- 1Department VI, Technical University of Berlin, Berlin, Germany
- 2Tegel Projekt GmbH, Berlin, Germany
- 3Department VI, Institute of Ecology, Technical University of Berlin, Berlin, Germany
The building and construction sector is responsible for a large share of carbon emissions resulting in the need to reduce them to mitigate climate change. Timber construction methods promise to lower emissions combined with biogenic carbon storage in the built environment. While there are several studies comparing the emissions of mineral-based and timber-based buildings, a consistent comparison of different timber-based building assemblies is still missing. This study compares carbon emissions from material production and carbon storage capabilities of four timber-based and two brick and reinforced concrete building assemblies. These assemblies were designed for a residential multi-storey building in Berlin, Germany. To compare and rank the carbon impacts of these assemblies we introduce a carbon storage-to-emission ratio. The calculations were performed using a Carbon Cycle Assessment Model implementation in Python. The results indicate an average reduction in carbon emissions of timber-based building assemblies by 32.6% to “Brick” and 40.4% to “Reinforced Concrete”, respectively. Across the timber-based building assemblies, the carbon emissions range between 85 t and 115 t, leading to an average of 105 t per building. Pronounced differences were observed in carbon storage, with the “Dowel Laminated Timber” building assembly storing more than three times the amount of carbon compared to “Light Weight Timber” assembly. To further reduce emissions from buildings and the construction sector and potentially enhance urban carbon storage, “Glue Laminated Timber” and “Dowel Laminated Timber” building assemblies were identified as the most promising.
1 Introduction
Over the last century, the material stock in the built environment has increased significantly. Among these prevalent materials, reinforced concrete and brick are the predominantly used (Elhacham et al., 2020). In the rest of this article, the term “conventional mineral-based materials” will be used to refer to reinforced concrete and brick (Elhacham et al., 2020). These mineral-based building materials have become the standard for large scale house construction due to their compressive strength and stability. However, these building materials have a decisive disadvantage: large amounts of energy are used for their production and processing, which is mostly generated from the combustion of fossil fuels (Dangel, 2017). This issue underlines the urgent need to transform global building methods and create new pathways for sustainable construction. The choice of building materials and the energy performance of buildings offer great potential for reducing emissions (Churkina et al., 2020; IEA, 2022).
Unlike mineral-based building materials, wood is an organic and renewable resource and therefore a promising material to reduce carbon emissions (Dangel, 2017). During the process of photosynthesis, a tree uptakes carbon dioxide from air and binds it up in sugar, needed to build its stem, roots, and branches. Trees store carbon throughout their entire lifespan and thus play a crucial role in the carbon cycle, acting as a natural carbon sink. When trees are harvested, the stored carbon remains within the raw material. Even when a part of carbon stock is removed from the forest, carbon dioxide is released again only when aerobic decay occurs or during combustion (Organschi et al., 2016). Therefore, the storage of carbon in long-living wood products like building parts can potentially increase the urban carbon stock (Lauk et al., 2012).
Beyond their ability to store carbon, timber-based buildings and other long-living products can also positively impact the carbon cycle through substitution effects: an increased use of wooden materials for buildings would in consequence reduce CO2 emissions from the production of mineral-based materials like concrete or steel (Bowyer et al., 2012). It is important to recognize that there are opposing concepts to the substitution strategy, such as the forest conservation strategy. This approach emphasizes the significance of forests as potential active carbon sinks and advocates against harvesting wood or altering land use to fully exploit this potential. To conserve forests for effective emissions reduction, sustainable management practices are essential (Erb et al., 2022).
Additional advantages of timber construction include short construction times, a high degree of prefabrication, low material weight and comfortable indoor climate—altogether reasons why building with biomass-based or low-emitting materials is increasingly being recognized as a piece of the puzzle of climate change mitigation measures (Hildebrandt et al., 2017; Churkina et al., 2020). At the same time, regional forest management must also come into focus when addressing climate change mitigation measures. When looking at forest ecosystems globally, living trees, including their aboveground biomass and root systems, store 42% of the global carbon stock, while forest soils store 44%, and dead wood and leaf litter account for 13% (Hurteau, 2021). It is therefore important to maintain a forest ecosystem that is in balance and resilient to combat climate change.
Many studies have proven the advantages of using wooden materials in the construction sector to decrease carbon emissions compared to mineral-based materials, here reinforced concrete and brick, without deteriorating the natural carbon sink function of forests (Hildebrandt et al., 2017; Churkina et al., 2020; Hart and Pomponi, 2020; Arehart et al., 2021). However, there are still several obstacles, preventing the expansion of wooden construction methods for the mass market in Germany. Practitioners and legislators claim that more knowledge about materials is needed, education in the building sector should focus on wood construction and legal restrictions need to be put to the test (Bundesministerium für Wohnen, 2023; European Environment Agency, 2014). Practical science-based guidelines are needed to promote the use of timber in the construction sector and to support architects and urban planners in the long-term.
Previous studies have found that residential timber buildings with one up to twelve stories could reduce the amount of embodied energy by 28%–47% in comparison to concrete and steel buildings (Schenk and Amiri, 2022). Additionally, another study estimated a reduction in energy consumption by 25% for a 8-story residential timber building compared to a concrete building (Chen et al., 2021). There are also studies comparing different building typologies based on their construction materials, specifically mineral-based, timber-based, or hybrid compositions (Duan et al., 2022; Rinne et al., 2022). However, timber buildings often consist of different hybrid materials resulting in varying shares of wood being used (Svatoš-Ražnjević et al., 2022). A recent study examined different ceiling systems, including two timber ceilings such as cross laminated timber (clt) and wood-concrete-compound regarding their cradle-to-cradle greenhouse gas emissions. Most fossil emissions of ceiling systems result from the manufacturing phase. Because it is difficult to predict information accurately before construction and because there are uncertainties related to additional emissions, the authors suggest considering the emissions generated during manufacturing (Heckmann and Glock, 2023). Furthermore, in most of the Life Cycle Assessments, biogenic carbon storage is not specified, although it would increase the comparability between wooden buildings (Andersen et al., 2021). A review of the literature revealed gaps in knowledge, particularly regarding the carbon storage capabilities of different multi-story buildings. Often, there are comparative analyses between one mineral-based and one timber-based typology, a comparison showing different timber-based typologies could provide further information on carbon emissions and carbon storage across the range of timber-based typologies. Therefore, this paper aims to assess different wooden construction typologies, and their carbon emissions from building production as well as carbon storage. More precisely, the paper attempts to answer the following questions:
1. How do different timber- and mineral-based building typologies perform in terms of their carbon emissions and carbon storage capacity from cradle to gate?
2. Which materials and building parts of those typologies contribute the most to the carbon emissions during production and which materials store the most carbon?
2 Materials and methods
2.1 Scope
This study performs a cradle-to-gate analysis on carbon emissions (steps A1-A3 in Life Cycle Assessments based on an EPD-module) of two mineral-based and four timber-based building assemblies. Furthermore, the total amount of carbon storage in biomass-based materials is determined to provide potential implications for the built environment serving as a carbon sink. This study does not consider transportation, the building’s operational energy, its potential demolition, as well as potential recycling or reuse of materials from demolished building.
2.2 Description of plans for Schumacher Quartier
To answer the research questions, a specific example is used: the future Schumacher Quartier in Berlin. The Schumacher Quartier is part of the city planning project Berlin TXL, which aims at designing the conversion of the former Berlin Tegel Airport into a mixed-use area. It is located in the eastern part of the Berlin Tegel Airport with the coordinates of 52°32′59.8″N and 13°17′52.3″E. The total project area of Berlin TXL is about 5 km2 whereas the Schumacher Quartier covers 48 ha and constitutes one of the largest urban development projects in Europe (Ambrosius-Groß et al., 2023). The plans for the Schumacher Quartier comprise ecological and socially sustainable construction concepts aiming to build 5,000 apartments for more than 10,000 dwellers with 50% of apartments allocated to state-owned housing associations. The future buildings will be designed with timber materials and have green roofs with water retention capacity (pers. communication Tegel Projekt GmbH, 2023; Figure 1). The proposed construction strategy entails utilizing timber as the primary material for building construction, seeking to mitigate CO2 emissions throughout the construction phase and enable temporary carbon storage within the structures. The intention is to minimize the carbon footprint associated with the building process of this residential area.
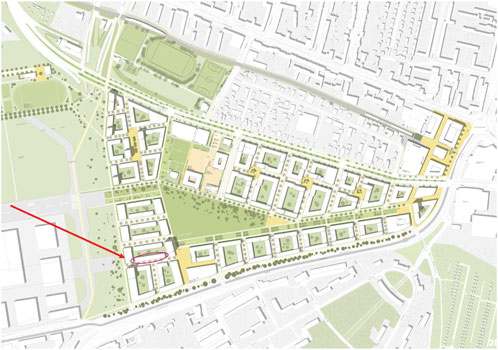
FIGURE 1. Plan of Schumacher Quartier, Tegel Projekt GmbH, Berlin 2023. The red arrow highlights the exemplary four-storey building shown in Figure 2.
2.3 Reference building design
The reference building refers to a four-storey residential building block with a floor area of 1,276.429 m2 per floor and an exterior wall area of 1,935.402 m2 (excluding windows), considering an estimated window proportion of 30% of the total exterior area (Figure 2). Indoor walls were not considered. The ceiling area amounts to 3,824.32 m2, while the roof area measures 1,274.77 m2. The roof is designed as a green roof, which will be covered by 80% with vegetation.
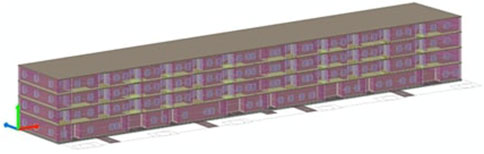
FIGURE 2. An exemplary four-storey building planned for Schumacher Quartier, Tegel Projekt GmbH, Berlin 2023.
The construction data for this study was provided by the Tegel Projekt GmbH, which is responsible for the conception of the six building typologies that served as the base for the investigations. Architectural specifications of the reference building including building materials, their quantities, densities, and mass fractions were developed by the Tegel Projekt GmbH. Our calculations are based on these specifications.
2.4 Building assemblies
The six building assemblies are equivalent in their function. They fulfill the same construction standards and requirements of thermal insulation, fire safety, noise protection, and moisture content. Each building consists of three parts: the walls, ceiling slabs and the roof. In this study, the assemblies are divided into three categories: mineral-based building typologies, light-frame timber building typologies and mass timber building typologies. An overview of the components and materials used are shown in Table 1. The configuration of the green roof is identical for all assemblies and is therefore not included. A more detailed table with all building materials in the building assemblies and their contributions by weight to the whole building and the single building parts (walls, ceiling slabs, roof) can be found in Supplementary Table S2. “Brick” and “Reinforced Concrete” are referred to as mineral-based typologies, “Light Weight Timber” and “Timber Frame” as light-frame timber typologies. The term mass timber typologies refer to “Glue Laminated Timber” and “Dowel Laminated Timber”.
2.5 Carbon cycle assessment—analysis tool
To estimate carbon storage and emissions from material production we used “Carbon Cycle Assessment” (CCA), which is an algorithm that calculates the carbon emissions and carbon storage of construction projects from material and transport based input data (Churkina et al., 2023). The numerical algorithm described below is a further development of the methodology established and applied for assessing the carbon benefits of a transition to timber construction to meet global housing needs (Churkina et al., 2020). The storage of carbon in buildings and associated emissions from materials’ production were estimated for the whole building as well as for its materials and parts such as exterior walls, ceiling slabs, and roof. The algorithm was implemented in python, version 3.10.11 and pandas, version 2.0.1. The data was visualized with matplotlib, version 3.7.1.
The carbon storage in a building (
CW—carbon to biomass ratio [dimensionless].
The carbon emissions associated with manufacturing materials (
2.6 Input data
The input data needed for calculations of the carbon emissions included carbon coefficients in CO2- equivalents, which were obtained from the database ÖKOBAUDAT, 2021-II and 2023I, maintained by the German federal ministry of housing, urban development and construction (BMWSB, 2023). If data was not available on ÖKOBAUDAT, the environmental product declarations (EPD) provided by the manufacturers of the respective materials were used. The EPD’s are regulated by the ISO 14025 (2006) (Bovea et al., 2014) and additionally by DIN EN 15804 on ÖKOBAUDAT (BBSR, 2021). As an exception the CO2 coefficients of Made of Air (MOA) was estimated in the course of this project (Supplementary Material of the report). MOA is a new biochar-based material with very high carbon storage (Made of Air, 2023), which is not listed in the ÖKOBAUDAT.
The ÖKOBAUDAT datasets offer a unified database encompassing various data types, spanning from individual products to broader product categories. These datasets are categorized as follows: manufacturer-specific datasets, industry association or multi-company average datasets, datasets that represent an entire country or region, template datasets tailored to specific products based on“template EPDs” and generic datasets derived from sources like literature and expert knowledge (Figl and Kusche, 2021, S. 12). Most datasets used in this study were averaged datasets representing either data from industry associations or product classes of one specific manufacturer (Supplementary Table S1). As transportation and construction on site were not considered in this study, only steps of production (A1–A3) were collected and used as input.
The CO2 coefficient describes the total global warming potential (GWP) of the building materials for the production phase, including the raw material supply, the transport to the production site and the production itself. As the CO2 coefficient refers to 1 kg of building material, the GWP was divided by the gross density or grammage of the material if the reference flow was not already referred to the mass. If more than one value for the GWP of a material was found during research, e.g., if different sources were available, the minimum and the maximum value found were used to determine a mean value for the GWP.
The GWP has negative values for some biomass-based, mainly wooden building materials, due to the amount of biogenic carbon stored within. Since the CO2 coefficient is only supposed to describe the carbon emissions, the carbon storage had to be excluded from the GWP. To do so, the mass of carbon contained in the building material was calculated using
Using the amount of substance, the mass of CO2 which could form out of the carbon in the building material, was calculated and added to the GWP of the material to eliminate the carbon storage and to determine the CO2 coefficient:
2.7 Assumptions
To compare the results of different typologies and material types, the groups “majority organic”, “partly organic” and “minority organic” are formed. The boundaries are the first and third quartile. However, there are no building materials between 25% and 75%, so the group “partly organic” is not used. Materials with a biomass content of more than 75% are referred to as “majority organic”, materials with a biomass content lower than 25% are referred to as “minority organic”. “Non-organic” materials have no biomass content at all. This applies especially to “intensive substrate light”, which has a biomass fraction of 10% and makes up 20% of the total weight of the “Light Weight Timber” assembly. The substrate is used as a growing medium in the green roof systems. It was chosen not to assess the potential changes in green roofs carbon storage potential in more detail since the green roofs are identical throughout all six assemblies.
Reinforced concrete itself has no EPD of its own and consequently no direct CO2 emission coefficient. In order to determine a coefficient nevertheless, we have assumed a reinforcing steel mass content of 2% and combined two EPDs for concrete and reinforcing steel. The cited concrete-dataset reflects the typical compositions and practices of concrete in the german industry with a cement share of 13.7 m-%. While there is no exact statement about the clinker content, the current average proportion of cement clinker in Germany is 70 m-% (Verein Deutscher Zementwerke e.V, 2023).
If no further information was provided in the material data sheets, the calculations were conducted with a carbon to wood ratio of 0.5, which is the global average of 0.476 ± 0.04 (Martin et al., 2018) rounded to the first decimal place. The biomass and carbon fraction for non-organic materials were assumed as zero, if not provided otherwise by respective material data sheets.
2.8 Assessment of carbon impacts: Storage-to-emission ratio
The carbon emissions and storage capacity of six building assemblies were compared from cradle to gate. In addition, we evaluated and compared the carbon impact of the building assemblies by estimating their carbon storage to emission ratio. The ratio is calculated by dividing total carbon storage of a building by total carbon emissions from its production. The higher this ratio, the better the building assembly performs, as more carbon is stored per unit of carbon emitted.
3 Results
The following section will outline the results, starting with the comparison of carbon emissions, followed by carbon storage and the resulting ratio. In the second part the contribution of different materials to the total weight, emissions and storage will be described for each assembly. In the last part, the carbon emissions and storage will be compared regarding the different building parts across the assemblies.
3.1 Comparison of carbon emissions, storage and storage-to-emission ratio
As can be seen in Figure 3 the mineral-based building typologies have the highest carbon emissions from production, with the highest value (200 tC) for the “Reinforced Concrete” followed by the “Brick” building assembly (155 tC).
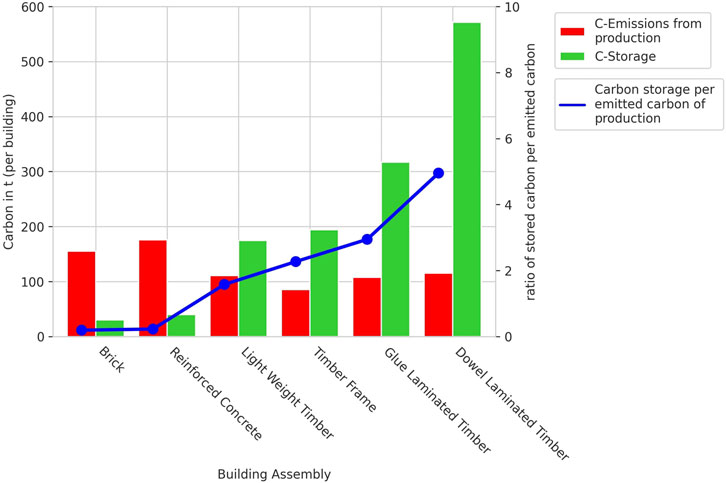
FIGURE 3. Carbon emissions from production (A1-A3) (left), carbon storage (right) and ratio of carbon storage and carbon emissions per building assembly (graph). Values from Table 1.
The timber-based building assemblies are ranked as the following: “Dowel Laminated Timber” (115 tC), “Light Weight Timber” (110 tC) and “Glue Laminated Timber” (107 tC). “Timber Frame” has the lowest production emissions (85 tC).
On average, the emissions of timber building assemblies are 32.8% lower than those of the “Brick” and 40.4% lower than of the “Reinforced Concrete” building assembly.
The timber-based building typologies, however, have higher carbon storage values (Figure 3). “Dowel Laminated Timber” has the highest carbon storage value with ∼571 tC, which is almost twice the carbon storage of “Glue Laminated Timber” which has a storage of ∼317 tC. “Brick” and “Reinforced Concrete” have substantially less storage with ∼30 and ∼40 tC (Table 2).
Therefore, these mineral-based building typologies have a much smaller Ratio of stored carbon per emitted carbon than the timber-based building typologies. This is not only because the emissions of the mineral-based construction typologies during production are much higher, but also because their carbon storage potential is six to 20-fold lower than that of the timber-based construction typologies.
While “Dowel Laminated Timber” has a ratio of 4.95, “Brick” and “Reinforced Concrete” have 0.19 and 0.2. Within the timber-based typologies, they rank as the following: “Dowel Laminated Timber”, “Glue Laminated Timber” with rd. 3, “Timber Frame” with rd. 2.3 and “Light Weight Timber” with rd. 1.6.
3.2 Contributions of materials to carbon emissions and storage
In this section the contributions of materials to the total weight, emissions and storage will be looked at, starting with the mineral-based building typologies. In order to show a concise overview, some materials were gathered into subgroups and are not listed individually in the pie charts. The complete list of materials, their grouping as well as individual contributions can be found in Supplementary Table S3 in the Supplementary Appendix.
For the “Brick” assembly, the non-organic materials (displayed in the grey coloring of the inner circles) outweigh the organic materials by far (Figure 4). Non-organic materials such as “brick” have the highest impact on the carbon emissions with a contribution of 37%. Other mineral-based materials, such as “mineral wool,” drive carbon emissions with a share of 11% of the total emissions despite constituting only 2% of the total weight. In contrast, more than half of the carbon storage is held by organic materials. Looking at the percentage organic materials are holding in total contribution to the weight, which is 2%, could explain why “Brick” has such a low carbon storage capacity.
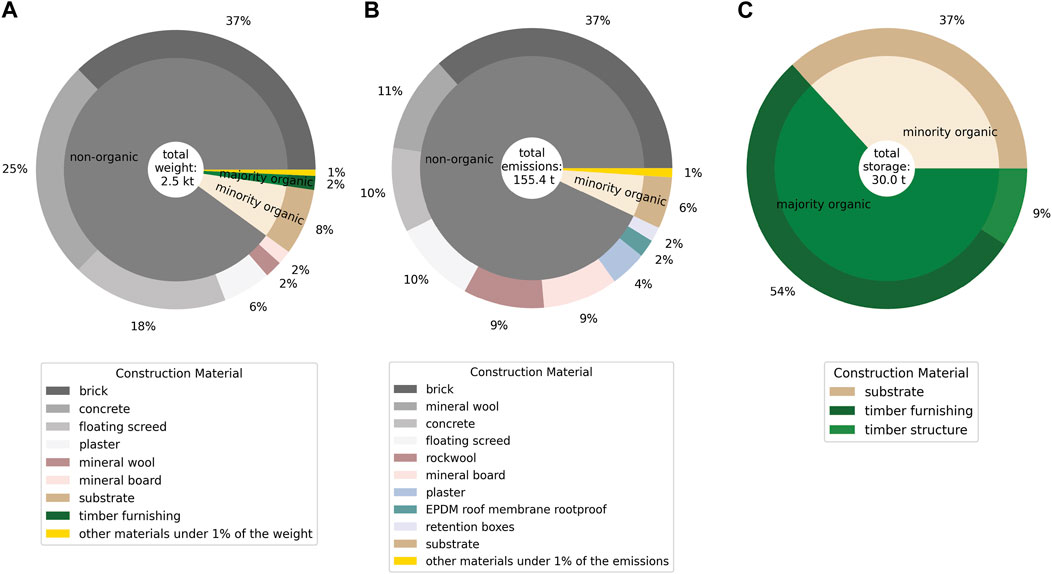
FIGURE 4. Brick Assembly: The pie charts display the (A) materials contribution to weight (B) Material contribution to carbon emissions (and C) Material contribution to carbon storage. The total weight (A), emissions (B) and storage (C) are displayed in the middle and the shares of non-organic and organic materials in the inner circle. The outer circles display the individual shares of the building materials. The contributions were calculate using the CCA model.
The “Reinforced Concrete” building assembly shows a similar picture, with slightly higher organic shares contributing to emissions with 8%. This building assembly is almost twice as heavy as the “Brick” building assembly, which could be due to the high amount of “reinforced concrete” (Figure 5). The carbon emissions are also dominated by “reinforced concrete” with 42%.
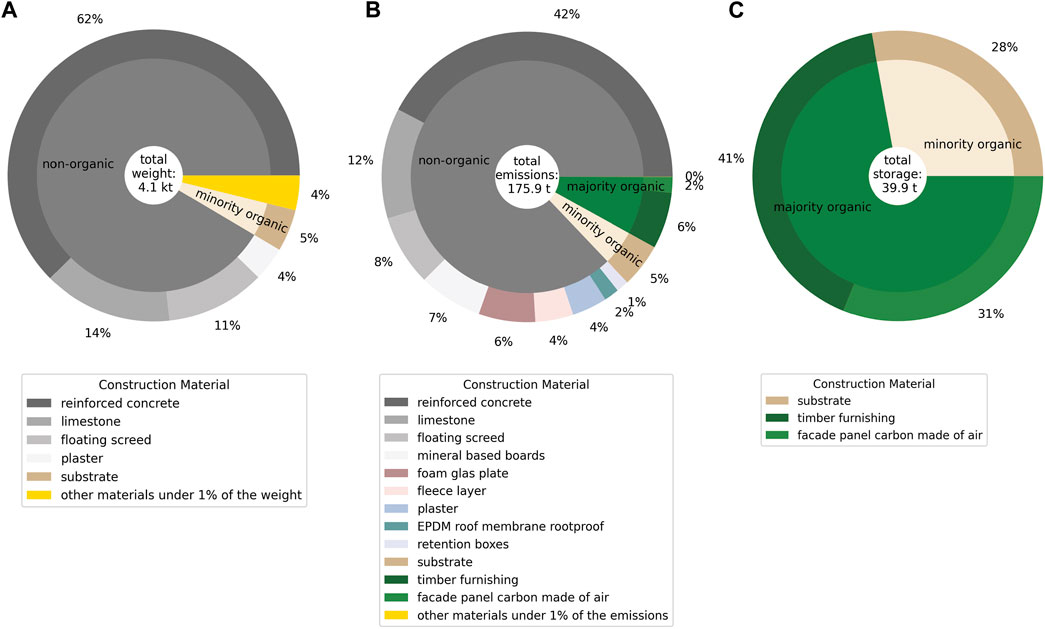
FIGURE 5. Reinforced Concrete Assembly: The pie charts display the (A) Material contribution to weight (B) Material contribution to carbon emissions (C) Material contribution to carbon storage. The total weight (A), emissions (B) and storage (C) are displayed in the middle and the shares of non-organic and organic materials in the inner circle. The outer circles display the individual shares of the building materials. The contributions were calculated using the CCA model.
The higher carbon storage (in comparison to “Brick”) is partly due to the higher share of organic materials in this building assembly but also because of the biochar-based materials “MOA”. This product stores high amounts of carbon over 70% by weight (Made of Air, 2023) but contributes little to the total weight of the building. Therefore, it is listed under the “other materials under 1% of the weight” category and can only be found in Figure 5©).
When examining Figures 6–9, it becomes evident that within timber-based building assemblies, organic materials constitute a significantly greater proportion of emissions compared to their mineral-based counterparts. Moreover, among the timber-based building typologies, the share of organic materials contributing to the overall weight is also higher. Notably, only in the case of the “Dowel Laminated Timber” assembly does the organic component account for more than half of the materials contributing to the total weight. This generally suggests that the higher the share of organic materials, the less carbon emissions and more carbon storage arises.
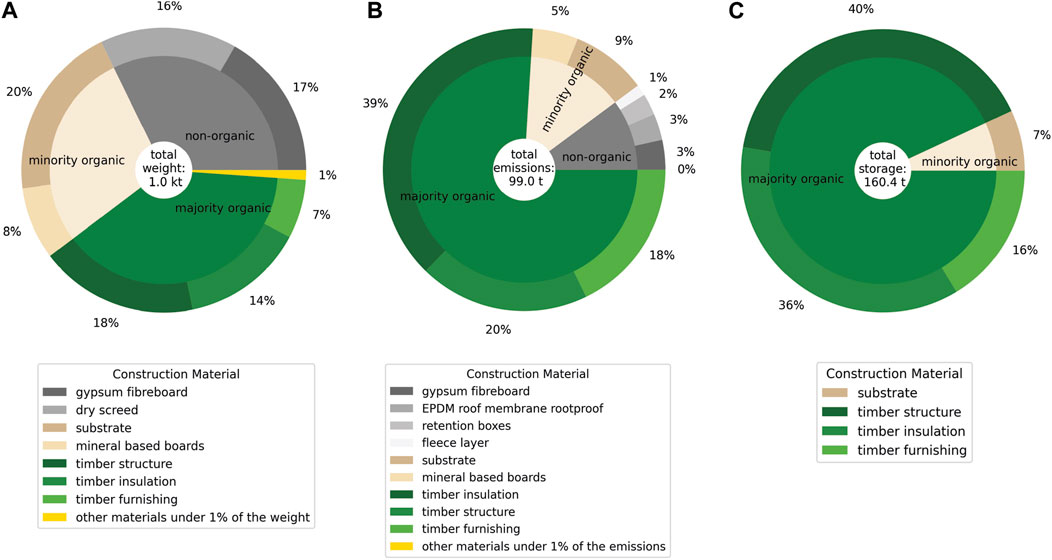
FIGURE 6. Light Weight Timber Assembly: The pie charts display the (A) Material contribution to weight (B) Material contribution to carbon emissions (C) Material contribution to carbon storage. The total weight (A), emissions (B) and storage (C) are displayed in the middle and the shares of non-organic and organic materials in the inner circle. The outer circles display the individual shares of the building materials. The contributions were calculate using the CCA model.
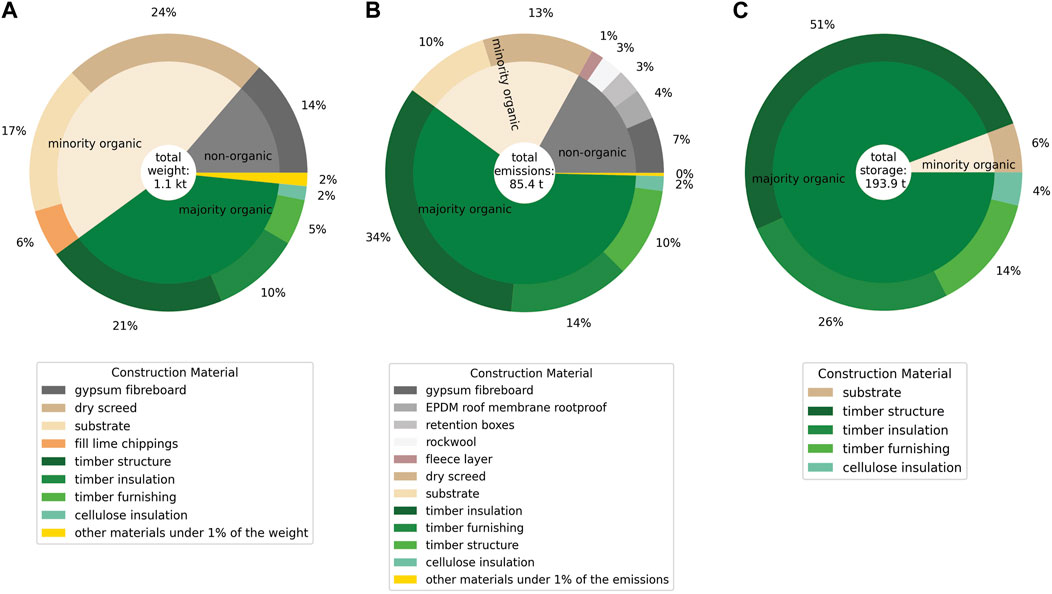
FIGURE 7. Timber Frame Assembly: The pie charts display the (A) Material contribution to weight (B) Material contribution to carbon emissions (C) Material contribution to carbon storage. The total weight (A), emissions (B) and storage (C) are displayed in the middle and the shares of non-organic and organic materials in the inner circle. The outer circles display the individual shares of the building materials. The contributions were calculate using the CCA model.
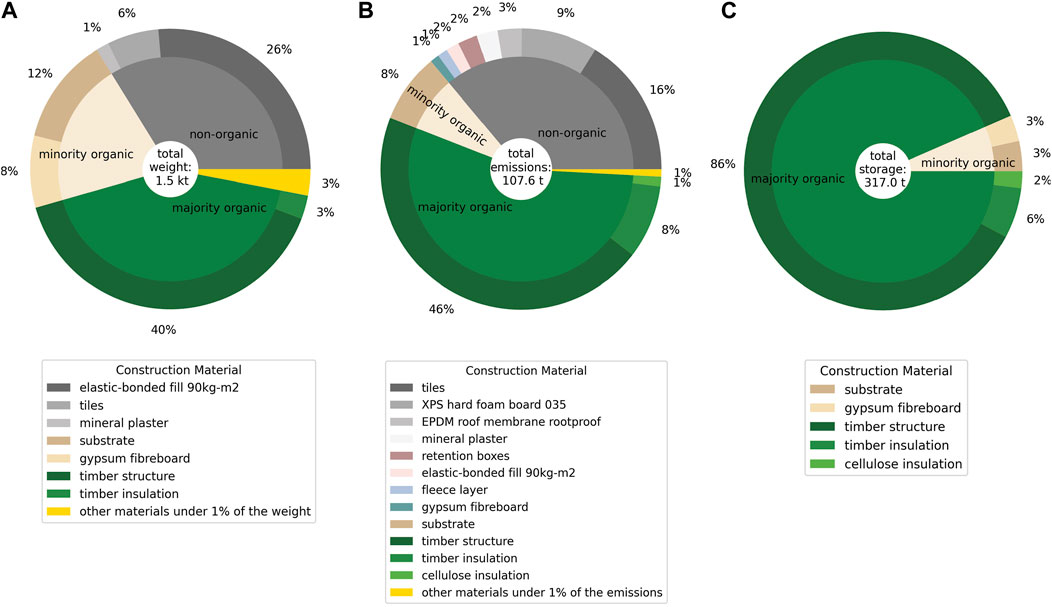
FIGURE 8. Glue Laminated Timber Assembly: The pie charts display the (A) Material contribution to weight (B) Material contribution to carbon emissions (C) Material contribution to carbon storage. The total weight (A), emissions (B) and storage (C) are displayed in the middle and the shares of non-organic and organic materials in the inner circle. The outer circles display the individual shares of the building materials. The contributions were calculate using the CCA model.
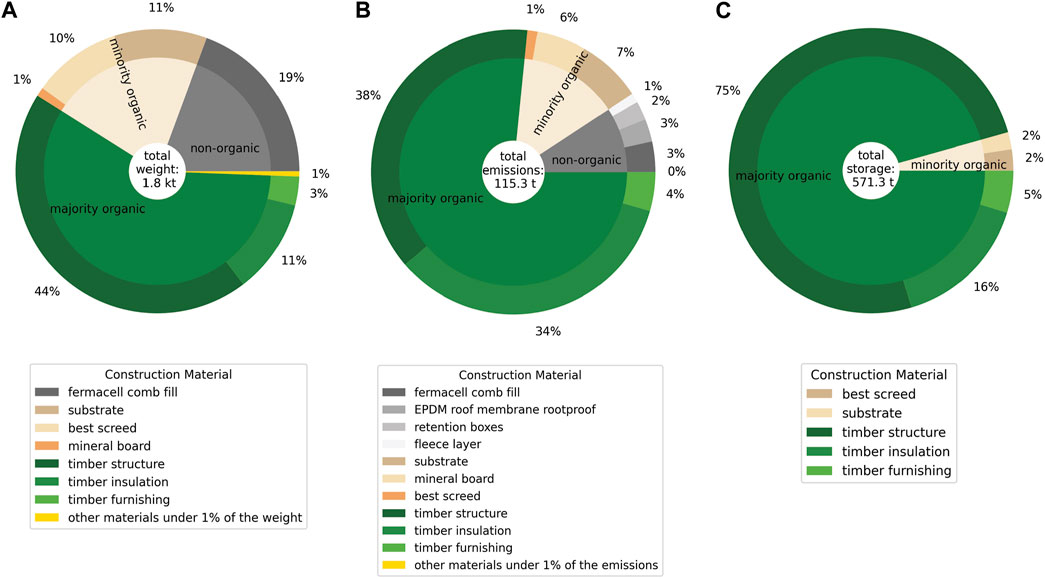
FIGURE 9. Dowel Laminated Timber Assembly: The pie charts display the (A) Material contribution to weight (B) Material contribution to carbon emissions (C) Material contribution to carbon storage. The total weight (A), emissions (B) and storage (C) are displayed in the middle and the shares of non-organic and organic materials in the inner circle. The outer circles display the individual shares of the building materials. The contributions were calculate using the CCA model.
Looking at the “Light Weight Timber” assembly, organic materials have a share of 39% of the total weight, 71% of the total emissions and 93% of the total storage (Figure 6). While many of the organic materials used in the construction have high carbon storage capacity, certain materials are nearly offset by their high carbon emissions. This applies, for example, to “wood fiber insulation board steico dry”, which stores only 1.81 t more than it emits during production. This material is included in the “timber insulation” material category.
For the “Timber Frame” assembly the organic materials have almost a similar share of organic materials to the total weight with 38%, while the share contributing to the total emissions is lower by rd. 10% in comparison to “Light Weight Timber”.
However, the high carbon storage capacity of the materials “wooden beam,” “osb,” and “wooden substructure,” which account for rd. 50% of the total storage should be emphasized. As can be seen in Figure 7, they are grouped under the category “timber structure”. In contrast to the “Light Weight Timber” assembly, especially the timber structure leads to less carbon emissions and considerably higher carbon storage. The positive effect on the ratio of stored carbon per emitted carbon is less attenuated by emissions than in the case of the materials with high carbon storage“in “Light Weight Timber”. This results in the better carbon ratio values “or “Timber Frame” than “Light Weight Timber”, which are shown in Figure 3.
Coming to the assessment of the “Glue Laminated Timber” building assembly, the results are broadly comparable to those of “Light Weight Timber”. It has the same distribution of organic and non-organic materials, however, outstanding values for the carbon storage of the timber structure “clt” change the overall picture. This material contributes to 81% of the total storage of this building assembly. In consequence, the storage capacity exceeds the emissions by far, as can be seen in Figure 8.
Regarding the building assembly “Dowel Laminated Timber,” which has the highest share (by weight) of organic materials compared to all building assemblies. As displayed in Figure 9 carbon emissions and carbon storage are highest for the category “timber structure” including materials like “thoma wood100”, “wood fibre insulation board bestwood schneider 180”, and “cross diagonally glued board layers”. They account for almost 80% of the total storage together. The carbon storage capacity for the “thoma wood100” is particularly high compared to all other materials. It is used in the ceiling and accounts for rd. 40% of the total storage.
3.3 Distribution of emissions and storage across all building components
The distribution of carbon emissions and storage among the different building components walls, ceiling slabs and roof can be seen in Figure 10. Across all building assemblies the ceiling has the highest carbon emissions and the highest storage. This could potentially be caused by the total weight of the ceilings, which are in total heavier than walls and the roof. The walls have the lowest amount of carbon emissions except for the “Dowel Laminated Timber” building assembly, where walls have slightly larger carbon emissions than roof.
However, in terms of carbon storage the walls store less carbon than the ceilings but more than the roof except for the “Brick” and “Light Weight Timber” building assemblies. The low storage values for the roof are due to the usage of materials, e.g., the “intensive substrate light” contributes much more to emissions than to carbon storage.
4 Discussion
4.1 Carbon storage and emissions throughout different timber building assemblies
This study compared four timber-based building typologies with different timber construction systems. It was shown that the mass timber assembly “Dowel Laminated Timber” has the most effective combination of building materials as it combines the highest amount of carbon stored within the construction at 571.26 t with the highest ratio of stored carbon to carbon emitted during the production phase at 4.95 (Table 1). These findings are in accordance with a study done by Amiri et al. (2020) who concluded that the amount of wooden materials within a building’s structure significantly influences the carbon storage capacity of its structure. In this present study the difference between both mass timber typologies mainly lies in the fact that “Dowel Laminated Timber” incorporates more solid wood components, as well as wooden dowels instead of chemical glues and lamination (Figures 9, 10), ensuring a higher percentage of organic material in the overall mass of the building. Generally, the results of this study are consistent with other reviews and studies, that using timber based building assemblies can reduce the carbon emissions in comparison to mineral based building assemblies. The comparison with other studies is limited because of inter alia the different scopes, study locations, origin of the LCI input data and carbon estimations. Therefore we refrained to comparing relations between the different building assemblies. Younis and Dodoo calculated with a Life Cycle Assessment a 40% reduction when using cross-laminated timber instead of concrete. This fits roughly into the reduction of ca. 60% from glue-laminated timber (clt) to concrete, we calculated. The smaller amount from Younis and Dodoo could be due to the relatively high amount of concrete in all of their building assemblies, that was significantly higher than the amount of concrete in our building assemblies (Younis and Dodoo, 2022). Jayalath et al. investigated the Life Cycle Assessment of two building types, reinforced concrete and cross-laminated timber, in different buildings for different areas of Australia and calculated similar values for the life cycle phases A1–A5 as we did. Their reinforced concrete building had ca. 50% more emissions than the cross-laminated timber building (Jayalath et al., 2020). Giving a benchmark for CO2 equivalents/m2 of the different assemblies is more difficult than just giving the percentage reductions. However, a study by Dodoo (2019) can be used to provide a comparative value for the different assemblies. In this study, the values for reinforced concrete of 254 CO2eq/m2 are significantly higher than our value of 126 CO2eq/m2 (Dodoo, 2019). Nevertheless, significantly more reinforced concrete was used in this study, and the mass fraction of 88% is remarkably higher than in our construction method (62%). The same applies to the timber construction methods compared. The reference values are between 156 and 125 CO2eq/m2 and therefore higher than ours (between 61 and 83 CO2eq/m2). The big difference here again is that reinforced concrete was used in every timber construction method and even accounts for the largest proportion of mass in all of them. Therefore, the higher emissions per square meter could potentially be caused by the reinforced concrete. Overall, the reduction between the average of all timber construction methods and reinforced concrete is also 44%.
One of the minority-organic materials, which contributes to the carbon storage of each of the building assemblies, is the intensive substrate light. This substrate is used as a growing medium for the green roofs which are part of all the building typologies. The carbon storage capacity of the substrate originates from an organic component within the substrate mixture. However, since the amount of that component is below 25% of the material’s weight it falls under our definition of a minority-organic material (Section 2.7). With the inclusion of green roofs within each assembly, there is the potential to increase the carbon storage of the building through future carbon uptake and storage by plants growing on the green roof. A recent review of green roof substrates highlighted the substantial gap in understanding the lifecycle of the substrate layer supporting sustainable vegetation in green roofs, because most experiments with substrates were conducted in greenhouse and laboratory conditions (Kader et al., 2022). Within all the timber-based building typologies, the green roof initially contributes up to ten % to the respective buildings carbon storage capacity. Therefore, the use of timber materials has a more substantial effect on carbon storage capacity in the exemplary four-storey building than the green roof. On the other hand, when looking at the same data for the mineral-based building typologies, the intensive substrate light accounts for a much larger portion of the total carbon storage capacity at 37% for “Brick” and 28% for “Reinforced Concrete” (Figures 4, 5). Taking this data into account, it can be concluded that the use of timber-based materials within a new building is a more efficient strategy to increase the carbon storage capacity of a building than just the implementation of green roofs.
Organic materials with high carbon storage, can thus potentially contribute to increasing the urban carbon stock. This positively impacts the carbon cycle as it prevents or delays the release of CO2 back into the atmosphere. This especially applies for multistorey buildings, as they make more efficient use of floor area per capita, preventing further soil sealing and allowing for greater carbon storage volumes by using more wooden materials (Churkina et al., 2020; Pittau et al., 2022).
However, our results are not only limited to the application of whole building concepts as they are presented in this study. Comparing the various materials’ carbon emissions during production and carbon storage potential it becomes evident, that a part-by-part substitution is also a valid strategy to incorporate more materials from renewable, biomass-based resources into otherwise mineral-based constructions. For example, conventional insulation materials such as rockwool could easily be substituted by wood fiber insulation products within, otherwise, mineral-based constructions.
4.2 Biogenic carbon
We were able to show that the overall carbon emissions of the six different building typologies vary substantially. The consideration of biogenic carbon within our analysis enables a clear comparison between the carbon emissions of timber-based and conventional mineral-based building materials during the production phase (life cycle stages A1–A3) without including the misleading fact that timber has a negative GWP to begin with due to the tree’s ability to sequester carbon within its biomass. The lack of distinction between the GWPs of timber-based materials and mineral-based materials has so far led to some discussion concerning their comparability (Andersen et al., 2021; Younis and Dodoo, 2022). By calculating and subtracting the approximate amount of carbon present in the source material from the given GWP it was ensured that the calculated CO2 coefficient includes all production emissions of the wooden materials. It can therefore be stated, that within the comparison of the six building typologies and the project scope of Schumacher Quartier the production of all materials of the mineral-based building typologies would result in 71–116 t more carbon emissions than that of the timber-based building typology with the least carbon emissions (“Timber Frame”) and even 40–85 t more carbon emissions than “Dowel Laminated Timber” which is the timber-based typology with the highest production carbon emissions (Figure 10). The calculated carbon storage of the building parts further proves the point that timber-based typologies secure more carbon within its material components than is emitted during their production (Figures 3, 10).
4.3 Limitations and uncertainties
Through the results of this study, it has become clear that the “Glue Laminated Timber” and “Dowel Laminated Timber” building assemblies have an especially high potential for carbon storage due to their increased use of clt and solid wood components resulting in a high amount of organic mass being locked within the building structure. At 1.5 kt and 1.8 kt of weight per building, these assemblies are also the heaviest of the four analyzed timber-based building assemblies. However, the increased mass of the mass timber building typologies can in turn become a hindrance if the additional emissions for product transport are taken into consideration. Transport to the construction site is addressed in stage A4 of life cycle assessments, which was not a focus of this study. Dzhurko and others recently found that carbon emissions during transport to the construction site have a notable influence on the overall carbon emissions (Dzhurko et al., 2024). In the specific case of Schumacher Quartier, the transport of materials used in the “Dowel Laminated Timber” building assembly accumulated to a point, where if put into context of carbon emissions during production and total carbon storage, “Dowel Laminated Timber’s” ratio decreased, to the point where it is barely outperforming the “Glue Laminated Timber” assembly (Dzhurko et al., 2024). This is due to the fact that materials used in the “Dowel Laminated Timber” building assembly are produced only by a specific manufacturer, Thoma Holz GmbH, located in Austria. Since, in the case of Schumacher Quartier, the distance between the factory and the production site is especially high and the building materials are especially heavy, the transport carbon emissions contribute significantly to the overall carbon ratio of the “Dowel Laminated Timber” building assembly. It is therefore advisable to consider the origin of the primary building materials as to keep transport carbon emissions of heavier materials as low as possible.
Another factor that needs to be taken into consideration is the expected service life and end-of-life stages of wooden construction. These are represented by Stages B and C in life cycle assessments which were not within the focus of this study. In a recent review Younis and Dodoo have concluded an average service life of 50–60 years for timber based constructions (Younis and Dodoo, 2022). Further research and long term studies are set to determine the durability of different types of wooden construction as well as the energy cost that might go into upkeep and maintenance of such buildings.
Concerning the comparison between organic and non-organic building assemblies it should be addressed, that this study focused on reinforced concrete and brick assemblies because they are still the widespread standard for construction projects of large building cooperatives, such as Schumacher Quartier. However, within the scope of non-organic building materials, there are some materials like rammed earth or stone that are proposed as more sustainable non-organic building materials. Fernandes et al. were able to show that rammed earth bricks for construction have significantly less embodied energy than conventional fired brick structures. Nevertheless, to reach comparable mechanical strength the material needs to be combined with stabilizers and binder materials such as cement or lime which then in turn affect the environmental impact (Fernandes et al., 2019). Structural stone is another viable possibility for non-organic construction as it has sufficient mechanical strength and is naturally available without much material preparation. Kerr et al. (2022) found that GWP values for structural stone were 45%–75% lower than the compared concrete products and even 99% lower than the compared steel products.
Moreover, the carbonation process in cement, as elucidated by Cao et al. (2020) highlights an often-overlooked environmental benefit where cement-based materials, through their lifecycle, reabsorb a significant proportion of CO2 from the atmosphere, thus partially offsetting their initial carbon footprint. However, unlike stone, earth or cement, timber has the further advantage that it is a renewable resource that actively stores carbon during its growth and to a greater extent than the abovementioned non-organic construction materials.
Finally, the results in this study present a case study that was done in Germany and can therefore only be applied to Central European standards and the current state of research and information available. It can be acknowledged that a similar construction project in a different area where climatic or economic situation differ from this case study might face a different set of hindrances. Colder or hotter climate, regular extreme weather events, or proximity to tectonic plates boundaries may impose special requirements for a building structure, for example, a need for an increased insulation, water repellency or seismic sensitive design (Liu et al., 2016). It should also be noted that a change of study area can have different implications for the energy demand and subsequent emissions depending on material availability and the area’s energy mix (Younis and Dodoo, 2022).
5 Conclusion
The results of this study demonstrated that switching from conventional mineral-based building materials towards biomass-based materials such as timber has a significant impact on the reduction of carbon emissions during the production stage of a building. It was shown that such new, timber-based building typologies have a high potential for carbon storage within the primary structure of the building, which can contribute to the carbon sink capacity of residential areas or cities in general. This effect is further increased by the inclusion of a green roof structure. The dynamic nature of green roofs can further enhance the carbon storage of the buildings during its later life stages as plants grow and additional biomass accumulates on the roof area. The results of this study provide concise proof for the benefits of using timber-based materials instead of conventional mineral-based ones such as reinforced concrete and bricks during the production stage. Further research is needed to assess the carbon emissions that occur during the buildings’ later life stages like maintenance and repair activities. A cascade-usage approach can be recommended in which the timber-based building materials are deconstructed and reused multiple times before thermal or biological decomposition in order to keep the sequestered carbon stored within the material for as long as possible. Mass timber building modules are often mechanically connected, for example, by use of screws. This facilitates the cascade-usage as it enables deconstruction, as opposed to chemically bound materials in more conventional building approaches. Concurrently with this the material mix and use of mineral-based layers within the two light frame typologies is thought to complicate the separation of materials and therefore thwarts or impedes cascade usage of these materials. Lastly, it should be added that a shift towards the use of more timber for building construction can only be beneficiary to the climate if the harvesting of timber is done in a way that is non-exploitive and sustainable. Deforestation of larger forest segments is known to negatively affect forest productivity and resilience. It is therefore crucial that policymakers ensure that timber is sourced in a sustainable manner.
Data availability statement
The original contributions presented in the study are included in the article/Supplementary Material, further inquiries can be directed to the corresponding author.
Author contributions
DD: Writing–original draft, Data curation, Investigation. BH: Writing–original draft, Data curation, Investigation. AH: Writing–original draft, Data curation, Investigation. LK: Writing–original draft, Data curation, Investigation. NK: Writing–original draft, Data curation, Investigation, Visualization. FL: Writing–original draft, Data curation, Investigation, Writing–review and editing. AM: Writing–original draft, Data curation, Investigation. LM: Writing–original draft, Data curation, Investigation, Visualization, Writing–review and editing. NN: Writing–original draft, Data curation, Writing–review and editing, Investigation. AN: Writing–original draft, Data curation, Investigation, Visualization. HP: Writing–original draft, Data curation, Investigation. LS: Writing–original draft, Data curation, Investigation, Writing–review and editing. PV: Writing–original draft, Data curation, Investigation. KW: Writing–original draft, Data curation, Investigation. FT: Conceptualization, Writing–original draft. CB: Writing–original draft, Conceptualization, Methodology, Validation. GC: Funding acquisition, Project administration, Supervision, Writing–original draft, Conceptualization, Methodology, Validation.
Funding
The author(s) declare that financial support was received for the research, authorship, and/or publication of this article. This research work was supported by the Tegel Projekt GmbH through the project “Forest, cities and their carbon cycles”. Funding was provided to get insights into local forestry and timber manufacturing practices. We acknowledge support by the German Research Foundation and the Open Access Publication Fund of TU Berlin.
Acknowledgments
We would like to thank Sebastian Schubert for his contribution to the development of the CCA Numerical Algorithm. Other thanks go out to the team of the Tegel Projekt GmbH, in particular Stephanie Ambrosius-Groß for kindly giving insights into the plans of Schumacher Quartier and offering data for our research. Furthermore, we want to thank Dirk Riestenpatt from Berliner Forsten as well as Falk Stähr, Ulrike Hagemann and Stefan Kruppke of Landesforstbetrieb Brandenburg for giving us informative insights into the local forestry practices and management. Our thanks also go to Burkhardt Schröder from the company Max Holzbau and Jan Pfeiffer, Bernd Ebert and Volker Berg from Binderholz Baruth, Brandenburg who fostered our interests in the field of wooden constructions. We are grateful to Yuma Amecke, Moritz Zafir Balk, Tatjana Balschus, Simon Barchewitz, Nary Götze, Melina Höfling, Emma Krög, Leonardo Ochoa, Maren Roos, and Oliver Simon from the Technical University of Berlin for the groundwork and preliminary data they collected for the Schumacher Quartier. We are particularly grateful to the reviewers for their timely and insightful comments that have helped shape the final version of this paper.
Conflict of interest
Author FT was employed by Tegel Projekt GmbH.
The remaining authors declare that the research was conducted in the absence of any commercial or financial relationships that could be construed as a potential conflict of interest.
Publisher’s note
All claims expressed in this article are solely those of the authors and do not necessarily represent those of their affiliated organizations, or those of the publisher, the editors and the reviewers. Any product that may be evaluated in this article, or claim that may be made by its manufacturer, is not guaranteed or endorsed by the publisher.
Supplementary material
The Supplementary Material for this article can be found online at: https://www.frontiersin.org/articles/10.3389/fbuil.2024.1330105/full#supplementary-material
References
Ambrosius-GroB, S., Thoma, F., and Churkina, G. (2023). Klimaneutral Bauen in der Urban tech republic [presentation]. Berlin: Tegel Projekt GmbH.
Amiri, A., Ottelin, J., Sorvari, J., and Junnila, S. (2020). Cities as carbon sinks—classification of wooden buildings. Environ. Res. Lett. 15 (9), 094076. doi:10.1088/1748-9326/aba134
Andersen, C. E., Rasmussen, F. N., Habert, G., and Birgisdóttir, H. (2021). Embodied GHG emissions of wooden buildings—challenges of biogenic carbon accounting in current LCA methods. Front. Built Environ. 7, 729096. doi:10.3389/fbuil.2021.729096
Arehart, J. H., Hart, J., Pomponi, F., and D’Amico, B. (2021). Carbon sequestration and storage in the built environment. Sustain. Prod. Consum. 27, 1047–1063. doi:10.1016/j.spc.2021.02.028
BBSR (2021). Grundsätze zur Aufnahme von Ökobilanzdaten in die Online-Datenbank ÖKOBAUDAT. Bundesamt für Bauwesen und Raumordnung. Available at: https://www.oekobaudat.de/fileadmin/downloads/Einreichung/2021-10-04_Grundsaetze_OEBD_A1_A2.pdf.
BMWSB (2023). Ökobaudat [database]. ÖKOBAUDAT informationsportal nachhaltiges bauen. Available at: https://www.oekobaudat.de/no_cache/datenbank/suche.html.
Bovea, M. D., Ibáñez-Forés, V., and Agustí-Juan, I. (2014). “Environmental product declaration (EPD) labelling of construction and building materials,” in Eco-efficient construction and building materials (Amsterdam, Netherlands: Elsevier). doi:10.1533/9780857097729.1.125
Bowyer, J., Bratkovich, S., Frank, M., Howe, J., Stai, S., and Fernholz, K. (2012). Carbon 101: understanding the carbon cycle and the forest carbon debate. United States: Dovetails Partners.
Bundesministerium für Wohnen (2023). in Stadtentwicklung und Bauwesen (Handreichung Holzbauinitiative). (S. 21).
Cao, Z., Myers, R. J., Lupton, R. C., Duan, H., Sacchi, R., Zhou, N., et al. (2020). The sponge effect and carbon emission mitigation potentials of the global cement cycle. Nat. Commun. 11 (1), 3777. doi:10.1038/s41467-020-17583-w
Chen, C. X., Pierobon, F., Jones, S., Maples, I., Gong, Y., and Ganguly, I. (2021). Comparative life cycle assessment of mass timber and concrete residential buildings: a case study in China. Sustainability 14 (1), 144. doi:10.3390/su14010144
Churkina, G., Bothe, C., and Schubert, S. (2023). Carbon cycle assessment. Available at: https://git.tu-berlin.de/urbanoikos/models/cca.
Churkina, G., Organschi, A., Reyer, C. P. O., Ruff, A., Vinke, K., Liu, Z., et al. (2020). Buildings as a global carbon sink. Nat. Sustain. 3 (4), 269–276. doi:10.1038/s41893-019-0462-4
Dodoo, A. (2019). Lifecycle impacts of structural frame materials for multi-storey building systems. J. Sustain. Archit. Civ. Eng. 24 (1), 17–28. doi:10.5755/j01.sace.24.1.23229
Duan, Z., Huang, Q., Sun, Q., and Zhang, Q. (2022). Comparative life cycle assessment of a reinforced concrete residential building with equivalent cross laminated timber alternatives in China. J. Build. Eng. 62, 105357. doi:10.1016/j.jobe.2022.105357
Dzhurko, D., Haacke, B., Haberbosch, A., Köhne, L., König, N., Lode, F., et al. (2024). Forest, city and their carbon cycle – quantification of the carbon impacts of different construction types for Schumacher Quartier. Berlin: Urban Tech Republic.
Elhacham, E., Liad, B.-U., Grozovski, J., Bar-On, Y. M., and Milo, R. (2020). Global human-made mass exceeds all living biomass. Nature 588, 442–444. doi:10.1038/s41586-020-3010-5
Erb, K. -H., Haberl, H., Le Noe, J., Tappeiner, U., Tasser, E., Gingrich, S., et al. (2022). Changes in perspective needed to forge “no-regret” forest-based climate change mitigation strategies. GCB Bioenergy 14. doi:10.1111/gcbb.12921
Fernandes, J., Peixoto, M., Mateus, R., and Gervásio, H. (2019). Life cycle analysis of environmental impacts of earthen materials in the Portuguese context: rammed earth and compressed earth blocks. J. Clean. Prod. 241, 118286. doi:10.1016/j.jclepro.2019.118286
Figl, H., and Kusche, O. (2021). ÖKOBAUDAT-Handbuch Technisch/formale Informationen und Regeln zur ÖKOBAUDAT-Datenbank. Bundesinstitut für Bau-, Stadt-und Raumforschung (BBSR) im Bundesamt für Bauwesen und Raumordnung (BBR). Available at: https://www.oekobaudat.de/fileadmin/downloads/2021-11-29_OEBD-Handbuch_v2.1.pdf.
Hart, J., and Pomponi, F. (2020). More timber in construction: unanswered questions and future challenges. Sustainability 12 (8), 3473. doi:10.3390/su12083473
Heckmann, M., and Glock, C. (2023). Ökobilanz im Bauwesen – treibhausgasemissionen praxisüblicher Deckensysteme. Beton- Stahlbetonbau 118 (2), 110–123. doi:10.1002/best.202200102
Hildebrandt, J., Hagemann, N., and Thrän, D. (2017). The contribution of wood-based construction materials for leveraging a low carbon building sector in europe. Sustain. Cities Soc. 34, 405–418. doi:10.1016/j.scs.2017.06.013
Hurteau, M. D. (2021). “The role of forests in the carbon cycle and in climate change,” in Climate change (S. 561–579) (Elsevier). doi:10.1016/B978-0-12-821575-3.00027-X
IEA (2022). CO2 emissions. Buidlings. Sectoral overview. Available at: https://www.iea.org/reports/buildings.
Jayalath, A., Navaratnam, S., Ngo, T., Mendis, P., Hewson, N., and Aye, L. (2020). Life cycle performance of Cross Laminated Timber mid-rise residential buildings in Australia. Energy Build. 223, 110091. doi:10.1016/j.enbuild.2020.110091
Kader, S., Chadalavada, S., Jaufer, L., Spalevic, V., and Dudic, B. (2022). Green roof substrates—a literature review. Front. Built Environ. 8, 1019362. doi:10.3389/fbuil.2022.1019362
Kerr, J., Rayburg, S., Neave, M., and Rodwell, J. (2022). Comparative analysis of the global warming potential (GWP) of structural stone, concrete and steel construction materials. Sustainability 14 (15), 9019. doi:10.3390/su14159019
Lauk, C., Haberl, H., Erb, K.-H., Gingrich, S., and Krausmann, F. (2012). Global socioeconomic carbon stocks in long-lived products 1900–2008. Environ. Res. Lett. 7 (3), 034023. doi:10.1088/1748-9326/7/3/034023
Liu, Y., Guo, H., Sun, C., and Chang, W.-S. (2016). Assessing cross laminated timber (CLT) as an alternative material for mid-rise residential buildings in cold regions in China—a life-cycle assessment approach. Sustainability 8 (10), 1047. doi:10.3390/su8101047
Made of Air (2023). Made of Air. Learn how we put carbon to work. Available at: https://www.madeofair.com/our-process.
Martin, A. R., Doraisami, M., and Thomas, S. C. (2018). Global patterns in wood carbon concentration across the world’s trees and forests. Nat. Geosci. 11 (12), 915–920. doi:10.1038/s41561-018-0246-x
Organschi, A., Ruff, A., Carbone, C., Herrmann, E., and Oliver, C. (2016). “Timber city: growing an urban carbon sink with glue, screws, and cellulose fiber,” in World Conference on Timber Engineering, Vienna, Austria, August 22-25, 2016.
Pittau, F., Habert, G., Savi, D., and Klinger, M. (2022). Holzbau als Kohlenstoffspeicher – potenzial des Schweizer Gebäudeparks: synthesebericht (S. 34). ETH Zurich. doi:10.3929/ETHZ-B-000554239
Rinne, R., Ilgın, H. E., and Karjalainen, M. (2022). Comparative study on life-cycle assessment and carbon footprint of hybrid, concrete and timber apartment buildings in Finland. Int. J. Environ. Res. Public Health 19 (2), 774. doi:10.3390/ijerph19020774
Schenk, D., and Amiri, A. (2022). Life cycle energy analysis of residential wooden buildings versus concrete and steel buildings: a review. Front. Built Environ. 8, 975071. doi:10.3389/fbuil.2022.975071
Svatoš-Ražnjević, H., Orozco, L., and Menges, A. (2022). Advanced timber construction industry: a review of 350 multi-storey timber projects from 2000–2021. Buildings 12 (4), 404. doi:10.3390/buildings12040404
Verein Deutscher Zementwerke (2023). Zementindustrie im Überblick 2023/2024. Available at: https://www.vdz-online.de/fileadmin/wissensportal/publikationen/zementindustrie/zementindustrie_ueberblick/VDZ_Zementindustrie_im_Ueberblick_2023-2024.pdf.
Keywords: carbon storage, carbon emissions, wood construction, timber building, built environment, brick, reinforced concrete, comparative analysis
Citation: Dzhurko D, Haacke B, Haberbosch A, Köhne L, König N, Lode F, Marx A, Mühlnickel L, Neunzig N, Niemann A, Polewka H, Schmidtke L, Von der Groeben PLM, Wagemann K, Thoma F, Bothe C and Churkina G (2024) Future buildings as carbon sinks: Comparative analysis of timber-based building typologies regarding their carbon emissions and storage. Front. Built Environ. 10:1330105. doi: 10.3389/fbuil.2024.1330105
Received: 30 October 2023; Accepted: 04 March 2024;
Published: 18 March 2024.
Edited by:
Graziano Salvalai, Polytechnic University of Milan, ItalyReviewed by:
Viktoria Mannheim, University of Debrecen, HungaryAlastair T. M. Marsh, University of Leeds, United Kingdom
Copyright © 2024 Dzhurko, Haacke, Haberbosch, Köhne, König, Lode, Marx, Mühlnickel, Neunzig, Niemann, Polewka, Schmidtke, Von der Groeben, Wagemann, Thoma, Bothe and Churkina. This is an open-access article distributed under the terms of the Creative Commons Attribution License (CC BY). The use, distribution or reproduction in other forums is permitted, provided the original author(s) and the copyright owner(s) are credited and that the original publication in this journal is cited, in accordance with accepted academic practice. No use, distribution or reproduction is permitted which does not comply with these terms.
*Correspondence: Galina Churkina, churkina@tu-berlin.de
†These authors have contributed equally to this work