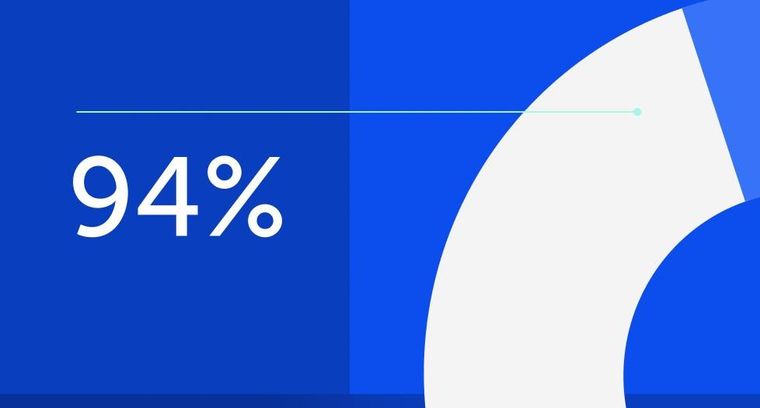
94% of researchers rate our articles as excellent or good
Learn more about the work of our research integrity team to safeguard the quality of each article we publish.
Find out more
ORIGINAL RESEARCH article
Front. Built Environ., 04 July 2022
Sec. Sustainable Design and Construction
Volume 8 - 2022 | https://doi.org/10.3389/fbuil.2022.914227
Ground Heat Exchangers (GHEs), buried in the ground either horizontally or vertically (in a borehole), are coupled with a heat pump to form a Ground Source Heat Pump (GSHP) system, which is a type of Renewable Energy System that exploits geothermal energy for space heating and cooling. GSHP systems are proposed as an alternative to conventional Air Source Heat Pumps (ASHPs) as they exhibit a higher efficiency. In this study, this difference in efficiency is tested in order to determine how the systems perform in terms of environmental impact. Three types of GSHP systems (with different GHE configuration), each compared to ASHPs, undergo a Life Cycle Analysis using the ReCiPe method from both mid-point and end-point perspectives. The heating and cooling loads required for a single residential building of area 220 m2, with nearly Zero Energy Building technical characteristics, is used as a Functional Unit, for seven cases (locations/countries) from South to North Europe. Additionally, a Simple Payback Period method is employed to investigate the CO2 payback time for the GSHPs. It is concluded that the use of GSHP systems in residential buildings, even with nZEB (nearly Zero Energy Buildings) characteristics of low heating/cooling demand, can be a more environmentally friendly solution than that of an ASHP system, depending on the factors affecting the system, namely the ground thermal characteristics, the heating/cooling demand, the heating/cooling peak loads and electricity mix.
Renewable Energy Systems (RES) have been recruited against the use of fossil fuels in order to achieve a reduction of the Global warming effect. Geothermal Energy, a form of renewable energy, exploits the Earth’s heat and finds applications through the use of Ground Source Heat Pump (GSHP) systems.
The GSHP systems extract/reject heat from/to the ground when the HP is coupled with Ground Heat Exchangers (GHEs). GHEs essentially consist of a network of underground tubes with a circulating refrigerant fluid; heat is exchanged between the circulating fluid and the ground. GHEs come in different types and configurations and are designed according to the available land area, the ground thermal characteristics, and the building’s heating and cooling loads. They can be classified into two main categories, based on source and orientation. GHEs are either of open or close type and can be of vertical or horizontal orientation. The conventional types for residential use are the vertical closed systems (Christodoulides et al., 2020), mainly due to the small land area required, and they may be of single or multiple U-tubes, coaxial, spiral or complex configurations.
In recent years, GSHP systems have attracted further attention from governments, especially in the European Union (EU), due to the EU energy-related targets set. Specifically, carbon dioxide (CO2) emissions should be reduced by 80%–95% by 2050 compared to the 1990 levels. Starting from 2020, a milestone has been set every 10 years. A clear trend toward CO2 reduction can be seen from the available data on space heating and cooling in the EU, where 51% of the final energy consumption is responsible for heating and cooling, of which space heating and cooling has a share of 69% and water heating 14% (Fleiter et al., 2016). Furthermore, in northern countries, such as United Kingdom, where heating is required for most months of the year, space heating and domestic hot water account for 78% of the domestic energy consumption and 40% of CO2 emissions (Le et al., 2019). HPs, and in particular Air Source Heat Pumps (ASHPs), have been recruited as a promising technology to reduce CO2 emissions in the EU, mainly replacing natural gas boilers. ASHPs dominate the market toward a building carbon footprint reduction due to their higher coefficient of performance (COP) compared to gas boilers. Although GSHPs have an even higher COP than conventional ASHP systems, they require extra materials/products and processes, as well as available land for GHE installation, which can be confined to a dense urban environment.
In an effort to promote the GSHP systems, some EU countries have provided financial support/initiatives, but with restrictions on technical and licensing standards (Tsagarakis et al., 2020). Such technical and licensing restrictions are set for the benefit of the homeowner; for example, in some cases it can be guaranteed that there will be no interaction with a neighboring system or, when there is an underground aquifer, there will be an interference of the systems downstream. Furthermore, the countries’ initiatives do not only aim at complying with EU guidelines, but also to meet the requirements of owners/developers/buyers in relation to environmental sensitivities. In the not so distant past, the selection of HVAC (Heating, Ventilation and Air Conditioning) systems was solely based on the capital cost (Alanne et al., 2007). Nowadays, in the age of renewables, the selection criteria may also include CO2 reduction and the environmental impact of the system. Decision analyses based on multiple criteria for residential buildings have been investigated by several researches (Seddiki and Bennadji, 2019; D'Agostino et al., 2019; Babatunde et al., 2019), with the buildings’ HVAC systems playing an important role in the overall environmental impact of the buildings. Also, decision making tools have recently been developed for buildings’ RES in order to reduce the energy requirements, while maintaining a cost- and environmentally-effective solution (Hong et al., 2014; Petrillo et al., 2016; Karunathilake et al., 2019).
It should be noted that a RES does not automatically become an environmentally friendly alternative to conventional systems. To this end, Life Cycle Analysis (LCA) and Life Cycle Impact Assessment (LCIA) constitute a tool for comparison of such systems in relation to their environmental friendliness. Previous studies have also raised the aforementioned concern in the case of promoting GSHP systems versus ASHP systems. A general approach for the LCA estimation of the systems could be to evaluate the CO2 savings based on an overall COP difference between the systems (Bayer et al., 2012), while the acidification and eutrophication potential could also be taken into account (Huang and Mauerhofer, 2016). Greening and Azapagic (Greening and Azapagic, 2012), for example, compared the impact of three different HP systems, namely ASHPs, GSHPs and Water Source Heat Pump, and a gas boiler, for several impact categories. The gas boiler provided a lower environmental impact than all HPs, while the ASHP provided the highest impact among the HPs. Another noteworthy finding was that for GSHPs the operation process was 84% of the overall impact. Blum et al. (2010), investigated the CO2 savings when using a GSHP system instead of conventional electric heating systems. The authors used a steady COP value of 4, and an operation time of 2,000 h annually. They hence demonstrated an average 1,800–4,000 kg of CO2 saving per year per unit installed GSHP system. This large variation however is due to the electricity mix. Also, Marinelli et al. (2019), though the use of a general and critical review of the systems, reported a higher environmental impact of the ASHP systems, over the GSHP systems, due to their higher energy requirement in the operation process.
Other recent relevant findings were presented by Sevindik et al. (2021) for a comparative environmental assessment of ASHP, GSHP and gas boilers in the United Kingdom. Similar results were produced by Greening and Azapagic (Greening and Azapagic, 2012), with the exception of reporting lower emissions due to more renewable energy sources used in the electricity mix during the last decade. The gas boilers were reported to produce the lowest environmental impact but not in the category of climate change, while regarding HPs, GSHPs had a lower impact than ASHPs. Having used a FU of one kWh however, the above studies did not capture the complexity of the GSHPs, where the GHEs are designed according to the building’s heating and cooling loads. A similar study, by Blom et al. (2010), compared ASHPs and gas boilers in the Netherlands through a LCA. The authors found that the use of gas boilers produced a lower environmental impact compared to ASHPs. On the other hand, Koroneos and Nanaki (Koroneos and Nanaki, 2017) reported that raw materials produced the highest impact during the use of GSHPs. The Eco-indicator95 impact category was selected by the authors with the commercially available SimaPro software for a case study of a town hall in Greece with a life cycle of 25 years. Their study, however, was focused on the production of one kWh of thermal energy, which was used as a Functional Unit (FU). The electricity mix was reported to play an important role in the environmental impact of the GSHP systems in the study of Smith et al. (2021). The electricity mix may be different from study to study and from country to country, and therefore a comparison among different countries may be helpful in giving a deeper insight on the impact of GSHP systems. Finally, Genchi et al. (2002) used a simple payback method to address the CO2 payback period (PBP) of a GSHP system at a regional scale. A PBP of 1.7 years was reported by the authors, with the replacement of ASHP systems by GSHP systems yielding a CO2 emissions reduction of 54%.
The use of water solution with different antifreeze additives in the GHE circulating fluid provides a different environmental impact, depending on the case. This allows the circulating fluid and the system to operate at temperatures below pure water freeze conditions (0°C), leading to the reduction of the overall GHE length. However, this solution could come at a high cost, when there is a leakage in the closed system and the groundwater is contaminated. Johnson (Johnson, 2011) demonstrated that the refrigerant (circulating fluid) in an ASHP system accounts for approximately 16% of the CO2 emissions by the system. The long term potential for groundwater contamination with different antifreeze solutions, namely propylene glycol (PG), ethylene glycol (EG), and betaine, was examined by Klotzbucher et al. (2007). The authors reported that EG and PG—as they are readily biodegradable in many environments—are not at risk of contamination in the long run. The case is different with the use of betaine, where there is a potentially higher risk for groundwater quality. Such effects were also investigated by Bartolini et al. (2020), where three different antifreeze agents, namely PG, Calcium Chloride (CaCl2) and pure water, were tested. Pure water was clearly the best solution with the use of CaCl220%, leading to reduced emissions compared to PG25%. Using an antifreeze solution could endanger the groundwater in the case of leakage, but also—through its ability to operate at very low temperatures—could potentially lead to the freezing and damaging of the grouting materials of the GHE (Erol and François, 2016; Dalla Santa et al., 2019).
Following the above, and as the literature indicates, one could expect that the use of GSHPs would be of a lower environmental impact compared to ASHPs in most cases. However, as every GSHP system is custom-designed per case and depends on the residential building’s characteristics, the ground thermal properties, climate conditions, and so on, such a comparison does not lead to so obvious results. The present study attempts to fill such a gap, by investigating—for the first time—the environmental impact of GSHP systems versus ASHP systems in residential buildings with nearly Zero Energy Building (nZEB) characteristics (mandatory for new buildings in the EU). The comparison is made for a “typical” three-bedroom dwelling for a household of four in seven different locations (countries) covering the whole of Europe geography-wise, from South to North. The goal is to assess whether three specific different GSHP systems (RES) are environmentally more beneficial than conventional ASHP systems, and to which extent in each country with regard to the EU targets mentioned above.
The methodology followed in this research is given in Section 2, where first a case study, as explained above, is examined in each country in order to size the required GHEs, and secondly LCA characteristics used for assessing the environmental impact of all GSHP systems are described. The results of the LCA are then presented in detail in Section 3 for both a mid-point and an end-point perspectives. Conclusions are discussed in Section 4.
An important step for the LCA and the estimation of the LCIA is the relevant data collection. The choice of a heating and cooling system for any dwelling is based on the estimated building’s loads and peak loads. The data (heating and cooling loads) for seven cases (European cities in different countries), namely Lefkosia–Cyprus (CY), Seville–Spain (ES), Bologna–Italy (IT), Lisbon–Portugal (PT), Belgrade–Serbia (SRB), Berlin-Germany (DE), Stockholm–Sweden (SE), are provided in the literature (Aresti et al., 2020; Bartolini et al., 2020). Specifically, the thermal loads from several case studies in six different countries were used to perform GHE sizing from an economic and environmental point of view (Bartolini et al., 2020), while analogous data for Cyprus were used for a study of foundations as GHEs (Aresti et al., 2020). The residential heating and cooling loads data, as well as the ground thermal characteristics for each area, are used as input to GLD (Ground Loop Design, Thermal Dynamics Inc., MN, United States). GLD is a commercially available software package for GSHP systems design professionals. In all seven cases/locations, a high insulated residential building (detached house) for a household of four is considered, with an area of 220 m2, a U-value (thermal transmittance) of less than 0.4 W m2 K−1 for the external wall and the ceiling, and less than 2.25 W m−2 K−1 for the doors and windows. Note that these technical characteristics fall under the nZEB characteristics, according to Derivative 366/2014 (Ministry CIET, 2017). The heating and cooling loads per country as well as the relevant COPs are shown in Figure 1. The ASHP COPs are assumed values based on previous ambient temperature and HP performance at entering fluid temperature (Christodoulides et al., 2019), whereas the GSHP COP values are elaborated values from GLD. The COP values depend on the HP characteristics and capacity; therefore, the HPs were sized according to the peak load and varied for each case. The capacity of the HPs to satisfy the peak heating or cooling loads, are 9, 10.5, 7.8, 9, 11.3, 12.4, and 14.7 kW for the cases of CY, ES, PT, IT, SRB, DE, and SE respectively.
As previously mentioned, GSHPs consisting of vertical closed-loop GHEs are the conventional type in residential areas (Aresti et al., 2018). Here, three different GHE configurations are considered, namely single U-tube, double U-tube and coaxial (all borehole GHEs). Depending on the application, there may be multiple numbers of boreholes to reach the necessary depth of the GHE required to meet the heating and cooling demands. For the current application, the borehole number was set to 3 (i.e., there were three boreholes), for all cases, with a separation (distance) of 4 m between each borehole, a 0.2 m diameter borehole and a 32 mm-outer-diameter P100 SDR11-OD pipe applied. The ground temperatures were 22.7°C, 21.5°C, 19.5°C, 16.0°C, 15.0°C, 12.5°C, and 9.0°C, for CY, ES, PT, IT, SRB, DE, and SE respectively. The selected HP varied from country to country due to the difference in the heating/cooling demand, but is kept the same for each country for all configurations. A major difference in system design between southern and northern Europe is the use of a higher percentage of antifreeze solution as circulating fluid in the GHEs. The further north in Europe, the lower the ambient and ground temperatures, and therefore an increased antifreeze solution is required.
An environmental impact assessment of the systems under investigation, which is essentially an estimation of the impact on the examined processes, products or services, is performed using Life Cycle Analysis (LCA) in accordance with the ISO 14040 (ISO 14040, 2006) and ISO 14044 (ISO 14044, 2006). LCA consists of: 1) the goal and scope definition, 2) the life cycle inventory (LCI) analysis, 3) the LCIA, and 4) the interpretation of results. The chosen LCA methodology was based on previous studies, such as (ISO 14040, 2006; ISO 14044, 2006; Aresti et al., 2021; Sutman et al., 2020; Ren et al., 2018; Bartolozzi et al., 2017; Kljajić et al., 2020; Goedkoop et al., 2008).
The scope of the LCA is set to be the examination of the overall environmental load of different vertical GHE types for a detached residential building, and the evaluation of the different impacts in relation to the systems’ installation location in Europe. The overall goal is to identify whether a GSHP system is environmentally beneficial as a heating and cooling system across Europe and how geographic location (countries from southern to northern Europe) affects this impact. Another question to answer is whether the change from an ASHP (conventional) system to a GSHP (RES) system would be beneficial to EU countries toward their goal to reach the EU climate targets.
The system boundaries for the GSHP systems with different types of GHEs are presented in Figure 2. A cradle-to-grave approach impact has been adopted with the boundaries including the extraction and process of raw materials, transportation, installation and operation related only to the GHEs and not the HPs. The HPs are assumed to have the same material volume and processes for all cases, and were therefore neglected from the study. The LCA flow of the systems starts with the extraction of raw material related to GHEs, continues with the production and processes of the products, and finishes with the installation and operation of the system. The distribution and transportation of the products to and from the construction site are not taken into account because of their negligible impact, as observed in previous studies (Sutman et al., 2020; Aresti et al., 2021). The operation stage is considered for the lifetime of the system. In this study the lifetime of the HP is set at 15 years (Christodoulides et al., 2019; Aresti et al., 2021) (note that for the GHE, it can be higher).
A key step in LCA estimation is the classification of the functional unit (FU), which serves as a reference where inputs and outputs of the systems are measured upon. In this study, FU is represented by the energy required to satisfy the yearly demand (for heating and cooling) of the building under consideration. Note that this demand varies by country. A similar choice of FU has already been observed in the literature (Bartolozzi et al., 2017; Ren et al., 2018; Sutman et al., 2020; Aresti et al., 2021). It should be emphasized here that, if a fixed energy was selected (for example one kWh), the difference between the cases would only lie in the extra material and processes required for drilling the boreholes.
The open source and user friendly OpenLCA software (http://www.openlca.org/) was selected for the sustainability assessment and LCA. OpenLCA, being a freeware software, does not contain any databases or methods. Free or commercial databases and methods can be imported. Such a database is the Ecoinvent 3.6 database (Steubing et al., 2016; Wernet et al., 2016), which is used in this study (with educational license), along with the Cut-off system model (Wernet et al., 2016).
The GSHP systems, with different GHEs and characteristics, are connected to input and output flows based on the Ecoinvent 3.6 database, while the background LCI data are also obtained from the Ecoinvent 3.6 database. The LCI with the input and output (emissions) processes of the system is described in Table 1. The LCI table is divided into three stages, namely the materials production/manufacturing, the installation of the system and the operation. The transportation of the system has not been included, as it was found to have a neglected effect in previous studies (Aresti et al., 2021). The data shown in Table 1, but also in Tables 2 and 3, are all linked to the FU for each different case.
TABLE 3. Total borehole length with different GHE configurations and different rate of antifreeze solution at each city, estimated using the GLD software.
The operation of the system depends on the annual heating energy demand and the performance of the system (related to operation process, see Table 1). The electricity demand of each system, estimated using the COP of each system, are presented in Table 2, where the COPs for the different GSHP systems were estimated using the GLD software and are presented in Figure 1. Based on the heating and cooling loads, the percentage difference (saving) in electricity demand between using a GSHP instead of an ASHP system, is 32%–39% for southern European countries and 41%–50% for central and northern European countries. The electricity demand was assumed to be contant for the lifetime of the system, with similar assumptions performed in literature (Sutman et al., 2020; Aresti et al., 2021).
Several methods are available for use with the LCIA, depending on the scope of the problem under consideration. Such methods can be found in the literature with both mid-point and end-point impact perspectives (Dreyer et al., 2003; Monteiro and Freire, 2012; Weidema, 2015). Examples of available LCIA methods for the Ecoinvent 3.6 dataset are CML2001, Cumulative Energy Demand, Eco-indicator 99, Eco-Scarcity 2006, ILCD 2011, ReCiPe and the TRACI 2.1 (Acero et al., 2016).
In particular, CML (Guinée et al., 2001) and Eco-indicator (Goedkoop and Spriensma, 1999) are two widely accepted impact methods, with different approaches, the former being a problem-oriented method with a mid-point perspective and the latter being a damage-oriented method with an end-point perceptive. In mid-point impact perspective methods, one has to interpret each impact category (from dozens) through different indicators (such as CO2 for climate change), while in end-point perspective methods, one has to simply use weighted results of only three to four damage categories, which arise from a set of impact categories converted into a point impact Eco-indicator system (Dong and Ng, 2014). In such a point system, every 1,000 points (Pt) represent the yearly environmental load of an average European citizen. Although the end-point perspective may seem like an ideal approach, it exhibits higher uncertainties compared to the mid-point method.
ReCiPe, on the other hand, builds upon the two methods and provides a harmonized mid-point and end-point consistent methodology. A more detailed analysis, the areas of protection, as well as the methodology used for the ReCiPe impact method can be found in (Goedkoop et al., 2008). In the current study the selected LCIA method is the ReCiPe methodology with the Hierarchist (H) perspective, which is a consensus model with regard to time frame and plausibility of impact mechanisms (Goedkoop et al., 2008; Foteinis et al., 2018). The impact categories were selected according to the goal and scope of this study, which is to identify the effect of a change from ASHP systems to GSHP systems throughout Europe and how it would benefit the EU climate targets. Therefore, the main impact categories of the study, associated with the climate change, are the Global Warming Potential (GWP) for the midpoint perspective, and the ecosystem quality for the endpoint perspective. Evaluation of the human health and resources was also selected for the endpoint perspective for comparison reasons.
Before proceeding to the LCA, the different GHE configurations for each city/country were estimated using the GLD software and are presented in Table 3. The southern Mediterranean countries, which have higher average temperatures, require a lower percentage antifreeze solution than the central and northern European countries. Additionally, based on the FU, it can be seen that the Mediterranean countries exhibit lower heating and cooling demand (see Table 2), but not necessarily shallower boreholes, due to the fact that the peak loads may be higher than northern countries because of the hot summers in the Mediterranean countries. The calculated values are then incorporated into the openLCA software for the estimation of the systems’ LCA and LCIA.
Generally, one of the most important impact categories is the GWP, which is associated with climate change and the greenhouse effect, having an impact on air quality, ecosystems and human health. GWP can arise during fuel combustion and industrial processes, and therefore can occur at all stages, namely the manufacturing, installation and operation of the GSHP system. GWP has potential mid-point impact effects such as the increased radiative forcing. Moreover, from an end-point perspective, GWP has major effects such as sea level increase, wind and ocean currents change, and increased extreme weather frequency.
The investigated GWP impact of manufacturing (material processing) the different types of GHEs in different European locations is presented in Figure 3, where 100% is represented by the SE coaxial configuration at 2683 kg CO2-Eq per FU. The results, as expected, demonstrate that with the increased borehole length (see Table 3), there is an increased impact, regardless of geographical location (i.e., south or north), as explained above. Notably, among all configurations, the coaxial GHE produces the highest environmental impact in all cases, despite requiring the lowest total borehole length, as it requires higher pipe volume compared to the other configurations. These results are in line with previous studies (Aresti et al., 2021).
FIGURE 3. GWP impact for the manufacturing process of the boreholes (GHEs), with the coaxial GHE in SE representing 2,683 kg CO2-Eq. per FU.
Having noted that the highest manufacturing impact was produced by the coaxial configuration in all cases (countries) and with the highest being in SE, the overall impact comparison between the differences of GSHP and ASHP systems is investigated and plotted in Figure 4. It is evident that for all cases the ASHP system produces the highest impact, except for the case of SE where all types of GSHP systems have a higher GWP impact than ASHP. The ASHP system corresponding to 100% has different values (per FU) for each country, namely 3.12 × 104, 1.09 × 104, 2.05 × 104, 7.63 × 103, 2.49 × 104, 4.38 × 104, and 6.71 × 103 kg CO2-eq for CY, ES, IT, PT, SRB, DE and SE respectively. The GWP impacts for all cases, except SE, are lower by 13%–43%, while for SE higher by 11%–18%. The higher heating loads in the northern countries lead to higher differences in electricity demand between GSHP and ASHP systems, and hence the GWP impact produced was expected to be lower; this is though not the case for SE where a higher impact is observed, owing to the high share of renewables in the electricity mix of that country. The installation and manufacturing processes, with the exception of SE, exhibit lower emissions than the operation process, and this could be justified by the low time required for these processes compared to the operation time required for the lifespan of the system.
Next, the share of each process in the overall system impact is examined. The results for CY and SE can be seen in Figure 5, where the operation process for all cases ranges between 91%–93% and 42%–45% for each country respectively. For the rest of the countries under investigation, the operation process share ranged between 74% and 91% for all cases. Similar behaviors were observed in previous studies, where the operation process was predominant in terms of environmental impact (Bartolozzi et al., 2017; Koroneos and Nanaki, 2017; Sutman et al., 2020; Aresti et al., 2021), due to its longer lifespan. In the northern countries, due to the use of environmentally friendlier ways and RES for electricity generation, the grid electricity mix has lower carbon emissions (Saner et al., 2010; Greening and Azapagic, 2012) than the southern countries, but higher heating demand. In cases where the lifespan of the system is longer than 15 years, the operation process is practically the one that counts for the environmental impact; such cases were reported by Sutman et al. (2020) for 20 years lifespan and Bartolozzi et al. (Bartolozzi et al., 2017) for 50 years lifespan.
FIGURE 5. Percentage share of the different processes for the GWP impact per FU in two different cases/countries, CY (top row) and SE (bottom row), for single U-tube GHE (first column), double U-tube GHE (middle column), and coaxial GHE configurations (last column).
By setting the ASHP performance (COP) shown in Figure 1 as benchmark, an analysis of the effect of the GSHP to ASHP ratio on the environmental impacts can be performed by increasing the ASHP COP. This scenario is presented in Figure 6 for the case of the GWP impact per FU (recall Figure 4), where all cases/countries are compared with increments of 10%, 20% and 30% in the ASHP COP, with one representing the ASHP system environmental impact for each country/case for a double U-tube GHE. It can be observed that, even with a 30% increase in the ASHP performance (i.e., a reduction in electricity demand), yielding a lower difference in the operation process, the GSHP systems in most cases continue to produce a lower environmental impact. In particular, for the cases of CY, IT, SRB and DE, the GSHP systems are still superior in the range of 7%–26% even for the “worst”-case scenario (30% increase in ASHP performance).
FIGURE 6. Percentage ratio of GSHP systems to ASHP for various increments in ASHP efficiency (COP), for the GWP impact per FU.
Following the results of the mid-point impact (shown in Figure 4), the end-point-impact was also examined using the ReCiPe method with the Hierarchist perceptive for the ecosystem quality impact category. As before, the ASHP system, corresponding to 100%, has different values (per FU) for each country, namely 5.95 × 102, 3.36 × 102, 4.45 × 102, 1.64 × 102, 5.52 × 102, 8.79 × 102, and 4.62 × 102 points for CY, ES, IT, PT, SRB, DE, and SE respectively. The results (shown for all configurations in Figure 7) follow the same trend as the mid-point impact, and constitute a verification that the ASHP systems exhibits a higher impact than all GSHP systems in all countries. The impact reduction between the ASHP system and the GSHP system for all cases, with the exception of SE, ranges between 8% and 43%. Low impact differences are observed between GHE configurations, with only 0.5%–3.5% difference noted between the single U-tube and coaxial configurations. The operation process plays the most important role in the environmental impact showing the highest percentage, ranging from 52% to 68%.
FIGURE 7. Percentage ratio of the different types of GSHP systems to the ASHP per country, using ReCiPe end-point method for the ecosystem quality impact per FU.
By further investigating the three damage weighted impact categories, namely the ecosystem quality, human health and resources, once more all GSHP systems outperform the ASHP system in all cases, except for SE, as shown in Figure 8. As before, the operation process is crucial as it exhibits the highest impact in all cases and follows the same pattern observed in Figure 7. Regarding the human health category, the impact reduction between the ASHP system and the GSHP system for all cases, including SE, ranges between 7% and 43%. Finally, regarding the resources category, the impact reduction between the ASHP system and the GSHP system for all cases, with the exception of SE, ranges between 2% and 39%, and is always smaller than the ecosystem quality respective impact reduction. As for SE, it seems that the high impact in this category (a 26% increase compared to ASHP) is due to the high share of renewables in the electricity mix.
FIGURE 8. Percentage ratio of the double U-tube GSHP system to the ASHP per country, using ReCiPe end-point method for all three damage categories per FU.
All the above agree with the findings of the mid-point perspective, where all GSHPs are environmentally beneficial compared to ASHPs, for all cases except SE.
Clearly, the operation process, which provides the highest impact, could be decreased with the use of other RES, such as Photovoltaic (PV) systems, in the residential building. In such a case, GSHP systems may not be environmentally friendlier solutions than ASHP systems, as there will be very low emissions during the operation process; hence ASHPs will be a preferable choice. On the other hand, ASHP systems might not be sufficient in areas of very low ambient temperatures like the case of SE, and a secondary heating system (such as gas boiler, etc.) would be required. This would raise even further the environmental impact, thus making the GSHP systems a clearly environmentally friendlier system.
Furthermore, performing a simple payback period (PBP) method, one can investigate the CO2 PBP when using a GSHP system instead of an ASHP system. A simple method for a comparable evaluation, similar to the simple PBP used for economic evaluation, is applied, where the years required for the ASHP system to produce higher CO2-eq emissions than the GSHP system, are calculated. The PBP in years can be described by the following equation:
where k is the number of years,
where
The COP values were assumed to be constant in the 15 year lifetime of the systems, although in some cases for the GSHP systems, when unbalanced loads are observed, the ground temperature could rise, yielding a COP difference [in some cases reported at +1.76% (Marinelli et al., 2020)]. The effect of change in COP difference on PBP is studied for various increments in the COP values of the two compared systems.
Figure 9 presents the estimated CO2 PBP based on the COP values of Figure 1 for the double U-tube configuration. The PBP for all cases, except SE, is within an acceptable time frame, always below the lifetime of the system of 15 years. Even for SE, the PBP of 20.4 years is not prohibitive of installing a GSHP system, as the GHE have life expectancies of 50+ years; hence by replacing the HP, the system would extend its lifetime much further than 15 years. Once more there is no specific trend for southern or northern countries, as the factors affecting the whole analysis, in addition to the ground thermal characteristics, are the heating/cooling demands, the heating/cooling peak loads and electricity mix. Of note is the particularly low PBPs for the cases of DE, CY, SRB and IT.
FIGURE 9. CO2 payback period per country for the double U-tube GSHP system (compared to ASHP), with baseline COPs as presented in Figure 1, for the GWP impact per FU.
Figure 10 presents a kind of “sensitivity” analysis of the PBP baseline presented in Figure 9 for all countries except SE, where incremental factors of 10% are applied for the ASHP COP values for fixed GSHP COP values. For increased COPs up to 10%, all GSHP cases exhibit a lower CO2 PBP than the specified lifetime (15 years) of the system. Moreover, for the cases of DE, SRB and CY, all GSHP cases exhibit a lower CO2 PBP than the specified lifetime (15 years) of the system even for increased COPs up to 40%.
FIGURE 10. CO2 payback period per country for the double U-tube GSHP system (compared to ASHP), with various increments added in ASHP efficiency (COP) from values presented in Figure 1, for the GWP impact per FU.
A different approach is followed in Figure 11, where the CO2 PBP per country is presented for percent differences between GSHP and ASHP, using the GSHP COP values of Figure 1 as baseline. For example, for the GSHP heating and cooling COP values of CY, namely 5.1 and 4.4, and ASHP values smaller by 10%, i.e., 4.59 and 3.96, respectively, one observes a PBP of 13 years. More specifically, the GSHP cases of CY, DE, SRB and IT exhibit a lower PBP than the specified lifetime (15 years) of the system for percent differences of 15% or more. Moreover, the GSHP cases of ES and PT exhibit a lower PBP than the specified lifetime of the system for percent differences of 30% or more, while a 60% percent difference is needed for SE. For comparison reasons, with a factor of 30%, which is reported in the literature (Aresti et al., 2021), the PBP time can be reduced to a most desirable 3.4 years in the best case (CY), not far from 1.7 years reported by (Genchi et al., 2002).
FIGURE 11. CO2 payback period per country for the double U-tube GSHP system (compared to ASHP), with various drops in ASHP efficiency (COP), for the GWP impact per FU.
During this research the environmental impact of different GSHP systems in different European countries was investigated. Specifically, GSHP and ASHP systems were subjected to a comparison to investigate whether the GSHP systems would be an environmentally beneficial alternative to ASHP systems in terms of their performance, depending on their geo-location.
Initially, data from several case studies in seven European countries (CY, ES, IT, PT, SRB, DE and SE) were incorporated into the GLD software, where the depth and the performance of each GSHP/GHE system (for single U-tube, double U-tube, and coaxial configurations) were estimated. The obtained data were then used as input for the LCA estimation of the different systems using the openLCA software with the Ecoinvent 3.6 database and methods. The cut-off approach with the ReCiPe methodology and the GWP impact category were selected for the LCIA calculation. The selected FU was the annual heating and cooling loads required to satisfy a three-bedroom detached residential building with an area of 220 m2.
The results from a mid-point impact perspective, indicated that the ASHP system exhibited the highest impact among all cases, except SE, and that GSHP systems could be an environmentally beneficial alternative. The GSHP systems of six out of the seven investigated European countries (namely, CY, ES, IT, PT, SRB, DE) outperformed (having a lower GWP impact) the ASHP system by 13%–43%, while the GSHP systems in SE were outperformed by the ASHP system by 11%–18%. The further north the location of the case is, the higher heating load is required, therefore the higher difference in performance between ASHP and GSHP, and the higher is the difference in electricity demand. However, the results are affected by the electricity mix, which seems to be a factor out-shadowing the geographical location. The operation process was demonstrated to have the highest impact, ranging between 42% and 93%, with the environmental impact highly depending on the electricity mix composure, where the higher the renewable energy share in the electricity mix the lower the operation percentage impact. Similar results obtained using an end-point impact perspective confirm the results of the mid-point impact perspective.
Also, a simple PBP method was employed to investigate the CO2 PBP period of the GSHP systems, compared to ASHP, for different ASHP COPs. It turns out that the PBP varies between 2.0 and 20.4 years, with the GSHP systems of four countries (namely, DE, CY, SRB, IT) yielding very low PBP of less than 4 years. Also, the GSHP systems of PT and ES yield acceptable PBPs considerably lower than the 15-year specified lifetime of the system. Furthermore, two “sensitivity” analysis scenarios were investigated, one where the ASHP COP was increased by increments of 10%, and one where the difference in COP between GSHP and ASHP was increased by increments of 10%. It turns out that for the realistic scenarios considered, all GSHPs can exhibit a lower CO2 PBP than the specified lifetime of the system, according to the chosen increment. In particular, for the first scenario, the “best-case” is exhibited by DE with a PBP period of less than 5 years even for a 40% increase of the ASHP COP. For the second scenario, the “best-case” is exhibited by CY with a PBP period of less than 15 years even for a 10% COP difference between GSHPs and ASHPs.
Concluding, the use of GSHP systems in residential buildings, even the ones with nZEB characteristics of low heating/cooling demand, can be an environmentally friendlier solution than that of an ASHP system. However, this depends on the factors affecting the whole analysis, namely the ground thermal characteristics, the heating/cooling demand, the heating/cooling peak loads and electricity mix.
The study presented in this paper has addressed new grounds in the usefulness and practical significance of implementing GSHP systems in relation to environmental benefits. Consequently, one could think of several future directions that such an analysis could follow. One such direction is the adoption of more locations/cases, based on data availability. That would require collecting data such as thermal loads of case studies, ground thermal characteristics of the case area, etc., in various countries. Another future direction could be the examination of how potential novel GSHP designs would affect the results of a similar study.
Equally important is the dynamic distribution of the electricity mix in the production and supply. With the ongoing increase in the mandated adoption of RES by EU countries, the electricity mix has been varying continuously and has become a particularly important factor in LCA.
The raw data supporting the conclusion of this article will be made available by the authors, without undue reservation.
Conceptualization, LA and PC; methodology, LA; software, LA and AS; validation, LA; formal analysis, LA, PC, and GF; investigation, LA; writing–original draft preparation, LA; writing–review and editing, LA, GF, and PC; supervision, PC. All authors have read and agreed to the published version of the manuscript.
The authors declare that the research was conducted in the absence of any commercial or financial relationships that could be construed as a potential conflict of interest.
All claims expressed in this article are solely those of the authors and do not necessarily represent those of their affiliated organizations, or those of the publisher, the editors and the reviewers. Any product that may be evaluated in this article, or claim that may be made by its manufacturer, is not guaranteed or endorsed by the publisher.
ASHP, Air Source Heat Pump; CO2,Carbon Dioxide; COP, Coefficient of Performance; CY, Cyprus; DE, Germany; EG, Ethylene Glycol; EQ, Ecosystem Quality; ES, Spain; EU, European Union; FU, Functional Unit; GHE, Ground Heat Exchanger; GSHP, Ground Source Heat Pump; GWP, Global Warming Potential; IT, Italy; LCA, Life Cycle Analysis; LCI, Life Cycle Inventory; LCIA, Life Cycle Impact Assesment; nZEB, nearly Zero Energy Buildings; PBP, Payback Period; PG, Propylene Glycol; PT, Portugal; RES, Renewable Energy Systems; SE, Sweden; SRB, Serbia.
Acero, A. P., Rodríguez, C., and Ciroth, A. (2016). LCIA Methods: Impact Assessment Methods in Life Cycle Assessment and Their Impact Categories. Berlin, DE: GreenDelta GmbH.
Alanne, K., Salo, A., Saari, A., and Gustafsson, S.-I. (2007). Multi-criteria Evaluation of Residential Energy Supply Systems. Energy Build. 39, 1218–1226. doi:10.1016/j.enbuild.2007.01.009
Aresti, L., Christodoulides, P., and Florides, G. (2018). A Review of the Design Aspects of Ground Heat Exchangers. Renew. Sustain. Energy Rev. 92, 757–773. doi:10.1016/j.rser.2018.04.053
Aresti, L., Christodoulides, P., and Florides, G. A. (2021). An Investigation on the Environmental Impact of Various Ground Heat Exchangers Configurations. Renew. Energy 171, 592–605. doi:10.1016/j.renene.2021.02.120
Aresti, L., Christodoulides, P., Panayiotou, G. P., and Florides, G. (2020). Residential Buildings' Foundations as a Ground Heat Exchanger and Comparison Among Different Types in a Moderate Climate Country. Energies 13, 6287. doi:10.3390/en13236287
Babatunde, O. M., Munda, J. L., and Hamam, Y. (2019). Selection of a Hybrid Renewable Energy Systems for a Low-Income Household. Sustainability 11, 4282–4324. doi:10.3390/su11164282
Bartolini, N., Casasso, A., Bianco, C., and Sethi, R. (2020). Environmental and Economic Impact of the Antifreeze Agents in Geothermal Heat Exchangers. Energies 13, 5653. doi:10.3390/en13215653
Bartolozzi, I., Rizzi, F., and Frey, M. (2017). Are District Heating Systems and Renewable Energy Sources Always an Environmental Win-Win Solution? A Life Cycle Assessment Case Study in Tuscany, Italy. Renew. Sustain. Energy Rev. 80, 408–420. doi:10.1016/j.rser.2017.05.231
Bayer, P., Saner, D., Bolay, S., Rybach, L., and Blum, P. (2012). Greenhouse Gas Emission Savings of Ground Source Heat Pump Systems in Europe: A Review. Renew. Sustain. Energy Rev. 16, 1256–1267. doi:10.1016/j.rser.2011.09.027
Blom, I., Itard, L., and Meijer, A. (2010). LCA-based Environmental Assessment of the Use and Maintenance of Heating and Ventilation Systems in Dutch Dwellings. Build. Environ. 45, 2362–2372. doi:10.1016/J.BUILDENV.2010.04.012
Blum, P., Campillo, G., Münch, W., and Kölbel, T. (2010). CO2 Savings of Ground Source Heat Pump Systems - A Regional Analysis. Renew. Energy 35, 122–127. doi:10.1016/j.renene.2009.03.034
Christodoulides, P., Aresti, L., and Florides, G. (2019). Air-conditioning of a Typical House in Moderate Climates with Ground Source Heat Pumps and Cost Comparison with Air Source Heat Pumps. Appl. Therm. Eng. 158, 113772. doi:10.1016/j.applthermaleng.2019.113772
Christodoulides, P., Vieira, A., Lenart, S., Maranha, J., Vidmar, G., Popov, R., et al. (2020). Reviewing the Modeling Aspects and Practices of Shallow Geothermal Energy Systems. Energies 13, 4273. doi:10.3390/en13164273
D'Agostino, D., Parker, D., and Melià, P. (2019). Environmental and Economic Implications of Energy Efficiency in New Residential Buildings: A Multi-Criteria Selection Approach. Energy Strategy Rev. 26, 100412. doi:10.1016/j.esr.2019.100412
Dalla Santa, G., Farina, Z., Anbergen, H., Rühaak, W., and Galgaro, A. (2019). Relevance of Computing Freeze-Thaw Effects for Borehole Heat Exchanger Modelling: A Comparative Case Study. Geothermics 79, 164–175. doi:10.1016/j.geothermics.2019.02.001
Dong, Y. H., and Ng, S. T. (2014). Comparing the Midpoint and Endpoint Approaches Based on ReCiPe-A Study of Commercial Buildings in Hong Kong. Int. J. Life Cycle Assess. 19, 1409–1423. doi:10.1007/s11367-014-0743-0
Dreyer, L. C., Niemann, A. L., and Hauschild, M. Z. (2003). Comparison of Three Different LCIA Methods: EDIP97, CML2001 and Eco-Indicator 99. Int. J. LCA 8, 191–200. doi:10.1007/bf02978471
Erol, S., and François, B. (2016). Freeze Damage of Grouting Materials for Borehole Heat Exchanger: Experimental and Analytical Evaluations. Geomechanics Energy Environ. 5, 29–41. doi:10.1016/j.gete.2015.12.002
Fleiter, T., Steinbach, J., and Ragwitz, M. (2016). Mapping and Analyses of the Current and Future (2020 - 2030) Heating/cooling Fuel Deployment (Fossil/renewables) - Work Package 1: Final Energy Consumption for the Year 2012. Brussels, Belgium: European Commission
Foteinis, S., Monteagudo, J. M., Durán, A., and Chatzisymeon, E. (2018). Environmental Sustainability of the Solar Photo-Fenton Process for Wastewater Treatment and Pharmaceuticals Mineralization at Semi-industrial Scale. Sci. Total Environ. 612, 605–612. doi:10.1016/j.scitotenv.2017.08.277
Genchi, Y., Kikegawa, Y., and Inaba, A. (2002). CO2 Payback-Time Assessment of a Regional-Scale Heating and Cooling System Using a Ground Source Heat-Pump in a High Energy-Consumption Area in Tokyo. Appl. Energy 71, 147–160. doi:10.1016/S0306-2619(02)00010-7
Goedkoop, M., Heijungs, R., Huijbregts, M., De Schryver, A., Struijs, J., Van Zelm, R., et al. (2008). A Life Cycle Impact Assessment Method Which Comprises Harmonised Category Indicators at the Midpoint and the Endpoint Level. Availableat: http://www.pre-sustainability.com/download/misc/ReCiPe_main_report_final_27-02-2009_web.pdf.
Goedkoop, M., and Spriensma, R.RO, Communicatie (1999). The Eco-Indicator 99: A Damage Oriented Method for Life Cycle Impact Assessment. Netherlands: Ministerie van Volkshuisvesting, Ruimtleijke Ordening en Milieubeheer.
Greening, B., and Azapagic, A. (2012). Domestic Heat Pumps: Life Cycle Environmental Impacts and Potential Implications for the UK. Energy 39, 205–217. doi:10.1016/j.energy.2012.01.028
Hong, T., Koo, C., Kwak, T., and Park, H. S. (2014). An Economic and Environmental Assessment for Selecting the Optimum New Renewable Energy System for Educational Facility. Renew. Sustain. Energy Rev. 29, 286–300. doi:10.1016/J.RSER.2013.08.061
Huang, B., and Mauerhofer, V. (2016). Life Cycle Sustainability Assessment of Ground Source Heat Pump in Shanghai, China. J. Clean. Prod. 119, 207–214. doi:10.1016/j.jclepro.2015.08.048
ISO 14040 (2006). Environmental Management — Life Cycle Assessment — Principles and Framework. Geneva, Switzerland: ISO
ISO 14044 (2006). Environmental Management — Life Cycle Assessment — Requirements and Guidelines. Geneva, Switzerland: ISO
Johnson, E. P. (2011). Air-source Heat Pump Carbon Footprints: HFC Impacts and Comparison to Other Heat Sources. Energy Policy 39, 1369–1381. doi:10.1016/J.ENPOL.2010.12.009
Karunathilake, H., Hewage, K., Brinkerhoff, J., and Sadiq, R. (2019). Optimal Renewable Energy Supply Choices for Net-Zero Ready Buildings: A Life Cycle Thinking Approach under Uncertainty. Energy Build. 201, 70–89. doi:10.1016/j.enbuild.2019.07.030
Kljajić, M. V., Anđelković, A. S., Hasik, V., Munćan, V. M., and Bilec, M. (2020). Shallow Geothermal Energy Integration in District Heating System: An Example from Serbia. Renew. Energy 147, 2791–2800. doi:10.1016/j.renene.2018.11.103
Klotzbücher, T., Kappler, A., Straub, K. L., and Haderlein, S. B. (2007). Biodegradability and Groundwater Pollutant Potential of Organic Anti-freeze Liquids Used in Borehole Heat Exchangers. Geothermics 36, 348–361. doi:10.1016/j.geothermics.2007.03.005
Koroneos, C. J., and Nanaki, E. A. (2017). Environmental Impact Assessment of a Ground Source Heat Pump System in Greece. Geothermics 65, 1–9. doi:10.1016/j.geothermics.2016.08.005
Le, K. X., Huang, M. J., Shah, N., Wilson, C., Artain, P. M., Byrne, R., et al. (2019). High Temperature Air Source Heat Pump Coupled with Thermal Energy Storage: Comparative Performances and Retrofit Analysis. Energy Procedia 158, 3878–3885. doi:10.1016/j.egypro.2019.01.857
Marinelli, S., Lolli, F., Butturi, M. A., Rimini, B., and Gamberini, R. (2020). Environmental Performance Analysis of a Dual-Source Heat Pump System. Energy Build. 223, 110180. doi:10.1016/j.enbuild.2020.110180
Marinelli, S., Lolli, F., Gamberini, R., and Rimini, B. (2019). Life Cycle Thinking (LCT) Applied to Residential Heat Pump Systems: A Critical Review. Energy Build. 185, 210–223. doi:10.1016/J.ENBUILD.2018.12.035
Ministry CIET, (2017). 2nd National Plan fo Increasing 605 the Number of Nearly Zero-Energy Buildings (NZEBs). Nicosia, Cyprus: Ministry of Commerce Industry and Energy Tourism.
Monteiro, H., and Freire, F. (2012). Life-cycle Assessment of a House with Alternative Exterior Walls: Comparison of Three Impact Assessment Methods. Energy Build. 47, 572–583. doi:10.1016/j.enbuild.2011.12.032
Petrillo, A., De Felice, F., Jannelli, E., Autorino, C., Minutillo, M., and Lavadera, A. L. (2016). Life Cycle Assessment (LCA) and Life Cycle Cost (LCC) Analysis Model for a Stand-Alone Hybrid Renewable Energy System. Renew. Energy 95, 337–355. doi:10.1016/J.RENENE.2016.04.027
Ren, C., Deng, Y., and Cao, S.-J. (2018). Evaluation of Polyethylene and Steel Heat Exchangers of Ground Source Heat Pump Systems Based on Seasonal Performance Comparison and Life Cycle Assessment. Energy Build. 162, 54–64. doi:10.1016/j.enbuild.2017.12.037
Saner, D., Juraske, R., Kübert, M., Blum, P., Hellweg, S., and Bayer, P. (2010). Is it Only CO2 that Matters? A Life Cycle Perspective on Shallow Geothermal Systems. Renew. Sustain. Energy Rev. 14, 1798–1813. doi:10.1016/j.rser.2010.04.002
Seddiki, M., and Bennadji, A. (2019). Multi-criteria Evaluation of Renewable Energy Alternatives for Electricity Generation in a Residential Building. Renew. Sustain. Energy Rev. 110, 101–117. doi:10.1016/j.rser.2019.04.046
Sevindik, S., Spataru, C., Domenech Aparisi, T., and Bleischwitz, R. (2021). A Comparative Environmental Assessment of Heat Pumps and Gas Boilers towards a Circular Economy in the uk. Energies 14, 3027. doi:10.3390/en14113027
Guinée, J. B., Gorrée, M., Heijungs, R., Huppes, G., Kleijn, R., and de Koning, A. (2001). Life Cycle Assessment: An Operational Guide to the ISO Standards - Part 2a. Netherlands: Centre of Environmental Science - Leiden University.
Smith, M., Bevacqua, A., Tembe, S., and Lal, P. (2021). Life Cycle Analysis (LCA) of Residential Ground Source Heat Pump Systems: A Comparative Analysis of Energy Efficiency in New Jersey. Sustain. Energy Technol. Assessments 47, 101364. doi:10.1016/J.SETA.2021.101364
Steubing, B., Wernet, G., Reinhard, J., Bauer, C., and Moreno-Ruiz, E. (2016). The Ecoinvent Database Version 3 (Part II): Analyzing LCA Results and Comparison to Version 2. Int. J. Life Cycle Assess. 21, 1269–1281. doi:10.1007/s11367-016-1109-6
Sutman, M., Speranza, G., Ferrari, A., Larrey-Lassalle, P., and Laloui, L. (2020). Long-term Performance and Life Cycle Assessment of Energy Piles in Three Different Climatic Conditions. Renew. Energy 146, 1177–1191. doi:10.1016/j.renene.2019.07.035
Tsagarakis, K. P., Efthymiou, L., Michopoulos, A., Mavragani, A., Anđelković, A. S., Antolini, F., et al. (2020). A Review of the Legal Framework in Shallow Geothermal Energy in Selected European Countries: Need for Guidelines. Renew. Energy 147, 2556–2571. doi:10.1016/J.RENENE.2018.10.007
Weidema, B. P. (2015). Comparing Three Life Cycle Impact Assessment Methods from an Endpoint Perspective. J. Industrial Ecol. 19, 20–26. doi:10.1111/jiec.12162
Keywords: GSHP, environmental impact, GHE, openLCA, geothermal energy, LCA, CO2 payback period
Citation: Aresti L, Florides GA, Skaliontas A and Christodoulides P (2022) Environmental Impact of Ground Source Heat Pump Systems: A Comparative Investigation From South to North Europe. Front. Built Environ. 8:914227. doi: 10.3389/fbuil.2022.914227
Received: 06 April 2022; Accepted: 09 June 2022;
Published: 04 July 2022.
Edited by:
Sudhakar Babu Thanikanti, Chaitanya Bharathi Institute of Technology, IndiaReviewed by:
Serik Tokbolat, Nottingham Trent University, United KingdomCopyright © 2022 Aresti, Florides, Skaliontas and Christodoulides. This is an open-access article distributed under the terms of the Creative Commons Attribution License (CC BY). The use, distribution or reproduction in other forums is permitted, provided the original author(s) and the copyright owner(s) are credited and that the original publication in this journal is cited, in accordance with accepted academic practice. No use, distribution or reproduction is permitted which does not comply with these terms.
*Correspondence: Lazaros Aresti, bGcuYXJlc3RpQGVkdS5jdXQuYWMuY3k=
Disclaimer: All claims expressed in this article are solely those of the authors and do not necessarily represent those of their affiliated organizations, or those of the publisher, the editors and the reviewers. Any product that may be evaluated in this article or claim that may be made by its manufacturer is not guaranteed or endorsed by the publisher.
Research integrity at Frontiers
Learn more about the work of our research integrity team to safeguard the quality of each article we publish.