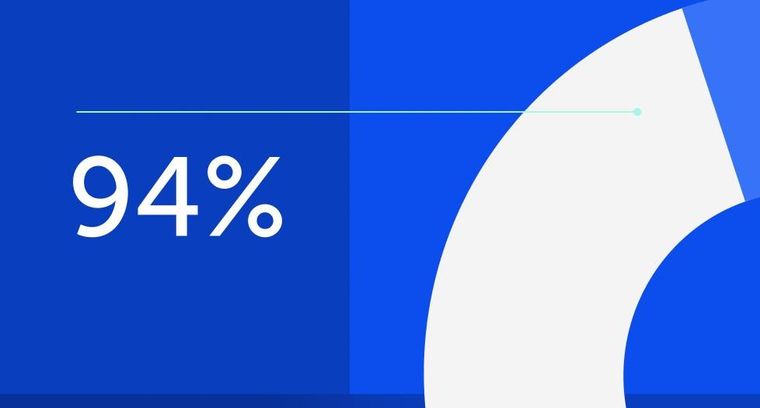
94% of researchers rate our articles as excellent or good
Learn more about the work of our research integrity team to safeguard the quality of each article we publish.
Find out more
REVIEW article
Front. Built Environ., 20 June 2023
Sec. Sustainable Design and Construction
Volume 9 - 2023 | https://doi.org/10.3389/fbuil.2023.1211131
This article is part of the Research TopicResilience of the Built Environment to Climate ChangeView all 5 articles
The urgent need to mitigate carbon dioxide (CO2) emissions and address climate change has led to increasing interest in renewable energy technologies. There are other promising energy generation systems, including photovoltaic/thermal (PV/T) systems. This paper provides a comprehensive review of PV/T systems for CO2 mitigation applications. PV/T systems are reviewed according to their principles, their design configurations, and their performance characteristics. Various types of PV/T systems, including flat-plate, concentrating, hybrid, and novel designs, are discussed, along with their advantages and limitations. In addition to examining PV/T systems as part of the integration of building systems with renewable energy sources and energy storage technologies. Furthermore, the environmental and economic aspects of PV/T systems, as well as their potential for CO2 mitigation in various applications such as residential, commercial, industrial, and agricultural sectors, are critically analyzed. Finally, future research directions and challenges in the field of PV/T systems for CO2 mitigation are outlined. The purpose of this review is to provide researchers, policymakers, and practitioners with information on how PV/T systems can be applied to reduce CO2 emissions and promote sustainable building design.
Photovoltaic/thermal systems, as shown in Figure 1, are becoming increasingly popular as they supply both electricity and hot air or water, and subsequently, reduce energy costs, and alleviate carbon dioxide emissions (Al-Waeli et al., 2017). This system consists of a PV module connected to pipes, which circulate fluid through them and cool the PV module. The result of the previous operation is more electricity and thermal energy production. These systems are easy to install at homes, commercial buildings, and industries with minimal maintenance. Not to mention, they are highly efficient and reliable, making them a great source of clean energy (Sopian et al., 2020). PV/T can be employed in residential and commercial structures (Moreno et al., 2021), solar water heating (Obalanlege et al., 2022), industrial processes (Sornek et al., 2023), desalination of water (Al-Hrari et al., 2020), farming (Wang et al., 2020), and solar cooling (Mohsenzadeh and Hosseini, 2015), etc. A hybrid system can also be created by combining PV/T systems with other renewable energy sources, such as wind and biomass (Al-Waeli et al., 2017). Electric vehicles are also a potential use for PV/T (Al-Waeli et al., 2019a). From the other side, Under the title Carbon Dioxide Capture and Mitigation, it describes various industrial processes and technologies that capture and store carbon dioxide. This is done in order to reduce the amount of CO2 released into the atmosphere and therefore mitigate the effects of climate change. These processes include carbon capture and storage, direct air capture, bioenergy with carbon capture and storage, and afforestation/reforestation (McLaughlin et al., 2023). Each of these methods has its own set of challenges and potential benefits. CO2 and energy efficiency are a concern and interest for industries, as they emit high “greenhouse gases” (GHG) such as CO2. International treaties such as Kyoto Protocol motivated many companies and industries to reduce their CO2 emissions (Shu et al., 2023). There are many methods used to mitigate CO2.
According to Yang et al. (Yang et al., 2023), BIPV systems are more energy efficient and perform better under different environmental conditions. The authors analyze factors such as solar radiation, temperature, wind speed, and humidity to identify the most suitable design parameters. Nevertheless, the optimization described in this paper was limited to a specific range of values for both Uin “U-value of the window” and SHGCout “solar heat gains coefficient of the outer window”. The simulation software used in this study (TRNSYS) relied on the International Glazing Database. It would be beneficial to explore a broader database of window components specifically tailored for BIPV-DSF in the future to enhance the optimization process. The research findings provide valuable insights into optimizing the BIPV double-skin facade for specific climate zones, contributing to the development of sustainable and energy-efficient building designs. The study is published in the journal Buildings.
Peng et al. (Peng et al., 2013) investigated the thermal performance of a unique “photovoltaic double-skin façade” (PV-DSF) in the context of Hong Kong. The study involves conducting experiments to evaluate the effectiveness of the PV-DSF in terms of its thermal performance. The authors analyze parameters such as heat transfer, solar radiation, and ambient temperature to assess the facade’s ability to regulate indoor temperature and reduce energy consumption. This study suggests that with a double skin facade system that consists of a single clear pane on the inside and a double reflective pane on the outside, the building cooling energy consumption can be reduced by 26 percent compared to traditional single-face facades with one absorptive glazing. PV-DSF in a specific geographical setting was evaluated in this study and valuable insights were gained.
Rounis et al. (Rounis et al., 2022) modelled convection in BIPV/T systems using an advanced approach. The study focuses on improving the accuracy of modelling convective heat transfer within BIPV/T systems. The authors propose a methodology that combines “Computational Fluid Dynamics” (CFD) simulations and experimental data to develop a more reliable and precise model. The paper discusses the implementation and validation of the proposed approach, highlighting its advantages in capturing convective heat transfer effects within BIPV/T systems. The air temperature rises predictions obtained from the suggested modelling approach demonstrated a strong correlation with the experimental outcomes, as indicated by an R2 value of 0.93. The proposed methodology can be customized for specific systems and climates by calibrating it through monitoring key temperatures. It has the potential to play a significant role in optimizing control strategies and enhancing heat utilization within a coupled system of BIPV/T and “heating, ventilation, and air conditioning” (HVAC). The research contributes to the advancement of modelling techniques for BIPV/T systems.
An active and passive effects analysis of BIPV/T systems’ on building facades has been conducted by Athiens et al. (Athienities et al., 2018). The study focuses on dynamic modelling and simulation techniques to evaluate the BIPV/T systems’ achievable performance and energy savings. The authors investigate both active and passive functions of BIPV/T systems, which include suppling electrical power and contributing to heat generation. An extensive case study is carried out on a multi-floor building located in several European locations with diverse climatic conditions in order to estimate the numerical model effectiveness and the viability of the system under investigation. Based on the height of the building, a parametric and comparative analysis is also conducted to assess active and passive effects. Heating demand can be reduced significantly by using BIPV/T systems, according to simulation results. Variations in thermal and electrical efficiency are caused by both passive and active effects. According to the innovative system, heating and cooling energy consumption were reduced by 56.8%–104.4% across all weather zones examined. This is close to the zero energy standards for buildings in the southern climatic regions. The study was analyzed in depth the dynamic behaviour of BIPV/T systems and provides insights into their potential benefits for energy efficiency in buildings.
Renno et al. (Renno et al., 2019) investigated the performance of a “concentrated photovoltaic/thermal” (CPV/T) system integrated with a DC system, specifically for meeting the energy demands of a supermarket. CPV/T-DC system efficiency and performance under different operating conditions are the subjects of the study. It explores the potential of this integrated system to efficiently generate both electricity and heat for the supermarket. In addition to electrical, thermal, and total efficiencies, the authors also examined the system’s energy output. The research employs a combination of experimental measurements and numerical simulations to evaluate the system’s performance. Various factors affecting the system’s operation, such as solar irradiance, ambient temperature, and system configuration, are taken into account during the analysis. The study’s results indicated that the CPV/T-DC integrated system exhibits favourable performance characteristics, including high electrical and thermal efficiencies. The system demonstrates promising potential in meeting the energy requirements of a supermarket by simultaneously providing electricity and thermal energy for heating or cooling applications.
This paper proposed and revises PV/T system applications and utilized solar energy for advance CO2 capturing techniques. The paper aims to give an outline of how PV/T hybrid solar collectors can contribute to reducing CO2 emissions. It covers the history, concept, and advantages of the PV/T system. It also employs different methods to explain the various types of PV/T systems. Moreover, it evaluates and talks about techniques for capturing and mitigating CO2. Finally, it examines how electrical and thermal energy from PV/T systems can be utilized in CO2 capture and mitigation applications which is one of the mechanisms to be resilience to climate change through sustainable built environment.
Here are the steps in the research methodology:
• Identify and gather relevant sources: a thorough search was conducted of academic databases, research journals, conference proceedings, and reputable sources to identify literature related to “PV/T systems”, and “CO2 mitigation” as a main keyword. Also, renewable energy technologies, system configurations, performance characteristics, integration with other energy sources, and environmental/economic aspects has been considered.
• Review and analyze literature: an evaluation of the selected literature, extracting key information on PV/T system principles, design configurations, performance characteristics, advantages, limitations, integration options, and CO2 mitigation potential. In addition, identifying gaps, inconsistencies, and areas for further exploration.
• Organize and synthesize findings: the reviewed literature has been organized based on different aspects of PV/T systems, such as system types (flat-plate, concentrating, hybrid, novel designs), integration with renewable energy sources and energy storage technologies, environmental and economic considerations, and application sectors (residential, commercial, industrial, agricultural).
• Analyze and interpret data: the gathered information, identify trends, patterns, and relationships, and draw conclusions about the current state of PV/T systems for CO2 mitigation has been analyzed. The strengths, limitations, and potential challenges associated with the technology was identified.
• Identify research gaps and future directions: Based on the analysis, identify research gaps, unanswered questions, and areas where further investigation is needed. The potential future research directions and challenges in the field of PV/T systems for CO2 mitigation was outlined.
• Present and discuss findings: the paper structured to present a clear overview of PV/T systems, their design, performance, integration, environmental and economic aspects, CO2 mitigation potential, and applications. The implications of the findings and their relevance for researchers, policymakers, and practitioners interested in promoting sustainable built environments and addressing CO2 emissions was discussed.
• Conclusions and suggestions future work: the main findings, restate the importance of PV/T systems for CO2 mitigation was summarized, and recommendations for future research and development in this field where proposed.
Sunlight is converted into electricity by a solar cell, also known as a photovoltaic (PV) cell. Using the PV effect, it directly converts light energy into electrical energy. When light shines on a solar cell, it can cause electrons in the semiconductor material to be excited and move, creating an electric current (Kazem et al., 2013). A PV system typically consists of a number of solar cells connected together to form a solar panel or module as shown in Figure 2A. Depending on the desired voltage and current output characteristics, these modules can be connected in series or parallel as shown in Figure 2B. A complete PV system may also include other components such as batteries, charge controllers, inverters, and monitoring systems.
Monocrystalline (or single crystalline), polycrystalline, and thin-film solar cells are all types of solar cells. The efficiency and durability of monocrystalline cells are attributed to their single crystal of silicon. Compared to monocrystalline cells, polycrystalline cells are less expensive, but less efficient. They are made from multiple crystals of silicon. Compared to crystalline cells, thin-film cells are more flexible and lightweight and make use of a thin layer of semiconductor material.
PV technology has become increasingly popular in recent years as solar panels’ cost has decreased and the efficiency and reliability of solar cells have improved. Among the applications of PV systems are residential and commercial electricity production, remote power supply for telecommunications systems and other off-grid applications, and portable power for camping and other outdoor activities (Kazem et al., 2014).
Different parameters used to evaluate PV performance such as “Fill Factor” (FF), and “Efficiency” (ɳ) as follows:
Imp and Vmp are the current and voltage at maximum power, Isc and Voc are the short circuit current and open circuit voltage. Pinc is the incident solar energy.
There are two types of solar thermal collectors, concentrating and non-concentrating, with non-concentrating accounting for 89.6% of global volume in 2016. As the product’s application scope expands into industrial applications, this segment is expected to grow at the fastest rate (Saini et al., 2023). Utilizing abundant solar energy to produce thermal energy that fits the end-user demands is an increasingly popular way of providing cost-effective, environmentally friendly heat energy. Solar collectors convert solar radiation into heat in solar thermal energy systems. Various uses are then made of thermal energy, including water heating, space heating, and industrial process heating. The cost and efficiency of solar thermal energy systems are improving, making them a viable option for many households and businesses. Additionally, utilizing solar energy for thermal energy production eliminates the need for burning fossil fuels, reducing emissions, and promoting sustainability.
Based on the temperature of the working fluid, solar thermal collectors can also be classified into three main categories as shown in Figure 3 (Al-Waeli et al., 2017):
I. Low-temperature collectors: These are used for applications that require temperatures up to 80°C, such as space heating, domestic hot water, and pool heating. Examples of low-temperature collectors include flat-plate collectors, evacuated tube collectors, and unglazed collectors.
II. Medium-temperature collectors: These collectors are used for applications that require temperatures between 80°C and 200°C, such as industrial process heating, absorption cooling, and solar drying. such as parabolic trough collectors, Fresnel collectors, and concentrating dish collectors.
III. High-temperature collectors: These collectors are used for applications that require temperatures above 200°C, such as power generation, chemical processing, and solar cooking, such as solar towers, solar furnaces, and concentrating dish collectors with Sterling engines.
There are several types of solar thermal collectors, but flat-plate collectors are the most common (Al-Hrari et al., 2020). They typically consist of a shallow, insulated box with a glass or plastic cover. Inside the box is a dark absorber plate, which is usually painted black to absorb maximum radiation. Heat-transfer fluid (water, antifreeze, or air) is circulated through piping attached to the absorber plate to absorb the heat energy. Evacuated tube collectors are a type of solar thermal collector that uses a series of tubes to absorb the Sun’s energy. The tubes are made of glass and are filled with a heat-transfer fluid. The tubes are mounted in an insulated box with an evacuated chamber between the glass layers, which reduces heat loss. Through the use of curved mirrors, parabolic trough collectors concentrate the Sun’s energy onto a receiver tube. Through the receiver tube, heat is transferred to a fluid or air that circulates through a long, curved trough.
Curved mirrors are used in solar concentrating systems, such as parabolic dish systems, to focus the Sun’s energy. Heat energy is transferred to air, oil, or molten salt through the receiver located in the centre of the dish. In commercial applications, parabolic trough collectors (PTCs) are the most commonly used type of concentrated solar thermal technology. The parabola’s focal line runs along a curved mirror that concentrates the Sun’s energy. A heat exchanger liquid (like Bromine) absorbs concentrated solar energy and transfers it to a receiver tube filled with a heat transfer fluid. Through the heat exchanger, solar energy is transferred to a heat storage system or directly to the application requiring thermal energy. Fluidized beds were surveyed for their applications to collection, storage, and exploitation using concentrated solar collectors (Tregambi et al., 2021). Using fluidized bed solar receivers/reactors, the authors developed novel and “creative” designs. Many applications of fluidized beds are powered by solar energy. An example would be the conversion of energy from one form to another, the storage of thermal energy, or the use of solid rings to store energy, whether chemical or thermal. Aside from producing fuels and chemicals, it also produces materials. To meet morning and evening demand, Brazilian researchers studied the performance of parabolic solar concentrators coupled with thermoelectric and voltaic hybrid systems (Martines et al., 2023). They investigated different configurations of heliothermic plants using an organic Rankine cycle. According to the diode model analysis for examining different commercial photovoltaic cells, R245fa with Urea-NaCl is the best thermal energy storage fluid for the Rankine cycle. An evaporation temperature of 80°C and 2,600 rpm were forecasted to produce 1580 W of electrical power for the hydride cycle. In this way, it is possible to generate power for 1 h and 20 min without solar radiation.
The efficiencies of solar thermal collectors vary according to the design, installation, weather conditions, and operating parameters. Optimizing the design of a solar thermal collector is essential to maximizing solar energy collection. Proper installation and orientation of the collector are also important factors in maximizing efficiency. Depending on the weather conditions, solar energy can be collected differently depending on the amount of sunlight and the air temperature. A solar thermal collector’s efficiency can also be affected by its operating parameters, such as temperature, flow rate, and pressure (Al-Waeli et al., 2016).
The formula below is used to calculate the thermal efficiency (ηth) of conventional flat plate solar collectors:
Ac is area of the collector, FR is the heat removal factor, S is absorbed solar radiation, UL is heat transfer coefficient, Ti is water inlet temperature and Ta is ambient Temperature.
The term PV/T is used to describe systems that combine photovoltaics with solar thermal energy. By converting solar energy into both electricity and thermal energy, PV/T systems can make better use of solar power. PV cells convert solar radiation into direct current (DC) electricity as part of a PV/T system. Solar energy that is not converted to electricity is captured and used to heat water or air through the thermal component of the system.
Figure 4 shows the two main types of PV/T systems: air-based and liquid-based. An air-based system absorbs heat from the PV modules by passing air over their backs. A liquid-based system circulates a heat transfer fluid through a flat-plate collector or tube collector behind the PV module to absorb heat, which can be used for space heating or producing hot water. The PV modules also benefit from the cooling effect of the circulating fluid, which can improve their electrical efficiency.
FIGURE 4. The classification of PV/T systems (Al-Waeli et al., 2016).
PV/T systems have several advantages over separate PV and solar thermal systems. PV and thermal components work together to capture more solar energy, resulting in higher overall energy output per unit surface area (Al-Waeli et al., 2019b). They also have a smaller environmental footprint and require less installation space than separate PV and solar thermal systems.
Residential and commercial buildings can be heated and cooled with PV/T systems, and hot water can be generated for residential and industrial processes. However, they may be more expensive to install than separate PV and solar thermal systems, and their performance can be affected by factors such as temperature and shading. Total efficiency combined (ηcombined) is the measure of a system’s overall performance (Al-Waeli et al., 2020a):
The following formula calculates the thermal efficiency (ηth) of conventional flat plate solar collectors:
Where Qu is heat gain, I(t) is solar irradiance, and Ac is collector area. As a function of temperature, the electrical efficiency of a PV module (ηel) can be expressed as follows (Brottier et al., 2016):
Where
I. Advanced PV cell technologies: The development of advanced PV cell technologies, such as multi-junction cells and tandem cells, can increase the efficiency of PV/T systems. This type of cell captures a wider spectrum of sunlight, making it more efficient in converting solar energy into electricity.
II. Using nanofluids to improve thermal conductivity: Nanofluids can improve both electrical and thermal efficiency by improving heat conductivity. Researchers have also shown that photovoltaic cells that use “phase change material” (PCM) along with nanofluid show increased efficiency. Additionally, binary nanofluids show even greater improvements. Researchers studied nanofluids over permeable convective surfaces in order to enhance their energy efficiency. In order to improve the efficiency of photovoltaic cells, nanofluids can be added to their surfaces. In addition to reducing the negative effects of solar thermal radiation and viscous dissipation, these fluids are also able to reduce the negative effects of convectional heat transfer (Adnan et al., 2022).
III. Integration with energy storage: Adding energy storage systems, such as batteries or thermal storage, to PV/T systems can improve their performance. PV/T systems can provide more consistent and reliable energy output by balancing intermittent solar energy production with energy storage (Modi et al., 2017).
IV. Improved thermal management: Improved thermal management techniques, such as microchannel heat exchangers and phase-change materials, can increase the thermal efficiency of PV/T systems. These techniques can help to more effectively transfer heat from the PV modules to the working fluid, improving the overall energy output of the system (Kazem et al., 2020).
V. Hybrid solar systems: It is possible to produce even more reliable and sustainable energy from hybrid solar systems. Wind and geothermal energy can be combined with PV/T systems. These systems can also help to balance the energy output of different renewable sources, providing a more consistent supply of energy (Pugsley et al., 2020).
In general, PV/T systems will contribute significantly to the transition to a more sustainable and renewable energy future. With improved efficiency and reliability, these systems can help reduce greenhouse gas emissions, increase energy security, and improve access to clean energy worldwide—as shown in Figure 5.
It is worth mentioning that PV/T efficiencies and PV module temperatures vary across different cases. To ensure accurate comparisons, it is crucial to have consistent system details and environmental conditions. For instance, the cases presented in Figure 5 were conducted under varying test conditions. Nonetheless, Figure 5 highlights the following key aspects:
• PV/T systems’ efficiency is mainly dependent on their temperature, the type of PV cells used, and the design of their modules. Thermal efficiency generally increases with higher temperatures, and the type of PV cell, the module design, and the integration of thermal and PV components can also affect efficiency (Al-Waeli et al., 2019c) and (Fudholi and Sopian, 2018).
• The efficacy of PV/T systems can vary based on factors such as the type of system, energy source, system size, location, and sunlight availability. Due to their ability to directly convert sunlight into electricity, PV systems are usually more efficient than thermal systems. Conversely, thermal systems use sunlight to heat up a working fluid, which is then used to generate electricity through a turbine. An efficient Solar System can be enhanced by installing tracking systems, optimizing module designs, and using materials that absorb and reflect more sunlight (Sopian et al., 2019) and (Al-Waeli et al., 2016).
• PV/T systems can experience temperature fluctuations due to a variety of factors. Depending on the ambient temperature, the PV material type, and the direct solar radiation intensity, the temperature of PV modules changes. Depending on the module’s temperature, the irradiance, the material used, and the system’s insulation, temperature variation may occur in thermal systems. Furthermore, both thermal and PV systems can experience temperature variation depending on their orientation (Tonui and Tripanagnostopoulos, 2007) and (Chaichan et al., 2021).
PV/T technology got more attention and interest from researchers. The literature shows many innovative directions as illustrated in Figure 6. The innovation related to the solar thermal part reflected on the PV electrical output as well. Recent innovations in PV/T technology have focused on improving the efficiency and cost-effectiveness of these systems. One notable advancement is the use of nanotechnology in PV/T systems as shown in Figure 6A, which increases their energy conversion efficiency by reducing energy losses due to thermal radiation (Kazem et al., 2022). Additionally, advancements in materials science have led to the development of more durable and weather-resistant PV/T panels, which have longer lifespans and require less maintenance. Passive cooling using fins as another option shows promising results (Refaey et al., 2022).
FIGURE 6. PV/T technology (A) enhancement innovation, (B) emerging fields, (C) nanofluids, (D) machine learning prediction.
It is also being noted that conducted PV/T technology research has more than one aspect as shown in Figure 6B. Much research was conducted to investigate the different nano-fluid using different nano-particles and sometimes binary nano-particles (Maatoug et al., 2023). Fluids containing nano-scale particles, usually in the range of 1–100 nm, are called nano-fluids. It has been proposed to use nano-fluids in PV/T applications to enhance the performance and efficiency of these systems (Al-Waeli et al., 2019d). PV/T systems generate electricity and heat using solar panels. During the heating process, a fluid, such as water, is heated. This fluid is then circulated through a heat exchanger to provide hot water or space heating. It is important to note that the heat transfer properties of the fluid used in this process influence the efficiency of the process. It is possible to improve heat transfer properties of the fluid by adding nano-particles as shown in Figure 6C. Compared to larger particles, nano-particles are more efficient at transferring heat because they have a high surface area to volume ratio (Jiang et al., 2022). Additionally, the small size of the nano-particles means that they can penetrate the boundary layer around the heat transfer surface, reducing thermal resistance and increasing heat transfer. Nano-fluids can increase heat transfer rates and overall efficiency of PV/T systems by increasing thermal conductivity and convective heat transfer coefficient. Nano-fluids have been reported to increase efficiency by up to 20% in some studies (Jiang et al., 2022). However, nanofluids also pose challenges, such as issues with nanoparticle stability and aggregation, which can lead to clogging and reduced performance. Additionally, the cost of producing and using nanofluids is higher than traditional fluids, which can make it less economically feasible.
PCM and nano-PCM are used to enhance heat observation and cooling (Cui et al., 2022). PCMs are materials that can absorb and release large amounts of heat during the phase transition from solid to liquid or liquid to gas, without a significant change in temperature (Al-Waeli et al., 2020b). This property allows them to store thermal energy and regulate temperature in many applications. In photovoltaic/thermal systems, PCMs can be integrated into the heat transfer system to store excess heat generated by the PV panel during the day and release it as needed, such as during the night or periods of low sunlight. As a result, there is no need to use external heat sources or air conditioning sources, thereby improving the system’s total efficiency (Amalu and Fabunmi, 2022). One way to integrate PCMs into a PV/T system is through a thermal storage tank, which contains the PCM material and is connected to the heat exchanger. During the day, when the solar panel generates excess thermal energy, it is transferred to the PCM in the storage tank, causing the PCM material to melt and store the energy. At night or during periods of low sunlight, the PCM solidifies and releases the stored energy to maintain the desired temperature (Sharaf et al., 2022). Research has shown that the use of PCMs in PV/T systems can improve efficiency and reduce energy consumption. Some studies have reported up to a 40% increase in efficiency with the use of PCMs. However, there are also challenges associated with the use of PCMs, such as the need for proper material selection and sizing to ensure optimal performance (Homlakorn et al., 2022). Additionally, the cost of PCMs can be higher than traditional heat transfer fluids, which can impact the economic feasibility of the technology.
In addition, using Machine Learning (ML) methods to model, predict and optimize PV/T shows interesting direction (Al-Waeli et al., 2018). PV/T technologies can be improved by using machine learning, according to research. An artificial intelligence technique known as Machine Learning allows computers to make predictions or decisions based on data without explicitly programming them. In PV/T systems, ML algorithms can be used to optimize the performance and control of the system (Cao et al., 2022). For example, ML can be used to predict the output of the PV/T based on factors such as weather conditions, time of day, and energy demand as shown in Figure 6D. This can allow for better control and management of the system, leading to improved efficiency and energy savings (Alghamdi et al., 2023). Additionally, ML can be used to identify patterns and trends in the data generated by the PV/T, such as energy consumption and generation, temperature, and weather conditions (Yousif and Kazem, 2021). This information can be used to optimize the system design and operation, such as by adjusting the orientation and tilt of the solar panel or adjusting the heat transfer fluid flow rate. Furthermore, ML can be used for fault detection and diagnosis in PV/T systems. By analyzing data from the system, ML algorithms can detect and diagnose faults, such as a malfunctioning solar panel or a clogged heat exchanger. This can allow for timely and effective maintenance and repair, leading to increased reliability and reduced downtime. It is worth mentioning that some of the conducted research uses more than one method/aspect as illustrated in Table 1. These are:
Energy plants, industrial processes, and transportation are some of the sources of CO2 emissions. Three technologies and processes are used to mitigate CO2 emission, these are Carbon Capture, Utilization, and Storage (CCUS) (McLaughlin et al., 2023). It involves capturing CO2 emissions from these sources, utilizing the captured CO2 for various purposes, and then storing it in a safe and permanent manner to prevent it from entering the atmosphere and contributing to climate change as shown in Figure 7A. The following is brief description on each of the three technologies;
I. Carbon Capture: Prior to entering the atmosphere, carbon dioxide emitted from point sources, such as transportation, power plants or industrial facilities, are captured by CCUS. A variety of methods exist for capturing CO2, including post combustion capture, pre-combustion capture, and combustion using oxy-fuels. After fossil fuels are burned, CO2 is removed from flue gases through post-combustion capture (Xinmin et al., 2023). Capturing CO2 before combustion, CO2 is emitted from the fuel. A combustion process using oxygen-rich environments results in a flue gas largely composed of carbon dioxide.
II. Carbon Utilization: CCUS’ second step involves utilizing the captured CO2 for a variety of purposes (Dziejarski et al., 2023). It is possible to use captured CO2 in a number of ways, including enhancing oil recovery (EOR). The process involves the capture of CO2 and its injection into oil reservoirs in order to increase oil production. CCU is the process of capturing and utilizing carbon to produce chemicals, materials, and fuels. Additionally, “direct air capture” (DAC) technologies that remove CO2 directly from the atmosphere may be possible with it as shown in Figure 7B.
III. Carbon Storage: Thirdly, CCUS stores the captured CO2 in a safe and permanent manner to prevent its release into the atmosphere. In addition to deep saline aquifers and depleted oil and gas reservoirs, CO2 can also be stored in unmineable coal seams. It is called carbon storage or carbon sequestration (Shiyi et al., 2022). Monitoring ensures that stored CO2 remains safe and does not leak back into the atmosphere.
FIGURE 7. (A) Carbon capture, utilization, and storage, (B) CO2 from source to storage, (C) negative emissions technologies (de Oliveira et al., 2022).
Climate change mitigation and greenhouse gas reduction can be achieved through CCUS. In addition to capturing and storing CO2, CCUS can significantly reduce CO2 emissions from a variety of sources, including heavy industry and existing infrastructure (Wei et al., 2022). However, CCUS also faces challenges, such as high costs, technological limitations, and concerns about the safety and long-term storage of captured CO2. Nevertheless, research and development efforts continue to advance CCUS technologies with the aim of making them more efficient, cost-effective, and environmentally sustainable (de Oliveira et al., 2022).
Negative emissions technologies (NETs) are strategies for removing carbon from the atmosphere, are approaches aimed at removing CO2 from the atmosphere to help mitigate climate change as shown in Figure 7C. These strategies can be broadly categorized into biological strategies, which involve using natural or biological processes to remove CO2 from the atmosphere, and technological/chemical strategies, which involve using engineered processes to capture and store CO2 (Fan et al., 2020). These are:
I. Biological Strategies: Biological strategies for carbon removal utilize natural or biological processes to sequester CO2 from the atmosphere. This can include:
• Afforestation and reforestation: Planting trees or restoring forests can help remove CO2 from the atmosphere through a process known as photosynthesis, where trees absorb CO2 and store it as carbon in their roots, stems, and leaves (Daneshvar et al., 2022).
• Soil carbon sequestration: Practices such as sustainable agriculture, improved land management, and soil conservation can enhance the storage of carbon in soils, thus removing CO2 from the atmosphere and promoting soil health (de Oliveira Maciel et al., 2022).
• Ocean fertilization: Certain nutrients can be added to the ocean to stimulate the increase in phytoplankton that absorbs CO2 through photosynthesis. However, such approach is controversial due to potential ecological and environmental impacts (Bajpai et al., 2022).
II. Technological/Chemical Strategies: Technological or chemical strategies for carbon removal involve using engineered processes to capture and store CO2 from the atmosphere (Balch et al., 2017). These can include:
• Direct air capture (DAC): DAC technologies use specialized equipment to capture CO2 directly from the ambient air, typically using chemical or physical processes. The captured CO2 can then be stored or utilized in various ways (Ma et al., 2022).
• Carbon capture and storage (CCS): Similar to the carbon capture step in CCUS, CCS involves capturing CO2 from industrial or power plant emissions and storing it in geological formations such as deep saline aquifers, depleted oil and gas reservoirs, or non-mineable coal seams (Becattini et al., 2022).
• Enhanced weathering: This approach involves accelerating the natural weathering process, where CO2 reacts with certain minerals to form stable carbonates, by grinding and spreading rocks such as basalt on land or in the ocean. This can help sequester CO2 over long timescales (Ding et al., 2023).
Both biological and technological/chemical strategies have the potential to contribute to carbon removal and help mitigate climate change (Rosa et al., 2022). However, they also have their own limitations, including scalability, costs, potential environmental impacts, and social considerations. It is important to carefully evaluate and implement these strategies with a comprehensive understanding of their benefits and risks to ensure their effectiveness and sustainability in addressing the challenge of climate change (Gabrielli et al., 2022).
Direct air capture (DAC) involves capturing CO2 directly from the atmosphere using specialized equipment and then storing the captured CO2 in a safe and permanent manner, typically through geological storage or utilization in other products or processes as shown in Figure 8A. The key aspects of CO2 mitigation using direct air capture (Castro-Muñoz et al., 2022):
• CO2 Capture: DAC technologies use various methods to capture CO2 from the atmosphere. These methods can include chemical, physical, or biological processes (Sodiq et al., 2023). For example, some DAC systems use adsorbents or solvents to selectively capture CO2 from the air, while others use chemical reactions or catalysts to convert CO2 into a solid form that can be easily captured. Once the CO2 is captured, it is typically separated from other gases and purified as shown in Figure 8B.
• CO2 Storage or Utilization: CO2 can be stored or utilized after it is captured from the atmosphere. In deeper saline aquifers, depleted oil and gas reservoirs, and unmineable coal seams, carbon dioxide can be stored through a process known as carbon storage or carbon sequestration (Zhang et al., 2022). Other applications of captured CO2 include chemical production, materials, and fuels, as well as the production of CCU. By utilizing captured CO2 in other processes, DAC costs can be offset and value can be created.
• Energy Requirements: DAC technologies require energy to operate, which is typically obtained from external sources, such as electricity. The energy requirements of DAC systems can vary depending on the specific technology and scale of operation (Jiang et al., 2023). Some DAC technologies may use renewable energy sources, such as solar or wind power, to minimize their carbon footprint, while others may rely on fossil fuels, which can affect the overall carbon footprint of the process.
• Scalability and Costs: DAC is still a relatively emerging technology, and its scalability and costs are important considerations. Scaling up DAC to a level that can have a significant impact on global CO2 emissions would require substantial investments in infrastructure and operations (Goldman et al., 2023). The costs of DAC can vary depending on factors such as the technology used, the size of the system, and the availability of local resources. Currently, DAC is generally considered to be more expensive compared to other CO2 mitigation strategies, but costs may decrease with further technological advancements and deployment at larger scales.
• Environmental Impacts: DAC technologies may have potential environmental impacts, including land use requirements, water use, and potential emissions from the energy sources used. It is important to carefully assess and manage these environmental impacts to ensure that the overall benefits of DAC in terms of CO2 mitigation are not outweighed by potential negative environmental consequences (Fasihi et al. , 2019).
FIGURE 8. Capturing CO2 directly from the atmosphere using specialized equipment and then storing the captured CO2 in a safe and permanent manner, typically through geological storage or utilization in other products or processes (A). Once the CO2 is captured, it is typically separated from other gases and purified (B) (Cho, 2018) (Castro-Muñoz et al., 2022).
Climate change can be mitigated by removing CO2 from the atmosphere using direct air capture (Yang and Dai, 2021). However, it is still a developing technology with various challenges, including scalability, costs, energy requirements, and environmental impacts. Continued research, development, and deployment efforts are needed to further improve DAC technologies and make them more effective, efficient, and sustainable in the broader context of climate change mitigation.
The PV/T system used to provide thermal part of DAC to capture CO2 from the atmosphere. After the capture medium has absorbed CO2, it needs to be regenerated, or desorbed, in order to release the captured CO2 for further processing or storage. This regeneration step usually involves applying PV/T heat to the capture medium to release the adsorbed CO2, which can then be collected, stored, or utilized in various ways as shown in Figure 9A. The thermal regeneration process in CO2 DAC depends on the type of capture medium or sorbent used. Some common methods for regenerating the capture medium include:
• Thermal Desorption: In this method, the capture medium is heated to high temperatures, typically ranging from 100 to 300°C, which causes the adsorbed CO2 to absorb or release from the surface of the capture medium. The released CO2 can then be collected and processed further. Thermal desorption is commonly used in chemical absorption-based DAC processes where liquid solvents, such as amines, are used as capture media.
• Calcination: This method involves heating the capture medium to even higher temperatures, typically above 500°C, which causes the sorbent material to undergo a chemical reaction, resulting in the release of the adsorbed CO2 as a gas (Hornberger et al., 2021). The regenerated sorbent can then be reused for further CO2 capture. Calcination is commonly used in solid sorbent-based DAC processes where materials like “metal-organic frameworks” (MOFs) or zeolites are used as capture media.
• Cryogenic Desorption: This method involves cooling the capture medium to very low temperatures, typically below −70°C, which causes the adsorbed CO2 to desorb as a gas. The desorbed CO2 can then be collected and processed further (Jiang et al., 2023). Cryogenic desorption is commonly used in DAC processes that utilize cryogenic separation methods.
FIGURE 9. (A) PV/T integrated in DAC, (B) PV/T efficiency, (C) nanofluid based PV/T, (D) cost over 25 years.
The thermal regeneration step in CO2 DAC is energy-intensive and requires careful optimization to balance the energy input for regeneration with the amount of CO2 captured. The captured CO2 is typically collected and processed further for storage or utilization, such as for enhanced oil recovery, production of synthetic fuels, or other industrial applications. It's worth noting that the specific details of the thermal regeneration process may vary depending on the type of capture medium, process design, and other factors, and ongoing research and development efforts are focused on improving the efficiency and cost-effectiveness of the thermal regeneration step in CO2 DAC.
Figure 9B shows PV/T system, which utilizes solar thermal energy and provides heat to the DAC. It is worth mentioning that the overall PV/T efficiency is higher than separate PV and thermal collector. A nanofluid increases thermal conductivity as well as heat capture and electricity generation. Nanofluid improves the yield and power production of PV/T as shown in Figure 9C. In Figure 9D, PV/T is compared to feed-in-tariffs and its cost effectiveness is shown.
Integrating PV/T systems into DAC processes can have several benefits. It can reduce the carbon footprint of the DAC process by using renewable energy, which can help mitigate climate change. It can also potentially lower operational costs by reducing the dependence on fossil fuels and electricity, depending on the availability and cost of solar energy in a given location. However, it's worth noting that the feasibility and efficiency of using PV/T systems for DAC depend on factors such as the availability and intensity of solar radiation, the specific DAC technology being used, used nanofluid, and the overall system design. DAC processes are being optimized for optimal PV/T integration and scalability and cost-effectiveness with respect to PV/T systems.
There is a significant potential of photovoltaic/thermal systems for carbon dioxide mitigation applications. PV/T systems hold great promise for CO2 mitigation applications, and further research and development efforts are needed to unlock their full potential. By addressing the challenges and exploring the future directions outlined in this review, PV/T systems can contribute significantly to mitigating CO2 emissions and promoting sustainable energy systems. Our review has demonstrated the versatility of PV/T systems in simultaneously generating electricity and thermal energy, making them well-suited for a wide range of applications in various sectors. The research on PV/T systems for CO2 mitigation is still evolving, and several future directions can be identified. First, there is a need for further research on the performance optimization of PV/T systems, including improving their efficiency, reliability, and durability. Advanced materials, designs, and manufacturing techniques can be explored to enhance the performance of PV/T systems and reduce their costs.
Other renewable energy sources can be integrated with PV/T systems, energy storage technologies, and building systems can be further investigated, can potentially provide more reliable and sustainable energy solutions. Moreover, the development of effective energy storage technologies can enable better utilization of PV/T systems and facilitate their integration into the grid.
The environmental and economic aspects of PV/T systems need to be carefully evaluated to ensure their sustainability. Ecological and economic assessments can reveal insights into the performance of PV/T systems and guide decision-making. In order to validate their performance and assess their feasibility, PV/T systems need to be tested in different climates and applications. Long-term monitoring and performance evaluation of PV/T systems can provide valuable data for improving their design, operation, and maintenance. Ashraf et al., 2022.
All authors listed have made a substantial, direct, and intellectual contribution to the work and approved it for publication.
The Sultanate of Oman’s Research Council funded this study with a Research Grant Agreement No. ORG SU EI 11010. A partial financial support is provided by Arabian Gulf University for publication in an Open Access Journal.
The authors declare that the research was conducted in the absence of any commercial or financial relationships that could be construed as a potential conflict of interest.
All claims expressed in this article are solely those of the authors and do not necessarily represent those of their affiliated organizations, or those of the publisher, the editors and the reviewers. Any product that may be evaluated in this article, or claim that may be made by its manufacturer, is not guaranteed or endorsed by the publisher.
Al-Hrari, M., Ceylan, İ., Nakoa, K., and Ergün, A. (2020). Concentrated photovoltaic and thermal system application for fresh water production. Appl. Therm. Eng. 171, 115054. doi:10.1016/j.applthermaleng.2020.115054
Al-Waeli, A. H., Chaichan, M. T., Sopian, K., and Kazem, H. A. (2019a). Influence of the base fluid on the thermo-physical properties of PV/T nanofluids with surfactant. Case Stud. Therm. Eng. 13, 100340. doi:10.1016/j.csite.2018.10.001
Al-Waeli, A. H., Chaichan, M. T., Sopian, K., Kazem, H. A., Mahood, H. B., and Khadom, A. A. (2019c). Modeling and experimental validation of a PVT system using nanofluid coolant and nano-PCM. Sol. Energy 177, 178–191. doi:10.1016/j.solener.2018.11.016
Al-Waeli, A. H., Kazem, H. A., Chaichan, M. T., and Sopian, K. (2019b). Experimental investigation of using nano-PCM/nanofluid on a photovoltaic thermal system (PVT): Technical and economic study. Therm. Sci. Eng. Prog. 11, 213–230. doi:10.1016/j.tsep.2019.04.002
Al-Waeli, A. H., Kazem, H. A., Sopian, K., and Chaichan, M. T. (2018). Techno-economical assessment of grid connected PV/T using nanoparticles and water as base-fluid systems in Malaysia. Int. J. Sustain. Energy 37 (6), 558–575. doi:10.1080/14786451.2017.1323900
Al-Waeli, A. H., Kazem, H. A., Yousif, J. H., Chaichan, M. T., and Sopian, K. (2020b). Mathematical and neural network modeling for predicting and analyzing of nanofluid-nano PCM photovoltaic thermal systems performance. Renew. Energy 145, 963–980. doi:10.1016/j.renene.2019.06.099
Al-Waeli, A. H., Sopian, K., Kazem, H. A., and Chaichan, M. T. (2020a). Evaluation of the electrical performance of a photovoltaic thermal system using nano-enhanced paraffin and nanofluids. Case Stud. Therm. Eng. 21, 100678. doi:10.1016/j.csite.2020.100678
Al-Waeli, A. H., Sopian, K., Kazem, H. A., and Chaichan, M. T. (2016). Photovoltaic solar thermal (PV/T) collectors past, present and future: A review. Int. J. Appl. Eng. Res. 11 (22), 10757–10765. doi:10.5281/zenodo.8040609
Al-Waeli, A. H., Sopian, K., Kazem, H. A., and Chaichan, M. T. (2017). Photovoltaic/Thermal (PV/T) systems: Status and future prospects. Renew. Sustain. Energy Rev. 77, 109–130. doi:10.1016/j.rser.2017.03.126
Al-Waeli, A. H., Sopian, K., Yousif, J. H., Kazem, H. A., Boland, J., and Chaichan, M. T. (2019d). Artificial neural network modeling and analysis of photovoltaic/thermal system based on the experimental study. Energy Convers. Manag. 186, 368–379. doi:10.1016/j.enconman.2019.02.066
Alghamdi, H., Maduabuchi, C., Albaker, A., Alatawi, I., Alsenani, T. R., Alsafran, A. S., et al. (2023). A prediction model for the performance of solar photovoltaic-thermoelectric systems utilizing various semiconductors via optimal surrogate machine learning methods. Eng. Sci. Technol. Int. J. 40, 101363. doi:10.1016/j.jestch.2023.101363
Amalu, E. H., and Fabunmi, O. A. (2022). Thermal control of crystalline silicon photovoltaic (c-Si PV) module using Docosane phase change material (PCM) for improved performance. Sol. Energy 234, 203–221. doi:10.1016/j.solener.2022.02.001
Ashraf, W., Junaid Anjum, H., Khan, I., Mousa, M., and Mehrez, S. (2022). Energy transformation and entropy investigation in the nanofluid composed by γ-nanomaterial over a permeable convective surface with solar thermal radiation: A numerical analysis. Front. Energy Res. 10, 507.doi:10.3389/fenrg.2022.888389
Athienitis, A. K., Barone, G., Buonomano, A., and Palombo, A. (2018). Assessing active and passive effects of façade building integrated photovoltaics/thermal systems: Dynamic modelling and simulation. Appl. Energy 209, 355–382. doi:10.1016/j.apenergy.2017.09.039
Bajpai, S., Shreyash, N., Singh, S., Memon, A. R., Sonker, M., Tiwary, S. K., et al. (2022). Opportunities, challenges and the way ahead for carbon capture, utilization and sequestration (CCUS) by the hydrocarbon industry: Towards a sustainable future. Energy Rep. 8, 15595–15616. doi:10.1016/j.egyr.2022.11.023
Balch, R., McPherson, B., and Grigg, R. (2017). Overview of a large scale carbon capture, utilization, and storage demonstration project in an active oil field in Texas, USA. Energy Procedia 114, 5874–5887. doi:10.1016/j.egypro.2017.03.1725
Becattini, V., Gabrielli, P., Antonini, C., Campos, J., Acquilino, A., Sansavini, G., et al. (2022). Carbon dioxide capture, transport and storage supply chains: Optimal economic and environmental performance of infrastructure rollout. Int. J. Greenh. Gas Control 117, 103635. doi:10.1016/j.ijggc.2022.103635
Brottier, L., Naudin, S., Veeser, V., Terrom, G., and Bennacer, R. (2016). Field test results of an innovative PV/T collector for solar domestic hot water. Energy Procedia 91, 276–283. doi:10.1016/j.egypro.2016.06.219
Cao, Y., Kamrani, E., Mirzaei, S., Khandakar, A., and Vaferi, B. (2022). Electrical efficiency of the photovoltaic/thermal collectors cooled by nanofluids: Machine learning simulation and optimization by evolutionary algorithm. Energy Rep. 8, 24–36. doi:10.1016/j.egyr.2021.11.252
Castro-Munoz, R., Ahmad, M. Z., Malankowska, M., and Coronas, J. (2022). A new relevant membrane application: CO2 direct air capture (DAC). Chem. Eng. J. 446, 137047. doi:10.1016/j.cej.2022.137047
Chaichan, M. T., Kazem, H. A., Al-Waeli, A. H., and Sopian, K. (2021). Controlling the melting and solidification points temperature of PCMs on the performance and economic return of the water-cooled photovoltaic thermal system. Sol. Energy 224, 1344–1357. doi:10.1016/j.solener.2021.07.003
Cho, R. (2018). Can removing carbon from the atmosphere save us from climate catastrophe. Columbia, NY, USA: Earth Institute at Columbia University.
Cui, Y., Zhu, J., Zhang, F., Shao, Y., and Xue, Y. (2022). Current status and future development of hybrid PV/T system with PCM module: 4E (energy, exergy, economic and environmental) assessments. Renew. Sustain. Energy Rev. 158, 112147. doi:10.1016/j.rser.2022.112147
Daneshvar, E., Wicker, R. J., Show, P. L., and Bhatnagar, A. (2022). Biologically-mediated carbon capture and utilization by microalgae towards sustainable CO2 biofixation and biomass valorization–A review. Chem. Eng. J. 427, 130884. doi:10.1016/j.cej.2021.130884
de Oliveira Maciel, A., Christakopoulos, P., Rova, U., and Antonopoulou, I. (2022). Carbonic anhydrase to boost CO2 sequestration: Improving carbon capture utilization and storage (CCUS). Chemosphere 299, 134419. doi:10.1016/j.chemosphere.2022.134419
Ding, H., Zhang, Y., Dong, Y., Wen, C., and Yang, Y. (2023). High-pressure supersonic carbon dioxide (CO2) separation benefiting carbon capture, utilisation and storage (CCUS) technology. Appl. Energy 339, 120975. doi:10.1016/j.apenergy.2023.120975
Dziejarski, B., Krzyżyńska, R., and Andersson, K. (2023). Current status of carbon capture, utilization, and storage technologies in the global economy: A survey of technical assessment. Fuel 342, 127776. doi:10.1016/j.fuel.2023.127776
Fan, J. L., Shen, S., Xu, M., Yang, Y., Yang, L., and Zhang, X. (2020). Cost-benefit comparison of carbon capture, utilization, and storage retrofitted to different thermal power plants in China based on real options approach. Adv. Clim. Change Res. 11 (4), 415–428. doi:10.1016/j.accre.2020.11.006
Fasihi, M., Efimova, O., and Breyer, C. (2019). Techno-economic assessment of CO2 direct air capture plants. J. Clean. Prod. 224, 957–980. doi:10.1016/j.jclepro.2019.03.086
Fudholi, A., and Sopian, K. (2018). Review on solar collector for agricultural produce. Int. J. Power Electron. Drive Syst. 9 (1), 414. doi:10.11591/ijpeds.v9.i1.pp414-419
Gabrielli, P., Campos, J., Becattini, V., Mazzotti, M., and Sansavini, G. (2022). Optimization and assessment of carbon capture, transport and storage supply chains for industrial sectors: The cost of resilience. Int. J. Greenh. Gas Control 121, 103797. doi:10.1016/j.ijggc.2022.103797
Goldman, M., Kota, S., Gao, X., Katzman, L., and Farrauto, R. (2023). Parametric and laboratory aging studies of direct CO2 air capture simulating ambient capture conditions and desorption of CO2 on supported alkaline adsorbents. Carbon Capture Sci. Technol. 6, 100094. doi:10.1016/j.ccst.2022.100094
Homlakorn, S., Suksri, A., and Wongwuttanasatian, T. (2022). Efficiency improvement of PV module using a binary-organic eutectic phase change material in a finned container. Energy Rep. 8, 121–128. doi:10.1016/j.egyr.2022.05.147
Hornberger, M., Moreno, J., Schmid, M., and Scheffknecht, G. (2021). Experimental investigation of the calcination reactor in a tail-end calcium looping configuration for CO2 capture from cement plants. Fuel 284, 118927. doi:10.1016/j.fuel.2020.118927
Jiang, L., Liu, W., Wang, R. Q., Gonzalez-Diaz, A., Rojas-Michaga, M. F., Michailos, S., et al. (2023). Sorption direct air capture with CO2 utilization. Prog. Energy Combust. Sci. 95, 101069. doi:10.1016/j.pecs.2022.101069
Jiang, T., Zou, T., Wang, G., Wang, B., and Chen, Z. (2022). Preparation and application study in a novel solar PV/thermal system of indium tin oxide nanofluid optical filter. Energy Rep. 8, 5668–5677. doi:10.1016/j.egyr.2022.04.026
Kazem, H. A., Al-Waeli, A. H., Chaichan, M. T., Al-Waeli, K. H., Al-Aasam, A. B., and Sopian, K. (2020). Evaluation and comparison of different flow configurations pvt systems in Oman: A numerical and experimental investigation. Sol. Energy 208, 58–88. doi:10.1016/j.solener.2020.07.078
Kazem, H. A., Chaichan, M. T., and Sopian, K. (2022). Design and experimental evaluation of a PV/T system cooled by advanced methods. Int. J. Energy Res. 46 (7), 9684–9709. doi:10.1002/er.7838
Kazem, H. A., Khatib, T., Sopian, K., and Elmenreich, W. (2014). Performance and feasibility assessment of a 1.4 kW roof top grid-connected photovoltaic power system under desertic weather conditions. Energy Build. 82, 123–129. doi:10.1016/j.enbuild.2014.06.048
Kazem, H. A., Khatib, T., and Sopian, K. (2013). Sizing of a standalone photovoltaic/battery system at minimum cost for remote housing electrification in Sohar, Oman. Energy Build. 61, 108–115. doi:10.1016/j.enbuild.2013.02.011
Ma, J., Li, L., Wang, H., Du, Y., Ma, J., Zhang, X., et al. (2022). Carbon capture and storage: History and the road ahead. Engineering 14, 33–43. doi:10.1016/j.eng.2021.11.024
Maatoug, S., Moulahi, A., Bazuhair, N., Alqarni, S., Selimefendigil, F., Aich, W., et al. (2023). Pulsating multiple nano-jet impingement cooling system design by using different nanofluids for photovoltaic (PV) thermal management. Case Stud. Therm. Eng. 41, 102650. doi:10.1016/j.csite.2022.102650
McLaughlin, H., Littlefield, A. A., Menefee, M., Kinzer, A., Hull, T., Sovacool, B. K., et al. (2023). Carbon capture utilization and storage in review: Sociotechnical implications for a carbon reliant world. Renew. Sustain. Energy Rev. 177, 113215. doi:10.1016/j.rser.2023.113215
Modi, A., Bühler, F., Andreasen, J. G., and Haglind, F. (2017). A review of solar energy based heat and power generation systems. Renew. Sustain. Energy Rev. 67, 1047–1064. doi:10.1016/j.rser.2016.09.075
Mohsenzadeh, M., and Hosseini, R. (2015). A photovoltaic/thermal system with a combination of a booster diffuse reflector and vacuum tube for generation of electricity and hot water production. Renew. Energy 78, 245–252. doi:10.1016/j.renene.2015.01.010
Moreno, A., Chemisana, D., and Fernández, E. F. (2021). Hybrid high-concentration photovoltaic-thermal solar systems for building applications. Appl. Energy 304, 117647. doi:10.1016/j.apenergy.2021.117647
Obalanlege, M. A., Xu, J., Markides, C. N., and Mahmoudi, Y. (2022). Techno-economic analysis of a hybrid photovoltaic-thermal solar-assisted heat pump system for domestic hot water and power generation. Renew. Energy 196, 720–736. doi:10.1016/j.renene.2022.07.044
Peng, J., Lu, L., and Yang, H. (2013). An experimental study of the thermal performance of a novel photovoltaic double-skin facade in Hong Kong. Sol. Energy 97, 293–304. doi:10.1016/j.solener.2013.08.031
Pugsley, A., Zacharopoulos, A., Mondol, J. D., and Smyth, M. (2020). BIPV/T facades–A new opportunity for integrated collector-storage solar water heaters? Part 1: State-of-the-art, theory and potential. Sol. Energy 207, 317–335. doi:10.1016/j.solener.2020.06.025
Refaey, H. A., Abdelrahman, M. A., Alharthi, M. A., Bendoukha, S., Khan, S. G., and Emam, M. (2022). Passive cooling of highly-concentrator triple-junction solar cell using a straight-finned heat sink: An experimental investigation. Case Stud. Therm. Eng. 40, 102521. doi:10.1016/j.csite.2022.102521
Renno, C., D'Agostino, D., Minichiello, F., Petito, F., and Balen, I. (2019). Performance analysis of a CPV/T-DC integrated system adopted for the energy requirements of a supermarket. Appl. Therm. Eng. 149, 231–248. doi:10.1016/j.applthermaleng.2018.12.007
Rosa, L., Becattini, V., Gabrielli, P., Andreotti, A., and Mazzotti, M. (2022). Carbon dioxide mineralization in recycled concrete aggregates can contribute immediately to carbon-neutrality. Resour. Conservation Recycl. 184, 106436. doi:10.1016/j.resconrec.2022.106436
Rounis, E. D., Ioannidis, Z., Sigounis, A. M., Athienitis, A., and Stathopoulos, T. (2022). A novel approach for the modelling of convective phenomena for building integrated photovoltaic thermal (BIPV/T) systems. Sol. Energy 232, 328–343. doi:10.1016/j.solener.2021.12.058
Saini, P., Ghasemi, M., Arpagaus, C., Bless, F., Bertsch, S., and Zhang, X. (2023). Techno-economic comparative analysis of solar thermal collectors and high-temperature heat pumps for industrial steam generation. Energy Convers. Manag. 277, 116623. doi:10.1016/j.enconman.2022.116623
Sharaf, M., Huzayyin, A. S., and Yousef, M. S. (2022). Performance enhancement of photovoltaic cells using phase change material (PCM) in winter. Alexandria Eng. J. 61 (6), 4229–4239. doi:10.1016/j.aej.2021.09.044
Shiyi, Y., Desheng, M., Junshi, L., Tiyao, Z. H. O. U., Zemin, J. I., and Haishui, H. A. N. (2022). Progress and prospects of carbon dioxide capture, EOR-utilization and storage industrialization. Petroleum Explor. Dev. 49 (4), 955–962. doi:10.1016/s1876-3804(22)60324-0
Shu, D. Y., Deutz, S., Winter, B. A., Baumgärtner, N., Leenders, L., and Bardow, A. (2023). The role of carbon capture and storage to achieve net-zero energy systems: Trade-offs between economics and the environment. Renew. Sustain. Energy Rev. 178, 113246. doi:10.1016/j.rser.2023.113246
Sodiq, A., Abdullatif, Y., Aissa, B., Ostovar, A., Nassar, N., El-Naas, M., et al. (2023). A review on progress made in direct air capture of CO2. Environ. Technol. Innovation 29, 102991. doi:10.1016/j.eti.2022.102991
Sopian, K., Al-Waeli, A. H., and Kazem, H. A. (2020). Energy, exergy and efficiency of four photovoltaic thermal collectors with different energy storage material. J. Energy Storage 29, 101245. doi:10.1016/j.est.2020.101245
Sopian, K., Alwaeli, A. H. A., and Kazem, H. A. (2019). Advanced photovoltaic thermal collectors. Proc. Institution Mech. Eng. Part E J. Process Mech. Eng. 34 (2), 206–213. doi:10.1177/0954408919869541
Sornek, K., Żołądek, M., Papis-Frączek, K., Szram, M., and Filipowicz, M. (2023). Experimental investigations of the microscale concentrated photovoltaic/thermal system based on a solar parabolic trough concentrator. Energy Rep. 9, 86–97. doi:10.1016/j.egyr.2023.03.089
Tonui, J. K., and Tripanagnostopoulos, Y. (2007). Improved PV/T solar collectors with heat extraction by forced or natural air circulation. Renew. energy 32 (4), 623–637. doi:10.1016/j.renene.2006.03.006
Wang, K., Pantaleo, A. M., Herrando, M., Faccia, M., Pesmazoglou, I., Franchetti, B. M., et al. (2020). Spectral-splitting hybrid PV-thermal (PVT) systems for combined heat and power provision to dairy farms. Renew. Energy 159, 1047–1065. doi:10.1016/j.renene.2020.05.120
Wei, N., Liu, S., Jiao, Z., and Li, X. C. (2022). A possible contribution of carbon capture, geological utilization, and storage in the Chinese crude steel industry for carbon neutrality. J. Clean. Prod. 374, 133793. doi:10.1016/j.jclepro.2022.133793
Xinmin, S. O. N. G., Feng, W. A. N. G., Desheng, M. A., Ming, G. A. O., and Zhang, Y. (2023). Progress and prospect of carbon dioxide capture, utilization and storage in CNPC oilfields. Petroleum Explor. Dev. 50 (1), 229–244. doi:10.1016/s1876-3804(22)60383-5
Yang, S., Fiorito, F., Sproul, A., and Prasad, D. (2023). Optimising design parameters of a building-integrated photovoltaic double-skin facade in different climate zones in Australia. Buildings 13 (4), 1096. doi:10.3390/buildings13041096
Yang, Z., and Dai, S. (2021). Challenges in engineering the structure of ionic liquids towards direct air capture of CO2. Green Chem. Eng. 2 (4), 342–345. doi:10.1016/j.gce.2021.08.003
Yousif, J. H., and Kazem, H. A. (2021). Prediction and evaluation of photovoltaic-thermal energy systems production using artificial neural network and experimental dataset. Case Stud. Therm. Eng. 27, 101297. doi:10.1016/j.csite.2021.101297
Keywords: photovoltaic/thermal, CO2 mitigation, CO2 capture, environmental effects, PV/T technology innovation
Citation: Kazem HA, Al-Waeli AHA, Chaichan MT and Alnaser WE (2023) Photovoltaic/thermal systems for carbon dioxide mitigation applications: a review. Front. Built Environ. 9:1211131. doi: 10.3389/fbuil.2023.1211131
Received: 24 April 2023; Accepted: 12 June 2023;
Published: 20 June 2023.
Edited by:
Bao-Jie He, Chongqing University, ChinaReviewed by:
Siliang Yang, Leeds Beckett University, United KingdomCopyright © 2023 Kazem, Al-Waeli, Chaichan and Alnaser. This is an open-access article distributed under the terms of the Creative Commons Attribution License (CC BY). The use, distribution or reproduction in other forums is permitted, provided the original author(s) and the copyright owner(s) are credited and that the original publication in this journal is cited, in accordance with accepted academic practice. No use, distribution or reproduction is permitted which does not comply with these terms.
*Correspondence: Waheeb E. Alnaser, d2FsbmFzZXJAYWd1LmVkdS5iaA==
Disclaimer: All claims expressed in this article are solely those of the authors and do not necessarily represent those of their affiliated organizations, or those of the publisher, the editors and the reviewers. Any product that may be evaluated in this article or claim that may be made by its manufacturer is not guaranteed or endorsed by the publisher.
Research integrity at Frontiers
Learn more about the work of our research integrity team to safeguard the quality of each article we publish.