- 1School of Architecture, Tianjin University, Tianjin, China
- 2China Academy of Building Research, Beijing, China
Light adaptation is very important to keep the visual system acutely sensitive to scene content over a wide range of illumination, and has strong influence on brightness perception, visual performance and glare evaluation. The estimation method for the light adaptation state under real circumstances is a big pending topic for lighting industry. The discovery of Intrinsically Photosensitive Retinal Ganglion Cells (ipRGCs) has offered us an exciting entry point to comprehend how light adaptation is regulated. In this paper, we made a comprehensive and thorough analysis of forty-five studies focusing on light adaptation with the emerging knowledge on non-image-forming effect (NIF), to help researchers facilitate the research on light adaptation under complex luminance distribution. From the review, we can have the following outputs: 1) NIF may directly mediate the function of luminance adaptation, and we can take pupillary light reflex (PLR) as an objective indicator for the adaptation; 2) the action spectra of light adaptation may not be a fixed formula like V(λ), but may vary dynamically with luminance level; 3) there is high necessity to conduct research on light adaptation within a natural and complex environment. All these outputs can provide us a new perspective on light adaptation in visual system research.
1 Introduction
In natural conditions, the ambient light level of the prevailing light condition the human visual system may encounter, has an enormous range of luminance (from about 10−6 to 10+6 cd/m2 approximately 12 log units) (David et al., 2011a). But it is impossible for the biological hardware of the visual system to have a dynamic range to match such a large range of luminance distribution in our daily life (Wilson, 1997; Hung and Ciuffreda, 2002a). To keep the visual system acutely sensitive to scene content over a wide range of illumination, light adaptation is an important mechanism to prevent the situation that small signals drown in neuronal noise, and large signals saturate the system, by adjusting the gain or sensitivity of our visual system (Poot, 2000). The purpose of light adaptation is to offer an escape from the “saturation catastrophe”, so that we can keep the retinal response to contrast invariant with changes of illumination, and thereby achieves one major goal of vision: constancy of the visual perception of reflecting objects (Shapley and Enroth-Cugell, 1984a). The adaptation luminance describes the level of brightness to which the eye is adapted (prEN, 2019a).
1.1 Importance of light adaptation
The neural effects of adaptation are a realization and encoding of this context in the brain and thus represent a fundamental component of sensory information processing (Kohn, 2007). Adaptation occurs in a variety of forms in all sensory systems, and it definitely has significant meaning for our life. The light adaptation of visual systems has significant meaning in the following activities.
1.1.1 Brightness perception
Brightness is an attribute of visual perception according to which an area appears to emit, or reflect, more or less light (CIE, 2017). Brightness is the most fundamental visual perception and is central to illuminating engineering and lighting design (David et al., 2011b). According to the Weber-Fechner law introduced by Fechner, the subjective sensation of sight, sound and weight (all psychological elements) is not proportional to the intensity of the stimulus (a physical element) (Fechner, 1860). Brightness of an object or a light varies not only with its luminance but also with the state of the adaptation of the visual system (CIE 135/5:1999 Visual Adaptation to Complex Luminance, 1999), the main function of which is to keep the retinal response to contrast invariant with changes of illumination, and thereby achieves one major goal of vision: constancy of the visual perception of reflecting objects (Shapley and Enroth-Cugell, 1984b).
1.1.2 Visual performance
Pupil diameter has a large effect on the optical transfer function of the eye, depth of vision, as well as on retinal illuminance, which in turn influences contrast sensitivity (Watson and Yellott, 2012a). And a lot of research show that the most powerful determinant of pupil size is adaptation luminance (Bouma, 1962a; Loewenfeld, 1993; Clarke et al., 2003; Hall and Chilcott, 2018a). Luminance distribution in the field of view controls the adaptation level of the eyes, which affects the task visibility (prEN, 2019b):
— visual acuity (sharpness of vision);
— contrast sensitivity (discrimination of small relative luminance differences);
— efficiency of the ocular functions (such as accommodation, convergence, pupillary contraction, eye movements, etc.).
1.1.3 Discomfort glare
Glare is the sensation produced by luminance within the visual field that is sufficiently greater than the luminance to which the eyes are adapted to cause annoyance, discomfort or loss in visual performance and visibility (Rea, 2000a; Suk, 2014; CIE 2017, 2017). The discomfort effect of glare may lead to feelings of irritation and fatigue in interior lighting situations. The key factors influencing the discomfort glare perception can be (Wang, 2019):
_ luminance of glare source
_ Apparent size of the glare source (solid angle)
_ Location of glare source in the visual field
_ number of glare sources
_ Adaptation luminance, which describes the level of luminance to which the eye is adapted.
The adaptation luminance of the eye will play an important role on glare evaluation (CIBSE 2002, 2002).
1.2 Necessity of light adaptation research
In experimental research for light adaptation related topics, Goldmann perimeter is always used to produce a uniform background luminance for adaptation [ (Luckiesh and Guth, 1949), (Kim and Koga, 2004), (Eble-Hankins, 2008), (Tyukhova, 2015), (Uchida et al., 2016)]. But in the real situation, the luminance distribution of natural scenes are always complex. The measurement or estimation of adaptation level in natural environments is a very important topic, but there have been no studies directly dealing with this issue (International Commission On Illumination (CIE), 1999).
There have been several formulae used to predict discomfort based on stimulus parameters, and every formula has its own adaptation luminance computation method as table 1 shows (Rea, 2000b; CIE 112, 1994; CIE 117, 1995; Hopkinson, 1972), and none of these methods consider the influence of luminance distribution.
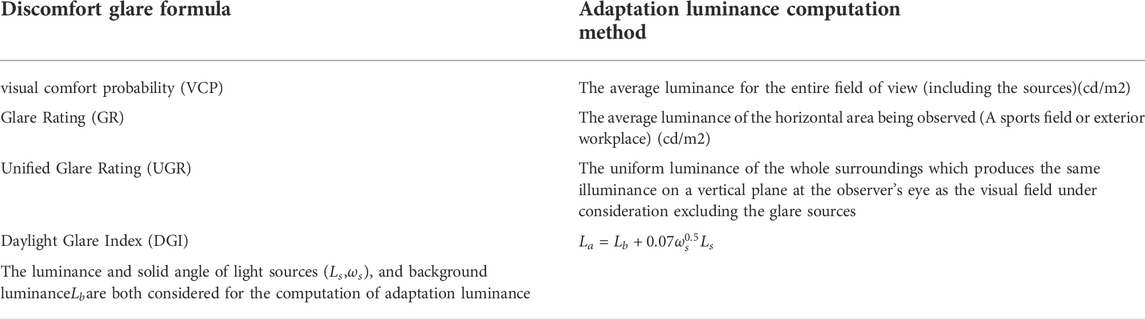
TABLE 1. Comparison on adaptation luminance computation methods of different discomfort glare formulae.
The reason why no consensus for the estimation of adaptation luminance has been established is that the mechanism on how visual adaptation is regulated is still unknown (Hankins and Hughes, 2014a; Dniel, 2016a).
2 Review of literatures
The search was designed to focus on the concept: light adaptation. Hence, we select the search terms related to light were chosen to be (“light adaptation” OR “visual adaptation”). The terms were searched in titles, abstracts and keywords of the papers. The search terms did not include only “light” and “visual” due to its ambiguity and frequent use in idioms and phrases. Due to the large number of papers and their variety in exposure details, additional quality criteria was determined to select the papers of highest relevance to our interest. The quality criteria include 1) focus on human subjective, 2) the light adaptation mediated by luminance. Forty-five papers were finally selected based on the criteria. From the reviewed paper, we can have much clearer understanding on mechanism on light adaptation regulation, the influence of ipRGC on light adaptation, and objective indicator for light adaptation.
2.1 Mechanism on light adaptation regulation
In the past, all attempts to understand the mechanism for this adaptation assumed that these adaptations were mediated by light detection of the classical photoreceptor cells (rods and cones) under all irradiances (Hankins and Lucas, 2002).
But the classical photoreceptors like cones have an intrinsic ability to adjust their sensitivity to the level of ambient illumination to which they are exposed, called light adaptation, which means that a cone photoreceptor has an automatic gain control similar to a camera, as shown in Figure 1 (Hung and Ciuffreda, 2002b; Luo et al., 2008). This means that the same cone response may have a different corresponding light intensity, so the classical photoreceptors do not retain information about the external irradiance required to detect changes in the solar day, but only keep the retinal response to contrast invariant with changes of illumination (Dniel, 2016b). And the analysis of Mante et al. on natural images further confirmed the possibility that the perception of contrast and absolute light level of the environment may come from separate pathways (Mante et al., 2005). In 1969, the retinal ganglion cell (RGC) found by Barlow and Levick offer us the opportunity to find the pathway for absolute luminance sensory, the mean firing rate of which increases monotonically with the adaptation luminance level (Barlow and Levick, 1969). But the discovery of ipRGCs and genetic proof for their important role in major NIF responses have offered an exciting entry point to comprehend how the light adaptation is regulated (Megumi and Satchidananda, 2010a).
2.2 The influence of ipRGC on light adaptation
Although the first identification of ipRGCs by Clyde Keeler was in 1923, it was not until the end of the 20th century that their significance was truly understood (Jeffrey, 2012). The discovery of melanopsin (encoded by the gene Opn4), a new photopigment within the mammalian retina, in 2000 b y Provencio and others, laid the foundation for the research of the light adaptation of visual systems (Provencio et al., 2000). Soon after its discovery, melanopsin was localized to a small population (less than 2%) of RGCs in the mammalian retina (Gooley et al., 2001; Hattar et al., 2002). Brainard and his colleagues, also confirmed the single photopigment may be primarily responsible for melatonin suppression, and the peak absorbance of which appears to be distinct from that of rod and cone cell photopigments for vision (Brainard et al., 2001).
Berson and his team conclusively identified the elusive third class of retinal photoreceptors with retrograde transport of fluorescent microspheres injected from the suprachiasmatic nucleus (SCN) in the hypothalamus, the master circadian pacemaker, and demonstrated that the electrophysiological and spectral characteristics of these cells match those observed behaviorally in measures of circadian photo-entrainment (Berson et al., 2002a). Further research found that ipRGCs also send signal via the retinohypothalamic tract (RHT) to other brain regions such as the shell of olivary pretectal nucleus (OPN) for mediation of the pupillary light reflex (PLR), the ventral lateral preoptic nucleus (VLPO) for sleep induction, and the dorsal lateral geniculate nucleus (dLGN) as shown in Figure 2 [ , (Megumi and Satchidananda, 2010b)].
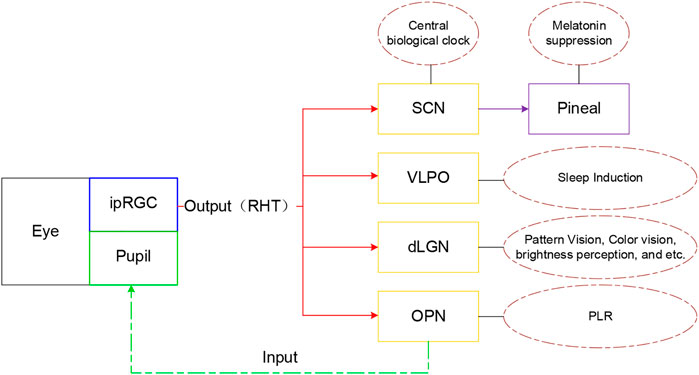
FIGURE 2. Diagram of brain regions projected by ipRGC and their function (Zhao et al., 2020)
Effective adaptation relies upon an accurate measure of light intensity. IpRGC can perform a role analogous to a photographer’s light meter, providing an independent measure of irradiance for the visual system to optimize light adaptation, through their following unique features (Berman and Clear, 2008; Do and Yau, 2010; Allen et al., 2014; Hankins and Hughes, 2014b):
■ Unlike rods and cones, ipRGCs do not adjust their sensitivity according to lighting conditions (’‘adaptation’‘) (Wong et al., 2006). The sustained and linear light response of ipRGCs under conditions of continuous bright illumination, as Barlow and Levick has found, make them faithfully encode stimulus energy over relatively long periods of time (; Dacey et al., 2005a; Wong, 2012).
■ ipRGCs are much less sensitive to light than classical photoreceptors, and signal with far slower kinetics, which may provide for long temporal integration, which may well suit the primary function of these cells, assessing ambient light levels via irradiance detection (Do et al., 2009).
■ The ipRGCs have large cell bodies, dendrite trees and large receptive fields (at least 5–10 times more extensive than those for classical RGCs) to reliably signal ambient illumination levels, at the cost of spatiotemporal resolution (Berson, 2003c; Dacey et al., 2005b).
2.3 Objective indicator for light adaptation
Adaptation is mediated by an autonomic nervous system, which is a sub-consciousness behavior that is not noticeable directly [ (Ben and David, 2014), (Newell and Shanks, 2014), (Pedro Cardinali, 2018)]. The pupillary light reflex (PLR) describes the constriction and subsequent dilation of the pupil in response to light, which mainly depends on an adapting luminance, modulated by other factors (Hall and Chilcott, 2018b). Pupil area decreases with increasing irradiance over a ∼9 log unit range, with a feature of its tonic nature in bright light: constriction is held steady under continuous illumination (Gamlin et al., 2007). So PLR under steady illumination provides a rapid, objective, non-invasive measure of light adaptation.
Historically, it was assumed that the light-invoked neural signals driving the PLR originated exclusively from rod and cone photoreceptors (Mcdougal and Gamlin, 2009). Watson and Yellott (2012) developed a unified formula to predict pupil size from luminance, with the covariation of other factors including age, emotion, etc. (Watson and Yellott, 2012b) But several observations have shown that V(λ)-weighted indicators do not predict the PLR very well, and Bouma proposed that PLR was controlled by S cones and rods (Bouma, 1962b; Berman et al., 1992).
The discovery of ipRGC and its projection to OPN, gives us a new explanation on the disestimation of PLR with V(λ) based indicators such as luminance or illuminance. The relative contribution of intrinsic melanopsin and its connected rods and cones on PLR at any given moment varies with light characteristics, such as wavelength, intensity, and exposure duration (Berson et al., 2002b; Ruby et al., 2002; Hattar et al., 2003; Barnard et al., 2006). There are three phases of PLR that are distinguishable on a pupillogram as shown in Figure 3 (Munch et al., 2012; Petteri, 2012; Adhikari et al., 2015; Hall and Chilcott, 2018c):
■ Transient response: Due to the long latency of melanopsin, following onset of an incremental light pulse, the initial PLR is predominantly mediated by the classical photoreceptors.
■ Steady state stage: After this peak constriction, the pupil gradually relaxes to a more dilated state because of the light adaptation for classical photoreceptors and the involvement of melanopsin if the light intensity is higher than the threshold of melanopsin. The individual contribution of each photoreceptor class to PLR remains unclear.
■ Post-illumination stage: Melanopsin controls the Post-Illumination Pupil Response (PIPR), the sustained pupil constriction after light removal.
Thanks to the new discovery of ipRGC, we can easily bridge the PLR with light adaptation, as the ipRGC has the function to encode the ambient light level to input into image-forming physiological responses, such as light adaptation and brightness perception (Brown et al., 2010). This further verifies the assumption that the pupil area under steady illumination can be the indicator for light adaptation.
3 Further discussion on factors influencing NIF effect
Light is the primary stimulus for regulating ipRGC-influenced responses including circadian rhythms, seasonal cycles, neuroendocrine responses and light adaptation in many species, including humans (Klein et al., 1991; Wehr, 1991), the response of which mainly depends on the intensity of the light stimuli (Adamsson, 2018). There has been a tradition of measuring vertical photopic illuminance or vertical irradiance at the corneal plane in many studies (Zhang, 2013). Research indicates that spectral distribution and the direction of the light received at the eye are relevant parameters in studies looking into NIF effects, so it seems to be necessary to consider these two parameters to reduce the inconclusiveness within these studies (Knoop et al., 2019).
3.1 Spectral distribution
The intrinsic photo-response of ipRGCs is mediated by the photopigment melanopsin and has been shown to be a good fit to a single pigment absorbance spectrum center at around 480 nm (HATTAR et al., 2002a; Mcdougal and Gamlin, 2008). Several research studies have been conducted to establish the action spectrum of melanopsin (Gall and Lapuente, 2002; REA et al., 2005; Tsai et al., 2009; Bailes and Lucas, 2013). In addition to the intrinsic signal of melanopsin, it is clear that ipRGCs receive rod and cone inputs, to mediate the NIF effects of light in humans (Lucas et al., 2013). On the basis of these pioneering works, the international standard CIE S 026/E:2018 defines spectral sensitivity functions of the five photoreceptor types (see Figure 4) (CIE026/, 2018a). However, the individual contribution of each photoreceptor class to irradiance responses remains unclear (Lall et al., 2010). Several findings were observed for ipRGC-influenced responses, where rods are necessary for entrainment at low light levels, and melanopsin-mediated light responses are the major, but not the only contributor to entrainment at high light levels (Altimus et al., 2010; Spitschan, 2019). So the action spectra of ipRGCs is not a fixed formula, but changes with wavelength, intensity, and exposure duration.
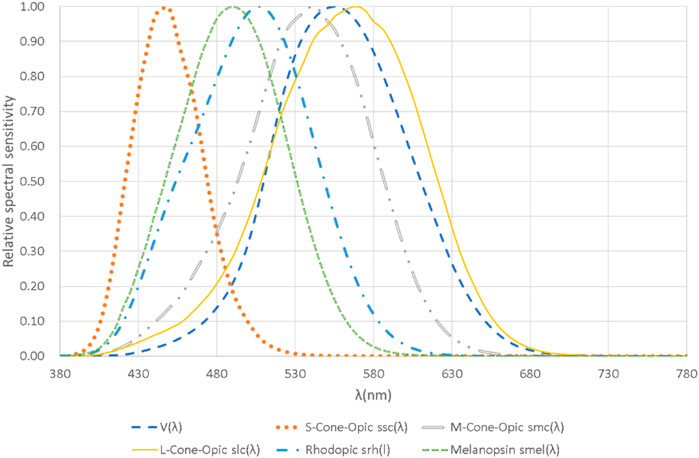
FIGURE 4. The five α-opic action spectra and V(λ) plotted against wavelength with a linear scale for the relative spectral sensitivity. Plotted on the basis of the data from CIE S 026/E:2018.
3.2 Light direction or luminance spatial distribution
There is an established practice of measuring Melanopic irradiance or Melanopic equivalent daylight (D65) illuminance at the corneal plane in many studies on NIF effect (Rea et al., 2005; Rea et al., 2012; International WELL building institute and V1, 2016). This gives a measure of cosine-weighted radiance averaged over a full 2π steradian field of view, but does not consider the directionality of light, which is an influential parameter in the field of NIF effects (Khademagha et al., 2016a; Khademagha et al., 2016b). Some research has found that direct light exposure by a small- and high-power light source on a limited area of retina might be less effective than more general, lower-intensity illumination because the response is likely to be saturated (CIE, 2016). Although the literature on the influence of light directionality to NIF effect is limited, distribution of ipRGCs on the retina and field of view (FOV) may contribute to the impact of lighting incidence direction on NIF effect:
■ Animal studies of ipRGCs have confirmed its non-homogenous distribution on the retina of rat, cat and primate, but there are difference among species in that rats show higher density of ipRGCs in the superior and temporal retina than in the inferior or nasal retina, and macaques show greater ipRGC density near the fovea rather than in the peripheral retina (HATTAR et al., 2002b; Hannibal et al., 2002; Dacey et al., 2005c; Hughes et al., 2013). Although the exact spatial distribution of ipRGCs is still under investigation, its non-uniform distribution highlights the importance of radiation directionality on NIF effects and light adaptation.
■ For the constraint of the eyelids, gaze angle, etc., our FOV actually cannot be a full 2π steradian, so we need to make a correction. CIE S 026/E:2018 recommends typical FOV values, for indoor and outdoor conditions, to mimic the viewing condition for assessments of a spatially averaged radiance for determining the retinal irradiance (CIE026/, 2018b).
4 New perspectives for luminance adaptation
The light adaptation of the visual system is a factor of great importance for our better understanding of vision, and better innovation of lighting application, and therefore the estimation method for the adaptation state under real conditions is a big pending topic for lighting industry. With the deeper understanding on ipRGCs and its related responses, we can have a new perspective to explain the mechanism for light adaptation of the visual system and establish the corresponding estimation method for a complex scene. From our review on the latest literature, we can have the following new insights on light adaptation in visual system research:
■ As a sub-conscious behavior, people cannot detect their light adaptation state. We can take the PLR under steady illumination as the objective indicator for light adaptation, for the reason that they are both mediated by the same signal from ipRGCs.
■ Melanopsin and the classical photoreceptors are all contributors to the PLR and light adaptation, but the individual contribution of each photoreceptor class changes with the intensity, spectra and duration. So the action spectra of light adaptation may not be a fixed formula like V(λ), but may vary dynamically with luminance level.
■ For the reason of nonhomogeneous distribution of ipRGCs on the retina and the limitation of FOV, different directions of incident radiation have different effectiveness on NIF effects and light adaptation. This reconfirms the traditional luminance adaptation indicators used in the glare calculation can not work well under real situation, so there is high necessity to conduct research on light adaptation within a natural and complex environment.
Further research may give us more insight on the mechanism of discomfort glare perception, which can offer a theoretical basis for an integrated glare metric for various lighting applications, listed as a top priority topic for research by CIE (Commission Internationale de l'Eclairage (CIE), 2020). In the meantime, because the adaptation level of the visual system also has a strong influence on visual performance and comfort, even for occupant health related to ipRGCs, the benefit of research on light adaptation may be not limited to the glare issue but may have much wider applicability.
Author contributions
Conceptualization and Organization, SW; Writing—SW; Writing—Review and Editing, JZ; Funding Acquisition, JZ; Supervision, JZ.
Funding
This research was funded by National Key R&D Program of China (2018YFC0705100).
Acknowledgments
We would like to thank Peter Thorns and Kees Teunissen (PhD) for helpful comments on the manuscript.
Conflict of interest
The authors declare that the research was conducted in the absence of any commercial or financial relationships that could be construed as a potential conflict of interest.
Publisher’s note
All claims expressed in this article are solely those of the authors and do not necessarily represent those of their affiliated organizations, or those of the publisher, the editors and the reviewers. Any product that may be evaluated in this article, or claim that may be made by its manufacturer, is not guaranteed or endorsed by the publisher.
References
Adamsson, M. (2018). Non-image-forming effects of light: Implications for the design of living and working environments[D]. Lund: Department of Architecture and Built Environment, Lund University.
Adhikari, Prakash, Zele, Andrew, and Feigl, Beatrix (2015). The post-illumination pupil response (PIPR). Invest. Ophthalmol. Vis. Sci. 56 (6), 3838–3849. doi:10.1167/iovs.14-16233
Allen, A., Storchi, R., Martial, F., el, at, Montemurro, M., Brown, T., et al. (2014). Melanopsin-driven light adaptation in mouse vision. Curr. Biol. 24, 2481–2490. doi:10.1016/j.cub.2014.09.015
Altimus, C. M., Guler, A. D., Alam, N. M., Arman, A. C., Prusky, G. T., Sampath, A. P., et al. (2010). Rod photoreceptors drive circadian photoentrainment across a wide range of light intensities. Nat. Neurosci. 13, 1107–1112. doi:10.1038/nn.2617
Bailes, H. J., and Lucas, R. J. (2013). Human melanopsin forms a pigment maximally sensitive to blue light ( λmax ≈ 479 nm) supporting activation of G q/11 and G i/o signalling cascades. Proc. R. Soc. B 280, 20122987. doi:10.1098/rspb.2012.2987
Barlow, H. B., and Levick, W. R. (1969). Changes in the maintained discharge with adaptation level in the cat retina. J. Physiology 202, 699–718. doi:10.1113/jphysiol.1969.sp008836
Barnard, A., Hattar, S., Hankins, M., and Lucas, R. (2006). Melanopsin regulates visual processing in the mouse retina. Curr. Biol. 16, 389–395. doi:10.1016/j.cub.2005.12.045
Ben, R, N., and David, R, S. (2014). Unconscious influences on decision making: A critical review. Behav. Brain Sci. 37, 1–19. doi:10.1017/s0140525x12003214
Berman, S. M., and Clear, R. D. (2008). Past vision studies can support a novel human photroreceptor. Light and Eng. 16 (2), 88–94.
Berman, S. M., Fein, G., Jewett, D. L., Saika, G., and Ashford, F. (1992). Spectral determinants of steady-state pupil size with full field of view. J. Illum. Eng. Soc. 21 (2), 3–13. doi:10.1080/00994480.1992.10747995
Berson, D. M., Dunn, F. A., and Takao, M. (2002). Phototransduction by retinal ganglion cells that set the circadian clock. Science 295, 1070–1073. doi:10.1126/science.1067262
Berson, D. M., Dunn, F. A., and Takao, M. (2002). Phototransduction by retinal ganglion cells that set the circadian clock. Science 295 (5557), 1070–1073. doi:10.1126/science.1067262
Berson, D. M. (2003). Strange vision: Ganglion cells as circadian photoreceptors. Trends Neurosci. 26, 314–320. doi:10.1016/S0166-2236(03)00130-9
Bouma, H. (1962). Size of the static pupil as a function of wave-length and luminosity of the light incident on the human eye. Nature 193 (4816), 690–691. doi:10.1038/193690a0
Bouma, H. (1962). Size of the static pupil as a function of wavelength and luminosity of the light incident on the human eye. Nature 193, 690–691. doi:10.1038/193690a0
Brainard, G. C., Hanifin, J. P., Greeson, J., Byrne, B., Glickman, G., Gerner, E., et al. (2001). Action spectrum for melatonin regulation in humans: Evidence for a novel circadian photoreceptor. J. Neurosci. 21 (16), 6405–6412. doi:10.1523/jneurosci.21-16-06405.2001
Brown, Timothy M., Gias, Carlos, Hatori, Megumi, Keding, Sheena R., Semo, Ma’ayan, Coffey, Peter J., et al. (2010). Melanopsin contributions to irradiance coding in the thalamocortical visual system. PLoS Biol. 8 (12), e1000558. doi:10.1371/journal.pbio.1000558
CIE 112:1994 , glare evaluation system for use within outdoor sports and area lighting, Commission Internationale de l'Eclairage CIE, Vienna, Austria.
CIE 117 -1995 Discomfort glare in interior lighting, Commission Internationale de l'Eclairage CIE, Vienna, Austria.
CIE 135/5:1999 Visual Adaptation to Complex Luminance (1999). International commission on illumination (CIE), vienna. Austria: CIE.
CIE (2016). CIE 218:2016 Research roadmap for healthful interior lighting application. Austria: COMMISSION INTERNATIONALE DE L'ECLAIRAGE(CIE) Vienna..
CIE Cie dis 017:2017, ilv: International lighting vocabulary. 2nd Edition. Vienna, Austria: Commission Internationale de l'Eclairage CIE.
Cie026/, S. E. (2018). CIE system for metrology of optical radiation for ipRGC-influenced responses to light. Vienna, Austria: COMMISSION INTERNATIONALE DE L'ECLAIRAGECIE.
Cie026/, S. E. (2018). CIE system for metrology of optical radiation for ipRGC-influenced responses to light. Vienna, Austria: COMMISSION INTERNATIONALE DE L'ECLAIRAGE CIE.
Clarke, R. J., Zhang, H. Y., and Gamlin, P. D. R. (2003). Primate pupillary light reflex: Receptive field characteristics of pretectal luminance neurons. J. Neurophysiology 89, 3168–3178. doi:10.1152/jn.01130.2002
Commission Internationale de l'Eclairage (Cie), (2020). CIE research strategy. Vienna, Austria: CIE.
Dacey, D. M., Liao, H. W., Peterson, B. B., Robinson, F. R., Smith, V. C., Pokorny, J., et al. (2005). Melanopsin-expressing ganglion cells in primate retina signal colour and irradiance and project to the LGN. Nature 433, 749–754. doi:10.1038/nature03387
Dacey, D. M., Liao, H. W., Peterson, B. B., Robinson, F. R., Smith, V. C., Pokorny, J., et al. (2005). Melanopsin-expressing ganglion cells in primate retina signal colour and irradiance and project to the LGN. Nature 433, 749–754. doi:10.1038/nature03387
Dacey, D. M., Liao, H. W., Peterson, B. B., Robinson, F. R., Smith, V. C., Pokorny, J., et al. (2005). Melanopsin-expressing ganglion cells in primate retina signal colour and irradiance and project to the LGN. Nature 433, 749–754. doi:10.1038/nature03387
David, L. D., Kevin, W. H., Richard, G. M., et al. (2011). The lighting handbook. 10th Edition. New York, NY: Illuminating Engineering Society. IES HB-10-11.
Dniel, S. J. (2016). Temporal spatial and adaptation characteristics of melanopsin input to the human pupil light reflex. Brisbane, QL: Queensland University of Technology.
Do, M. T., Kang, S. H., Xue, T., Zhong, H., Liao, H. W., Bergles, D. E., et al. (2009). Photon capture and signalling by melanopsin retinal ganglion cells. Nature 457, 281–287. doi:10.1038/nature07682
Do, M. T. H., and Yau, K.-W. (2010). Intrinsically photosensitive retinal ganglion cells. Physiol. Rev. 90, 1547–1581. doi:10.1152/physrev.00013.2010
Eble-Hankins, M. L. (2008). Subjective impression of discomfort glare from sources of non-uniform luminance. Lincoln: ETD collection for University of Nebraska - Lincoln. https://digitalcommons.unl.edu/dissertations/AAI3315315.
Gall, D., and Lapuente, V. (2002). Licht.Aspects involving illumination in the selection of an effective lamp spectrum.
Gamlin, P. D., McDougal, D. H., Pokorny, J., Smith, V. C., Yau, K. W., and Dacey, D. M. (2007). Human and macaque pupil responses driven by melanopsin-containing retinal ganglion cells. Vis. Res. 47 (7), 946–954. doi:10.1016/j.visres.2006.12.015
Gooley, J. J., Lu, J., Chou, T. C., Scammell, T. E., and Saper, C. B. (2001). Melanopsin in cells of origin of the retinohypothalamic tract. Nat. Neurosci. 4, 1165. doi:10.1038/nn768
Hall, C., and Chilcott, R. (2018). Eyeing up the future of the pupillary light reflex in neurodiagnostics. Diagnostics 8 (1), 19. doi:10.3390/diagnostics8010019
Hall, C., and Chilcott, R. Eyeing up the future of the pupillary light reflex in neurodiagnostics[J]. Diagnostics, 2018, 8(1):19–67.doi:10.3390/diagnostics8010019
Hankins, Mark W., and Hughes, S. (2014). Vision: Melanopsin as a novel irradiance detector at the heart of vision. Curr. Biol. 24, R1055–R1057. doi:10.1016/j.cub.2014.09.034
Hankins, Mark W., and Hughes, S. (2014). Vision: Melanopsin as a novel irradiance detector at the heart of vision. Curr. Biol. 24, R1055–R1057. doi:10.1016/j.cub.2014.09.034
Hankins, M. W., and Lucas, R. J. (2002). The primary visual pathway in humans is regulated according to long-term light exposure through the action of a nonclassical photopigment. Curr. Biol. 12, 191–198. doi:10.1016/s0960-9822(02)00659-0
Hannibal, J., Hindersson, P., Knudsen, S. M., Georg, B., and Fahrenkrug, J. (2002). The photopigment melanopsin is exclusively present in pituitary adenylate cyclase-activating polypeptide-containing retinal ganglion cells of the retinohypothalamic tract. J. Neurosci. 22 (1), RC191. doi:10.1523/jneurosci.22-01-j0002.2002
Hattar, S., Liao, H. W., Takao, M., Berson, D. M., and Yau, K. W. (2002). Melanopsincontaining retinal ganglion cells: Architecture, projections, and intrinsic photosensitivity. Science 295, 1065–1070. doi:10.1126/science.1069609
Hattar, S., Lucas, R. J., Mrosovsky, N., Thompson, S., Douglas, R. H., Hankins, M. W., et al. (2003). Melanopsin and rod-cone photoreceptive systems account for all major accessory visual functions in mice. Nature 424, 75–81. doi:10.1038/nature01761
Hattar, S., Liao, H. W., Takao, M., Berson, D. M., and Yau, K. W. (2002). Melanopsincontaining retinal ganglion cells: Architecture, projections, and intrinsic photosensitivity. Science 295, 1065–1070. doi:10.1126/science.1069609 no
Hattar, S., Liao, H. W., Takao, M., Berson, D. M., and Yau, K. W. (2002). Melanopsincontaining retinal ganglion cells: Architecture, projections, and intrinsic photosensitivity. Science 295, 1065–1070. doi:10.1126/science.1069609
Hopkinson, R. (1972). Glare from daylighting in buildings. Appl. Ergon. 3 (4), 206–215. doi:10.1016/0003-6870(72)90102-0
Hughes, S., Watson, T., Foster, R., Peirson, S., and Hankins, M. (2013). Nonuniform distribution and spectral tuning of photosensitive retinal ganglion cells of the mouse retina. Curr. Biol. 23 (17), 1696–1701. doi:10.1016/j.cub.2013.07.010
Hung, George K., and Ciuffreda, Kenneth J. (2002). Model of visual system[M]. New York, USA: Kluwer Academic/Plenum Publishers, 125–127.
Hung, George K., and Ciuffreda, Kenneth J. (2002). Model of visual system[M]. New York, USA: Kluwer Academic/Plenum Publishers, p125–p127.
International Commission On Illumination (CIE) (1999). CIE 135/5:1999 visual adaptation to complex luminance. Vienna, Austria: CIE.
Jeffrey, H. (2012). “The melanopsin-dependent direct non-circadian effects of light : A third principal mechanism for the regulation of sleep and wake,” in Neurobiology. (Strasbourg, France: Université de Strasbourg). English. NNT : 2012STRAJ094.
Khademagha, P., Aries, M. B. C., Rosemann, A. L. P., and van Loenen, E. J. (2016). Implementing non-image-forming effects of light in the built environment: A review on what we need. Build and Environ. 108, 263–272. doi:10.1016/j.buildenv.2016.08.035
Khademagha, P., Aries, M., Rosemann, A., and Loenen, E. V. (2016). Why directionality is an important light factor for human health to consider in lighting design? Int. J. Sustain. Light. 18, 3–8. doi:10.26607/ijsl.v18i0.15
Kim, W., and Koga, Y. (2004). Effect of local background luminance on discomfort glare. Build. Environ. 38, 1435–1442. doi:10.1016/j.buildenv.2004.05.003
Klein, D. C., Moore, R. Y., and Reppert, S. M. (1991). Suprachiasmatic nucleus: The mind’s clock. Oxford: Oxford UP.
Knoop, M., Broszio, K., Diakite, A., Liedtke, C., Niedling, M., Rothert, I., et al. (2019). Methods to describe and measure lighting conditions in experiments on non-image-forming aspects. Leukos 15 (1-4), 163–179. doi:10.1080/15502724.2018.1518716
Kohn, A. (2007). Visual adaptation: Physiology, mechanisms, and functional benefits. J. Neurophysiol. 97 (5), 3155–3164. doi:10.1152/jn.00086.2007
Lall, G. S., Revell, V. L., Momiji, H., Al Enezi, J., Altimus, C. M., Guler, A. D., et al. (2010). Distinct contributions of rod, cone, and melanopsin photoreceptors to encoding irradiance. Neuron 66, 417–428. doi:10.1016/j.neuron.2010.04.037
Loewenfeld, I. E. (1993). The pupil. Anatomy, physiology, and clinical applications, 1. Ames, Detroit: Iowa State University Press, Wayne State University Press.
Lucas, R. J., Peirson, S. N., Berson, D. M., Brown, T. M., Cooper, H. M., Czeisler, C. A., et al. (2013). Measuring and using light in the melanopsin age. Trends Neurosci. 37 (1)–9. doi:10.1016/j.tins.2013.10.004
Luckiesh, M., and Guth, S. K. (1949). Brightnesses in visual field at borderline between comfort and discomfort. Illum. Eng. 44 (11), 650–670. PMID: 24536275.
Luo, D-G., Kefalov, V., and Yau, K-W. (2008). Phototransduction in rods and cones. The senses: A comprehensive reference. Editors R. Masland, and T. D. Albright (Oxford): Academic Press), Vol. 1, 269–301.
Mante, V., Frazor, R. A., Bonin, V., Geisler, W. S., and Carandini, M. (2005). Independence of luminance and contrast in natural scenes and in the early visual system. Nat. Neurosci. 8 (12), 1690–1697. doi:10.1038/nn1556
Mcdougal, David, and Gamlin, P. (2009). The influence of intrinsically photosensitive retinal ganglion cells on the spectral sensitivity and response dynamics of the human pupillary light reflex. Vis. Res. 50, 72–87. doi:10.1016/j.visres.2009.10.012
Mcdougal, D. H., and Gamlin, P. D. R. (2008). Pupillary control pathways. Senses A Compr. Reference 1, 521–536. doi:10.1016/b978-012370880-9.00282-6
Megumi, H., and Satchidananda, P. (2010). The emerging roles of melanopsin in behavioral adaptation to light. Trends Mol. Med. 16, 435–446. doi:10.1016/j.molmed.2010.07.005
Megumi, H., and Satchidananda, P. (2010). The emerging roles of melanopsin in behavioral adaptation to light. Trends Mol. Med. 16 (10), 435–446. doi:10.1016/j.molmed.2010.07.005
Munch, M., Leon, L., Crippa, S. V., and Kawasaki, A. (2012). Circadian and wake-dependent effects on the pupil light reflex in response to narrow-bandwidth light pulses. Invest. Ophthalmol. Vis. Sci. 53 (8), 4546–4555. doi:10.1167/iovs.12-9494
Newell, B. R., and Shanks, D. R. (2014). Unconscious influences on decision making: A critical review – addendum. Behav. Brain Sci. 37 (1), 24–19. doi:10.1017/s0140525x12099980
Pedro Cardinali, Daniel (2018). Autonomic nervous system-- basic and clinical aspects. Cham: Springer.
Poot, Lieke. (2000). Light adaptation and early processing in the human visual system. Doctoral dissertation.
prEN (2019). prEN 12464-1:2019, Light and lighting - lighting of work places - Part 1: Indoor work places[S], Brussel.
prEN (2019). prEN 12464-1:2019, Light and lighting - lighting of work places - Part 1: Indoor work places[S], Brussel.
Provencio, I., Rodriguez, I. R., Jiang, G., Hayes, W. P., Moreira, E. F., and Rollag, M. D. (2000). A novel human opsin in the inner retina. J. Neurosci. 20 (2), 600–605. doi:10.1523/jneurosci.20-02-00600.2000
Rea, M. S., Figueiro, M. G., Bierman, A., and Hamner, R. (2012). Modelling the spectral sensitivity of the human circadian system. Light. Res. Technol. 44, 386–396. doi:10.1177/1477153511430474
Rea, M. S., Figueiro, M. G., Bullough, J. D., and Bierman, A. (2005). A model of phototransduction by the human circadian system. Brain Res. Rev. 50, 213–228. doi:10.1016/j.brainresrev.2005.07.002
Mark S. Rea (Editor) (2000). The IESNA lighting handbook reference and application. Ninth Edition (New York: IESNA).
Mark S. Rea (Editor) (2000). (New York: IESNA).The IESNA lighting handbook reference and application
Rea, M. S., Figueiro, M. G., Bullough, J. D., and Bierman, A. (2005). A model of phototransduction by the human circadian system. Brain Res. Rev. 50, 213–228. doi:10.1016/j.brainresrev.2005.07.002
Ruby, N. F., Brennan, T. J., Xie, X., Cao, V., Franken, P., Heller, H. C., et al. (2002). Role of melanopsin in circadian responses to light. Science 298, 2211–2213. doi:10.1126/science.1076701
Shapley, R., and Enroth-Cugell, C. (1984). Chapter 9 Visual adaptation and retinal gain controls. Prog. Retin. Res. 3, 263–346. doi:10.1016/0278-4327(84)90011-7
Shapley, R. M., and Enroth-Cugell, C. (1984). Chapter 9 Visual adaptation and retinal gain controls. Prog. Retin. Res. 3, 263–346. doi:10.1016/0278-4327(84)90011-7
Suk, Jae Yong (2014). Absolute glare and relative glare factors: Predicting and quantifying levels of interior glare and exterior glare caused by sunlight and daylight[J]. Diss. Theses - Gradworks 14 (2), 322.
Petteri, T. (2012). “Spectral modulation of melanopsin responses : Role of melanopsin bistability in pupillary light reflex. Human health and pathology,” in Human health and pathology. (Villeurbanne, France: Université Claude Bernard - Lyon I). English. NNT : 2012LYO10037.
Tsai, J. W., Hannibal, J., Hagiwara, G., Colas, D., Ruppert, E., Ruby, N. F., et al. (2009). Melanopsin as a sleep modulator: Circadian gating of direct effects of light on sleep and altered sleep homeostasis in opn4-/-mice. PLoS Biol 7 (6), e1000125. doi:10.1371/journal.pbio.1000125
Tyukhova, Y. (2015). Discomfort glare from small, high luminance light sources in outdoor nighttime environments [D]. Lincoln, NE: University of Nebraska‒Lincoln.
Uchida, T., Ayama, M., Akashi, Y., Hara, N., Kitano, T., Kodaira, Y., et al. (2016). Adaptation luminance simulation for cie mesopic photometry system implementation. Light. Res. Technol. 48 (1), 14–25. doi:10.1177/1477153515626210
Wang, S. X. (2019). Preliminary research on mathematic model of eye’s adaptation luminance. CIE x046.
Watson, A. B., and Yellott, J. I. (2012). A unified formula for light-adapted pupil size. J. Vis. 12 (10), 12. doi:10.1167/12.10.12
Watson, A. B., and Yellott, J. I. (2012). A unified formula for light-adapted pupil size. J. Vis. 12 (10), 12. doi:10.1167/12.10.12
Wehr, T. A. (1991). The durations of human melatonin secretion and sleep respond to changes in daylength (photoperiod). J. Clin. Endocrinol. Metab. 73, 1276–1280. doi:10.1210/jcem-73-6-1276
Wilson, H. R. (1997). A neural model of foveal light adaptation and afterimage formation. Vis. Neurosci. 14, 403–423. doi:10.1017/s0952523800012098
Wong, K. Y. (2012). A retinal ganglion cell that can signal irradiance continuously for 10 hours. J. Neurosci. 32 (33), 11478–11485. doi:10.1523/JNEUROSCI.1423-12.2012
Wong, K. Y., Dunn, F. A., and Berson, D. M. (2006). Photoreceptor adaptation in intrinsically photosensitive retinal ganglion cells. Neuron 48 (6), 1001–1010. doi:10.1016/j.neuron.2005.11.016
Zhang, Jiaxiang (2013). Circadian rhythm modeling, estimation and control based on dynamic lighting[D]. New York: Rensselaer Polytechnic Institute.
Keywords: light adaptation, ipRGCs, non-image-forming effect, pupillary light reflex, complex luminance distribution, action spectrum
Citation: Wang S and Zhao J (2022) New prospectives on light adaptation of visual system research with the emerging knowledge on non-image-forming effect. Front. Built Environ. 8:1019460. doi: 10.3389/fbuil.2022.1019460
Received: 15 August 2022; Accepted: 27 September 2022;
Published: 10 October 2022.
Edited by:
Wenye Hu, The University of Sydney, AustraliaReviewed by:
Peng Xue, Beijing University of Technology, ChinaPimkamol Mattsson, Lund University, Sweden
Copyright © 2022 Wang and Zhao. This is an open-access article distributed under the terms of the Creative Commons Attribution License (CC BY). The use, distribution or reproduction in other forums is permitted, provided the original author(s) and the copyright owner(s) are credited and that the original publication in this journal is cited, in accordance with accepted academic practice. No use, distribution or reproduction is permitted which does not comply with these terms.
*Correspondence: Shuxiao Wang, d2FuZ3NodXhpYW80MTdAMTYzLmNvbQ==