) industry through virtual design and construction (VDC) and digital Twin,” in Energy and built environment (Amsterdam, Netherlands: Elsevier).
Rahman, , Mazedur, Al, Bhardwaj, A., Pei, Z., Ufodike, C., and Castell-Perez, E. (2022). The 3D printing of biomass–fungi composites: effects of waiting time after mixture preparation on mechanical properties, rheological properties, minimum extrusion pressure, and print quality of the prepared mixture. J. Compos. Sci. 6 (8), 237. doi:10.3390/jcs6080237
Reichert, S., Menges, A., and Correa, D. (2015). Meteorosensitive architecture: biomimetic building skins based on materially embedded and hygroscopically enabled responsiveness. Computer-Aided Des. 60, 50–69. doi:10.1016/j.cad.2014.02.010
Revell, A., and Blackburn, R. (2007). The business case for sustainability? An examination of small firms in the UK’s construction and restaurant sectors. Bus. Strategy Environ. 16, 404–420. doi:10.1002/BSE.499
Robertson, O., Høgdal, F., Mckay, L., and Lenau, T. (2020). Fungal future: a review of mycelium biocomposites as an ecological alternative insulation material. Balancing Innovation Operation. Des. Soc., doi:10.35199/NORDDESIGN2020.18
Rosenbaum, M. A., and Franks, A. E. (2014). Microbial catalysis in bioelectrochemical technologies: status quo, challenges and perspectives. Appl. Microbiol. Biotechnol. 98, 509–518. doi:10.1007/s00253-013-5396-6
Salifu, E., Gutteridge, F., and Witte, K. (2021). Recent advances in engineered microbial technologies for the construction industry. Young Researchers’ Forum V., Available at: https://strathprints.strath.ac.uk/76352/.
Sarkar, M., Maiti, M., Mandal, S., and Xu, S. (2023). Enhancing concrete resilience and sustainability through fly ash-assisted microbial biomineralization for self-healing: from waste to greening construction materials. Chem. Eng. J. 481, 148148. doi:10.1016/j.cej.2023.148148
Schalk, M. (2014). The architecture of metabolism. Inventing a culture of resilience. Arts 3, 279–297. doi:10.3390/arts3020279
Sender, R., Fuchs, S., and Milo, R. (2016). Are we really vastly outnumbered? Revisiting the ratio of bacterial to host cells in humans. Cell 164 (3), 337–340. doi:10.1016/j.cell.2016.01.013
Sharma, T. K., Alazhari, M., Heath, A., Paine, K., and Cooper, R. M. (2017). Alkaliphilic Bacillus species show potential application in concrete crack repair by virtue of rapid spore production and germination then extracellular calcite formation. J. Appl. Microbiol. 122 (5), 1233–1244. doi:10.1111/jam.13421
Shi, S., and Xu, G. (2019). Identification of phosphorus fractions of biofilm sludge and phosphorus release, transformation and modeling in biofilm sludge treatment related to pH. Chem. Eng. J. 369, 694–704. doi:10.1016/j.cej.2019.03.120
Shittu, E., Tibrewala, A., Kalla, S., and Wang, X. (2021). Meta-analysis of the strategies for self-healing and resilience in power systems. Adv. Appl. Energy 4, 100036.
Soares, S., and Puccinelli, E. 2023. “Material futures: an introduction of regenerative design principles to product design students.”
Song, T., Jiang, B., Li, Y., Ji, Z., Zhou, H., Jiang, D., et al. (2021). Self-healing materials: a review of recent developments. doi:10.30919/esmm5f465
Stohl, L., Manninger, T., Von Werder, J., Dehn, F., Gorbushina, A., and Meng, B. 2023. “Bioreceptivity of concrete: a review.” , 76, 107201.
Storefront (2023). Storefront for art and architecture. (2019) subculture: microbial metrics and the multi-species city. Available at: http://storefrontnews.org/programming/subculture-microbial-metrics-and-the-multi-species-city/.
Sydor, M., Cofta, G., Doczekalska, B., and Bonenberg, A. (2022). Fungi in mycelium-based composites: usage and recommendations. Materials 15, 6283. Available at:. doi:10.3390/ma15186283
Tamari, T. (2014). Metabolism: utopian urbanism and the Japanese modern architecture movement. Theory, Cult. &Society 31, 201–225. doi:10.1177/0263276414547777
The Human Microbiome Project Consortium (2012). Structure, function and diversity of the healthy human microbiome. Nature 486 (7402), 207–214. doi:10.1038/nature11234
Timmis, K., Ricardo, C., José, L. G., Balbina, N., Max, C., Lisa, S., et al. (2019). The urgent need for microbiology literacy in society. Environ. Microbiol. 21 (5), 1513–1528. doi:10.1111/1462-2920.14611
Turner, C. J., Oyekan, J., and Stergioulas, L. (2020). Utilizing industry 4.0 on the construction site: challenges and opportunities. Available at: https://ieeexplore.ieee.org/abstract/document/9117064/.
Walker, B., Crawford, S. H., Stephen, R. C., and Kinzig, A. (2004). Resilience, adaptability and transformability in social–ecological systems. Ecol. Soc. 9 (2), art5. doi:10.5751/es-00650-090205
Walter, , Alexis, X., Merino-Jiménez, I., Greenman, J., and Ieropoulos, I. (2018). PEE POWER® urinal II – urinal scale-up with microbial fuel cell scale-down for improved lighting. J. Power Sources 392, 150–158. doi:10.1016/j.jpowsour.2018.02.047
Wei-Haas, M. (2016). Life and rocks may have Co-evolved on earth, january 13. Available at: https://www.smithsonianmag.com/science-nature/life-and-rocks-may-have-co-evolved-on-earth-180957807/.
Whelan, T., and Fink, C. (2016). The comprehensive business case for sustainability. Harv. Bus. Rev., Available at: https://everestenergy.nl/new/wp-content/uploads/HBR-Article-The-comprehensive-business-case-for-sustainability.pdf.
Wuit, Y. K., Hnin, A. C., Jocelyne, H., Michael, B., Johannes, H., and Michael, H. (2023). Multifactorial evaluation of spatial suitability and economic viability of light green bridges using remote sensing data and spatial urban planning criteria. Remote Sens. 15 (3), 753. doi:10.3390/rs15030753
Yang, Q., Yang, D., Li, P., Liang, S., and Zhang, Z. (2021). Resilient city: a bibliometric analysis and visualization. Discrete Dyn. Nat. Soc. 2021, 1–17. doi:10.1155/2021/5558497
You, J., Arjuna, M., John, G., Julie, F., Stephen, W., Armstrong, R., et al. (2022). Development of a bio-digital interface powered by microbial fuel cells. Sustainability 14 (3), 1735. doi:10.3390/su14031735
Keywords: regenerative design, resilient systems, bioelectricity, new materialism, environmental and climate adaptation, computational modelling, earth based materials, microbes
Citation: Bühler M, Hollenbach P, Köhler L and Armstrong R (2024) Unlocking resilience and sustainability with earth-based materials: a principled framework for urban transformation. Front. Built Environ. 10:1385116. doi: 10.3389/fbuil.2024.1385116
Received: 11 February 2024; Accepted: 30 April 2024;
Published: 04 July 2024.
Edited by:
Shabtai Isaac, Ben-Gurion University of the Negev, IsraelReviewed by:
Mariarosaria Angrisano, Pegaso University, ItalyMarta Maria Sesana, University of Brescia, Italy
Copyright © 2024 Bühler, Hollenbach, Köhler and Armstrong. This is an open-access article distributed under the terms of the Creative Commons Attribution License (CC BY). The use, distribution or reproduction in other forums is permitted, provided the original author(s) and the copyright owner(s) are credited and that the original publication in this journal is cited, in accordance with accepted academic practice. No use, distribution or reproduction is permitted which does not comply with these terms.
*Correspondence: Michael Bühler, bWJ1ZWhsZXJAaHR3Zy1rb25zdGFuei5kZQ==