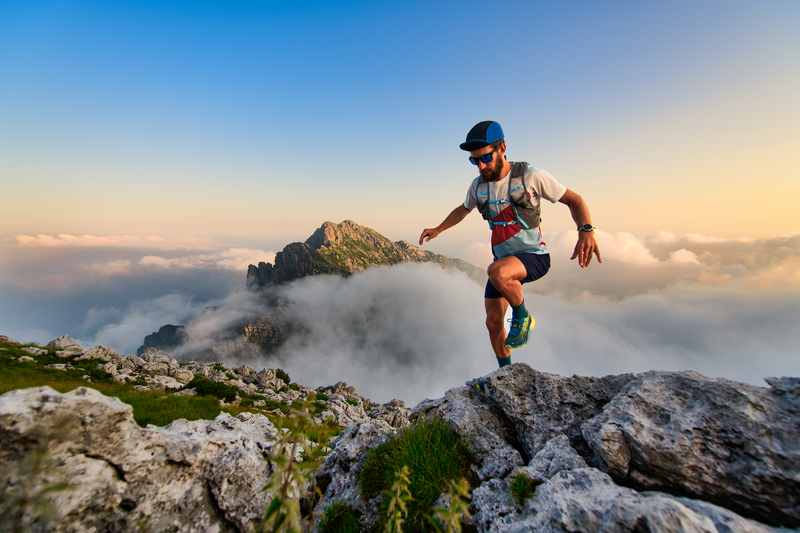
95% of researchers rate our articles as excellent or good
Learn more about the work of our research integrity team to safeguard the quality of each article we publish.
Find out more
ORIGINAL RESEARCH article
Front. Built Environ. , 15 September 2022
Sec. Computational Methods in Structural Engineering
Volume 8 - 2022 | https://doi.org/10.3389/fbuil.2022.945944
This article is part of the Research Topic Machine Learning Applications in Civil Engineering View all 10 articles
Blockchain is an emerging technology that has demonstrated great uptake potential in Peer-to-Peer (P2P) energy trading. The revolution of blockchain brings substantial benefits and innovation to sustainability energy transitions through P2P trading. Blockchain enables energy commodities to be traded. However, perceptions of this technology’s impact on the environment and its associated costs have garnered recent adverse publicity. This paper aims to look at the linkages between blockchain technology and energy systems in terms of blockchain power consumption against blockchain advantage over renewable energy transitions via peer-to-peer energy trading. The amount of energy used and carbon released during the blockchain validation process is estimated, and the cost of blockchain is computed to assess its economic benefit in a peer-to-peer energy trading scenario. Real data from running peer-to-peer energy trading systems are used, and numerous insights on the transformation of peer-to-peer energy trading utilising various blockchain scaling methods are provided. Based on the analysis, this paper concludes that the cost of processing trading transactions is lower using blockchain than current coordination costs. Also, blockchain-based energy can be traded more frequently than current regulations allow in order to reap the full benefits of renewable energy. A secure blockchain-enabled P2P trading environment would lead to fair rates for energy providers and prosumers resulting in stimulating the renewable energy market.
As of March 2022, Australia had over 3.12 million solar photovoltaic (PV) installations with a total capacity of over 26 gigawatts (AustralianPVInstitute 2022). Since 2010, this sector has grown at a breakneck pace, and it has continued to do so. Because of Australia’s large number of PV installations, distributed energy marketplaces have sprung up to take advantage of the available energy. Peer-to-peer (P2P) energy trading, retail and wholesale energy, business-to-business (B2B) energy trading, power trading, and other marketplaces are being tested around the world. P2P trading, which allows people to trade electricity, is the most frequent market (Dudjak et al., 2021; Abu-Salih et al., 2022).
P2P energy trading has evolved as a new paradigm for energy management, allowing prosumers to sell excess energy and consumers to purchase inexpensive, locally generated renewable power (Wongthongtham, Morrison, and Liu 2019). The rising quantity of decentralized electricity production from solar installations opens the door to new electricity markets and allows consumers to share electricity (Christidis, et al., 2021a). P2P electricity markets give users the freedom to choose their energy source, such as investing in locally generated renewable energy.
Innovative technologies are being investigated in order to provide a safe and auditable P2P electricity market. Blockchain is a form of distributed ledger technology characterised as an encrypted, distributed, decentralised, transparent, trustless, and immutable database (Wongthongtham et al., 2021; Zheng et al., 2017; Kuo, Kim, and Ohno-Machado 2017a). The technology has demonstrated significant potential in P2P energy trading, with a rising number of commencement firms, projects, experiments, and research projects including blockchain as part of the operational plan. (Wu et al., 2022). Energy supply companies, technology developers, financial organisations, national governments, and academic institutions are among the more than 140 blockchain research projects and start-ups that are using blockchain for energy applications (Andoni et al., 2019; Shamsi and Paul 2020). The blockchain revolution promotes innovation and allows for a low-carbon transition and long-term sustainability (Juri et al., 2016). Furthermore, the use of blockchain technology in peer-to-peer electricity trading allows for a transition away from a highly centralised market dominated by a few businesses and towards a truly decentralised market dominated by microgrids according to Deloitte (Grewal-Carr and Marshall, 2016) and PWC (Hasse et al., 2016) research, by allowing energy commodities to be traded as digital assets.
Prosumers can sell their excess electricity directly to local customers via blockchain-enabled P2P energy trading, enabling mutually beneficial transactions. Customers spend less per kWh for their electricity and can use renewable energy sources without having to invest in the equipment. Consumers profit from this plan because they spend less on their electricity per kWh and benefit from renewable energy sources without owning the technology. Prosumers benefit from this plan because they make more than they otherwise would with feed-in tariffs. Because a battery can store untraded power, auctions for renewable electricity can create a dynamic market that benefits both prosumers and consumers. A more efficient market with reduced infrastructure costs also benefits electricity retailers and network providers. By eliminating market intermediaries, blockchain-based systems also enable anonymity and security to both prosumers and consumers. Agents with comparable energy demand profiles match supply and demand in real time, and trade is made through smart contracts (Damisa, Nwulu, and Siano 2022).
Blockchain technology and peer-to-peer trading have been extensively studied in the context of climate change policy, and have been implemented in a number of climate-related fields, including climate investment and carbon pricing (Abu-Salih et al., 2021; Alao and Paul 2021; Hua et al., 2022). As the cost of PV modules declines, the number of homes installing PV systems rises; P2P energy trading has become one of the most popular blockchain-based applications (Wang et al., 2021). However, questions about blockchain’s energy use, carbon footprint, and cost have lately sparked debate. Blockchain’s carbon footprint and cost come from its validation process, which necessitates specialised hardware with high computing power and large quantities of electricity. Public impressions of the technology’s impact on the environment and its associated expenses have recently received critical attention. This distorts people’s perceptions of blockchain’s benefits. It is motivating to compare the amount of energy used to maintain blockchain with the amount of energy saved from the electrical grid as a result of P2P energy trading.
In this study, we look at how blockchain technology affects the environment in terms of energy usage, carbon emissions, and economic value. Additionally, numerous blockchain scalability approaches are examined in order to examine various options for the conversion of P2P energy trading platforms. We consider both the blockchain base layer model and the second layer solution, unlike other approaches that solely report on the base layer model. We examine blockchain mining energy consumption and compare it to household energy consumption as well as energy savings achieved through the implementation of peer-to-peer energy trading. The amount of carbon emitted by blockchain use is quantified and compared to the amount of carbon emitted by residential consumption, as well as the emission reductions achieved through peer-to-peer energy trading. Therefore, this paper aims to investigate the interconnections between blockchain technology and energy systems in terms of blockchain electricity consumption against blockchain benefit over renewable energy transitions through P2P energy trading. This research also offers numerous perspectives on the evolution of peer-to-peer energy trading through the use of various blockchain scalability solutions. The cost and advantages of blockchain-based P2P energy trading are calculated using real data from running P2P energy trading systems.
The remainder of the paper is organised as follows. Section 2 provides an overview background of the topic of this paper and the motivation to conduct this research. Section 3 summarises P2P energy trading case study (the RENeW Nexus project) and discusses blockchain energy use and carbon footprint. Section 4 presents the results of the analysis along with a discussion. Finally, Section 5 concludes the work.
This section furnishes a background on the topic of this paper. First, Blockchain and its benefits and impact in terms of P2P energy trading are discussed, followed by presenting Blockchain Trilemma and its impact on P2P energy trading. Lastly, relevant studies are examined and scrutinised, along with the motivation to carry out this research.
Blockchain is a type of distributed ledger technology that has been defined as a trust-less, distributed, decentralised, transparent, encrypted, and immutable database (Zheng et al., 2017; Kuo et al., 2017a). The ledger comprises a collection of all transactions (Christidis, Derbakova, et al., 2021). No central authority controls the transactions, which means that the control and influence are distributed through the network. The transactions are encrypted and stored in chronological order of blocks once a transaction is verified. In time, a chain of blocks is formed known as a “blockchain.” A block cannot be altered once it is verified and added to the chain; hence, it is immutable. Blockchain uses a distributed and decentralised ledger for verifying and recording digital transactions, agreements, contracts, sales, and the like in a P2P network setting (Kakavand et al., 2017).
Blockchain-enabled P2P energy ensures prosumers and consumers to exchange energy excess and demand interchangeably on a virtual P2P basis. Energy is still delivered through a physical grid since demand and supply are regulated and controlled to maintain power system stability. Prosumers and consumers are linked directly in a trustless network via a trading platform that uses a distributed ledger. They have some mistrust towards one another. A consumer or prosumer is hesitant to enable others to alter trade transactions or to allow fraudulent transactions to take place. Prosumers are encouraged to send their surplus energy into the electrical grid, while consumers may buy energy from their peers via the grid at competitive prices. In blockchain-based peer-to-peer trading, specific criteria or conditions can be defined to allow transactions to be independently and automatically validated. To avoid fraudulent actions and duplicate spending, the amounts available in the ledger must be the same before and after each transaction. The flexibility of the power market model may be demonstrated by establishing a set of regulations.
Energy billing between prosumers and customers may be performed automatically using smart contracts in real time with blockchain and IoT support utilising smart meters. Without a central billing authority, payments can be sent directly to consumers and prosumers. In contrast to the standard 2-month billing period, payments can be made more often, such as every 5 s. Consumers and utility corporations both benefit from energy micropayments and pay-as-you-go alternatives (Mylrea and Gourisetti, 2017). It is verifiable and transparent to create a time-stamped trade transaction. Energy trade transactions are stored in multi-location data structures, giving the blockchain system stability and robustness. On the other side, boosting renewable energy quantities and developing flexible and efficient electricity trading platforms can help ensure energy supply security (Ometoruwa 2018).
Renewable energy is seldom gathered after it has been transported to the grid, where it is mixed with electricity generated by hydrocarbon power plants. In terms of energy blend procurement, a market share of renewable energy may be produced throughout the whole energy mix. Blockchain technology can be used to monitor the origins of the energy that is consumed or supplied. The transparency and provenance properties of blockchain technology may affect consumer behaviour, leading in a change in energy use between peak and off-peak hours when possible. Consumers might, for example, run energy-intensive appliances during peak solar energy production hours, incentivizing them to save energy.
In summary, possible blockchain effects and benefits for energy trading include 1) an effective, adaptable, and rigorous virtual P2P sustainable electricity trade system, 2) energy security, including cyber defence and electricity generation security, 3) energy lower cost through improving energy efficiency, and 4) sustainable development by enabling renewable energy production and reduced carbon solutions.
The challenge of P2P energy trading (also for the energy sector as a whole) could be around the sheer volume of data produced, e.g., the volume of P2P data transactions run concurrently. This is the case of information of kWh produced, kWh consumed, transactions, and so on being tracked in real-time. Energy generation or consumption every 30 min would create numerous ledger entries per second for the households (Wongthongtham et al., 2021).
The performance of blockchain technology is limited, as are the tradeoffs that surround the blockchain trilemma (scalability, security and decentralisation). According to (Chitchyan and Murkin, 2018; Ometoruwa 2018), the present blockchain technology can only solve two out of three of the trilemma’s problems. The greatest long-term challenge to the viability of blockchain technology is regarded to be scalability (Abu-Salih 2021). With great security and the benefit of decentralisation, the scalability issue for blockchain technology is compromised. Even though there are techniques to address the scalability problem, security and/or decentralisation may be harmed. In the trilemma view, it is critical to investigate the consequences of blockchain-based energy trade.
In this paper, a combination of scaling solutions, which are complimentary, is modelled for P2P energy trading consisting of on-chain, off-chain, and side-chain solutions as depicted in Figure 1. Other solutions are not covered in this paper which are sharding, alternative consensus models, and directed acyclic graph. The exclusion is due to its trade-offs being compromised. Sharding reduces the load on network nodes by partitioning the blockchain into smaller sub-chains, so-called shards. This would result in more transactions being processed and verified simultaneously. The throughput increases as the mining network expands, this the transactions complete faster. However, there are some challenges such as the high-security standard, shards communication method, shards synchronisation and consensus model (Andresen 2015). Theoretically, with sharding, there is a security risk for invalid transactions being recorded by a subset of malicious nodes which might then remain unchecked. There is also a risk for validator collusion in the consensus model. To mitigate the risks, each transaction should be processed by a sufficient number of nodes. The vulnerability depends on the sharding techniques and blockchain platforms used. Complications would be challenged over cross-shard communications and synchronisation over two or more shards. Thus, sharding, in general, traded off scalability issues for security risk.
In the subsequent sections, blockchain-based three approaches, viz., on-chain, off-chain and side-chain are discussed in detail. P2P electricity trading transactions can be expedited using blockchain technology. The P2P trading cost can also be reduced by off-chain and side-chain techniques. Further, the blockchain-based P2P trading solution can be customised by setting threshold on trading time period, number of trading transcations, etc. Hence, let us discuss each solution in detail.
The Bitcoin blockchain was designed with a 1 megabyte block size restriction. Setting a block limit was designed to make sure that a malicious attacker could not effectively shut down the network by launching a denial of service attack. A denial of service attack without a block size restriction might hypothetically clog the network by sending very massive bogus data to be mined. Block sizes more than the block size limit are automatically rejected by the network when using a block size restriction.
When the blockchain requires many transactions per second, the transaction blocks may fill faster than the transactions can be processed. This causes transaction congestion and elevated transaction fees. It was then proposed to increase the block size (Andresen 2015; Garzik 2015) in order to bring down transaction fees and increase the transaction throughput. The proposed change to the block size required a software hard-fork. This meant that nodes on the network running the proposed changes would not be compatible with nodes that had not included the software upgrade. Another scaling issue arises with increasing the block size. For certain transactions, signature hash operations scale quadratically. This means that doubling the transaction size can double both the number of signature operations and the amount of data that needs to be verified. This is to be discussed further in the segregated witness protocol. The proposed solution was highly divisive and Bitcoin was forked into two different projects. The forked project, Bitcoin Cash, used the larger 8-megabyte block size as a solution to increase transaction speed and has risen since the block size to 32 megabytes.
Segregated witness (SegWit) (Shaolin, 2008) is a protocol that increased both the security and block size without requiring a hard-fork of the current Bitcoin Cash protocol (Wuille et al., 2015). All transactions on the Bitcoin Cash network are identified by a 64-digit transaction identifier. Without the SegWit protocol, it is possible for the transaction identifier to be altered by miners or relay nodes, without altering the transaction amount or wallet addresses. This is called scriptSig malleability (Song 2017). SegWit works by segregating the malleable witness parts of the identifier from the transaction identifier and introducing a new witness identifier.
Nodes not upgraded to use the SegWit protocol that still enforce the 1-megabyte block size limit can still confirm transactions by processing the transaction identifier and completely ignoring the new witness identifier. As transaction identifiers produced by the SegWit protocol do not include any of the witness data structure, mining nodes enforcing the 1-megabyte block size can fit more transactions into the block effectively increasing the transaction throughput. SegWit2x has been proposed (Garzik 2015) to force the activation of SegWit and increase the block size to 2 Mb.
The quadratic scaling of the increased block size is targeted by changing the hash function that calculates the transaction signature. The SegWit protocol ensures that any byte in a transaction is only calculated, at most, twice. In summary, SegWit increases security by fixing malleability, increases block size without requiring a hard-fork and ensures backwards compatibility, allows greater transaction throughput with increased security, makes unconfirmed transactions being able to be spent, and makes it easier in creating a lightning network, smart contracts and scripts.
The Off-chain transactions allow for low-cost instantaneous transactions across a network of participants. Off-chain transactions use smart contracts and multi-signature wallets to provide P2P transactions between participants. Off-chain transactions are often referred to as a second layer solution. This is because no fundamental changes are made to the actual blockchain, i.e., base layer or the first layer. Rather the off-chain transactions work, on the top of the main blockchain, as second layer. Therefore, the transactions between participants are not recorded on the blockchain while the payment channel is open. Only the final settlement transaction between parties is recorded to the blockchain.
Off-chain transactions are made up of two parties who first bind a committed transaction to a multi-signature wallet before entering a smart contract. The transaction is signed and sent to the blockchain for consensus and confirmation. Either party or both can agree to enforce the contract at a later date. Both parties can execute transactions with each other by first committing to the blockchain with a “commitment payment.” The parties can then conduct business as usual as long as the value of the pledge payment is not exceeded. Both parties must work together and agree on business transactions. As long as both parties have funds available within the payment channel, they can transmit as many payments to their counter-party as they like.
The parties can opt to safely end the channel after the last transaction, and the contract will pay the participants based on the final balance. The final transaction balance state is the only balance written to and broadcast on the blockchain, and the contract is completed. Transactions between participants not directly connected via a payment channel, but rather connected by proxy, are possible using off-chain networks. Off-chain networks (lightning network or Raiden network) are interconnected networks of bidirectional payment channels. Mediated payments are first secured by the sender by hashing the payment with an encrypted secret known as a preimage, known only to the recipient, locked into the contract and valid only after a particular date. This is known as a Hashed Timelock (Bowe and Hopwood 2017). Payments are securely routed by making multiple hops through payment channels connecting the participants of the payments. Intermediate network participants can claim a transaction fee and are rewarded as such by passing on the transaction to the next node along the route and by decrementing the time-lock. The final recipient can claim the transaction balance from their payment channel by producing the secret and passing it back along the chain so all nodes can claim their balance.
The Hashed Timelock Contract is revoked and the payment is claimed when the recipient can produce the secret preimage within a certain timeframe. If the balance of the transactions is not claimed by producing the preimage, the participants are refunded their bonded payment. The Bitcoin protocol is called the lightning network (Poon and Dryja 2016) and the Ethereum implementation is called the Raiden network. The benefits of off-chain transactions include 1) close to Instantaneous non-revokable transactions are possible; 2) Due to very low transaction fees, micropayments are possible; 3) Escrow services can be achieved without a third party; 4) Cross-chain payments, also known as atomic swaps are possible using Hashed Timelock Contract (Herlihy 2018); and 5) Transactions can be made anonymously (Green and Miers 2017). Limitations of off-chain transactions include 1) If a payment channel closes unexpectedly, participants are refunded their stake and the transactions are not processed; 2) Bitcoin requires SegWit to be deployed on participating nodes in order to use the Lightning Network; 3) Hardforks in the underlying blockchain may break second layer solutions; 4) Payment routing in second layer solutions are not part of the protocol and are still very early in development; and 5) Bonding payments in a smart contract ties up capital.
Side chains are an attempt to scale the enforcement and execution of smart contracts (Poon and Buterin 2017). The side chains are incentivised to continue working faithfully and autonomously by network fees enforced by the parent blockchain with bonded fraud proofs. Just like state channels, contracts are required to be bonded to the parent chain by staking a coin or token in an on-chain smart contract.
After the contract is updated or closed, participants must produce a Merkle tree proof in order for them to move their final transactions back to the main chain and settle their balance. Side chains can be used not only for payments, but extended to computation and, therefore, can be used for off-chain smart contracts. Side chains have the ability to form both nested chains and web-chains where transactions can take place across side chains (Todd 2014). The computation posed in the smart contract is broken up and distributed using mapReduce (Dean and Ghemawat 2008). Side chains do not need to use PoW and are encouraged to use PoS.
In the event of fraudulent or erroneous behaviour, the blockchain will punish the offending party. Side chains are intended to work in tandem with other scaling methods such as Raiden and the Lightning Network and are seen as complementary. In essence, side-chain lowers computation expenses and allows smart contracts to be executed quickly. However, if a large number of participants attempt to quit the contract at the same time, the network may become overwhelmed and unable to process all of the contracts.
Depending on their energy demand and production balance, individuals input energy into the electrical grid or withdraw energy from the grid in the classic form of energy trading. At a feed-in-tariff rate, electricity retailers compensate households for surplus electricity produced by rooftop solar. Prosumers and consumers trade energy directly via P2P energy trading. Prosumers are rewarded for providing renewable energy to the grid by receiving compensation in the form of a crypto-currency or a crypto-utility token. Consumers are also motivated since they can use crypto-currency to acquire energy from their neighbors at a low tariff price. The tariff price is determined by the availability and demand of renewable energy sources. A cryptocurrency trading market or a set token supply and the changeable quantity of renewable energy could determine the tariff price. When the demand for energy on the P2P network exceeds the available supply, the energy distributor feeds the network at a higher price than the market price. This further incentivises network participants to generate excess renewable energy in order to lower energy prices and reward prosumers. It is vital to remember that prosumers can be both energy users and producers.
However, there are worries about blockchain’s carbon footprint (Jiang et al., 2021; Erdogan, Ahmed, and Sarkodie 2022; Truby et al., 2022), which stems from its validation process (Mora et al., 2018a), which necessitates specialised hardware with high computing power and massive quantities of electricity. Challenges also arise from the transaction charges required to preserve the decentralised blockchain’s integrity. Few studies have accepted mathematical proof and predicted that blockchain emissions could contribute to global warming (Mora et al., 2018a; Alofi et al., 2022; Dogan, Majeed, and Luni 2022) and that it uses more energy to produce an equivalent market value as mineral mining (Krause and Tolaymat 2018). Foteinis (2018) explored the blockchain carbon footprint. They estimated the global carbon emissions of 43.9 Mt in 2017 for Bitcoin and Ethereum mining using the life-cycle impact-assessment technique (Hileman and Rauchs 2017). According to Digiconomist’s Bitcoin and Ethereum energy consumption indexes, Bitcoin consumes 55.76 TWh and Ethereum consumes 8.38 TWh of electricity yearly, equating to 0.25% and 0.04% of global electricity consumption, respectively (Digiconomist 2019a; Digiconomist 2019b).
Several studies attempted to conceptualise the linkage between Blockchain technology and carbon emission in P2P energy trading (Lu et al., 2022). For example, He et al. (2020) developed a Blockchain-based transactive energy and carbon market solutions for distributed solar systems. However, the detailed carbon market is limited and its mechanism is not addressed. Hua et al. (2020) presented an Ethereum blockchain-based decentralised energy trading platform, and its suggested pricing scheme achieves regional energy balance and carbon emission reduction. However, their approach focuses on a day-ahead scheduling whereby the outcomes may violate carbon emission limits and operating constraints in the intra-day phase (Zhong et al., 2022).
We assess the environmental impact of blockchain technology in terms of short-term energy consumption, carbon emissions, and economic value in this research. Furthermore, various blockchain scalability methods are investigated in order to explore multiple choices for the conversion of P2P energy trading platforms (Luu et al., 2016; Ledger 2018). However, salability has not been confirmed (Teng et al., 2021). Unlike many existing studies that only report on the base layer model (Jiang et al., 2021; Lu et al., 2022), we consider both the blockchain base layer model and the second layer solution. We assess blockchain mining energy consumption and compare it to residential energy usage as well as energy savings via P2P energy trading adoption. The amount of emissions emitted by blockchain use is calculated and compared to the amount of carbon emitted by residential use and the emission savings via peer-to-peer energy trading.
Through the RENeW Nexus project, the Western Australian government is the first to allow peer-to-peer energy trading across the grid. The Smart Cities and Suburbs Program of the Australian Government is funding the project (Sundararajan 2017). The project’s goal is to look into the value and efficiency of peer-to-peer energy trading in Fremantle. As part of the RENeW Nexus initiative, electric smart metres have been installed in 50 homes throughout Fremantle for a P2P energy trading trial. Energy data is collected at regular intervals (down to 5 s) and sent via the Internet of Things. The energy received from the grid, the energy given to the grid, the energy produced by rooftop PV, the energy used in homes, and so on are all included.
Throughout the trial’s research and development stage, the RENeW Nexus project employs a private consortium blockchain with no transaction fees. However, because the RENeW Nexus may switch to a public network once the trial is over, the cost of using a public blockchain is estimated for the purposes of this study. Between 1 August 2018 and 28 February 2019, data on energy production and consumption were collected at 30-min intervals from 50 participants’ residences. The study’s purpose is to compare and contrast the amount of transactions, energy consumption, and carbon emissions connected with blockchain mining, as well as transaction costs, across the two blockchain architectures (i.e., base layer model and second layer model).
The energy balance between prosumers and consumers is determined by energy supply and demand, as shown by the number of transactions in the RENeW Nexus project. There is a high level of energy consumption when the amount of energy imported exceeds the amount of energy exported. Significant levels of energy supply are indicated when the amount of energy imported is less than the amount of energy exported. The number of transactions will be calculated based on demand and supply matching. The min and max number of transactions (No.TX) are shown in Eqs 1, 2, respectively:
Where D indicates total electricity demand, S indicates total electricity supply, kt indicates the estimated transactions number, t denotes the day time slots, and i denotes number of days (i.e., 61 days).
In the event that energy demand exceeds supply, energy demand will be prioritised and matched with supply. When you rank energy demand in ascending order, you get the most transactions, and when you rank it in descending order, you get the least. In the situation of a demand gap between supply and demand, energy supply will be ranked and matched with demand. The highest number of transactions is obtained by ranking energy supply in descending order, while the smallest number of transactions is obtained by ranking energy supply in ascending order. Figure 2 depicts the minimum and greatest number of transactions during a period of 7 months. According to the distribution of transaction numbers, the lowest number of transactions is typically between 63 and 116, while the largest number of transactions is typically between 276 and 407 transactions.
In a blockchain, miners are compensated for validating transactions and preserving the network’s integrity, removing the need for a trusted third party. Blockchain is used to develop a decentralised administrative data protocol. On the other hand, the validation process demands a great deal of processing and electricity. To effectively calculate the carbon footprint of blockchains, we must first comprehend their power consumption and the carbon emissions produced by their mining activities. The carbon footprint of blockchain mining energy consumption is contrasted to the carbon footprint of electricity acquired from the grid as a result of peer-to-peer energy trading.
The technique used to calculate the amount of electricity and energy required to mine blockchain in this study follows the steps outlined in (Krause and Tolaymat 2018). To calculate the amount of power required by the entire blockchain network, the daily network hash rate was raised by the power efficiency of the mining rig (computer hardware), as shown in Eq. 3. The blockchain energy demand was then calculated by multiplying the blockchain energy requirement by the time it takes to generate a block, as shown in Eq. 4 (block completion time). As shown in Eq. 5, the transactions in the RENeW Nexus project were multiplied by the energy required to mine blockchain, and then divided by the total transactions in the entire blockchain network (5).
In general, a blockchain network’s energy consumption is the bare minimum. It is worth mentioning that the energy consumption estimates for blockchain mining do not include the energy used to cool and maintain mining equipment.
The Ethereum blockchain and its related fees were used to calculate the cost of blockchain transactions on a public blockchain. The Ethereum blockchain is a public network that uses Ether (ETH) as its cryptocurrency to pay for network transactions. The “gas” unit is used by the Ethereum blockchain network to measure the computing work involved in completing transactions or smart contracts. The price of power and processing hardware used to run the codes and complete the transactions is referred to as computational work. The cost per unit of gas, or gas price, is a critical component in the Ethereum transaction execution process. Based on the current exchange rate, the gas price in Wei units is converted into ETH units, and subsequently into USD. A total of 21,000 units of gas were used in this article for a single transaction. The expected number of transactions is then multiplied by the blockchain cost. Eqs 6, 7 demonstrate how to calculate the cost of a blockchain
Where i represents number of days (i.e., n days).
Power is exchanged in a P2P fashion on any electricity trading platform, allowing customers to adjust their consumption to meet desired objectives such as lowering electricity bills, reducing grid imports, and lowering carbon emissions. Figure 3 compares the energy saved (households’ energy import) to the energy consumed to mine blockchain grids on a daily basis.
FIGURE 3. Power expended to mine blockchain compared with households’ energy import (energy saving).
The energy consumed to mine blockchain, on an average, is less than half the energy required for households’ import, nearly 0.2 MWh. At few instances, the energy import for households’ (∼1 MWh) is more than five times the energy consumed for blockchain mining (<0.2 MWh). Not a single sample depicts more power consumption for blockchain mining in comparison to households’ import. Thus, it can be inferred that blockchain-based P2P trading is highly efficient as the households’ energy import is comparatively larger than the power consumed for blockchain mining.
Figure 4 compares the average energy consumption of the blockchain to that of the ordinary household. While residential energy usage has seasonal patterns, blockchain’s energy consumption is usually consistent. In comparison to home energy consumption, which is 1,234 kWh (average of 724 kWh) and a minimum of 446 kWh, blockchain consumers use a maximum of 226 kWh (average of 100 kWh) and a minimum of 54 kWh.
Mining can take place anywhere as long as there are electrical power available, an internet connection, and the appropriate mining rig machines. Hence miners can locate anywhere; ideally, they would be where electricity cost is cheap. It would be sustainable if miners locate where electricity is being generated by renewable energy, e.g., in Iceland, to take advantage of cleaner energy. As the miner location cannot be determined, the designation of emission in this paper is estimated using emission factors from selected countries (i.e., Australia, United States, Canada, and New Zealand). The amount of energy required is locality independent, but environmental impacts are country-specific can depend on the primary energy source.
Blockchain mined in Australia would generate approximately double the amount of carbon compared to the amount generated in New Zealand. Figure 5 shows the percentage of carbon emission from blockchain mining in the four countries compared with carbon emission saving from Australia in the P2P energy trading model.
FIGURE 5. Carbon emissions from blockchain mining in several countries are compared to carbon emission savings from Australia in the P2P energy trading system.
Household power bills are computed using a normal Western Australia tariff rate of 28 cents per unit. Figure 6 compares the average blockchain cost to the cost of electricity in a home. It is worth noting that on 19 February 2019, the average Ethereum blockchain cost climbed at a rate that was even higher than the overall cost of residential power on that day. There were four transactions on the same day with a coding problem from the same wallet address, therefore, this could be a mistake by an Ethereum developer. One spent nearly half a million dollars in transaction costs in just 4 h, making it the most costly transaction fees ever paid for a cryptocurrency payment (Williams, 2019). It is thought that the developer mixed up the transaction value and the gas price (Sukhomlinova 2019).
Because the cost of blockchain can be quite high at times, the second layer technique is an excellent option. The block finality time (i.e., the time it takes to add transactions to the chain) can be set to avoid incurring the cost of computation for each transaction added to the blockchain. As a result, a significant amount of money is saved.
For monthly block finality time, Table 1 displays the minimum and maximum base layer blockchain costs against second layer solution costs. The second layer approach saves AUD $1,749.67 on the low end and AUD $5,682.00 on the high end.
TABLE 1. A comparison in terms of the expenses of a base layer blockchain solution to the costs of a second layer blockchain solution.
Figure 7 shows the cost reductions from P2P trade with a base layer blockchain versus a second layer solution in terms of household electricity costs. When compared to the savings in family electricity from P2P trade, the second layer solution is virtually free.
FIGURE 7. Comparison of the cost of electricity saved by homes, the cost of the base layer blockchain, and the cost of the second layer solution in percentages.
Based on above graphical comparisons, blockchain-based P2P energy trading seems to be an efficient solution. However, for blockchain-based energy trade, two issues are a matter of grave concern, viz., the carbon footprint of blockchain technology and its accompanying costs. The cost and utility of implementing blockchain technology for peer-to-peer energy trading are evaluated. The energy saved in upscale P2P energy trade is compared to the energy consumed to support blockchain systems. Blockchain accounted for almost 20% of energy savings and 14% of residential energy consumption.
In addition, comparison among carbon emission savings from peer-to-peer trade and blockchain carbon emissions is accomplished. Mining activities can be carried out anywhere in the world, but it is preferable if they are carried out in locations where power is inexpensive or renewable energy sources are used to create electricity. If energy is mined in Australia, blockchain produces a 20% reduction in carbon emissions. In the United States, 15%, in Canada, 12%, and in New Zealand, 9%. We evaluate the costs of two models to examine the cost of blockchain (i.e., base layer blockchain model and second layer solution model). When compared to the base layer blockchain, the second layer solution model delivers a large cost savings (almost 90%). As a result, the second layer solution costs about 1% of current family electricity prices, whereas the base layer blockchain costs about 23% of current household electricity expenses.
Thus, it can be inferred that blockchain energy consumption cost is substantially less compared to the cost of electricity saved using blockchain-based P2P energy trading. This gap can be further widened by employing second layer blockchain solutions. Carbon emission can also be significantly reduced by utilizing blockchain technology. Hence, blockchain-based P2P energy trading considerably reduces electricity cost while saving carbon emission.
Blockchain is a very efficient technology in reducing electricity cost and carbon emission for P2P energy trading. Yet few constraints of technology and domain hinder the huge potential of blockchain in P2P electricity trading. The privacy of the users on blockchain is preserved as the users are identified using pseudonyms, such as public key, instead of real identities. But, pattern of user’s activity such as electricity consumption in different season, energy cost, energy import, etc. can be inferred to reveal more sensitive information (Wu et al., 2022). Blockchain trilemma of scalability, security and decentralization still stands as a challenge. While on-chain solutions are decentralized and secure, they lack scalability and speed to meet the demand of P2P electricity trading market. Also, on-chain transactions are costlier than off-chain transaction. Second layer solutions (off-chain and sidechain) provide scalability but are less decentralized. Concentration of power in blockchain can result in malicious activites such as inflated electricity prices. P2P electricity trading is not fully commercial and is restricted by the country regulations. Moreover, professional regulators dominate the B2B and B2C domain of the electricity market. For novice prosumers, it is a challenge to coordinate with distributed actors and well-established regulators to increase market participation while adhering to the regulations and competition in the traditional market. Overcoming these limitations can result in a secure, scalable, cost-efficient P2P energy trading. Further, it is worth indicating that Ethereum are computationally and energy-intensive with sometimes slow and expensive transactions (Buterin 2016; Chan and Olmsted 2017). In the future work we will incporporate newer and more computationally efficient blockchains which can solve this problem, such as Algorand (Gilad et al., 2017).
Blockchain is a major distributed ledger technology with a lot of promise for P2P energy trade. This paper investigates and examines blockchain-based P2P energy trade, given the growing interest in leveraging blockchain and distributed technology for P2P trading. P2P energy trading that is scalable, robust, and secure is still in its infancy, but blockchain technology promises a prospective breakthrough for energy trade and distribution. Important economic, legal, and regulatory aspects must be examined to assure the viability of blockchain-based energy trade. In this paper, economic aspects of blockchain-based P2P trading have been examined. Power expended to mine blockchain is 20% of energy savings and accounts for almost 14% of residential energy consumption. Although carbon footprint of blockchain technology and its accompanying costs have been issues of concern, blockchain produces a 20% reduction in carbon emissions when energy is mined in Australia. This reduction can be further increased at locations with renewable energy resources. Also, the blockchain average cost is 23% compared to the households’ electricity expenses. Further, this cost can be drastically diminished to 1% by employing second layer blockchain solutions, i.e., off-chain solutions and sidechain solutions. Thus, blockchain-based P2P energy trading is a secure, cost-efficient, environment-friendly solution resulting in economical electricity with reduced carbon emissions.
The raw data supporting the conclusion of this article will be made available by the authors, without undue reservation.
PW contributed to conception and design of the study. KC and BA-S organized the database. PW performed the statistical analysis. PW, KC, BA-S, MA, and NK wrote the first draft of the manuscript. All authors contributed to manuscript revision, read, and approved the submitted version.
The project is funded by the Australian Government through the Smart Cities and Suburbs Program.
KC and NK were employed by Alphabright Digital.
The remaining authors declare that the research was conducted in the absence of any commercial or financial relationships that could be construed as a potential conflict of interest.
The handling editor HH declared a shared affiliation with the author BA at the time of review.
All claims expressed in this article are solely those of the authors and do not necessarily represent those of their affiliated organizations, or those of the publisher, the editors and the reviewers. Any product that may be evaluated in this article, or claim that may be made by its manufacturer, is not guaranteed or endorsed by the publisher.
Abu-Salih, B., Al-Tawil, M., Aljarah, I., Faris, H., Wongthongtham, P., Chan, K., et al. (2021). Relational learning analysis of social politics using knowledge graph embedding. Data Min. Knowl. Discov. 35, 1497–1536. doi:10.1007/s10618-021-00760-w
Abu-Salih, B. (2021). Domain-specific knowledge graphs: A survey. J. Netw. Comput. Appl. 185, 103076. doi:10.1016/j.jnca.2021.103076
Abu-Salih, B., Wongthongtham, P., Morrison, G., Coutinho, K., Al-Okaily, M., and Huneiti, A. (2022). 'Short-term renewable energy consumption and generation forecasting: A case study of western Australia. Heliyon 8, e09152. doi:10.1016/j.heliyon.2022.e09152
Alao, O., and Paul, C. (2021). “Towards a blockchain weather derivative financial instrument for hedging volumetric risks of solar power producers,” in 2021 IEEE Madrid PowerTech, Madrid, Spain, 28 June 2021 - 02 July 2021 (Piscataway, New Jersey, United States: IEEE), 1–6.
Alofi, A., Bokhari, M., Bahsoon, R., and Hendley, B. (2022). 'Optimizing the energy consumption of blockchain-based systems using evolutionary algorithms: A new problem formulation. IEEE Trans. Sustain. Comput., 1. doi:10.1109/tsusc.2022.3160491
Andoni, M., Robu, V., Flynn, D., Abram, S., Geach, D., Jenkins, D., et al. (2019). 'Blockchain technology in the energy sector: A systematic review of challenges and opportunities. Renew. Sustain. Energy Rev. 100, 143–174. doi:10.1016/j.rser.2018.10.014
Andresen, G. (2015). Increase maximum block size. Available at: https://github.com/bitcoin/bips/wiki/Comments:BIP-0101.
AustralianPVInstitute (2022). 'Australian PV market since april 2001', Australian PV institute (APVI) solar map. Australia: funded by the Australian Renewable Energy Agency.
Bowe, S., and Hopwood, D. (2017). Hashed time-locked contract transactions. Available at: https://github.com/bitcoin/bips/wiki/Comments:BIP-0199.
Buterin, V. (2016). 'Ethereum: Platform review', opportunities and challenges for private and consortium blockchains.
Chan, W., and Olmsted, A. (2017). “Ethereum transaction graph analysis,” in 2017 12th international conference for internet technology and secured transactions (ICITST), Cambridge, UK, 11-14 December 2017 (Piscataway, New Jersey, United States: IEEE), 498–500.
Chitchyan, R., and Murkin, J. (2018). Review of blockchain technology and its expectations: Case of the energy sector. arXiv preprint arXiv:1803.03567.
Christidis, K., Anna, D., Gaur, N., Jayachandran, P., and Srinivasan, M. (2021). “Using ledger sensors to enable contextual contracts across various enterprise blockchain applications,”. Google Patents. United States;US11188883B2.
Christidis, K., Sikeridis, D., Wang, Y., and Devetsikiotis, M. (2021a). 'A framework for designing and evaluating realistic blockchain-based local energy markets. Appl. Energy 281, 115963. doi:10.1016/j.apenergy.2020.115963
Damisa, U., Nwulu, N., and Siano, P. (2022). 'Towards blockchain-based energy trading: A smart contract implementation of energy double auction and spinning reserve trading. Energies 15, 4084. doi:10.3390/en15114084
Dean, J., and Ghemawat, S. (2008). 'Mapreduce: Simplified data processing on large clusters. Commun. ACM 51, 107–113. doi:10.1145/1327452.1327492
Digiconomist (2019a). Bitcoin energy consumption index. Digiconomist.net. Available at: https://digiconomist.net/bitcoin-energy-consumption (Accessed April 24, 2022).
Digiconomist (2019b). Ethereum energy consumption index (beta). Digiconomist.net. Available at: https://digiconomist.net/ethereum-energy-consumption (Accessed April 24, 2019).
Dogan, E., Tariq Majeed, M., and Luni, T. (2022). 'Are clean energy and carbon emission allowances caused by bitcoin? A novel time-varying method. J. Clean. Prod. 347, 131089. doi:10.1016/j.jclepro.2022.131089
Dudjak, V., Neves, D., Alskaif, T., Khadem, S., Pena-Bello, A., Saggese, P., et al. (2021). Impact of local energy markets integration in power systems layer: A comprehensive review. Appl. Energy 301, 117434. doi:10.1016/j.apenergy.2021.117434
Erdogan, S., Ahmed, M., and Samuel, A. (2022). Analyzing asymmetric effects of cryptocurrency demand on environmental sustainability. Environ. Sci. Pollut. Res. 29, 31723–31733. doi:10.1007/s11356-021-17998-y
Foteinis, S. (2018). Bitcoin's alarming carbon footprint. Nature 554, 169–69. doi:10.1038/d41586-018-01625-x
Gilad, Y., Hemo, R., Micali, S., Vlachos, G., and Zeldovich, N. (2017). “Algorand: Scaling byzantine agreements for cryptocurrencies,” in Proceedings of the 26th symposium on operating systems principles (New York, New York, United States: Association for Computing Machinery), 51–68.
Green, M., and Miers, I. (2017). “Bolt: Anonymous payment channels for decentralized currencies,” in Proceedings of the 2017 ACM SIGSAC Conference on Computer and Communications Security, Dallas Texas USA, 30 October 2017- 3 November 2017 (ACM), 473–489.
Grewal-Carr, V., and Marshall, S. (2016). Blockchain enigma paradox opportunity. London, United Kingdom: Deloitte.
Hasse, F., Axel Hillebrand, T., Smole, E., Lay, L., and Charlet, M. (2016). PwC global power & utilities, Blockchain - an opportunity for energy producers and consumers? London, United Kingdom: PwC Glopal.
He, H., Zhao, L., Wang, Q., Chen, M., He, H., Gao, L., et al. (2020). 'Joint operation mechanism of distributed photovoltaic power generation market and carbon market based on cross-chain trading technology. IEEE Access 8, 66116–66130. doi:10.1109/access.2020.2985577
Herlihy, M. (2018). “Atomic cross-chain swaps,” in Podc'18: Proceedings of the 2018 acm symposium on principles of distributed computing (New York, New York, United States: Association for Computing Machinery), 245–254.
Hileman, G., and Rauchs, M. (2017). Global cryptocurrency benchmarking study. Camb. Centre Altern. Finance 33.
Hua, W., Chen, Y., Qadrdan, M., Jiang, J., Sun, H., and Wu, J. (2022). 'Applications of blockchain and artificial intelligence technologies for enabling prosumers in smart grids: A review. Renew. Sustain. Energy Rev. 161, 112308. doi:10.1016/j.rser.2022.112308
Hua, W., Jiang, J., Sun, H., and Wu, J. (2020). 'A blockchain based peer-to-peer trading framework integrating energy and carbon markets. Appl. Energy 279, 115539. doi:10.1016/j.apenergy.2020.115539
Jiang, S., Li, Y., Lu, Q., Hong, Y., Guan, D., Xiong, Y., et al. (2021). 'Policy assessments for the carbon emission flows and sustainability of Bitcoin blockchain operation in China. Nat. Commun. 12, 1938–2010. doi:10.1038/s41467-021-22256-3
Juri, M., Timo, S., Catarina, N., Riitta, S., Marianne, T., Alexandra, B., et al. (2016). “Industrial blockchain platforms: An exercise in use case development in the energy industry,” in ETLA Working Papers 43 (The Research Institute of the Finnish Economy).
Kakavand, H., Kost De Sevres, N., and Chilton, B. (2017). The blockchain revolution: An analysis of regulation and technology related to distributed ledger technologies. SSRN Electron. J. doi:10.2139/ssrn.2849251
Krause, M., and Tolaymat, T. (2018). 'Quantification of energy and carbon costs for mining cryptocurrencies. Nat. Sustain. 1, 711–718. doi:10.1038/s41893-018-0152-7
Kuo, T., Kim, H. E., and Ohno-Machado, L. (2017a). 'Blockchain distributed ledger technologies for biomedical and health care applications. J. Am. Med. Inf. Assoc. 24, 1211–1220. doi:10.1093/jamia/ocx068
Ledger, O. (2018). "A universal blockchain protocol enabling crossledger access through business modularization.
Lu, Y., Yue, L., Tang, X., Cai, B., Wang, H., Liu, L., et al. (2022). 'STRICTs: A blockchain-enabled smart emission cap restrictive and carbon permit trading system. Appl. Energy 313, 118787. doi:10.1016/j.apenergy.2022.118787
Luu, L., Narayanan, V., Zheng, C., Baweja, K., Gilbert, S., and Saxena, P. (2016). “A secure sharding protocol for open blockchains,” in Proceedings of the 2016 ACM SIGSAC Conference on Computer and Communications Security, Vienna Austria, October 24 - 28, 2016, 17–30.
Mora, C., Rollins, R. L., Taladay, K., Kantar, M. B., Chock, M. K., Shimada, M., et al. (2018a). Bitcoin emissions alone could push global warming above 2°C. Nat. Clim. Chang. 8, 931–933. doi:10.1038/s41558-018-0321-8
Mylrea, M., and Gourisetti, S. (2017). “Blockchain for smart grid resilience: Exchanging distributed energy at speed, scale and security,” in The resilience week (RWS) 2017 (Piscataway, New Jersey, United States: IEEE), 18–23.
Ometoruwa, T. (2018). Solving the blockchain trilemma: Decentralization, security & scalability. Available at: https://www.coinbureau.com/analysis/solving-blockchain-trilemma/.
Poon, J., and Dryja, T. (2016). The bitcoin lightning network: Scalable off-chain instant payments. draft version 0.5.9.2, 14.
Shamsi, M., and Paul, C. (2020). “Towards the use of blockchain prediction markets for forecasting wind power,” in 2020 6th IEEE international energy conference (ENERGYCon) (Piscataway, New Jersey, United States: IEEE), 847–852.
Shaolin, F. (2008). Mandatory activation of segwit deployment. Available at: https://github.com/bitcoin/bips/blob/master/bip-0148.mediawiki.
Song, J. (2017). Transaction malleability explained. Available at: https://bitcointechtalk.com/transaction-malleability-explained-b7e240236fc7.
Sukhomlinova, L. (2019). The mystery of 2100 ETH gas transaction, mistake or something else? Available at: https://blogs.airdropalert.com/who-gives-away-2100-eth/.
Sundararajan, S. (2017). Australian government grants $8 million for blockchain energy pilot. Coindesk. Available at: https://www.coindesk.com/australian-government-grants-8-million-for-blockchain-energy-pilot.
Teng, F., Zhang, Q., Wang, G., Liu, J., and Li, H. (2021). 'A comprehensive review of energy blockchain: Application scenarios and development trends. Int. J. Energy Res. 45, 17515–17531. doi:10.1002/er.7109
Todd, P. (2014). Near-zero fee transactions with hub-and-spoke micropayments. Available at: https://lists.linuxfoundation.org/pipermail/bitcoin-dev/2014-December/006988.html.
Truby, J., Brown, R., Dahdal, A., and Ibrahim, I. (2022). 'Blockchain, climate damage, and death: Policy interventions to reduce the carbon emissions, mortality, and net-zero implications of non-fungible tokens and Bitcoin. Energy Res. Soc. Sci. 88, 102499. doi:10.1016/j.erss.2022.102499
Wang, T., Guo, J., Ai, S., and Cao, J. (2021). 'RBT: A distributed reputation system for blockchain-based peer-to-peer energy trading with fairness consideration. Appl. Energy 295, 117056. doi:10.1016/j.apenergy.2021.117056
Williams, M. (2019). Developer’s mistake? Someone just paid $450K (3,150 ETH) for Ethereum transaction fees. cryptopotato.com. Available at: https://cryptopotato.com/developers-mistake-someone-just-paid-450k-3150-eth-for-ethereum-transaction-fees/.
Wongthongtham, P., Morrison, G., and Liu, X. (2019). The costs and benefits of blockchain based peer-to-peer energy trading: An evaluation from the perspective of carbon emission and economic value.
Wongthongtham, P., Marrable, D., Abu-Salih, B., Liu, X., and Morrison, G. (2021). 'Blockchain-enabled Peer-to-Peer energy trading. Comput. Electr. Eng. 94, 107299. doi:10.1016/j.compeleceng.2021.107299
Wu, Y., Wu, Y., Cimen, H., Vasquez, C., and Josep, M. (2022). 'P2P energy trading: Blockchain-enabled P2P energy society with multi-scale flexibility services. Energy Rep. 8, 3614–3628. doi:10.1016/j.egyr.2022.02.074
Wuille, P., Todd, P., Maxwell, G., and Russell, R. (2015). Version bits with timeout and delay. Available at: https://github.com/bitcoin/bips/wiki/Comments:BIP-0009.
Zheng, Z., Xie, S., Dai, H., Chen, X., and Wang, H. (2017). “An overview of blockchain technology: Architecture, consensus, and future trends,” in 2017 IEEE international congress on big data (BigData congress). Editors G. Karypis, and J. Zhang (Piscataway, New Jersey, United States: IEEE), 557–564.
Keywords: blockchain, blockchain carbon emission, blockchain cost, peer-to-peer energy trading, renewable energy market
Citation: Coutinho K, Wongthongtham P, Abu-Salih B, Abu Saleh MA and Khairwal NK (2022) Carbon emission and cost of blockchain mining in a case of peer-to-peer energy trading. Front. Built Environ. 8:945944. doi: 10.3389/fbuil.2022.945944
Received: 17 May 2022; Accepted: 23 August 2022;
Published: 15 September 2022.
Edited by:
Husam Abu Hajar, The University of Jordan, JordanReviewed by:
Bogdan Constantin Neagu, Gheorghe Asachi Technical University of Iași, RomaniaCopyright © 2022 Coutinho, Wongthongtham, Abu-Salih, Abu Saleh and Khairwal. This is an open-access article distributed under the terms of the Creative Commons Attribution License (CC BY). The use, distribution or reproduction in other forums is permitted, provided the original author(s) and the copyright owner(s) are credited and that the original publication in this journal is cited, in accordance with accepted academic practice. No use, distribution or reproduction is permitted which does not comply with these terms.
*Correspondence: Bilal Abu-Salih, Yi5hYnVzYWxpaEBqdS5lZHUuam8=
Disclaimer: All claims expressed in this article are solely those of the authors and do not necessarily represent those of their affiliated organizations, or those of the publisher, the editors and the reviewers. Any product that may be evaluated in this article or claim that may be made by its manufacturer is not guaranteed or endorsed by the publisher.
Research integrity at Frontiers
Learn more about the work of our research integrity team to safeguard the quality of each article we publish.