- 1Department of Architecture, Aalto University, Otakaari, Finland
- 2Department of Mechanical and Materials Engineering, University of Turku, Turku, Finland
This study focuses on finding suitable installation sites for vertical bifacial photovoltaic (VBPV) panels in urban low-rise neighborhoods at high latitudes. The power production of east-west-oriented VBPV systems matches well with domestic electricity consumption profiles, increasing the self-consumption of PV electricity. Furthermore, PV electricity adds economic value by avoiding transmission fees and taxes. These systems are especially beneficial in high-latitude locations characterized by a low solar elevation angle. However, these low angles expose VBPV panels to a high risk of shading losses from their surroundings, and it is unknown how much shading limits the number of suitable installation sites. Here, environmental shading on VBPV panels is quantified for three low-rise residential neighborhoods in Helsinki, a high-latitude location, by comparing the specific yields (annual electricity production per kilowatt peak) of VBPV and monofacial PV (MPV) systems. The results showed that unshaded VBPV systems have a higher specific yield than their MPV counterparts. However, in densely built neighborhoods with tall trees, the lack of suitable installation sites for VBPV panels severely limits the peak power of these systems. Roof ridge VBPVs usually yield high production, while façade- and ground-mounted systems lose between 30% and 70% compared to roof ridge VBPV systems depending on their installation locations. South-oriented MPVs perform better than VBPVs on north-south-facing roofs, both in terms of specific yield and total annual production. Conversely, VBPVs installed on the ridges of unshaded roofs aligned closely with the north-south axis outperform MPVs on east- and west-facing roofs by 20%–30%.
Introduction
Transitioning toward renewable energy sources has been on the agendas of many countries in recent years. One of the most prominent motivations for this transition is the climate crisis caused by anthropogenic activities. The objective of limiting the global average temperature increase to below 1.5°C–2°C compared to pre-industrial levels has been part of development plans in many countries. However, although it is attempting to limit global greenhouse gas emissions, the energy sector’s current transitioning plan will fails to limit the global temperature increase to below 1.5° (Shukla, 2022). The novel application of photovoltaic (PV) panels as a renewable energy source can help reduce greenhouse gas emissions from energy production process.
Finland is among the countries with the most ambitious climate change mitigation plans. The country aims to significantly reduce domestic greenhouse gas emissions and plans to reach carbon neutrality by 2035. Its installed capacity for PV panels is currently negligible (International Energy Agency, 2023). However, the installed capacity of PV panels in Finland has been rising rapidly in recent years, and solar energy is among Finland’s fastest-growing renewable energy sources. Evidence of this growth can be seen in the funding support that solar energy projects have received in Finland. From 2018 to 2022, solar PVs received the highest funding. The increase in PV capacity is expected to reach 5.3 GW by 2030, a significant increase from 1 GW in 2022 (International Energy Agency, 2023). Given current investments in PV installation but the low installed capacity of PVs, the novel application of PVs panels suitable to the geographic location of Finland has opportunities to be developed and established.
Literature
Solar angle is one of the major factors of consideration in PV installations in northern latitudes. Nonetheless, with proper installation techniques, low solar elevation angles can be leveraged to increase the energy yield of PV devices. For building-applied PVs (BAPVs) and building-integrated PVs (BIPVs), roofs are the most common locations for the installation of conventionally mounted PVs, while façades are a common location for the installation of vertically mounted PV panels. However, façade PVs experience a greater risk of shading from the surrounding environment. Lobaccaro et al. (2017) studied PV utilization in a neighborhood in Trondheim, Norway. Their results showed that, depending on the neighborhood, 22%–50% of the buildings’ façades were suitable for PV installation (Lobaccaro et al., 2017). This number indicates that PV installation on façades require pre-planning to avoid significant shading from the surroundings.
Although they have a high risk of shading loss, vertically mounted PVs offer many benefits when installed in northern latitudes. Good et al. (2014) compared façade and roof PV systems in Norway. The energy yield of façade-mounted PV systems was found to be lower than that of roof-mounted systems, but the peak energy production shifted from summer to spring and autumn. This shift is beneficial since electricity usage is higher in spring and autumn than in summer, thus making more electricity available for self-consumption (Good et al., 2014).
Another technology that benefits significantly from a low solar elevation angle is vertically mounted bifacial PVs (VBPVs). Bifacial PV panels utilize both their front and back sides to generate electricity. Although newer and less studied compared to conventional monofacial PV (MPV) panels, bifacial PV panels are considered a mature technology. Although they can be mounted conventionally, VBPVs can be installed vertically and have high electricity yields (Guerrero-Lemus et al., 2016) Guo et al. (2013) found that VBPVs performed better than MPVs in situations with a high diffuse fraction, high albedo, or high latitudes. Other studies have shown that the profitability of VBPV systems increased when they were installed in locations far away from the equator (Rodríguez-Gallegos et al., 2018).
It is important to note that snow coverage leads to an exceptionally high albedo (Brennan et al., 2014), increasing the electricity production of VBPVs during the winter in the Nordic countries. Additionally, vertical surfaces gather less snow and different types of soiling compared to horizontal surfaces and yield higher overall production without needing regular cleaning compared to conventional MPVs.
The peak electricity production of VBPVs also differs from that of MPVs. Jouttijarvi et al. (2023) showed that east-west facing VBPVs installed in southern Finland had higher production than MPVs and improved temporal matching with household electricity consumption. The temporal match of PV power production with peak electricity demand is important in increasing self-consumption, which is an important aspect of solar energy proliferation (Jouttijärvi et al., 2023). Self-consumption increases the economic value of PV electricity because transmission fees and taxes can be avoided and dependency on the grid can be reduced. However, the excessive production of PV power has, in some locations, dampened the electricity price around noon, increasing the payback time of PV modules and discouraging the adoption of PVs (Tselika, 2022). The combination of MPV and VBPV systems can spread out energy production peaks and avoid flooding the electricity market (Freitas and Brito, 2019).
Furthermore, Baumann et al. (2019) pointed out that each array of VBPVs may shade its neighboring panels and require ample space to avoid electricity loss from shading. The space requirement is much higher for VBPV than for MPV systems. However, due to their geometry, the area between each VBPV array is accessible, unlike for MPV arrays. In Baumann et al.'s (2019) study, the area between arrays was utilized as a roof garden. Other studies have simulated PVs using digital models without any surroundings or calculating VBPV power production from actual devices in ideal situations without considerable shading from surroundings (Guo et al., 2013; Baumann et al., 2019; Jouttijärvi et al., 2023). Currently, the installation of VBPVs on a commercial scale is still underdeveloped. Therefore, real-world case studies of the effects of shading on VBPVs are also unavailable.
Goal and scope
Contributing to the understanding of VBPV performance under the influence of shading from the Finnish urban environment is the goal of this study. This research focused on the performance of east-west-facing VBPV systems and compares them to MPV systems, focusing especially on VBPV systems in urban environments, an understudied but important and timely topic. Since the peak production of this technology matches the hours of peak electricity consumption for residential usage, this study focused on VBPV applications in residential buildings. Among the residential building types in Finland, small-scale houses occupy a significant share of the built area in Finland; therefore, this building type was chosen for the case studies (Statistics Finland, 2023).
Even though VBPV technology has the potential to be suitable in Finland, the effect of shading from the Finnish environment needs to be understood before this technology can be applied. The key indicator is specific yield (i.e., the total annual electricity production per kilowatt peak). This indicator allows for the efficient comparison of different PV systems with each other and with a rooftop monofacial PV system, identifying which locations are feasible for VBPV installation. The economic feasibility of VBPV systems in different sites can be estimated by comparing their specific yields to those of MPV systems in corresponding locations. However, the higher costs of VBPV technologies and those of supporting structures and installations, which have yet to be quantified in this context, are outside the scope of this study, as the focus was on energy production.
Helsinki was chosen as the preferred city in this study, since it has diverse types of neighborhoods, from relatively dense areas with little surrounding vegetation to groups of houses built near woodlands. The diversity of neighborhoods is important, since different environments cause different form of shading on PVs. In this contribution, three case study sites were selected for analysis. The sites are located in Helsinki, Finland, and represent different neighborhood typologies. The case study sites were selected for their representativeness of the common existing residential neighborhoods and the current trends in the design of residential areas. These chosen sites provided varying conditions in terms of shading, the distance between buildings, the amount of vegetation and vegetation height. Additionally, an investigation to find suitable locations to install VBPVs on buildings or inside building properties was part of this study. East-west VBPVs were simulated on building roofs, on building facades and on the ground. Different installation locations can be differently affected by shading; therefore, it is important to diversify the locations of PV installations.
Case studies and methods
Description of case studies
Case study sites in Helsinki
A type-house building was used for the analysis of each site. Since the geometry of buildings can affect shading considerably, making it difficult to determine the impact of shading from the environment, one house design was chosen for this study. The model building was designed created by the type-house design of Avanto Architects for the Finnish Cultural Foundation-funded K3 detached house project (Avanto Architects, 2011). The K3 project studied the possibilities for contemporary, sustainable, quality, and affordable housing. The designs in the projects utilized natural materials and traditional construction methods. The Finnish Cultural Foundation purchased the designs from the architects and published them open access.
The type-houses were placed at each site and replaced existing buildings in three different Helsinki neighborhoods (Figure 1). The sites were selected to represent three typical low-rise neighborhood typologies in the Helsinki area. The neighborhoods had uniform building heights due to zoning laws, which are common in low-rise residential areas in Helsinki and other Nordic cities. The major impacts of shading across all sites came from the proximity and height of neighboring buildings as well as the surrounding vegetation.
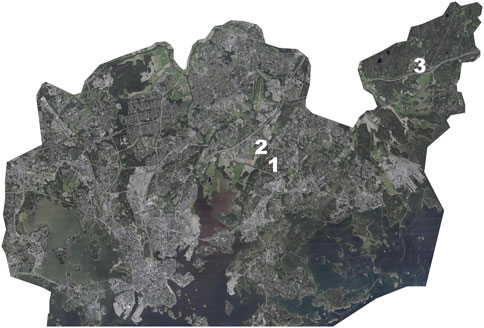
FIGURE 1. Helsinki map (City of Helsinki, 2022).
Case study site 1 in Myllypuro
Case Study Site 1 was located in Helsinki’s Myllypuro, a residential area consisting of detached houses constructed from the 1960s onward (Figure 2). The buildings were mostly one-story tall and located in relatively dense lots. Since the neighborhood was around 50 years old, the trees and vegetation had reached maximum growth. The neighborhood represents areas of detached houses within the borders of Helsinki built after the Second World War.
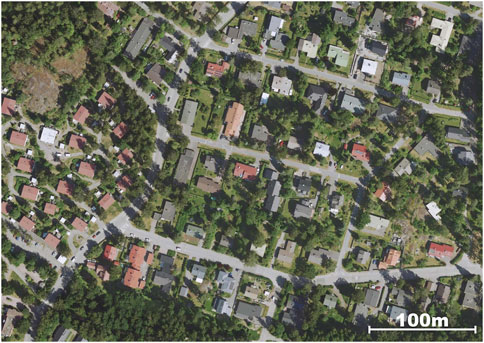
FIGURE 2. Case study site 1 in Myllypuro (City of Helsinki, 2022).
Case study site 2 in Viikki
Case Study Site 2 is located in Helsinki’s Viikki, a residential area built in the 2000 s (Figure 3). Viikki represents contemporary urban design trends in Helsinki, focusing on a dense urban fabric. The area consisted of detached and row houses located close to each other on small lots, forming relatively closed blocks. Viikki was defined by vast open fields that were still being cultivated. Vegetation had grown since the construction phase, but the selected site had a few tall trees that caused shading. In the south, the open field guaranteed minimal shading from vegetation for most of the buildings in the area.
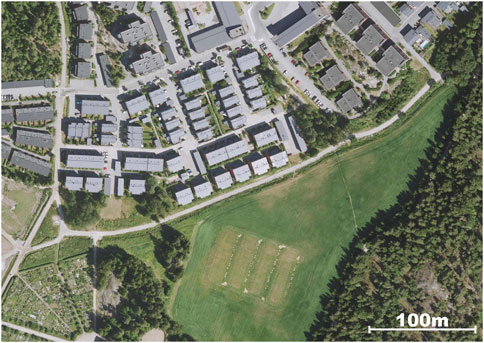
FIGURE 3. Case study site 2 in Viikki (City of Helsinki, 2022).
Case study site 3 in Landbo
Case Study Site 3 was located in Helsinki’s Landbo (Figure 4). The area was incorporated into Helsinki from Sipoo in 2009. The neighborhood represents the sparsely constructed urban areas in the municipalities surrounding Helsinki. The lots were relatively large, and the buildings were located quite far from each other. The vegetation in the area had grown since the construction phase, but the yards had been kept relatively clear of tall trees. This case study area had low density, opening up possibilities for ground-mounted PV installations.
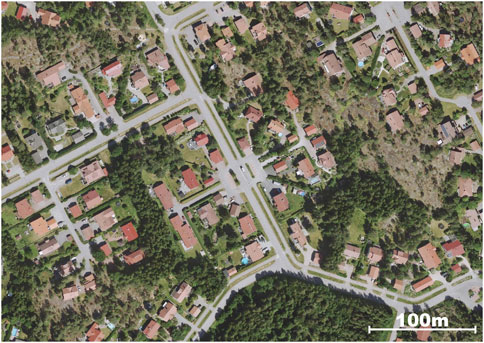
FIGURE 4. Case study site 3 in Landbo (City of Helsinki, 2022).
Computational methods
Commercial PVsyst software (version 7.4.2) (PVsyst, 2023) was used to calculate the annual energy production of different PV systems. Generic 144 half-cell 440 Wp bifacial panels from the PVsyst library were used for the simulations. The bifacial simulations were completed by simulating the panels as monofacial versions (bifaciality feature turned off in PVsyst) for the front and rear sides separately, and then calculating the production using a bifaciality factor of 80% for the rear side. The side with higher annual production was chosen as the front side for each simulation individually. The number of panels was chosen based on the physical limitations set by the installation site and the aim of the particular simulation. For all simulations, the panels were installed in one string, unless otherwise stated. The most suitable generic inverter from the PVsyst library (i.e., the one with the lowest nominal power without the need for curtailment) was chosen. If all available inverters were strongly oversized, the voltage and thus the nominal power of the inverter were decreased so that the nominal power was fixed to the smallest integer kilowatt peak, which allowed curtailment to be avoided. The weather data were imported from the Meteonorm database (Meteonorm, 2023) by the user interface of PVsyst.
The shading from buildings, vegetation, and other PV panels was calculated by creating detailed 3D models of all studied cases using the “Near Shadings” feature in PVsyst. All structures and vegetation that may cause shading of the panels were included. These models are presented in Figure 5, Figure 6, and Figure 8 for Cases 1–3, respectively. A separate model was built for each simulation. For example, for the Case 1 and Façade 1 simulations (Table 2), only the façade slot marked with “1” in Figure 5 was occupied. The exact shading losses were calculated with PVsyst using the “Detailed electrical calculation (acc. to module layout)” setting. This setting allowed us to include the effects of partial shading in the simulations.
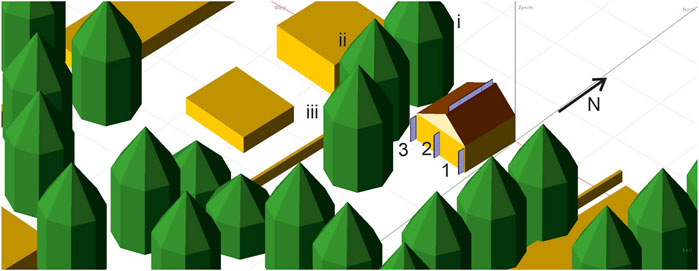
FIGURE 5. The target building and relevant surrounding objects for Case 1. The figure was created with PVsyst software (PVsyst, 2023).
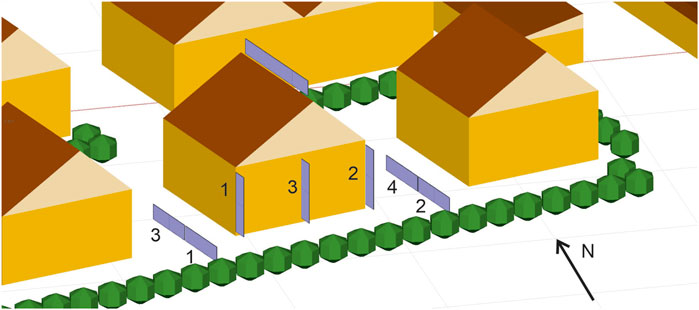
FIGURE 6. The target building and relevant surrounding objects for Case 2. The figure was created with PVsyst software (PVsyst, 2023).
For each case study, the parallel-to-roof MPV system (total of eight panels in two strings with separate inverters, 4 × 1 row for both roof faces) and a roof ridge VBPV system (four panels) were used as references. Since in Case 1, the shading even on the rooftop was severe, separate references without any shading objects were simulated. For Cases 2 and 3, the shading on the rooftop was insignificant (≤0.31% loss in annual irradiation due to shading) for all simulations, so no separate references were used.
Additional installation sites for VBPV systems on facades and the ground were searched for each case. The VBPV installation sites were studied separately for the two-panel blocks. The performance of the façade VBPV systems was compared to the façade MPV system, which consisted of four panels located on top of the south-facing façade. For Case 1, separate simulations were conducted, with reasonable land modifications; essentially some of the trees located in the plot were removed. The exact orientations of the panels are shown in Table 1. Finally, for Cases 2 and 3, recommended VBPV systems (i.e., systems that offer a reasonable compromise between the total production and specific yield [production per kilowatt peak]) were simulated. The azimuth angle for a solar panel was defined as follows in this study: an azimuth of 0° corresponded to a panel facing south. The positive direction was clockwise, meaning that an azimuth of 90° corresponds to the west and the azimuth −90° corresponded to the east. This notation is consistent with the PVsyst software.
Results
Case 1: old neighborhood with ample vegetation
Case 1 represented an old, low-rise neighborhood full of vegetation and was based on an existing residential area in Myllypuro, Helsinki (Figure 5). In this case, the number and height of trees made most of the suggested PV solutions impractical. The unshaded reference scenarios gave specific yields of 780 and 1,000 kWh/kWp for parallel-to-roof MPV and roof ridge VBPV systems respectively, but when shading was included, these values dropped to 460 and 470 kWh/kWp.
As a reasonable land modification, two of the tall trees close to the building were removed. The removed trees are marked in Figure 5: for rooftop PV scenarios, the removed trees were (i) and (ii), and those for façade and ground VBPV systems were (ii) and (iii). As a result, the rooftop MPV and VBPV systems had reasonable production (640 and 770 kWh/kWp), but façade and ground systems were still unfeasible. The key results pertaining to the simulated systems are presented in Table 2.
In this case, only the rooftop of the main building was suitable for PV installation, even with reasonable modifications to the environment. Even then, the shading losses were significant and could potentially accelerate the degradation of the panels. Ground-mounted VBPV systems were practically impossible to implement due to extensive shading.
Case 2: modern, dense neighborhood with an open field to the south
Case 2 represented a modern, densely packed low-rise neighborhood based on an existing area in Viikki, Helsinki (Figure 6). Here, the dense packing of buildings significantly reduced the possibility of utilizing ground-mounted VBPV systems. However, the open horizon to the south and the lack of tall trees made rooftops excellent for PV power production. Here, the target house was rotated slightly (15°) from the north-south axis, meaning that the azimuths of the rooftop systems were 75° (15° from west toward south) and −105° (15° from east toward north). The rooftop PV systems showed good yields (740 and 960 kWh/kWp for parallel-to-roof and roof-ridge VBPV systems, respectively). The shading losses were 0.11%–0.27% for these simulations, meaning practically unshaded situations.
Additional installation sites for VBPVs on the façade (three slots) and the ground (four slots, as fences between houses) were searched. As a suitable reference, a practically unshaded façade MPV system consisting of four panels installed parallel to façade (tilt: 90°, azimuth: −15°), yielding a specific production of 750 kWh/kWp, was used. For all VBPV slots, the shading losses were significant: over one-third of the total annual production was lost compared to the roof ridge VBPV system. However, when compared to that of the rooftop MPV system, the specific yields of the Façade and Ground Slots 1 and 2 were reasonable. Ground Slots 3 and 4 were unfeasible due to shading from the houses. The key performance indicators of the studied systems are summarized in Table 3.
If more than one of the façade slots were realized, there would be additional shading. Thus, systems with Façade 1 and 2 and all façade slots occupied were analyzed. The specific yield of PV panels in the Façade 2 slot when added to a system already including PV panels in the Façade 1 slot (i.e., the specific yield of Façade 2 minus the losses due to self-shading for Façades 1 and 2 when both slots are occupied) was 600 kWh/kWp. Similarly, the specific yield of Façade 3 when added to a system already including Façades 1 and 2 was 530 kWh/kWp. Therefore, when adding VBPV panels, the most efficient order is roof ridge-- > Facade 1-- > Facade 2-- > Ground 1-- > Ground 2-- > Façade 3.
Ultimately, a large VBPV system including a roof ridge installation site (four panels) and, Façade 1, Façade 2, Ground 1, and Ground 2 (two panels each), was investigated. A total of twelve 440 Wp panels were connected to three strings with separate inverters. The specific yield of the system was 700 kWh/kWp, resulting in 3,700 kWh of annual electricity production. This production would cover a large share of the electricity consumption for purposes other than heating in a Finnish detached house, which typically requires around 5,000 kWh a year. However, the contribution from the panels added to the façade and ground was low compared to roof ridge panels; increasing the number of panels from 4 to 12 (200% increase) resulted in an electricity production increase of only 120%. Thus, the roof ridge VBPV system is the first that should be implemented if possible, and the façade and ground sites should be occupied only if more PV production is needed.
One of the main motivations for favoring VBPVs is to improve the match between electricity consumption and load. This effect was investigated with the Case 2 house by producing hourly profiles of electricity generation. Four different systems were used: parallel-to-roof and parallel-to-façade MPV systems; a roof ridge VBPV system; and the suggested large VBPV system utilizing roof ridge, façade and ground installations. The total annual specific yield was plotted as a function of the hour of day in Figure 7 (e.g., the datapoints with “Hour = 10” show the total electricity production per kWp between 10 a.m. and 11 a.m. for 1 year).
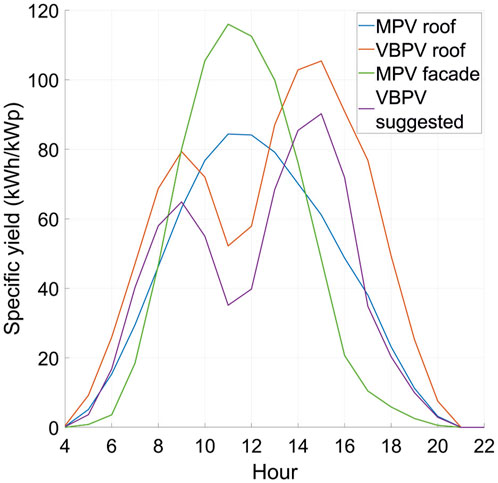
FIGURE 7. The total annual specific yield for the four PV systems as a function of the hour of the day. E.g., the datapoint for “Hour = 10” shows the specific yield for 1 year produced between 10 a.m. and 11 a.m.
Figure 7 shows that for the rooftop systems, the VBPV system had a slightly higher specific yield during the morning and a significantly higher specific yield during the evening when electricity consumption peaked. However, the roof ridge VBPV system could support only four panels, whereas the parallel-to-roof MPV system had eight panels and offered the possibility of installing more if needed. Thus, when suitable locations for VBPV systems were searched for elsewhere, this benefit was partly lost due to shading losses. When comparing MPV profiles, the façade system had a very high and narrow peak, whereas the roof system had a lower but wider peak. This was due to the orientation of the panels: the façade MPV system had an azimuth of −15°, whereas the rooftop MPV system had a 1:1 ratio of azimuths of 75° and −105°.
To differentiate the effects of the surrounding objects and the orientation of the neighborhood, an additional simulation was carried out with the suggested system in a hypothetical scenario where the area was aligned according to the north-south axis (i.e., the whole area was rotated clockwise by 15°). Surprisingly, the effect on power production was small. In the rotated scenario, the annual electricity production of the suggested system was 3,700 kWh, which was identical to the initial scenario (with accuracy of two significant digits). The small increase in production was due to the limited bifaciality of the panel; the 20% additional loss from the rear side was higher in the rotated scenario than in the initial one. When bifaciality was increased to 90%, the difference in the electricity production of the initial and rotated cases was 2.5%.
Case 3: modern, sparse neighborhood
Case 3 represented a relatively modern, sparse, urban low-rise neighborhood based on an existing area in Landbo, Helsinki (Figure 8). The target house was rotated by 25° counterclockwise (i.e., the azimuths of the roof slopes were 65° and 115°). The specific yields of the rooftop parallel-to-roof MPV and roof ridge VBPV systems were 690 and 910 kWh/kWp, respectively. The shading was insignificant (0%–0.31%), indicating that the reduced production rates when compared to Case 2 were due to the unfavorable orientation of the building.
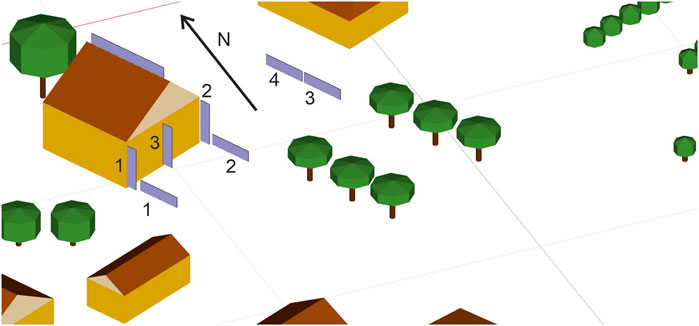
FIGURE 8. The target building and relevant surrounding objects for Case 3. The figure was created with PVsyst software (PVsyst, 2023).
Additional VBPV installation on the façade sites were searched for, similar to Case 2. The orientation of the building was strongly affected: Façade 1 was better in Case 3 than in Case 2, but Façades 2 and 3 were worse due to shading from the building itself. This was especially clear when PV panels in the Façade 2 slot were added to a system that already included PV panels in the Façade 1 slot: The specific yield of the additional panels, when considering the induced losses, was only 400 kWh/kWp. The key performance indicators of the studied systems are summarized in Table 4.
For the ground VBPV systems, fence structures to bordering the space for car parking in front of the house were studied. Ground 1 had significantly better performance than Ground 2 due to the orientation of the building. Moreover, the sparse nature of the area provided other possibilities for VBPV fences. For example, a fence between the road and the sidewalk (Grounds 3 and 4) was investigated. The specific yields were reasonable, but since the fence was located in a public area, this arrangement would require permission from the city.
Finally, a system similar to the suggested system in Case 2 (four roof ridge panels and two panels at slots Façade 1, Façade 2, Ground 1, and Ground 2 each, totaling twelve 440 Wp panels connected to three strings with separate inverters) was implemented. The total production was 3,600 kWh (680 kWh/kWp), slightly lower than in Case 2. This was likely due to the lower performance of the roof ridge panels. The increase in production when comparing the suggested system to the roof-ridge-only system was 130%. When the case study area was rotated by 25° clockwise (i.e., aligned according to the north-south axis), the suggested system still produced 3,600 kWh, identical to the initial system within the accuracy of two significant digits. With 90% bifaciality, the difference between the initial and rotated cases was 1.9%.
Discussion
The results of this study showed several possibilities for viable applications of VBPV systems in small-scale residential neighborhoods in Finland. The effects of shading from the surroundings were also determined. In the most favorable scenario, the VBPV system had a higher specific yield than the MPV system (Jouttijärvi et al., 2023). However, self-shading caused significant losses in VBPV systems, which is one of the findings of Baumann et al. study (2019). To avoid self-shading, fewer VPBV panels can be installed, but this would result in lower peak production.
The results showed that trees have a higher influence on VBPV systems than surrounding buildings, and Case 1 demonstrated this conclusion: by removing two trees near the house in Case 1, the specific yield of the PV system improved from 470 to 770 kWh/kWp. Since buildings in low-rise neighborhoods have homogeneous heights, their effect on roof PV systems is smaller compared to the effect of trees. The influence of the trees came from their closer proximity to the PV systems and their size. The trees that were outside of the Case 1 property had a lower impact than those inside the property. On the façade and ground systems, however, shading from other buildings impacted PV production considerably, depending on the installation location. In Cases 2 and 3, the trees were either planted farther away from the buildings or shorter, and their effect on the case studies was less significant.
However, trees are often desirable in the built environment. Cutting down all trees on a property can reduce shading but at the cost of removing the aesthetic and other benefits that trees provide. Pre-planning to minimize shading from trees during the building design process is required to optimize the electricity yield from PV systems for houses. Furthermore, if an entire property was fully shaded by trees, whether to utilize PV systems in this situation must be carefully considered.
Studying VBPV systems in new neighborhoods that have been optimally designed for VBPV integration can yield better results. Case 1 demonstrated that removing two nearby trees significantly improved the performance of the VBPV system. In an optimally designed neighborhood, houses, and trees can be strategically placed to provide space for ground systems without causing aesthetic or practical drawbacks, or the geometry of a house can be designed to minimize the shading of a façade system.
South-facing roof system
The roof systems-the roof ridge VBPV, and parallel-to-roof MPV systems-performed consistently well in Cases 2 and 3. VBPV installations in Case 1 suffered significant shading from nearby trees. However, the shading in Case 1 did not affect the VBPV system exclusively, since the MPV system was also shaded by the trees. Nonetheless, the VBPV system was more vulnerable to shading. In the area with fewer tall trees, which were represented in Cases 2 and 3, VBPVs on the roof had a higher specific yield than MPVs. However, the number of PV panels for the roof ridge system was limited to four, whereas the roof faces can theoretically fit over 40 MPV panels in total.
The VBPV systems tested in this study greatly benefited from the favorable geometry of the houses. All case study buildings featured similar gable roof shapes, and the slopes of the roofs all faced roughly east-west. This is a geometry that favors east-west VBPVs, since they can be installed on the pitch of the roof. In contrast, there were no roof surfaces that faced directly south, which is a more optimal installation condition for the MPV system.
To investigate a case featuring a favorable geometry for MPVs, additional simulations were conducted with a south-facing rooftop (Figure 9), and the results are shown in Table 5. Here, a south-facing rooftop was investigated as an installation site for VBPV panels. For simplicity, the simulation is done without any shading from the surroundings. The specific yield of the VBPV systems started to decrease quickly when the number of rows increased due to increased self-shading. Even with one row, the specific yield was lower than for the roof ridge system due to shading from the target building itself. Therefore, the MPV system was superior in terms of total and specific production due to the possibility of installing an extensive number of panels without any shading loss.
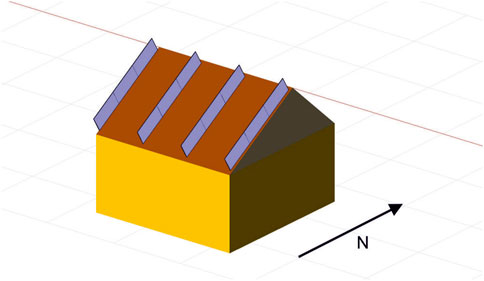
FIGURE 9. Example of a VBPV system on a South-facing roof. The figure was created with PVsyst software (PVsyst, 2023).
The results from the north-south-facing roof simulation indicated the importance of building orientation in the viability of VBPV systems. This means that the decision between installing an MPV or a VBPV system can be made based on roof orientation. Consequently, a VBPV system might allow building designers to have more flexibility over the design of their buildings. To maximize the performance of an MPV system, the existence of a south-facing roof is mandatory. However, utilizing a VBPV system creates the possibility of installing PV panels on buildings that have east-west-facing roofs.
In reality, aside from roof orientation, other factors, such as roof shape, can affect a PV system (Hachem et al., 2011). Here, only one building geometry was used to reduce the number of variables in the simulations and to enable direct comparisons of different real environments. VBPV systems placed on the roof ridge are expected to be less influenced by roof geometries than conventional MPV installations.
Ground and facade systems
For façade and ground systems, the specific yields were considerably lower than for roof systems. However, even with a low specific yield, they might be viable in certain circumstances, such as when the electricity price increases significantly or the cost of PV electricity is further reduced. The hourly electricity cost can play a more significant role in the future. Production in certain hours can have a better return on investment, justifying the lowering of overall production. Increasing the share of renewable energy consumption and reducing electricity consumption from fossil fuels is another motivation to employ façade and ground systems. In accordance with Finland’s ambitious climate targets, new regulations that will limit the carbon footprint of every new building in Finland have been announced. This limit on greenhouse gas emissions will gradually progress toward net zero-energy buildings in the near future (Ministry of the Environment, 2023). Furthermore, PVs have been integrated into building design by building designers to offset greenhouse gas emissions for net zero-energy building (Satola et al., 2022). Installing VBPVs on the ground and façades can create more design possibilities for net zero-energy buildings.
VBPVs on the façade can be more suitable for other building types. For most standard detached houses, energy consumption grows with a building’s floor area. In these houses, the roof area to total floor area ratio is high. This means that there will be ample roof space to install PV panels to cover a significant share of the electricity consumed by the house, with electricity from PV production. However, multistory buildings have a small roof area to total floor area ratio, and their roofs are often used for other building systems. This results in a lack of roof space for the installation of PV panels. Therefore, installation of PV panels on the façade should be considered if high total electricity generation is required. Although MPV systems have been used on multistory buildings’ facades to increase total PV power production, east-west-facing VBPV systems have an advantage over MPV systems. The southern façades of most buildings in Finland are designed with ample openings to provide natural light to interior spaces. Installing PV panels on the southern façade requires a significant area of solid walls, limiting the number of south-facing windows or balconies. Due to their geometry, east-west-facing VBPV systems require fewer solid walls on façades than MPV systems, and spaces between VBPV arrays can be used for windows or balconies (Jouttijärvi et al., 2022). Regardless of the possible advantages that VBPV systems appear to have over MPV systems for multistory building façades, further study is needed to compare the performance of the two PV systems.
Structural and aesthetic aspects
The viability of VBPV systems in Finland can also be affected by factors unrelated to their power production capabilities. The structural requirements of VBPV systems are drastically different from those of MPV systems and require different designs, materials, and supply chains. The structure of VBPV systems is likely to be more expensive than that of MPV systems, since it must handle a higher wind load. These factors affect the cost of VBPVs, which already have a higher module price than monofacial PVs (Rodríguez-Gallegos et al., 2018). However, the decreased labor costs and lack of scaffolding required for ground installations reduce expenses.
Aesthetics is an important part of the built environment. Lobaccaro, et al. (2019) conducted a multidisciplinary analysis of 34 different PV projects from 10 countries. The study emphasized architectural integrity as one of the most important factors in the success of PV projects (Lobaccaro et al., 2019). Most of the panels in VBPV systems are projected farther away from the building body than those in MPV systems. Therefore, VBPV panels will be more visible than MPV panels. This additional visibility require different design strategies to maintain the architectural integrity of VBPV-installed buildings, thus providing additional challenges to building designers. However, the proper design of VBPVs to leverage the benefits of VBPVs while maintaining architectural integrity is be possible.
Future studies
Since Helsinki is located in the southern part of Finland, which is the most populated region in the country, and has a similar latitude to the most populated areas of Sweden and Norway, the findings in this study are also applicable to these other two countries. However, due to VBPVs’ sensitivity to solar elevation angles, the influence of shade will differ in other latitudes, and further study is needed to understand the influence of shading on VBPVs in those locations.
The low-rise residential neighborhoods chosen in the study were intend to represent typical Nordic neighborhoods. The decision to focus on low-rise residential neighborhoods limited the building types studied to one. In other neighborhoods with different building types, the effects of shading will be drastically different, and future studies can focus on other neighborhoods to create a broader understanding of the influence of shading on VBPVs in built environments.
Building geometry is another area that future studies can address. The building design was consistent across all case studies, to determine and compare the effects of shading in different neighborhoods. Future studies can focus on the effects of shading on VBPVs for different building designs and fill this knowledge gap.
The economic viability of VBPVs was beyond the scope of this study due to uncertainties in the investment required to install VBPVs on a commercial scale. With the further development of VBPVs and the availability of new knowledge, the economic aspects of VBPVs should be prioritized.
Conclusion
This study compared the specific yields of VBPV and MPV systems in low-rise residential neighborhoods in Helsinki, aiming to identify the suitable installation sites for VBPV systems. Three neighborhoods, were used as case studies, each representing a different type of low-rise residential neighborhood. The fixed physical surroundings, especially the locations and heights of the buildings and trees, dominated the factors affecting the feasibility of the PV systems studied.
The results from Case 1, an old neighborhood with tall trees, showed that trees reduced the performance of VBPVs drastically when they were close to the building with PV systems. Compared to the ideal scenario of roof ridge VBPVs without shading, the specific yields of roof-mounted VBPV and MPV systems were reduced by 55%. Trees affects both MPV and VBPV systems, although VBPV panels were even more vulnerable to trees due to their 90° tilt angle. To investigate a less extreme case, a few trees were removed from Case 1. In Cases 2 and 3, shading from trees did not influence the PV systems. In the adjusted Case 1 and the other two cases, VBPVs outperformed MPVs by 20%–30%. Building orientation played an important role in the results. The houses in all case studies had roof surfaces facing east-west. Conversely, MPVs performed better than VBPVs on houses that had north-south-facing roofs in both specific yield and total production.
Façade and ground systems suffered from trees more than roof systems. In Case 1, all façade and ground-mounted PV systems suffered over a 65% loss compared to the roof ridge VBPV system, even with the two closest trees removed. Thus, they are not recommended in an environment with tall trees. Façade and ground systems were significantly more influenced by shading from their own buildings and surrounding houses than roof systems. In Case 2 and 3 the performance loss in façade and ground systems was between 30% and 70% compared to the roof ridge VBPV system. The wide range of results depended on the installation locations. Some VBPV installation locations on the ground and façade had acceptable performance and can be recommended for houses that require high electricity production. Other building types could potentially benefit from façade systems more than low-rise residential buildings an area for future research. Buildings that have small or limited roof spaces might require PV systems on their façades to achieve their targeted electricity production.
A major disadvantage of VBPVs is the amount of space required on their installed surfaces. Installing VBPV panels in arrays causes self-shading in PV systems. The results showed that the effect of self-shading on VBPV systems was considerable. This required spacing the VBPV panels further apart, reducing their potential total production. In the case of roof systems, the only available space for VBPV panels is on the roof ridge, and the number of PV panels that can be installed in this manner is far fewer than that for MPV systems. All houses in the case studies had gable roofs, and other roof shapes could influence the shading of PV systems differently.
The shifting of peak production of VBPV systems from noon to morning and evening is a desirable feature, as demonstrated in the results from Case 2. VBPV panels on the roof and façade offer peak production during the morning and evening, which matches the with peak energy consumption in residential buildings. To promote self-consumption, VBPV systems are suitable for residential buildings.
The structural and aesthetic aspects of VBPV systems are fundamentally different from those of MPV systems. The structural support for VBPVs has to be more robust than that of MPVs to withstand a higher wind load. VBPVs are installed perpendicular to the surface that hosts them, making them more visible than MPVs. Their higher visibility considerably affects the aesthetics of the buildings that host them. The influence of these two aspects of VBPVs needs to be considered and studied for future VBPV applications.
The findings of this study contribute to the understanding of novel applications of solar energy in built environments. The low-rise neighborhoods of Helsinki were the targets of this study, but the results can be applied to other locations with similar latitudes and similar urban contexts, especially other Nordic countries.
Data availability statement
The original contributions presented in the study are included in the article/Supplementary material, further inquiries can be directed to the corresponding author.
Author contributions
BV: Conceptualization, Investigation, Methodology, Writing–original draft. SJ: Conceptualization, Formal Analysis, Investigation, Methodology, Visualization, Writing–original draft. MJ: Conceptualization, Investigation, Methodology, Visualization, Writing–original draft. KM: Funding acquisition, Supervision, Validation, Writing–review and editing.
Funding
The author(s) declare financial support was received for the research, authorship, and/or publication of this article. We acknowledge financial support from the Finnish Cultural Foundation, the Research Council of Finland (347275 and 347277), and the Emil Aaltonen Foundation (SJ).
Conflict of interest
The authors declare that the research was conducted in the absence of any commercial or financial relationships that could be construed as a potential conflict of interest.
Publisher’s note
All claims expressed in this article are solely those of the authors and do not necessarily represent those of their affiliated organizations, or those of the publisher, the editors and the reviewers. Any product that may be evaluated in this article, or claim that may be made by its manufacturer, is not guaranteed or endorsed by the publisher.
References
Avanto Architects (2011). Tyyppitalo noppa selostus, suomen kulttuurirahaston pientalohanke. Available at: https://skr.fi/serve/k3-noppa-suunnitelma (Accessed October 26, 2023).
Baumann, T., Nussbaumer, H., Klenk, M., Dreisiebner, A., Carigiet, F., and Baumgartner, F. (2019). Photovoltaic systems with vertically mounted bifacial PV modules in combination with green roofs. Sol. Energy 190, 139–146. doi:10.1016/j.solener.2019.08.014
Brennan, M. P., Abramase, A., Andrews, R., and Pearce, J. (2014). Effects of spectral albedo on solar photovoltaic devices. Sol. Energy Mater. Sol. Cells 124, 111–116. doi:10.1016/j.solmat.2014.01.046
Freitas, S., and Brito, M. C. (2019). “Renewable and sustainable energy reviews,” in Non-cumulative only solar photovoltaics for electricity load-matching (Amsterdam, Netherlands: Elsevier Ltd), 271–283. doi:10.1016/j.rser.2019.04.038
Good, C. S., Lobaccaro, G., and Hårklau, S. (2014). “Optimization of solar energy potential for buildings in urban areas - a Norwegian case study,” in Energy procedia (Amsterdam, Netherlands: Elsevier Ltd), 166–171. doi:10.1016/j.egypro.2014.10.424
Guerrero-Lemus, R., Vega, R., Kim, T., Kim, A., and Shephard, L. E. (2016). ‘Bifacial solar photovoltaics - a technology review’, renewable and sustainable energy reviews. Amsterdam, Netherlands: Elsevier Ltd, 1533–1549. doi:10.1016/j.rser.2016.03.041
Guo, S., Walsh, T. M., and Peters, M. (2013). Vertically mounted bifacial photovoltaic modules: a global analysis. Energy 61, 447–454. doi:10.1016/j.energy.2013.08.040
Hachem, C., Athienitis, A., and Fazio, P. (2011). Parametric investigation of geometric form effects on solar potential of housing units. Sol. Energy 85 (9), 1864–1877. doi:10.1016/j.solener.2011.04.027
International Energy Agency (2023). Energy policy review Finland 2023. Available at: www.iea.org/t&c/.
Jouttijärvi, S., Lobaccaro, G., Kamppinen, A., and Miettunen, K. (2022). Benefits of bifacial solar cells combined with low voltage power grids at high latitudes. Renew. Sustain. Energy Rev. 161, 112354. doi:10.1016/j.rser.2022.112354
Jouttijärvi, S., Thorning, J., Manni, M., Huerta, H., Ranta, S., Di Sabatino, M., et al. (2023). A comprehensive methodological workflow to maximize solar energy in low-voltage grids: a case study of vertical bifacial panels in Nordic conditions. Sol. Energy 262, 111819. doi:10.1016/j.solener.2023.111819
Lobaccaro, G., Carlucci, S., Croce, S., Paparella, R., and Finocchiaro, L. (2017). Boosting solar accessibility and potential of urban districts in the Nordic climate: a case study in Trondheim. Sol. Energy 149, 347–369. doi:10.1016/j.solener.2017.04.015
Lobaccaro, G., Croce, S., Lindkvist, C., Munari Probst, M., Scognamiglio, A., Dahlberg, J., et al. (2019). A cross-country perspective on solar energy in urban planning: lessons learned from international case studies. Renew. Sustain. Energy Rev. 108, 209–237. doi:10.1016/j.rser.2019.03.041
Meteonorm (2023). Intro. Available at: https://meteonorm.com/(Accessed: October 26, 2023).
Ministry of the Environment (2023). Finland’s national climate policy. Available at: https://ym.fi/en/finland-s-national-climate-change-policy (Accessed November 13, 2023).
Pvsyst (2023). Logiciel photovoltaïque. Available at: https://www.pvsyst.com/(Accessed October 26, 2023).
Rodríguez-Gallegos, C. D., Bieri, M., Gandhi, O., Singh, J. P., Reindl, T., and Panda, S. (2018). Monofacial vs bifacial Si-based PV modules: which one is more cost-effective? Sol. Energy 176, 412–438. doi:10.1016/j.solener.2018.10.012
Satola, D., Wiberg, A. H., Singh, M., Babu, S., James, B., Dixit, M., et al. (2022). Comparative review of international approaches to net-zero buildings: knowledge-sharing initiative to develop design strategies for greenhouse gas emissions reduction. Energy Sustain. Dev. 71, 291–306. doi:10.1016/j.esd.2022.10.005
Shukla, P. R. (2022). Climate change 2022 mitigation of climate change working group III contribution to the sixth assessment report of the intergovernmental panel on climate change summary for policymakers. Available at: www.ipcc.ch.
Statistics Finland (2023). Housing and construction. Available at: https://www.tilastokeskus.fi/tup/suoluk/suoluk_asuminen_en.html (Accessed: October 26, 2023).
Keywords: vertical bifacial PV, built environment, building applied PV, high-latitude conditions, case studies
Citation: Viriyaroj B, Jouttijärvi S, Jänkälä M and Miettunen K (2024) Performance of vertically mounted bifacial photovoltaics under the physical influence of low-rise residential environment in high-latitude locations. Front. Built Environ. 10:1343036. doi: 10.3389/fbuil.2024.1343036
Received: 22 November 2023; Accepted: 12 January 2024;
Published: 24 January 2024.
Edited by:
Gilles Desthieux, University of Applied Sciences Western Switzerland, SwitzerlandReviewed by:
Priya Ranjan Satpathy, Council of Scientific and Industrial Research (CSIR), IndiaHasim Altan, Prince Mohammad bin Fahd University, Saudi Arabia
Copyright © 2024 Viriyaroj, Jouttijärvi, Jänkälä and Miettunen. This is an open-access article distributed under the terms of the Creative Commons Attribution License (CC BY). The use, distribution or reproduction in other forums is permitted, provided the original author(s) and the copyright owner(s) are credited and that the original publication in this journal is cited, in accordance with accepted academic practice. No use, distribution or reproduction is permitted which does not comply with these terms.
*Correspondence: Bergpob Viriyaroj, QmVyZ3BvYi52aXJpeWFyb2pAYWFsdG8uZmk=