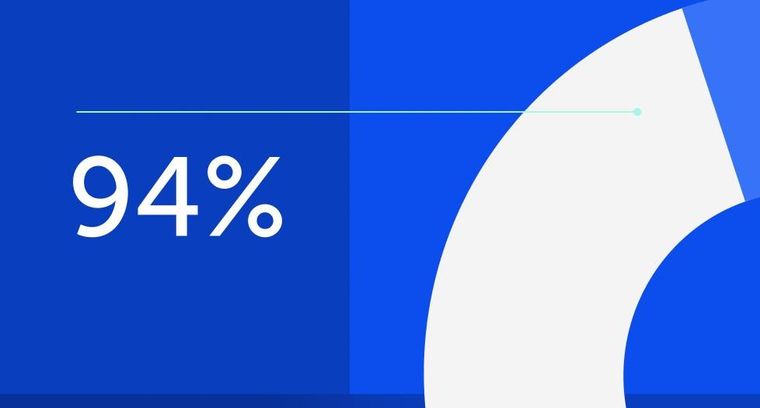
94% of researchers rate our articles as excellent or good
Learn more about the work of our research integrity team to safeguard the quality of each article we publish.
Find out more
ORIGINAL RESEARCH article
Front. Built Environ., 22 July 2022
Sec. Geotechnical Engineering
Volume 8 - 2022 | https://doi.org/10.3389/fbuil.2022.943614
This article presents experimental results and analysis of change in freezing characteristics of clays and silts with change in pH and moisture content in the pore structures. The plastic and non-plastic silts and clays in the cold regions undergo significant changes in thermal properties causing non-equilibrium thermal conditions which can lead to frost-heave, thaw-weakening, thawing-induced landslides, and mass wasting events. In geotechnical engineering, particularly in cold regions, a soil’s thermal properties play a large role in the design, functionality, and longevity of an earthen structure. The thermal properties of the soil will also govern the porous media phase changes influencing thermal hysteresis and heat capacity in soils. These variables will change with seasonal freeze–thaw cycles, which can lead to changes in a soil’s structure, fabric, density, moisture content, and strength over time. With global warming causing the temperatures to gradually rise over time, the rapidly varying seasonal freeze–thaw cycles are now becoming an issue in areas where the designs have relied heavily on the permafrost. This research study investigates the fundamental changes to freezing and thawing characteristics of plastic and non-plastic silts with changes in frost penetration rates (cooling rate); moisture content (liquid limit, plastic limit, and optimum moisture content); pH (2–7); and soil type with different percentages of fines content and specific surface area.
Climate change has a significant effect on land surface characteristics, especially in the permafrost and seasonally frozen ground regions where the topography is continuously changing due to changing weathering patterns (Zhang et al., 2003). As climate change continues to result in more extreme weather events (Venäläinen et al., 2001; Perera et al., 2020), a deeper understanding of soil-porous media interactions is needed to evaluate the resiliency and vulnerability of earthen infrastructure including dams, levees, and highway embankments (Gopalakrishna et al., 2013). The soil-porous media in the cold regions undergo complex thermo-chemical-mechanical processes associated with phase changes of porous media from water to ice and vice versa (Zhang and Michalowski, 2015; Zhang et al., 2016b; Lamontagne-Hallé et al., 2020). These phase changes in frost-susceptible soils can lead to frost-heave (Penner 1967; Ferris 2009; Peppin and Style 2013) and thaw-weakening (Gatto, 1995; Brams and Yao 1964) phenomena, causing significant damage to civil infrastructure. Chang and Liu (2013) reported that the frost-heave and thaw-weakening settlement rely on the density of soils, where both strain-hardening and strain-softening behavior is observed with freeze–thaw cycles. It was also observed that the variation of volumetric unfrozen water content or volumetric ice content in soils during the freeze–thaw process can directly determine the frost heave and thaw settlement of infrastructure in cold regions (Zhang et al., 2019).
Over the years, different techniques were implemented in the field to mitigate the frost-heave and thaw-weakening behavior. Briefly, these techniques are replacing frost-susceptible materials (AASHTO guide), chemically modifying the soils to lower the freezing point of the moisture (Lambe 1956; Christopher et al., 2006; Rahman and Bheemasetti 2021), using geosynthetics and gravel in subsurface soils to improve drainage and act as capillary barriers (Kuosa et al., 2014; Lin and Zhang 2016) and place the foundation below the frost-depth zone (Linell 1980). Despite several existing methods, still there are many challenges associated with the infrastructure in cold regions especially with changing weather patterns and significant variability in the interannual seasonal cycles. To understand, evaluate, and predict the macro-scale behavior of frozen soils, a fundamental understanding is required of the thermal properties and associated phase changes of porous media in frost-susceptible soils.
The thermal properties including conductivity, resistivity, specific heat, freezing point, thawing point, and hysteresis of soils play a vital role in mechanisms governing frost-heave, thaw-weakening, depth of frozen soil, and the rate at which heat extraction occurs in the seasonally frozen ground (Farouki 1981; Campbell et al., 1994; Ochsner et al., 2001). However, the thermal properties are influenced by variables such as soil pH, moisture content, density, and rate of cooling/thawing of the soil sample. Previous studies stated that the specific heat and volumetric heat capacity of soils typically increased with increasing moisture content and density (Abu-Hamdeh 2003). Few studies have studied the influence of soil mineralogy, pore-structure, and fabric of soils on the thermal properties of the soils (Tarnawski and Wagner 1992; Lu et al., 2016; Hussary et al., 2022). Along with this, the thermal properties and soil freezing process is coupled with the heat extraction and the presence of porous media in the forms of vapor, ice, and water. It was observed that the water in the vapor state does not have a large influence on the soil-freezing process (Harlan, 1973; O’Neill and Miller., 1985; Zhang et al., 2016a), and hence the phase changes between the ice and water are critical in governing the thermal properties.
The phase changes of the porous media in frost-susceptible soils rely on the intermolecular forces between soil–water, the geometry of fluids, and soil surface characteristics (Rempel et al., 2004). Earlier experimental studies on the phase changes in soils are limited to the evaluation of the freezing point and thawing point of control and stabilized soils (Rahman and Bheemasetti 2021). It was reported that the frost-heave of the soils can be mitigated by lowering the freezing point of the soils by using calcium-based stabilizers. However, a recently concluded study indicated that cement stabilizers can alter the thermal properties of the soils and can cause more distresses to the pavement infrastructure (Oman et al., 2018). Also, repeated freezing and thawing in cold regions strongly weathers and deteriorates the geotechnical properties of the densely compacted foundation soil, namely., change in micro-fabric and loss in strength (Li et al., 2012). It was reported that the propensity for frost-heave under freezing conditions is affected by properties such as grain size, rate of freezing, availability of water, applied loads, and coupling of heat flow and mass flow (Konrad & Morgenstern 1980; Horiguchi 1987). Along with this, the pH of the soil strongly governs the thermal–chemical–mechanical behavior of stabilized soils of the soils in the cold regions (So and Aylmore. 1993; Mitchell and Soga 2005; Tebaldi et al., 2016). It was reported that chemical stabilization, which is one of the predominant ground improvement techniques, strongly relies on the pH and soil plasticity characteristics (Puppala 2021). Based on the literature review, it can be observed that the phase change studies are a complex process, and more information and experimental evidence are required on how the changing climate and surface characteristics will influence the phase changes and corresponding thermal properties.
The main objective of this study is to evaluate the phase change points of the plastic and non-plastic silts and their corresponding thermal properties with variables including pH and water content. The plastic and non-plastic silts are susceptible to frost-action, and studies on these soils will contribute to essential information on thawing-induced mass-wasting events, differential settlement, cracking, and other pavement distresses. The following sections present the materials, experimental studies, results, and discussions.
Soils from two different geological formations within South Dakota (SD) were selected for these studies. Previous studies on several mass-wasting events in the South Dakota identified that the soils are predominantly isolated silt-sized grains in a matrix of clay size particles with both plastic and non-plastic behavior (Erskine 1959). In this research study, two different soil types of plastic and non-plastic silt were selected for this research. Using the Unified Soil Classification System (USCS), the two soils are classified as clayey sand (SC) and elastic silt (MH). Also, according to frost-susceptibility criteria, both soils are identified as high to relatively high frost susceptibility.
The MH elastic silt was collected from the Chadron formation and has been described as pale-gray-green bentonitic clay altering with layers of greenish-gray siltstone and basal conglomerate. The Chadron formation is a part of the larger White River group which was deposited during the late Eocene and early Oligocene and represents a depositional pulse in the great plains resulting from a great influx of volcanic ash and dust from the west (Larson and Evanoff, 1998; Evanoff et al., 2010; Stetler, 2018). The soil in this group also has been observed to have a high infiltration rate due to the high amount of desiccation cracks. The Chadron formation contains mostly clay-rich sediment and is typically a gray to an olive-gray sandy clay that was deposited on top of the weathered and oxidized Pierre shale (Stetler, 2018). The Chadron Formation can be found in North Dakota, South Dakota, Nebraska, Wyoming, Montana, and Colorado (Larson and Evanoff, 1998), representing a large geological depositional area.
The SC clayey sand was collected near Yankton, SD, and has been identified as part of the Loveland loess formation. It has been characterized as silt with some clay and fine-grained sand ranging in color from reddish-brown to yellowish-brown due to the oxidization characteristics of this soil. It lies between till and outwash of post-Kansan age and underlies yellowish-brown loess of post-Iowan pre-Mankato age soil (Simpson, 1960). The loess deposits in the area are derived primarily from glacial outwash and wind action. This has resulted in a high degree of particle sorting and decreasing layer thickness as the distance from the source increases. Due to its particle size and distribution, it has a relatively high permeability, allowing for moisture to build up within the soil during the seasonal weather patterns. If a large enough amount of moisture is stored in the soil during an FT cycle, the soil can become highly susceptible to frost heave and another frost-induced distresses.
X-ray diffraction (XRD) studies were performed on both the soils to identify the mineralogy. Figure 1 presents the XRD scans on two soils. It can be observed that different mixtures of minerals were present in both the representative soil samples. The XRD analysis is based on the mechanism that the x-rays will strike and displace an electron from the inner shell of the soil, and the outer shells then fall into the vacancy. In this process, the intensity of radiation and corresponding wavelength is determined which is presented in the Figure 1.
The percentage of minerals is determined by using counts per second to 100 percent using the procedure illustrated in earlier works (Schultz 1964). The clayey sand (Figure 1B) predominantly consists of quartz, whereas the elastic silt (Figure 1A) consists of montmorillonite. The mixtures of these minerals govern the thermal behavior and corresponding strength-volume change deformation characteristics of the soils. The montmorillonite soil tends to swell when in contact with the water, causing significant distress to the infrastructure, whereas the mixtures of fine sand silt-sized particles are frost-susceptible, which can result in frost-heave and thaw-weakening in the winter and spring seasons.
To investigate the interdependencies that exist with a soil’s thermal properties, its moisture content, cooling rate, pH, and soil type were varied and tested in multiple combinations. Three different moisture levels were chosen for each soil type based on the consistency of soils constituting semisolid and plastic state (plastic limit), plastic and liquid state (liquid limit), and optimum moisture content. Earlier studies have identified that these states rely on the fundamental soil characteristics such as soil mineralogy, particle size distribution, pore fluid chemistry, and pore structure, and freezing curves for soils were established based on soil sorptive potential (Zhang and Lu 2021). Hence, in this study, the optimum moisture content (OMC), plastic limit (PL), and liquid limit (LL) were used for testing SC soil, whereas for the MH plastic silt, the moisture content of 15%, PL, and LL was selected. In the MH soil, the plastic limit and optimum moisture content were within 5%, and hence lower moisture content was selected. The OMC of the soils was determined using a standard proctor test performed according to ASTM D698-12. Table 1 presents the moisture content values selected for phase change studies.
Based on the rate of cooling, soil type, and availability of unfrozen water content, three distinct frozen soil structures including homogeneous, layered, or lattice and with many gradations between soils can be formed in the subsurface environment (Taber 1929; Farouki 1981; Horiguchi 1987). However, there is little to no information on how the cooling rate will influence the freezing point in soils with different surface characteristics. This information is particularly critical; as with climate change, significant variability in the weathering patterns is observed which can further influence the magnitude of ice formation. Hence, in this study, the two candidate soils were tested to evaluate their freezing points at different cooling rates of −1°C/min, −1.5°C/min, −2°C/min, and −2.5°C/min at the three moisture contents (OMC, PL, and LL). These cooling rates were selected to provide a variety of testing values to see if the cooling rate altered the thermal properties of the soil.
The pH of the soil is another important parameter that can alter the thermal properties and influence the soil behavior (Lawrence et al., 2014; Bakhshipour et al., 2016; Momeni et al., 2022). Natural events such as acid rain (Tabatabai and Olson 1985), animals (Hole 1981), and organic processes (Yan et al., 1996) can increase the soil’s pH. To evaluate the influence of pH (2–6) on the freezing characteristics, a series of experiments were conducted on the two candidate soils and three different moisture contents at a cooling rate and thawing rate of −2.5°C/and 5°C/min. Oxalic acid, which is the smallest dicarboxylic acid, was selected as the organic acid for increasing the pH of the field soils. This acid is naturally produced by a variety of lichens and other plant life and plays an important role in soil processes (e.g., mineral weathering and metal detoxification in plants) (Studenroth et al., 2013). Table 2 below presents different variables that are considered in this study. A total of 252 tests were conducted that include three sample testings for each parameter.
The phase changes and corresponding freezing characteristics and heat flow of the soils were evaluated using differential scanning calorimetry (DSC) and a transient line source probe. To perform the abovementioned tests, first, the field soils were mechanically ground, oven-dried, and sieved over a No. 200 sieve. Approximately 20 mg of the sieved soil was placed in the zero pan, and de-ionized water was added to the soil to bring the soil mass and water content to the desired OMC, liquid limit, and plastic limit as provided in Table 1. After the samples were hydrated, they were sealed using a lid that was crimped on to help mitigate moisture loss during testing. To test the influence of pH, oxalic acid is added to the soil–water mixture. Once the samples were prepared and ready to be tested, they were placed into the DSC machine loading bay where they would then be loaded into the testing chamber. The minimum and maximum temperature along with its cooling/thawing rate was set to desired values. After each sample had undergone testing, the data were analyzed to find the soil’s freezing point, thawing point, thermal hysteresis, heat capacity, and heat flow.
This section presents the results and discussion on the influence of cooling rate on phase changes in plastic and non-plastic silts. Due to both the soils having high silt contents but different plastic/liquid limits, they were seen as ideal test specimens. Konrad & Morgenstern (1980) stated that “it is well-known that the propensity for [frost] heave of a soil under freezing conditions is affected by properties such as grain size, by the rate of freezing, by the availability of water, and by the applied loads.” However, it was never stated how different the cooling rates need to be to drastically influence the soils frost heave capabilities. The moisture contents tested for the MH and SC soil were OMC (15%, 14%), PL (37%, 22%), and LL (74%, 35%). Four different cooling ramping speeds were considered including −1.0, −1.5, −2.0, and −2.5C/min, and tested in conjunction with the varying moisture contents of the soil specimens. A thawing ramping speed of 5.0°C/min was selected and held constant throughout all testing processes. Figure 2 A, B presents the freezing point depressions recorded for each soil tested. For each soil, its lowest moisture content experienced the coldest freezing depression for each cooling ramp tested.
It can be observed from Figure 2 A that with an increase in water content, the freezing point is high, whereas with lower moisture content, the freezing point is decreased by an order of 1.5–2C. This can be mainly attributed to the interparticle forces between the soil surface and water. Based on the diffuse double layer theory, it can be inferred that the interparticle forces are a function of specific surface area and as the distance of water from the soil particle increases, the free water is subjected to thermal stresses. The equations and theory of diffuse double layer theory can be found elsewhere (Mitchell and Soga 2005). However, with less water, the interparticle forces dominate and influence the freezing characteristics of the water. Based on Figure 2 A, it can be observed that the rate of cooling does not have a pattern with respect to the freezing point in elastic silts. At a constant rate of cooling, the soils with more water content will freeze first than the soils with lower moisture content.
Unlike the results on elastic silt, the clayey sand depicted a clear pattern (Figure 2 B). As the rate of cooling increases, the freezing point of the soil is decreased. Similar to elastic silts, the soils with higher moisture content have undergone phase changes quickly compared to the soils with plastic limits and optimum moisture content. At a constant rate of cooling, the freezing point with high water content has gone through phase changes first. Overall, both the soils depicted that the soils with the lowest moisture content experienced the coldest freezing point, the highest moisture content experienced the warmest freezing point, and the PL was somewhere in between. Figure 3 presents the plot of freezing points of both the soils with the rate of cooling.
It can be observed from the abovementioned plot that the freezing point values within the outer envelopes converge as the rate of cooling decreases. An average linear trend (Equation 1) is obtained using regression analysis where the freezing point can be determined based on the rate of cooling. From the obtained data and Equation 1, it can be inferred that the freezing point of the soils on an average is −3.53C. This value is below the conventional ice-water phase change temperatures as the nucleation on soil particles is governed by the porous media characteristics, soil type, and soil-porous media intermolecular forces.
where dT is a change in temperature and dt is change in time.
Earlier studies have demonstrated the influence of pH on the mechanical behavior and aggregation of soil particles (Jepson 1984; Zhou and Gunter 1992; Carty 1999; Ma and Eggleton 1999; Wang and Siu 2006). It was observed that when the pH of the porous media is below the isoelectric point, the soil particle favors a more opened edge-to-face formation, whereas when the pH is more than the isoelectric point, the soil favors a more dispersed and deflocculated fabric. These formations indeed control the macro-behavior of the soils. The investigative studies on the effect of the pH on the phase changes from water to ice and vice versa are limited. Hence, to evaluate the influence of pH on freezing characteristics including phase changes, thermal hysteresis, and specific heat capacity, the soils with different pH values were studied.
In this study, the oxalic acid solution was used to alter the moisture content of the soil during sample preparation and was prepared on the day of testing to ensure accurate pH solutions were used. A cooling rate of −2.5°C/min was kept constant. Figure 4 presents the influence of pH on the freezing point of clayey sand and elastic silt collected from two different geologic formations. It can be observed from both these plots that the freezing behavior of the soil changed with the change in pH of the porous media. It shall be noted that the current studies have evaluated the freezing behavior of the soil only for pH values less than 7.0. From the plots, it can be observed that as pH is decreased, the freezing point of the soil has decreased; however, with pH greater than 4.0, the soil freezing point behavior is reversed. Also, it can be observed that the elastic silts are more reactive to the changes in pH of the porous media than the clayey sand soils. This can be attributed to the montmorillonite mineral and negative surface charges on the elastic silt, whereas the clayey sand has predominantly quartz, which is less reactive to the water with different pH conditions. Also, it can be observed that the elastic silts became independent of the water content where the freezing point is approximately the same at a pH value of 4-5.
Figure 5 presents the thermal hysteresis plots of SC soil as a function of the pH of the porous media. It can be observed that all the soils exhibited significant thermal hysteresis with a decrease in pH values. Also, a critical observation of the thawing points reveals that the melting of ice did not happen at the same temperature. This is critical in the subsurface environment that the thawing of ice (rate and corresponding pore pressures) depends not just on the temperature but on the soil conditions too and the internal structure of the ice that is formed due to the gradient in temperature.
FIGURE 5. Thermal hysteresis plots of clayey sand soil with different pH values and moisture contents: (A) plastic limit; (B) OMC; (C) LL.
Figure 6 presents the thermal hysteresis plots for the elastic silts as a function of pH and moisture content. Similar observations in clayey sand were observed with elastic silt soils. A close comparison of the thawing points on the clayey sand and elastic silt reveals that for the ice to thaw in the MH soils, the required temperatures are higher than those of the clayey sand. This can be attributed to the strong nucleation on the clay and silt surfaces.
FIGURE 6. Thermal hysteresis plots of elastic silt with different pH values and moisture contents: (A) plastic limit; (B) OMC; (C) LL.
In Figures 5, 6, a general trend emerges for the OMC and PL moisture contents. Soil with pH values 2, 3, and 7 have the coldest freezing points and soils with pH values 4, 5, and 6 act as transition values between pH 3 and 7. For the LL moisture content, it is observed that the soils with pH 2, 3, and 7 have warmer freezing points and pH 4, 5, and 6 have colder freezing depressions. These correlations can play an important role in determining the freezing depression a soil may experience in the field.
Figure 7 shows the changes in specific heat required for freezing and thawing, with varying pH values for all different moisture content tested for the SC soil type. The specific heat required for the freezing phase change to occur was always higher than the specific heat required for the same sample to thaw for all samples tested. It was observed that for soils with a pH of 2 and 3, the specific heat required to phase change increases with increasing moisture content and vice versa for samples with a pH of 5 and 6. The sample with a pH of 4 is behaving as a transitional value that exhibits traits seen on either side of it. When the soil sample had a pH of 3 at its LL, it had the highest specific heat capacity required for phase change with nearly double the value of all other samples tested.
Figure 8 shows different specific heat capacities observed for the MH soil for different soil pH values. The specific heat for the samples to freeze was either the same or higher than the specific heat for the samples to thaw. For soil pH values 2–3, similar trends can be seen as seen with the SC soil, and the pH 4 sample has its unique heat capacity relationship. However, the trend from the SC soil no longer exists with the pH 5 or 6 samples, pointing to a breakdown in the trend as the sample becomes more basic and closer to neutral conditions.
FIGURE 8. MH soil change in specific heat capacity with soil pH. Influence of fine content on freezing characteristics of soils.
Earlier studies have reported that the fine content present in the soil matrix plays a key role in the mechanical and hydraulic behavior of the soils. Especially predominant work has been performed to evaluate the liquefaction behavior and drained and undrained behavior of soils with an increase in the fine content (Tokimatsu and Yoshimi 1983; Bolton Seed et al., 1985). Also, it was observed that the thermal behavior of the fine-grained soils with silts is related to the amount of moisture content present in the soil (Salomone et al., 1984). However, sparse information was available on the influence of fine content on the phase changes of the porous media. In this study, the influence of fine content on freezing–thawing characteristics was observed. Figure 9 presents the plots on the freezing–thawing points and thermal hysteresis for both plastic and non-plastic soils with different three different moisture contents (OMC, PL, and LL).
FIGURE 9. Influence of fine content on freezing characteristics of plastic and non-plastic soils: (A) freezing point; (B) thawing point; (C) thermal hysteresis.
From the plots, it can be observed that the non-plastic silts undergo phases at temperatures below −4C, whereas the plastic silts undergo freezing approximately at −3C. The soils prepared at OMC are less susceptible to water to ice phase changes compared to the soils with PL and LL. On the contrary, the soils with OMC are more susceptible to thawing than the soils with PL and LL. Also, the plastic silts require higher warmer temperatures to completely thaw the samples compared to the non-plastic silts. Both the soils exhibited approximately the same thermal hysteresis; however, the freezing and thawing points are different which can be attributed to fundamental mineral threshold values and surface area of the soils.
Figure 10 presents the influence of the external specific surface area of each soil with respect to heat flow and corresponding phase changes in plastic and non-plastic silts. The heat flow (W/g) through the soils at the freezing and thawing stages is obtained from the differential scanning calorimetry tests. The specific surface area of the soils is determined from the correlations provided by Rieke (1982). To evaluate the effect of the specific surface area of the fines on the freezing and thawing point, the heat flow and normalized water content (ω) are plotted in Figure 10. A linear trend is observed between the heat flow and normalized water content, depicting that an increase in the water content increased the heat flow through the soils for the freezing phase change state, whereas during the thawing state, a negative trend was observed depicting that with an increase in normalized water content resulted in a decrease in the heat flow through the soils. Also, the heat flow through the silts is comparatively low than that of the non-plastic silts.
FIGURE 10. Influence of specific surface area on freezing–thawing characteristics of plastic and non-plastic silts. Influence of freeze–thaw cycles on thermal conductivity of soils.
The soil thermal properties, especially thermal conductivity, have been widely used parameters in geotechnical engineering for evaluating the heat transfer mechanisms. Previous studies have demonstrated different techniques to determine thermal conductivity using different methods. However, how the climate change with changing moisture content and freeze–thaw cycles influences the thermal conductivity is not comprehensively understood. This study presents the test results and analysis of thermal conductivity using a transient line heat source using TLS100. First, to simulate the field conditions a custom top–down freezing system was developed. To ensure there are no heat losses, the top–down freeze–thaw box was validated using a Type J/K Data Logging Thermocouple Meter. A probe was inserted at the bottom and top of the sample before placing the box into the freezer. Measurements were taken immediately after placing the box in the freezer and 12 h after to verify that a temperature gradient existed during the freezing process. The temperature readings of the top and bottom were (13.46°F, 67.82°F) and (−7.6°F, 29.3°F), respectively. It can be seen that a substantial temperature gradient existed between the top and bottom of the soil sample, thus simulating in situ freezing conditions that a soil mass will undergo in the field.
The SC and MH soil samples were compacted and prepared according to ASTM D6898. After compaction, each soil sample was placed in a sealed bag to prevent any moisture loss throughout the weathering cycles and then placed in the top–down freezing setup where each soil type was subjected to 0, 1, 2, 10, and 20 FT cycles. During the freezing stage, the soil was placed in a freezer with ambient air temperatures of −15°F for 12 h then removed and allowed to thaw for 12 h at 70°F. After each set of FT cycles, the samples were removed and tested for thermal conductivity using TLS100. Figure 11 presents the results of the MH and SC soils prepared at different moisture contents and subjected to 20 freeze–thaw cycles. It can be observed that the thermal conductivity of the SC soil prepared at ±5% OMC significantly increased after 10 freeze–thaw cycles. This can be attributed to the interparticle arrangement and degradation of the soils with more air voids through which the heat can conduct, whereas the MH soils showed consistency in the thermal conductivity values with repeated freeze–thaw cycles which can be attributed to the particle size and dominant clay fraction present in MH soils.
FIGURE 11. Influence of freeze–thaw cycles on thermal conductivity of MH and SC soils prepared at (A) −5% OMC; (B) OMC; (C) +5% OMC.
The main objective of this articleis to understand the influence of different parameters including soil type, moisture content, pH, and cooling rate on soil freezing–thawing characteristics. With changing climate, the subsurface environment will undergo different seasonal weather patterns compared to the past which can further lead to changes in a soil’s thermal properties over time. In cold regions, the thermal properties of soil play a key role in the geotechnical design and performance of structures that undergo seasonal freeze–thaw cycles. This study is aimed to understand how the phase changes would occur in plastic and non-plastic silts with changing conditions, and the following observations are made:
• The non-plastic clayey sand showed that with an increasing rate of cooling, the freezing point of the soil is lowered, whereas the plastic elastic silts did not exhibit a clear trend and indicated that the rate of cooling does not have a significant influence on the freezing point. Also, it was observed from Figure 2 A that with an increase in water content (at the liquid limit) the freezing point is high, whereas, with lower moisture content (OMC), the freezing point is decreased to 1.5–2C. A linear correlation was obtained to determine the freezing point of the soils with the change in the rate of cooling. The intercept −3.54 in equation 1 indicates that the soil’s freezing point is not just governed by the temperature but relies on the nucleation mechanisms and the adsorbed forces of water film on the soil particles.
• The effect of pH on the phase changes was studied by altering the soil’s pH using an oxalic acid solution. It was observed that the freezing behavior of the soils changed with the change in pH of the porous media. The pH value of 4.0 was identified as the transitioning point where the freezing behavior of the plastic and non-plastic soils changed. From Figure 4 plots, it was observed that the elastic silts in comparison to the clayey sands are more reactive to the changes in pH of the porous media. This can be attributed to the dominant montmorillonite mineral in elastic silts, whereas the clayey sand has predominantly quartz.
• Thermal hysteresis is observed for both the plastic and non-plastic soils at all pH values. The net thermal hysteresis was observed to be between −5C and −7.5C for both the soils; however, the range of freezing and thawing temperatures is different which are governed by the specific heat capacity of SC and MH soils, which can further govern the thermal properties and corresponding mechanical behavior.
• The effect of fines content on the freezing–thawing characteristics was analyzed with respect to the moisture content. It was observed that the soils prepared at OMC are less susceptible to water-to-ice phase change, whereas the soils with OMC are more susceptible to the ice-to-water phase changes in comparison to the soils with PL and LL. Also, the plastic silts require higher warmer temperatures to completely thaw the samples than the non-plastic silts. The effect of the specific surface area of the fines on the freezing and thawing point was analyzed by introducing the normalized water content parameter (ω). Positive and negative linear trends were observed (Figure 10) between the heat flow and normalized water content for both the freezing and thawing stages.
The raw data supporting the conclusion of this article will be made available by the authors, without undue reservation.
All authors listed have made a substantial, direct, and intellectual contribution to the work and approved it for publication.
The authors declare that the research was conducted in the absence of any commercial or financial relationships that could be construed as a potential conflict of interest.
All claims expressed in this article are solely those of the authors and do not necessarily represent those of their affiliated organizations, or those of the publisher, the editors, and the reviewers. Any product that may be evaluated in this article, or claim that may be made by its manufacturer, is not guaranteed or endorsed by the publisher.
Abu-Hamdeh, N. H. (2003). Thermal Properties of Soils as Affected by Density and Water Content. Biosyst. Eng. 86 (1), 97–102. doi:10.1016/s1537-5110(03)00112-0
Bakhshipour, Z., Asadi, A., Huat, B. B., Sridharan, A., and Kawasaki, S. (2016). Effect of Acid Rain on Geotechnical Properties of Residual Soils. Soils Found. 56 (6), 1008–1020. doi:10.1016/j.sandf.2016.11.006
Bolton Seed, H., Tokimatsu, K., Harder, L. F., and Chung, R. M. (1985). Influence of SPT Procedures in Soil Liquefaction Resistance Evaluations. J. Geotech. Engrg. 111 (12), 1425–1445. doi:10.1061/(asce)0733-9410(1985)111:12(1425)
Brams, B. B., and Yao, L. Y. (1964). Shear Strength of a Soil after Freezing and Thawing. J. Soil Mech. Found. Div 90 (Proc. Paper 3958). doi:10.1061/JSFEAQ.0000629
Campbell, G. S., Jungbauer, J. D., Bidlake, W. R., and Hungerford, R. D. (1994). Predicting the Effect of Temperature on Soil Thermal Conductivity. Soil Sci. 158 (5), 307–313. doi:10.1097/00010694-199411000-00001
Chang, D., and Liu, J. (2013). Review of the Influence of Freeze-Thaw Cycles on the Physical and Mechanical Properties of Soil. Sci. cold arid regions 5 (4), 457–460.
Christopher, B. R., Schwartz, C. W., Boudreaux, R., and Berg, R. R. (2006). Geotechnical Aspects of Pavements (No. FHWA-NHI-05-037). United States: Federal Highway Administration.
Erskine, C. F. (1959). Landslides in the Vicinity of the Fort Randall Reservoir, South Dakota (No. 675). US Govt. Print. Off.
Evanoff, E., Terry, D. O., Benton, R. C., Minkler, H., Terry, M. P., Duke, E. F., et al. (2010). Field Guide to Geology of the White River Group in the North Unit of Badlands National Park. S. D. Sch. Mines Technol. Bull. 21, 96–127.
Farouki, O. T. (1981). Thermal Properties of Soils. Hanover, New Hampshire: Cold Regions Research and Engineering Lab Hanover NH.
Ferris, G. (2009). Differential Frost Heave at Pipeline-Road Crossings Cold Regions Engineering 2009. Calgary, AB, 68–78.
Gatto, L. W. (1995). Soil Freeze-Thaw Effects on Bank Erodibility and Stability. Hanover, New Hampshire: U.S. Army Cold Regions Research and Engineering Laboratory.
Gopalakrishna, D., Schroeder, J., Huff, A., Thomas, A., and Leibrand, A. (2013). Planning For Systems Management & Operations As Part of Climate Change Adaptation (No. FHWA-HOP-13-030). Washington, D.C: United States. Federal Highway Administration. Office of Operations.
Harlan, R. L. (1973). Analysis of Coupled Heat-Fluid Transport in Partially Frozen Soil. Water Resour. Res. 9 (5), 1314–1323. doi:10.1029/wr009i005p01314
Hole, F. D. (1981). Effects of Animals on Soil. Geoderma 25 (1-2), 75–112. doi:10.1016/0016-7061(81)90008-2
Horiguchi, K. (1987). Effect of Cooling Rate on Freezing of a Saturated Soil. Cold Regions Sci. Technol. 14 (2), 147–153. doi:10.1016/0165-232x(87)90030-9
Hussary, J., Alowaisy, A., Yasufuku, N., Ishikura, R., and Abdelhadi, M. (2022). Pore Structure and Falling Rate Stage of Evaporation in Homogeneous Sandy Soil Profiles. Soils Found. 62 (2)–101108. doi:10.1016/j.sandf.2022.101108
Jepson, W. B. (1984). Kaolins: Their Properties and Uses. Philosophical Trans. R. Soc. Lond. Ser. A, Math. Phys. Sci. 311 (1517), 411–432.
Konrad, J. M., and Morgenstern, N. R. (1980). A Mechanistic Theory of Ice Lens Formation in Fine-Grained Soils. Can. Geotech. J. 17 (4), 473–486. doi:10.1139/t80-056
Kuosa, H., Niemeläinen, E., Kivikoski, H., and Törnqvist, J. (2014). Pervious Pavement Winter Performance-State-Of-The-Art and Recommendations for Finnish Winter Conditions. VTT Tutkimusraportti VTT.
Lambe, T. W. (1956). Modification of Frost-Heaving of Soils with Additives. Highw. Res. Board Bull. 135.
Lamontagne‐Hallé, P., McKenzie, J. M., Kurylyk, B. L., Molson, J., and Lyon, L. N. (2020). Guidelines for Cold‐regions Groundwater Numerical Modeling. Wiley Interdiscip. Rev. Water 7 (6), e1467. doi:10.1002/wat2.1467
E. E. Larson, E. Evanoff, D. O. Terry, H. E. LaGarry, and R. M. Hunt. (1998). Tephrostratigraphy and source of the tuffs of the White River sequence. Special Papers-Geological Society of America, 1-14.
Lawrence, C., Harden, J., and Maher, K. (2014). Modeling the Influence of Organic Acids on Soil Weathering. Geochimica Cosmochimica Acta 139, 487–507. doi:10.1016/j.gca.2014.05.003
Li, R., Shi, H., Flerchinger, G. N., Akae, T., and Wang, C. (2012). Simulation of Freezing and Thawing Soils in Inner Mongolia Hetao Irrigation District, China. Geoderma 173-174, 28–33. doi:10.1016/j.geoderma.2012.01.009
Lin, C., and Zhang, X. (2016). A Bio-Wicking System to Mitigate Capillary Water in Base Course. Center for Environmentally Sustainable Transportation in Cold Climates.
Linell, K. A. (1980). Design and Construction of Foundations in Areas of Deep Seasonal Frost and Permafrost. U. S. Army Cold Regions Res. Eng. Laboratory 80 (No. 34).
Lu, H., Zhang, Q., Dong, Y., Li, J., and Zhang, X. (20162016). The Adsorption Capacity, Pore Structure, and Thermal Behavior of the Modified Clay Containing SSA. Adv. Mater. Sci. Eng. doi:10.1155/2016/9894657
Ma, C., and Eggleton, R. A. (1999). Cation Exchange Capacity of Kaolinite. Clays Clay minerals 47 (2), 174–180. doi:10.1346/ccmn.1999.0470207
Mitchell, J. K., and Soga, K. (2005). Fundamentals of Soil Behavior, 3. New York: John Wiley & Sons. USA.
Momeni, M., Bayat, M., and Ajalloeian, R. (2022). Laboratory Investigation on the Effects of pH-Induced Changes on Geotechnical Characteristics of Clay Soil. Geomechanics Geoengin. 17 (1), 188–196. doi:10.1080/17486025.2020.1716084
Ochsner, T. E., Horton, R., and Ren, T. (2001). A New Perspective on Soil Thermal Properties. Soil Sci. Soc. Am. J. 65 (6), 1641–1647. doi:10.2136/sssaj2001.1641
Oman, M. S., Lund, N. G., and Intertec, B. (2018). Designing Base And Subbase To Resist Environmental Effects On Pavements (No. MN/RC 2018-06). Minnesota: Dept. of Transportation. Research Services & Library.
O’Neill, K., and Miller, R. D. (1985). Exploration of a Rigid Ice Model of Frost Heave. Water Resour. Res. 21 (3), 281–296. doi:10.1029/wr021i003p00281
Penner, E. (1967). Pressures Developed during the Unidirectional Freezing of Water-Saturated Porous Materials: Experiment and Theory. Phys. Snow Ice Proc. 1 (2), 1401–1414.
Peppin, S. S., and Style, R. W. (2013). The Physics of Frost Heave and Ice-Lens Growth. Vadose Zone J. 12 (1). doi:10.2136/vzj2012.0049
Perera, A. T. D., Nik, V. M., Chen, D., Scartezzini, J. L., and Hong, T. (2020). Quantifying the Impacts of Climate Change and Extreme Climate Events on Energy Systems. Nat. Energy 5 (2), 150–159. doi:10.1038/s41560-020-0558-0
Puppala, A. J. (2021). Performance Evaluation of Infrastructure on Problematic Expansive Soils: Characterization Challenges, Innovative Stabilization Designs, and Monitoring Methods. J. Geotechnical Geoenvironmental Eng. 147 (8)–04021053. doi:10.1061/(asce)gt.1943-5606.0002518
Rahman, R., and Bheemasetti, T. V. (2021) Evaluation Studies on Freezing Point Depression of Stabilized Frost-Susceptible Soil. IFCEE 2021, 203–212. doi:10.1061/9780784483411.020
Rempel, A. W., Wettlaufer, J. S., and Worster, M. G. (2004). Premelting Dynamics in a Continuum Model of Frost Heave. J. Fluid Mech. 498, 227–244. doi:10.1017/s0022112003006761
Rieke, R. D. (1982). The Role of Specific Surface Area and Related Index Properties in the Frost Susceptibility of Soils.
Salomone, L. A., Kovacs, W. D., and Kusuda, T. (1984). Thermal Performance of Fine‐Grained Soils. J. Geotech. Engrg. 110 (3), 359–374. doi:10.1061/(asce)0733-9410(1984)110:3(359)
Schultz, L. G. (1964). Quantitative Interpretation of Mineralogical Composition from X-Ray and Chemical Data for the Pierre Shale. Reston, VA: US Geol. Surv. Prof. Pap., 391-C.
Simpson, H. E. (1960). Geology of the Yankton Area. Reston, VA: South Dakota and Nebraska. No. 328).
So, H. B., and Aylmore, L. A. G. (1993). How Do Sodic Soils Behave - the Effects of Sodicity on Soil Physical Behavior. Soil Res. 31 (6), 761–777. doi:10.1071/sr9930761
Stetler, L. D. (2018). Impacts of Material Engineering Properties on Slope Wash and Stability in Fine-Grained Bedrock Slopes at Fossil-Bearing Sites, Badlands National Park, South Dakota, USA. Geosciences 8 (7), 267. doi:10.3390/geosciences8070267
Studenroth, S., Huber, S. G., Kotte, K., and Schöler, H. F. (2013). Natural Abiotic Formation of Oxalic Acid in Soils: Results from Aromatic Model Compounds and Soil Samples. Environ. Sci. Technol. 47 (3), 1323–1329. doi:10.1021/es304208a
Tabatabai, M. A., and Olson, R. A. (1985). Effect of Acid Rain on Soils. Crit. Rev. Environ. Control 15 (1), 65–110. doi:10.1080/10643388509381727
Tarnawski, V. R., and Wagner, B. (1992). A New Computerized Approach to Estimating the Thermal Properties of Unfrozen Soils. Can. Geotech. J. 29 (4), 714–720. doi:10.1139/t92-079
Tebaldi, G., Orazi, M., and Orazi, U. S. (2016). Effect of Freeze—Thaw Cycles on Mechanical Behavior of Lime-Stabilized Soil. J. Mater. Civ. Eng. 28 (6), 06016002. doi:10.1061/(asce)mt.1943-5533.0001509
Tokimatsu, K., and Yoshimi, Y. (1983). Empirical Correlation of Soil Liquefaction Based on SPT N-Value and Fines Content. Soils Found. 23 (4), 56–74. doi:10.3208/sandf1972.23.4_56
Venäläinen, A., Tuomenvirta, H., Heikinheimo, M., Kellomäki, S., Peltola, H., Strandman, H., et al. (2001). Impact of Climate Change on Soil Frost under Snow Cover in a Forested Landscape. Clim. Res. 17, 63–72. doi:10.3354/cr017063
Wang, Y. H., and Siu, W. K. (2006). Structure Characteristics and Mechanical Properties of Kaolinite Soils. I. Surface Charges and Structural Characterizations. Can. Geotech. J. 43 (6), 587–600. doi:10.1139/t06-026
Yan, F., Schubert, S., and Mengel, K. (1996). Soil pH Increase Due to Biological Decarboxylation of Organic Anions. Soil Biol. Biochem. 28 (4-5), 617–624. doi:10.1016/0038-0717(95)00180-8
Zhang, C., and Lu, N. (2021). Soil Sorptive Potential–Based Paradigm for Soil Freezing Curves. J. Geotechnical Geoenvironmental Eng. 147 (9), 04021086. doi:10.1061/(asce)gt.1943-5606.0002597
Zhang, M., Zhang, X., Lu, J., Pei, W., and Wang, C. (2019). Analysis of Volumetric Unfrozen Water Contents in Freezing Soils. Exp. Heat. Transf. 32 (5), 426–438. doi:10.1080/08916152.2018.1535528
Zhang, S., Sheng, D., Zhao, G., Niu, F., and He, Z. (2016a). Analysis of Frost Heave Mechanisms in a High-Speed Railway Embankment. Can. Geotech. J. 53, 1–10. doi:10.1139/cgj-2014-0456
Zhang, S., Teng, J., He, Z., and Sheng, D. (2016b). Importance of Vapor Flow in Unsaturated Freezing Soil: a Numerical Study. Cold Regions Sci. Technol. 126, 1–9. doi:10.1016/j.coldregions.2016.02.011
Zhang, T., Barry, R. G., Knowles, K., Ling, F., and Armstrong, R. L. (2003). “Distribution of Seasonally and Perennially Frozen Ground in the Northern Hemisphere,” in Proc. 8th Int. Conf. on Permafrost.
Zhang, Y., and Michalowski, R. L. (2015). Thermal-hydro-mechanical Analysis of Frost Heave and Thaw Settlement. J. geotechnical geoenvironmental Eng. 141 (7), 04015027. doi:10.1061/(asce)gt.1943-5606.0001305
Keywords: ice-water, thermal hysteresis, plastic, and non-plastic silts, pH, moisture content
Citation: Bheemasetti TV, Tohm C and Lingwall BN (2022) Ice-Water Phase Change Studies in Plastic and Non-Plastic Silts. Front. Built Environ. 8:943614. doi: 10.3389/fbuil.2022.943614
Received: 13 May 2022; Accepted: 22 June 2022;
Published: 22 July 2022.
Edited by:
Jie Huang, University of Texas at San Antonio, United StatesReviewed by:
SeonHong Na, McMaster University, CanadaCopyright © 2022 Bheemasetti, Tohm and Lingwall. This is an open-access article distributed under the terms of the Creative Commons Attribution License (CC BY). The use, distribution or reproduction in other forums is permitted, provided the original author(s) and the copyright owner(s) are credited and that the original publication in this journal is cited, in accordance with accepted academic practice. No use, distribution or reproduction is permitted which does not comply with these terms.
*Correspondence: Tejo V. Bheemasetti, dGVqby5iaGVlbWFzZXR0aUBzZHNtdC5lZHU=
Disclaimer: All claims expressed in this article are solely those of the authors and do not necessarily represent those of their affiliated organizations, or those of the publisher, the editors and the reviewers. Any product that may be evaluated in this article or claim that may be made by its manufacturer is not guaranteed or endorsed by the publisher.
Research integrity at Frontiers
Learn more about the work of our research integrity team to safeguard the quality of each article we publish.