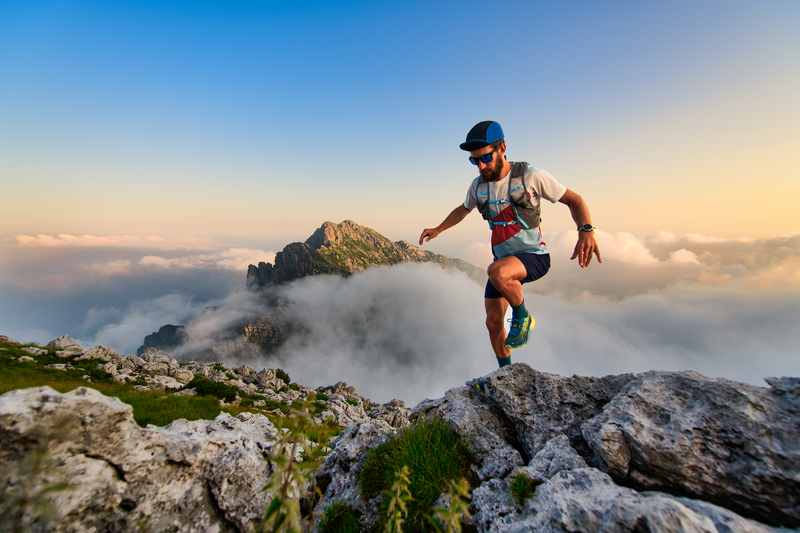
95% of researchers rate our articles as excellent or good
Learn more about the work of our research integrity team to safeguard the quality of each article we publish.
Find out more
ORIGINAL RESEARCH article
Front. Built Environ. , 16 July 2020
Sec. Transportation and Transit Systems
Volume 6 - 2020 | https://doi.org/10.3389/fbuil.2020.00106
This article is part of the Research Topic Advances in Planning for Emerging Transportation Technologies: Towards Automation, Connectivity, and Electric Propulsion View all 6 articles
In recent years, our surface transportation infrastructure is suffering from overuse, extreme traffic congestion, and roadway disrepair. Instead of following the traditional infrastructure expansion policy, current transportation research focuses on developing innovative and novel solutions to the aforementioned issues. Current pathways to overcoming these issues include the gradual transition toward a number of emerging transportation technologies, such as, autonomous motor vehicles for human transport, as well as unmanned aerial vehicles (UAV's) and “drone” technologies for surveillance, and package deliveries. However, as a long-term solution, transportation scientists are also investigating the once-seemingly futuristic notion of flying car technology—a convergent form of ground/air vehicle transportation, and assessing associated regulations. In this paper, an extensive review of current literature is conducted to explore the technological capabilities of flying cars—each requiring appropriate regulations and governance—to become fully sustainable. Specifically, issues pertinent to training, safety, environment, navigation, infrastructure, logistics/sustainability, and cybersecurity and human factors are explored. This paper concludes with a preliminary quantitative analysis exploring the public perceptions associated with flying cars—including anticipated benefits, concerns, and willingness to both hire and acquire the technology once available to consumers. Insights offered by this data will help inform next-generation policies and standards associated with the gradual advancement of flying cars.
The “Transportation network of Tomorrow” has long been a topic of discussion and debate, with numerous forward-thinking possibilities [e.g., Hyperloop and Personal Rapid Transit; (Cunningham, 2017)]. Since the depictions of flying cars were mostly confined in the science fiction movies, the notion of a real “Flying Car” has long-seemed nearer to science fiction than science fact. However, recent technological advances are slowly bringing these capabilities closer to reality (Covington, 2018). The surmised advantages of a Flying Car network are many, as it effectively combines ideal characteristics of both planes and cars. Specifically, a Flying Car is much more maneuverable and would be less susceptible to traffic jams while traversing three dimensional airspace as compared to two dimensional ground-based roadways (Soffar, 2018). However, regardless of the superior transportation capabilities likely to be offered by this technology, the widespread adoption of flying cars will be predominantly shaped by public perception. Evaluation and statistical analysis of public perception toward a forthcoming transportation technology pose significant methodological challenges in terms of unobserved heterogeneity and temporal instability (Mannering and Bhat, 2014; Mannering et al., 2016; Fountas et al., 2018; Mannering, 2018). A number of recent studies have demonstrated that people's perception toward potential benefits and concerns from the future use of flying cars, as well as the associated safety and security issues are multifaceted, and influenced by a broad range of socio-demographic factors (Eker et al., 2019, 2020a). In addition, whether general population is willing to embrace and pay for flying cars as personal vehicles and/or as a shared mobility service are major research questions that have been investigated as well (Ahmed et al., 2019; Eker et al., 2020b). In addition to survey-based approaches, virtual and/or live motion and simulation (M&S) based approaches are warranted for in-depth investigation of safety-, infrastructure-, sustainability-, environment-, and human factor-specific requirements (as shown in Figure 1).
In this context, the ongoing evolution of Flying Cars will have profound impacts upon various policies and standards that govern future development, test, evaluation, validation, and deployment of the technology (Lineberger et al., 2018). Forecasting existing regulations and establishing appropriate incentives that will serve to standardize and sustain a full-scale Flying Car Transportation network will be required. In the next section, an overview highlighting the applicability and potential impacts of M&S toward the future deployment of flying cars in the existing transportation fleet is presented.
Modern technological developments demonstrate that flying cars may be available for commercial use by 2025 (Becker, 2017; Bogaisky, 2018). Many of the associated challenges to sustain the technology will necessitate virtual and/or live M&S for testing and validation. For example, the evolution of flying cars will demand new policies and standards to regulate transition and handoff periods between manual and autonomous vehicle control and the complex transition between ground and flight dynamics (e.g., for takeoffs/landings). Furthermore, new policies and standards will be required to explore the complexities of airborne navigation safety, which will necessitate both computational M&S for virtual testing and physical M&S performed within a live setting. For the latter, prototyping (e.g., within a “drone dome” enclosure; refer to Figure 2) must be leveraged to emulate a functional miniature-scale infrastructure for forecasted flying car transport modes. Flying car deployment will likewise have profound impacts on training, which will demand novel regulations for safe operational and maintenance procedures. The ongoing development of flying car technologies will enable next-generation training methods within related technological domains, including: (a) pilot training and certification, (b) repair/service/upgrade procedures, (c) connected/automated vehicles, including advanced robotics and sensor fusion, and (d) machine learning and artificial intelligence (AI). Lastly, human response to autonomous features of next-generation transport modes remains uncertain. Through application of M&S, an improved understanding of the complex human factors associated with flying cars is required to manifest policies and standards that will govern future operation. Ultimately—human behavioral patterns ascertained (e.g., via human behavior models and simulations) in conjunction with live/virtual testing to explore the human-machine interface can be leveraged to clarify the infrastructure challenges associated with real-world deployment.
In this paper, we present an extensive overview of the capabilities and requirements for actionable regulations and governance for flying car technology to advise and dictate future test, evaluation, validation, and deployment of the technology. A brief forecast of the primary issues pertinent to key M&S domains of interest includes:
The most critical segment of flying car operation will be ground/air transitions (takeoff/landing), which will demand NAS/FAA regulation, and suitable governance for an integrated (rather than segregated) airspace. Another critical aspect would be addressing operational challenges and ensuring safety during adverse weather conditions (e.g., heavy rainfall, high wind, snowstorm, etc.).
For both manual and autonomous flying cars, the vehicle operator (or pilot), and the air/ground-based support systems (maintenance) will require appropriate certifications and governance.
Flying cars will require regulations for “vertiports” (takeoff/landing facilities) for land/air transitions, and this in turn, will dictate policies and standards for vertical takeoffs and landings operational features.
Governance must be mandated (e.g., NASA UAM) to ensure environmentally conscious best practices for flying cars. For instance, fully electrical powered operation, minimum operational noise, and minimum greenhouse gas emission.
Flying cars will require sustainable legal standards for operation, maintenance, control, and step-by-step adoption (e.g., as emergency vehicles, as a mode of ridesharing service, and as consumer vehicle).
Flying cars will be highly automated, computerized, and likely be connected to encrypted network for navigational purposes. Such a system will mandate policies for safeguarding against cybercrimes (e.g., unauthorized remote access through Trojans and malwares, DDoS attacks preventing network access).
Human preferences and attitudes will direct and dictate flying car sustenance, including financial (i.e., acquisition expenses; willingness to hire), operational benefits/concerns, and anticipated Use Case scenarios.
We begin with an overview of policies and standards related to safety (i.e., operational; mechanical)—a foremost concern for establishing and maintaining flying car sustainability.
Beginning with the M400 SkyCar (Moller, 2016), development of flying car technologies has been ongoing since the early 1980's, and numerous manufacturer technologies [PAL-V, 2019; e.g., (Aurora Flight Sciences, 2019)] are already beyond conceptual design. With the popularity of drones and UAV's steadily on the rise, and with associated demand for policies to support commercial application, flying cars are slowly inching toward reality. If critical regulatory obstacles can be overcome, passenger drones and flying cars could begin to be operational in the next decade (Lineberger et al., 2018). Obviating safety concerns (both human and autonomous) associated with flying car technology is therefore of paramount importance. As with autonomous ground vehicles, any publicized adverse safety incidents (e.g., Garsten, 2018) can taint public perception (Haboucha et al., 2017; Hulse et al., 2018; Sheela and Mannering, 2019), and limit the growth rate of consumer acceptance.
The most challenging questions regarding flying cars involve suitable procedures for going airborne (takeoffs) and returning to the ground (landings), and requirement of a complex safety risk analysis to determine the logistics of how flying cars should be regulated by the National Airspace System (NAS), the governing entity for United States airspace (Del Balzo, 2016). From a regulatory standpoint, much additional research is required to ensure that novel autonomous systems to operate, navigate, and control flying cars are equipped with redundancy (backup system), and have “safe mode” capabilities (i.e., “on-the-fly” decision-making) if they encounter unusual situations. Airspace logistics may further dictate that the primary regulatory body (i.e., the FAA) will assign minimum safety standards, and then each individual State would then mandate its own private air traffic controllers (Niller, 2018).
Ensuring operational safety during adverse weather conditions (e.g., snowstorm, heavy rain, high wind, etc.) is another critical safety aspect. Simulation and live testing to determine the thresholds of safe operational environment in terms of visibility, wind speed, precipitation intensity, etc. for different flying car types will be required to form the necessary regulations.
As outlined earlier, advanced models and simulations—in both live and virtual contexts—will be required to prototype common modes of flying car operation to establish baseline Safety guidelines. Additional notional specifics are offered throughout this paper, and in the next section, regulatory requirements for pilot training and certification are discussed.
As flying cars will involve airborne egress (i.e., aviation), regulations will be mandated by the Federal Aviation Administration (FAA) with a conservative Safety Management System (Federal Aviation Administration (FAA), 2016) to govern and manage effective risk controls (Del Balzo, 2016). For traditional aircraft, the FAA has a successful regulatory system for pilot licenses, aircraft certification and registration, takeoff and landing sites (airports), and a mechanism for air traffic control. With the anticipated introduction of flying cars, traffic control systems will have to accommodate for added complications, and compared to smaller drones, the path to regulating human flight will be challenging and time consuming (Stewart, 2018). For a ground vehicle, one requires separate driver's licenses to operate a sedan vs. a motorcycle vs. a multi-axle semi-truck. Conversely, a flying car operator will require licensure both to drive and fly, and will require appropriate vehicle registration and Type Certification. Proposed flying car technologies are essentially fixed-wing airplanes (e.g., the Aurora PAV), but others operate more as a motorcycle-gyrocopter hybrid (e.g., the PAL-V). Ultimately, certain proposed vehicles will operate as a car with wings (i.e., a flying car), while others will effectively serve as an airplane with wheels (i.e., a driving plane), which complicates regulatory matters relevant to the requisite skill of the flying car “operator,” as well as matters related to certification, airworthiness, and licensure (Del Balzo, 2015).
A wide range of flying car types are forecasted to eventually be allowed to operate within large, metropolitan areas. As such, their sustenance will largely depend on Certification procedures, which will dictate the urgency and tempo of this emergent, and disruptive technology as it evolves. Preliminary versions of flying cars will likely have a driver/pilot on board for the flight segment(s) of the journey. However, technologists are already developing concept models for future flying car models that will be remotely piloted and supervised either: (a) by live humans on the ground, or (b) by autonomous systems in the air and/or on the ground. To operate “urban air mobility (UAM)” vehicles (either with or without passengers) without a pilot would depend not only on the Certification of the vehicle, but likewise on the Certification of pilots and support systems on the ground—for which suitable policies have not yet been established (Thipphavong et al., 2018). Ultimately, advanced (virtual) M&S will be required to specify appropriate training systems (with suitable fidelity), and design standardized training scenarios for future flying car operators—particularly for handling complex ground-air and air-ground transitions. Regulation of air traffic issues across all governing bodies will be a unique and complex challenge. Accordingly, in the next section, a number of key policies and standards issues related to infrastructure and navigation are investigated in greater detail.
The navigational benefits of instituting a functional flying car network are obvious—a technology that allows civilians to transport from source to destination at a fraction of the overall time required to drive the same distance. Refer to Figure 3, which illustrates a sample journey that compares drive/flight times for a work commute. Here, the estimated 20 min drive path (shown in red) is constrained by 2D roads, ground congestion, and the natural limitations of land topography. The flight path (shown in green) obviates these constraints, and reduces the point-to-point straight path travel distance by ~2/3 (i.e., to 7 min). In this scenario, the prevalence of infrastructures that would permit safe takeoffs and landings, as well as infrastructure for vehicle storage (e.g., parking) is assumed. Naturally, such a vast network of vertical takeoff and landing facilities, or “vertiports” would necessitate standards and certifications for our infrastructure (e.g., helipads installed atop large public buildings; large segments of flat land designated for air-ground transitions) (Lineberger et al., 2018). Design, layout, and specification of such vertiports will require advanced M&S (e.g., Monte Carlo simulations and advanced heuristic optimization techniques) to guarantee human safety and likewise maximize operational effectiveness and efficiency. Accordingly, transportation authorities must mandate that flying car operators are constrained to selected flight corridors, such that a direct route might not always be an option. These corridors would likely be strategically located over reduced-risk areas of land that have minimal population (Roberts and Milford, 2017).
A related consideration is the need to regulate and mandate a functional range of motion for a flying car. Suitable design specifications will rely upon live and virtual testing, and M&S to determine technical standards that meet all functional requirements, and are likewise cost effective and sustainable. For example, we presume that in standard operational mode, the bottom of the vehicle is oriented downward (i.e., along the +Z axis), and it can traverse vertically while having the capacity to “hover,” and likewise remain stationary while airborne. Furthermore, we presume that flying cars would travel longitudinally (i.e., along an X-axis), and laterally (i.e., along a Y-axis) without having to orient the vehicle in that direction. Flying cars, like aircraft, will thus require rotational motion: to bank (roll), to tilt (pitch), and to revolve (yaw) to establish orientation within a plane parallel to the ground (World building, 2016). There will likely be situations where extended horizontal runways are not geometrically feasible, and will require a vertical takeoff and landing (VTOL) capability. Ridesharing companies (e.g., Uber and Lyft) are forecasting VTOL vehicles that are easier to fly than helicopters (Stewart, 2018), and have a “segregated airspace” dedicated for and managed by ridesharing entities. However, Federal regulators will likely mandate long-term policies involving a holistic integrated airspace, where everyone shares the skies (Stewart, 2018). Accordingly, idealizations of flying cars are such that they have the approximate size of a car, can drive on the road like a car, but also have VTOL capabilities.
Reliance on present-day battery science will be a limiting operational factor, as power constraints will dictate a brief (e.g., 10–20 min) flight duration prior to re-charge (Rathi, 2018). Uber (Uber, 2016) likewise concluded that batteries are not yet sufficient in terms of energy density, cycle life, nor cost-effectiveness, but supposes near-term improvement with economies of scale. A successful flying car engine is likely to be one that can successfully separate the source of rotational force from the speed of rotation (e.g., a “Split Power” engine Yeno, 2018). Commercial stakeholders, federal/state policymakers, and regional urban planning authorities therefore must envision an infrastructure that fully enables 3D egress within a densely populated (airborne) transportation grid. Likewise, to create a unified traffic management system, infrastructure for high-speed data communications and geolocation will be required along predefined flight corridors (World building, 2016). To this end, suitable policies and regulations will be required to establish guidelines to insure that scalability and operational efficiency are accounted for as a functional Flying Car network evolves.
Finally, to operationalize flying car aeronautics, policymakers and regulators must consider the in-vehicle user interface that will be required for flying car navigation. Instead of “floating” intersections, lane markers, and roadway signage—computer graphics technologists, virtual reality (VR)/Gaming enthusiasts, and M&S subject-matter experts are already evaluating and prototyping next-generation standards for flying car Heads-Up Display (HUD) navigation systems to support personal air travel (Frey, 2006). Such interfaces require customizable applications to permit airborne lane changes, and likewise, the augmented reality (AR) display would feature traffic information that will assist with safe navigation of changes in heading (i.e., turns). Policymakers therefore must establish guidelines for a robust human-machine interface such that on takeoff, the field-of-view will transform seamlessly into a display system appropriate for use in flight mode (AeroMobil, 2019).
Although UAV's were initially marketed as purely recreational devices, the prospect that passenger drones might soon be transporting civilians across large cities and vast rural landscapes (Ratti, 2017) has obvious advantages. However, it is difficult to fully comprehend the far-reaching environmental impacts likely to be imposed by flying cars, and flying car-based ridesharing services. Although flying cars will presumably be a clean (i.e., partial- or full-electric power) mode of human transport, a substantial fleet of such vehicles could demand substantial energy resources and appreciably increase the overall amount that humans travel. In this context, extensive research on self-driving vehicles demonstrated that due to the mobility convenience offered, personally owned self-driving cars would almost invariably increase the total vehicle miles traveled (VMT), which translates into significant increase in energy demand and emission, and perhaps increased congestions in roadways (Fagnant and Kockelman, 2015; Zhang et al., 2018). Self-driving vehicles may yield sustainable environmental benefit in terms of overall VMT reduction and greenhouse gas emission reduction only if they are deployed as shared mobility services (Fagnant and Kockelman, 2018). Environmental implications of electric vehicles (EVs) is also extensively investigated in the literature, and majority of the findings suggest that EVs would yield sustainable reduction in greenhouse gas emission only if the electricity production relies on renewable energy sources (hydro, nuclear, wind, solar, geothermal), instead of fossil fuels (Granovskii et al., 2006; Richardson, 2013). With the preceding findings regarding self-driving and electric vehicles in mind, life-cycle assessment of flying cars under different operational scenarios such as personal ownership, shared mobility service, and a mixture of both is warranted. In addition, environmental impact assessment under different energy sources, and propulsion systems is another significant direction toward future research. In this regard, findings from a recent study demonstrated the potential of flying cars in reducing greenhouse gas emission in a specific usage scenario, when compared against combustion engine based, and battery electric engine based personal vehicles (Kasliwal et al., 2019). However, to date, there have been no extensive analyses conducted upon flying cars that have attempted to quantify their systemic impact on the existing transportation network and environment as a whole (Stone, 2017). In this section, how flying cars might impact daily existence within highly urbanized environments, along with a dialogue concerning anticipated policy modifications, are explored.
Based upon the anticipated operational dynamics of flying cars, energy requirements are forecasted to be substantial. It is widely assumed that many flying car designs will require rotors, which are essentially large fans that force air downward to generate an upward propulsion. It will be difficult or impossible to achieve this lift force without creating air disturbance—and associated noise. As discussed previously, novel and substantial modifications to existing infrastructure must be governed to enable safe takeoffs and landings (with VTOL capabilities), as well as vehicle parking/storage. However, highly urbanized areas (e.g., New York City) already have substantial problems regulating aircraft noise. Recent noise complaints for residential helicopter tours along the Hudson River have resulted in increased regulation for tour operators (Bellafante, 2014), when prior to this legislation, there were fewer than 5,000 tourist helicopter flights per month. Extrapolating the prospect that flying cars could potentially serve as a daily transport mechanism for the ~8 million residents of metropolitan NYC, it becomes readily apparent that appropriate regulations (e.g., maximum sound decibels, at certain times-of-day and days-of-week, and within an appropriate distance of densely populated areas) will be required to inform a comprehensive noise ordinance to advise sustainable flying car operation (Ratti, 2017).
In addition to noise concerns, governance and oversight must be established to ensure that a network of flying cars will not result in undue burden of the existing Air Traffic Control (ATC) system. NASA's ongoing Urban Air Mobility (UAM) project aims to develop an efficient air transportation network for unmanned package delivery as well as manned flying passenger taxis within both rural and heavily urbanized regions (Thipphavong et al., 2018). UAM researchers are considering aeronautics issues to mitigate noise concerns associated with flying car operation, and are partnering with the FAA to develop rules and procedures that can manage the anticipated low-altitude operation of flying cars (Salazar, 2018). Finally, the capability of the technology to reduce reliance on fossil fuels, and tailpipe emissions measured as carbon dioxide equivalent or CO2e (Tischer et al., 2019; Union of Concerned Scientists USA, 2019) will help to establish the long-term sustainability of flying cars. It is reasonable to presume that through the application of e.g., human behavior modeling and discrete event simulation, this transportation analysis infographic is scalable to hybrid-style (flying car) vehicles that are capable of both driving and flight. Future policies and regulations (e.g., those governed by The Environmental Protection Agency, or EPA) will therefore demand that flying cars must comply with federal emissions and fuel-economy standards (Negroni, 2012).
Emergent flying car technologies will need to meet the technical and safety standards of both cars and airplanes, and at least initially, will be costly both to acquire and to maintain. In addition, the manner in which complex control devices are currently employed to direct and monitor road safety, allowable flight routes for flying cars will need to be mandated and regulated in a similar fashion. Likewise, as flying cars will exhibit exponential complexity in terms of vehicle design (e.g., propulsion/engine) and achievable speeds that are much faster than standard cars, it will be a major and multi-faceted challenge for policymakers to institute sustainable legal standards (e.g., operation, maintenance, control) for such vehicles (Soffar, 2018). In addition, from manufacturer's and commercial operator's point of view, an optimal balance between energy capacity (gasoline and/or battery), and speed-range combination for flying car production models would be a multidisciplinary challenge.
Technologists (e.g., Templeton, 2018) forecast that adoption logistics for flying cars will transpire in a staged manner, initially, to meet our most critical transportation requirements. Driven by regional/national policies and regulations, one could envision a gradual deployment scenario beginning first with adoption by specialty vehicles (e.g., law enforcement, construction, emergency fire response, ambulances), followed by ridesharing companies, and eventually followed by civilians. For example, a limited fleet of self-operating flying ambulances could be effective at quickly transporting a patient, along with a health professional and essential supplies, in a manner that is non-disruptive to traffic on the ground. Likewise, in certain situations, if the transport was completely without a paramedic onboard to tend to a patient, it might ultimately be a better choice to fly (i.e., above the traffic) for ~5 min than to have the commute consume 15 min (by ground) driving in a large vehicle with full gear and support team. Note that despite the idealized and academic expectation that flying car technologies should originate through emergency responders, a logical argument can be made that preliminary deployment might instead be driven by industry giants with substantial financial interests (e.g., Amazon, for package delivery; Uber, for consumer ridesharing applications). Regardless, proposed vertiports will require design standards (e.g., layout, features, geometries)—as advised by advanced M&S (e.g., multi-resolution models and macro/micro-simulations) to accommodate flying and landing hundreds of aircraft. Likewise, regulations for the associated airspace requirements to enable takeoff and landing patterns will be mandated.
Lastly, manufacturing challenges may inhibit the sustainability of flying cars as economies of scale will demand many aircraft flying as soon as possible. Leveraging advanced (e.g., lightweight, strong composite) volume-based manufacturing methods from automotive to aviation is required. However, it is anticipated that this transition will be a gradual process over time (Adams, 2018). From operational perspective, due to the complex engineering nature of flying cars, safety-certified, passenger-carrying flying vehicles will heavily rely on computers and autonomy. However, autonomous systems tend to lack the judgment, situational awareness, and instantaneous interventions often required from live human pilots—and will demand an extended period for development of regulatory standards.
It is forecasted that flying car operation will rely heavily upon computational AI for Detect and Avoid (DAA) technologies to recognize, distinguish, and track other aircraft, predict conflicts, and take corrective action as required. To realize such functionality will demand cognitive systems and computing; platforms that encompass machine learning/reasoning, human-machine interaction/automation, and network sensors for seamless and real-time vehicle-vehicle and vehicle-infrastructure communications. Beyond the prevailing safety concerns associated with a major system malfunction while flying over a densely populated area, we still lack a comprehensive understanding of how flying cars can be protected from hackers, terrorists, or other cyber criminals (Ratti, 2017). The establishment of cybersecurity policies and standards will be a major requirement for fully realizing flying cars sustainably.
Many present-day Communications, Navigation, and Surveillance (CNS) systems will require expansion to cover additional airspace requirements for flying cars. Fortunately, NASA (and other agencies) are developing operational policies for Urban Air Mobility (UAM) related to aircraft, airspace, and hazards, and to include provisions for security. As flying cars will drastically enhance the overall mobility of persons and goods within metropolitan regions, our air traffic management system must assign protocols for cybersecurity to assure reliable exchange of data (e.g., vehicle, navigation, command/control (C2) link, weather), and novel authentication mechanisms will be required to detect intrusions and data leaks (Thipphavong et al., 2018). Instatement of cybersecurity standards will be required to protect vehicle interfaces from attacks (both physical and electronic) to the networks that control flying cars. Stochastic M&S (Pokhrel and Tsokos, 2017) will be mandated to predict, quantify and assess risks to the overall network which will help to inform appropriate countermeasures. Cyber criminals have previously demonstrated the relative ease with which ground vehicles can be compromised after identifying access to its internal operating system (i.e., the Controller Area Network, or CAN bus). Accordingly, cybersecurity specialists for flying cars must impact policies for safeguarding against malwares and Trojans that attempt unauthorized remote access to its Electronic Control Unit (ECU) (Tabora, 2018). In the next section, a brief discussion concerning the critical human factors that interrelates to all of the relevant subdomains discussed so far is presented, which will drive and dictate the near-term adoption of flying cars.
In addition to the various technological policy and regulatory requirements summarized thus far, we must forecast the critical human element associated with our relationship with flying cars (i.e., the Human-Machine interface). For the technology to sustain, humans will be required to overcome psychological, attitudinal, perceptual or behavioral barriers (Fountas et al., 2019, 2020; Pantangi et al., 2019) that are associated with the concept of flying a car, or longer-term, being transported within a pilotless and fully autonomous flying vehicle. Furthermore, for flying cars to be widely accepted and adopted, they will have to be as flexible and convenient for daily transport as a modern-day automobile and quickly establish well-documented safety records (Lineberger et al., 2018). A survey was conducted to investigate the human factors associated with flying car technologies. It was conducted in an online platform called SurveyMonkey, and a total of 692 respondents from 19 different countries participated in the survey. A number of exploratory studies have been conducted so far, based on the data collected in the aforementioned survey (Ahmed et al., 2019; Eker et al., 2019, 2020a,b). Here, we briefly summarize and illustrate the key issues investigated in the aforementioned works, as they will directly influence future policies and regulations associated with emergent technological advances.
The first analysis (Eker et al., 2020b), provides a preliminary investigation of individuals' perceptions regarding the future adoption of flying cars. Figure 4 illustrates willingness to pay to purchase a flying car for personal use, forecasting what is expected to be common price points for this mode of transport. Just over 40% expressed an interest in acquiring a flying car vehicle at a ~$100k purchase value, and these numbers decline sharply with increased dollar amounts. In Figure 5, the anticipated use case scenarios for flying cars across three subcategories are explored: activity, duration of travel, and time-of-day. The figure illustrates the forecasted use of flying cars most often for entertainment and work activities; respondents seem more likely to use the technology for trips of longer duration (i.e., hundreds of miles) as opposed to short trips, and perhaps not surprisingly, remarked as being slightly more likely to use flying cars during daylight (i.e., morning/afternoon) periods than during darkness.
The second analysis (Eker et al., 2020a) provides a preliminary investigation in to the public perceptions of forecasted flying car technologies. Specifically, this effort explores the fact that the future adoption of flying cars is directly associated with individuals' perceptions of the benefits and concerns arising from key operational characteristics related to this complex and technologically disruptive technology. Figure 6 illustrates the anticipated benefits of flying car technologies, where respondents anticipated the potential of reduced travel time, and increased travel time reliability (e.g., reduced traffic), while being comparatively less anticipatory of the possible gains resulting from reduced fuel expenses and vehicle emissions. Likewise, Figure 7 illustrates the fundamental concerns for eventual flying car deployment, where respondents seemed most apprehensive about weather conditions, and more concerned about airborne (compared to ground) interactions with other vehicles, while somewhat surprisingly, expressing reduced concern regarding the forecasted requirement to fly one's own flying car.
Finally, the third analysis (Ahmed et al., 2019) explores human willingness to hire next-generation flying car based ridesharing services. This study investigates human perceptions and expectations involving flying cars with specific regards to shared mobility services, previously unexamined within travel demand literature. Figure 8 illustrates human preferences toward flying car based ridesharing service. The graph shows that the willingness toward human-driven flying cars is slightly bigger than that of fully autonomous counterparts. Figure 9 illustrates human expectations regarding the cost of flying car based ridesharing service. It shows that humans are willing to pay slightly more than current ground-based rates for ridesharing services. However, the current threshold for tolerated increase is slight, as indicated by the 4th order polynomial “trend line” displayed on the plot.
The discussion on the seven key domains of interest presented in this paper provides an overview of the challenges that need to be addressed for the successful integration of flying cars as a new mode in the existing transportation infrastructures. With safety and human behavioral related challenges being of utmost importance, recommendations and directions for future work are discussed below.
A well-balanced regulatory framework for flying cars is ideally the first step toward ensuring safety for all stakeholders (from passengers, to operators, to public or private infrastructure owners). With an objective to form a baseline for regulations and security measures, Eker et al. (2019) evaluated the feasibility of four security measures in terms of public acceptability and trust on the measures. These measures are: (a) use of existing FAA regulations for flying car air traffic control; (b) establishing air-road police force with flying police cars; (c) detailed profiling and background checking for flying car owners and operators; and (d) establishing no-fly zones for flying cars near sensitive locations, such as military bases, power/energy plants, government facilities, and major transportation hubs, to name a few. Findings from this study revealed that the majority of the participants had positive inclination toward these four measures (61%, 71%, 75%, and 79%, respectively). This makes the proposed measures ideal as a regulatory and policy starting point. By making appropriate safety-related adjustments, effective measures and regulations can be derived by the regulatory and legislative authorities.
Technological progress in flying car development is rapidly accelerating across the world, reaching an increasingly wider audience over time. Exposure to this information is expected to affect public perceptions toward flying car technologies. In this context, continuous assessment of public perception toward several aspects related to flying cars is warranted. A few relevant examples related to uncharted thematic topic that are specific to flying cars include willingness to use, willingness to pay, opinions regarding various deployment scenarios, perception toward potential benefits and concerns, effects on environment, and transformational effects on urban settings, to name a few. Such assessment should also take place at a micro level, with specific focus toward different geographic regions, and different socio-economic and demographic target audience groups. The outcomes from such assessment would ultimately aid the stakeholders (manufacturers, operators, legislative, and regulatory entities) to amend their respective plans, roadmaps and policies.
As our surface transportation infrastructure continues to suffer from overuse, congestion, disrepair, transportation scientists are already investigating the feasibility of passenger drone and flying car technologies. For these reasons, we have presented an extensive literature-based overview of the emergent capabilities of flying car, and critically—their requirement for actionable regulations and governance to advise and dictate future test, evaluation, validation, and deployment.
In this paper, we emphasized seven key M&S domains of interest (Safety, Training, Infrastructure, Environment, Logistics/Sustainability, Cybersecurity, and Human Factors) critical to the forecasted advancement of flying cars, and explored how these technologies will influence future policies, regulations, certifications, and governance. Moving forward, an excellent direction toward future research would be the development of a high-fidelity M&S framework—including both live and virtual testing aspects—to examine the emerging operational feasibility of flying cars. Such a capability will allow technologists and subject matter experts to prototype and validate ground/air traffic simulation-tools, and enable researchers to model and analyze complex egress scenarios within diverse operational settings. We anticipate that live physical test environments will be necessary to perform advanced scenario prototyping, once baseline feasibility has been achieved through virtual simulation. The outcomes of such M&S frameworks will further serve to influence policymakers and service providers toward achieving sustainable technological policies and standards.
The datasets for this study are available on request to the corresponding author.
All authors contributed in the preparation and the completion of the submitted paper.
UE was employed by Turkish Airlines.
The remaining authors declare that the research was conducted in the absence of any commercial or financial relationships that could be construed as a potential conflict of interest.
The contents of this paper reflect the views of the authors, who are responsible for the facts and the accuracy of the data presented herein. The contents do not necessarily reflect the official views or policies of any agency, nor do the contents constitute a standard, specification, or regulation. A preliminary version of this manuscript was presented in the I/ITSEC 2019 Conference.
Adams, E. (2018). Four Reasons Why We Don't Have Flying Cars – Yet”, Wired. Available online at: https://www.wired.com/story/four-reasons-we-dont-have-flying-cars-yet/ (accessed June 15, 2018).
AeroMobil (2019). Interested in Flying Car? Human Machine Interface. Available online at: https://www.aeromobil.com/aeromobil-4_0-stol/, Copyright 2019. (accessed August 25, 2019).
Ahmed, S. S., Fountas, G., Eker, U., Still, S. E., and Anastasopoulos, P. C. (2019). An Exploratory Empirical Analysis of Willingness to Hire and pay for Flying Taxis and Shared Flying Car Services. Working Paper.
Aurora Flight Sciences (2019). PAV – Passenger Air Vehicle. Available online at: http://www.aurora.aero/pav-evtol-passenger-air-vehicle/ (accessed August 25, 2019).
Bellafante, G. (2014). Big City: That Nuisance in the Sky, The New York Times, Available online at: https://www.nytimes.com/2014/03/23/nyregion/taking-on-noisy-new-york-city-tourist-helicopters.html (accessed March 21, 2014).
Bogaisky, J. (2018). Your Flying Car May Be Almost Here, Forbes. Available online at: https://www.forbes.com/sites/jeremybogaisky/2018/05/24/your-flying-car-is-almost-here/#473eb37e5724 (accessed July 17, 2019).
Covington, P. (2018). Look Up: Flying Cars Are Coming Sooner Than You Think!, TriplePundit. Available online at: https://www.triplepundit.com/2018/11/flying-cars-are-coming-sooner-than-you-think/ (accessed August 25, 2019).
Cunningham, A. (2017). Public Transportation of the Future: Four New Sustainable Technologies, Building Design + Construction. Available online at: https://www.bdcnetwork.com/blog/public-transportation-future-four-new-sustainable-technologies (accessed August 25, 2019).
Del Balzo, J. (2015). Cars That Can Fly Will Challenge the FAA as to Certification & Operation!!!, JDA Journal. Available online at: http://jdasolutions.aero/blog/flying-cars-will-challenge-the-faa/ (accessed December 23, 2015).
Del Balzo, J. (2016). Some Thoughts on a Road Map to Faa Safety Rules for Flying Cars in the NAS, JDA Journal. Available online at: http://jdasolutions.aero/blog/faa-flying-car-safety/ (accessed February 23, 2016).
Eker, U., Ahmed, S. S., Fountas, G., and Anastasopoulos, P. C. (2019). An exploratory investigation of public perceptions towards safety and security from the future use of flying cars in the United States, Anal. Methods Accident Res. 23:100103. doi: 10.1016/j.amar.2019.100103
Eker, U., Fountas, G., and Anastasopoulos, P. C. (2020b). An exploratory empirical analysis of willingness to pay for and use flying cars. Aerospace Sci. Tech. 105993. doi: 10.1016/j.ast.2020.105993
Eker, U., Fountas, G., Anastasopoulos, P. C., and Still, S. E. (2020a). An exploratory investigation of public perceptions towards key benefits and concerns from the future use of flying cars, Travel Behav. Soc. 19, 54–66. doi: 10.1016/j.tbs.2019.07.003
Fagnant, D. J., and Kockelman, K. (2015). Preparing a nation for autonomous vehicles: opportunities, barriers and policy recommendations. Transp. Res. Part A 77, 167–181. doi: 10.1016/j.tra.2015.04.003
Fagnant, D. J., and Kockelman, K. M. (2018). Dynamic ride-sharing and fleet sizing for a system of shared autonomous vehicles in austin, texas. Transportation 45, 143–158. doi: 10.1007/s11116-016-9729-z
Federal Aviation Administration (FAA) (2016). Safety Management System. Available online at: http://www.faa.gov/documentLibrary/media/Order/FAA_Order_8000.369B.pdf (accessed August 25, 2019).
Fountas, G., Anastasopoulos, P.C., and Abdel-Aty, M. (2018). Analysis of accident injury-severities using a correlated random parameters ordered probit approach with time variant covariates. Anal. Methods Accident Res. 18, 57–68. doi: 10.1016/j.amar.2018.04.003
Fountas, G., Fonzone, A., Gharavi, N., and Rye, T. (2020). The joint effect of weather and lighting conditions on injury severities of single-vehicle accidents. Anal. Methods Accident Res. 27:100124. doi: 10.1016/j.amar.2020.100124
Fountas, G., Pantangi, S.S., Hulme, K.F., and Anastasopoulos, P. C. (2019). The effects of driver fatigue, gender, and distracted driving on perceived and observed aggressive driving behavior: a correlated grouped random parameters bivariate probit approach. Anal. Methods Accident Res. 22:100091. doi: 10.1016/j.amar.2019.100091
Frey, C. (2006). zeroG-Autobahn: A 3D Traffic and Navigation System and a Heads-Up Display Design System for Airborne Individual Transport. Available online at: http://www.zerog-autobahn.com/site/about/index.php.
Garsten, E. (2018). Uber Resumes Autonomous Testing On Public Roads Months After Fatal Accident, Forbes. Available online at: https://www.forbes.com/sites/edgarsten/2018/12/21/uber-resumes-autonomous-testing-on-public-roads-months-after-fatal-accident/#45bf13cf6422 (accessed December 21, 2018).
Granovskii, M., Dincer, I., and Rosen, M. A. (2006). Economic and environmental comparison of conventional, hybrid, electric and hydrogen fuel cell vehicles. J. Power Sour. 159, 1186–1193. doi: 10.1016/j.jpowsour.2005.11.086
Haboucha, C. J., Ishaq, R., and Shiftan, Y. (2017). User preferences regarding autonomous vehicles, Transp. Res. Part C 78, 37–49. doi: 10.1016/j.trc.2017.01.010
Hulse, L. M., Xie, H., and Galea, E. R. (2018). Perceptions of autonomous vehicles: Relationships with road users, risk, gender and age. Safety Sci. 102, 1–13. doi: 10.1016/j.ssci.2017.10.001
Kasliwal, A., Furbush, N. J., Gawron, J. H., McBride, J. R., Wallington, T. J., De Kleine, R. D., et al. (2019). Role of flying cars in sustainable mobility. Nat. Commun. 10:1555. doi: 10.1038/s41467-019-09426-0
Lineberger, R., Hussain, A., Mehra, S., and Pankratz, D.M. (2018). Elevating the Future of Mobility – Passenger Drones and Flying Cars, Deloitte Insights. Available online at: https://www2.deloitte.com/insights/us/en/focus/future-of-mobility/passenger-drones-flying-cars.html (accessed August 23, 2019).
Mannering, F. (2018). Temporal instability and the analysis of highway accident data. Anal. Methods Accident Res. 17, 1–13. doi: 10.1016/j.amar.2017.10.002
Mannering, F. L., and Bhat, C. R. (2014). Analytic methods in accident research: methodological frontier and future directions. Anal. Methods Accident Res. 1, 1–22. doi: 10.1016/j.amar.2013.09.001
Mannering, F. L., Shankar, V., and Bhat, C. R. (2016). Unobserved heterogeneity and the statistical analysis of highway accident data, Anal. Methods Accident Res. 11, 1–16. doi: 10.1016/j.amar.2016.04.001
Moller (2016). SKYCAR® 400 Four passenger VTOL aircraft”. Available online at: https://moller.com/moller_skycar400.html (accessed November 10, 2018).
Negroni, C. (2012). Before flying Car Can Take Off, There's A Checklist, The New York Times. Available online at: https://www.nytimes.com/2012/04/29/automobiles/before-flying-car-can-take-off-theres-a-checklist.html (accessed April 27, 2012).
Niller, E. (2018). Congress May Love Flying Cars, But the Skies Still Need Traffic Cops”, Wired. Available online at: https://www.wired.com/story/congress-flying-cars-regulation/ (accessed July 25, 2018).
PAL-V (2019). Explore the PAL-V. Available online at: https://www.pal-v.com/en/explore-pal-v.
Pantangi, S. S., Fountas, G., Sarwar, M. T., Anastasopoulos, P. C., Blatt, A., Majka, K., et al. (2019). A preliminary investigation of the effectiveness of high visibility enforcement programs using naturalistic driving study data: a grouped random parameters approach. Anal. Methods Accident Res. 21, 1–12. doi: 10.1016/j.amar.2018.10.003
Pokhrel, N. W., and Tsokos, C. P. (2017). Cybersecurity: a stochastic predictive model to determine overall network security risk using markovian process. Sci. Res. 8, 91–105. doi: 10.4236/jis.2017.82007
Rathi, A. (2018). Uber Will Bring You Flying Taxis, If You Can Help Build a Magical Battery, Quartz. Available online at: https://qz.com/1243334/the-magical-battery-uber-needs-for-its-flying-cars/ (accessed April 11, 2018).
Ratti, C. (2017). Flying Cars Are Impractical and Unnecessary. Here's why”, World Economic Forum. Available online at: https://www.weforum.org/agenda/2017/11/heres-why-policy-makers-need-to-think-twice-about-flying-cars, (accessed November 8, 2017).
Richardson, D. B. (2013). Electric vehicles and the electric grid: a review of modeling approaches, Impacts, and renewable energy integration. Renew. Sustain. Energy Rev. 19, 247–254. doi: 10.1016/j.rser.2012.11.042
Roberts, J., and Milford, M. (2017). The Future of Flying Cars: Science Fact or Science Fiction?, Singularityhub. Available online at: https://singularityhub.com/2017/05/10/the-future-of-flying-cars-science-fact-or-science-fiction/#sm.0000160eqpiywvfjzwiwvqjala58z (accessed May10, 2017).
Salazar, D. E. (2018). NASA and Uber Are Getting Serious About Flying Cars”, Space.com. Available online at: https://www.space.com/40553-nasa-uber-flying-car-simulation-plan.html, (accessed May 11, 2018).
Sheela, P. V., and Mannering, F. (2019). The effect of information on changing opinions toward autonomous vehicle adoption: an exploratory analysis. Int. J. Sustain. Transp. 14, 475–487. doi: 10.1080/15568318.2019.1573389
Soffar, H. (2018). Future Flying Cars Advantages, Disadvantages, Design, Types & Developments, Online Sciences. Available online at: https://www.online-sciences.com/robotics/future-flying-cars-advantages-disadvantages-design-types-developments/ (accessed August 23, 2019).
Stewart, J. (2018). Uber's Flying Car Plan Meets the Regulator It Can't Ignore”, Wired. Available online at: https://www.wired.com/story/uber-flying-cars-faa-regulation/ (accessed May, 2018).
Stone, M. (2017). Flying cars could happen, but they'll probably create more problems than they solve, greentechmedia. Available online at: https://www.greentechmedia.com/articles/read/flying-cars-might-happen-but-they-might-create-more-problems#gs.1nhtia (accessed June 12, 2017).
Tabora, V. (2018). Flying Cars, Taking to the Skies to Avoid Traffic”, Hackernoon. Available online at: https://hackernoon.com/flying-cars-taking-to-the-skies-to-avoid-traffic-3d0f7f6ce0a2 (accessed May 17, 2018).
Templeton, B. (2018). The Flying Car – and Flying Ambulance – Is Closer Than We Thought”, Brad Ideas/Robocars and More. Available online at: https://ideas.4brad.com/flying-car-and-flying-ambulance-closer-we-thought (accessed January 18, 2018).
Thipphavong, D. P., Apaza, R. D., Barmore, B. E., Battiste, V., Burian, B. K., Dao, Q. V., et al. (2018). Urban Air Mobility Airspace Integration Concepts and Considerations”, Nasa White Paper. Available online at: https://ntrs.nasa.gov/archive/nasa/casi.ntrs.nasa.gov/20180005218.pdf (accessed August 25, 2019).
Tischer, V., Fountas, G., Polette, M. and Rye, T. (2019). Environmental and economic assessment of traffic-related air pollution using aggregate spatial information: a case study of Balneário Camboriú, Brazil”. J. Transp. Health 14:100592. doi: 10.1016/j.jth.2019.100592
Uber (2016). Elevate - Fast-Forwarding to A Future of on-Demand Urban Air Transportation, Uber White Paper. Available online at: https://www.uber.com/elevate.pdf (Accessed October 27, 2016).
Union of Concerned Scientists USA (2019). How Clean is Your Electric Vehicle?. Available online at: https://www.ucsusa.org/clean-vehicles/electric-vehicles/ev-emissions-tool#.VqzQD_krKUm
World building (2016). Designing a Traffic System for Flying Cars. Available online at: https://worldbuilding.stackexchange.com/questions/32697/designing-a-traffic-system-for-flying-cars, posted (accessed January 5, 2016).
Yeno, C. (2018). Flying Cars Now a Reality with New Innovative Engine from Corporation of Flight Inc., PRNewswire. Available online at: https://www.prnewswire.com/news-releases/flying-cars-now-a-reality-with-new-innovative-engine-from-corporation-of-flight-inc-300770092.html (accessed December 21, 2018).
Keywords: flying car, emerging technology, challenges, concerns, willingness to pay, willingness to use, Urban air mobility
Citation: Ahmed SS, Hulme KF, Fountas G, Eker U, Benedyk IV, Still SE and Anastasopoulos PC (2020) The Flying Car—Challenges and Strategies Toward Future Adoption. Front. Built Environ. 6:106. doi: 10.3389/fbuil.2020.00106
Received: 22 March 2020; Accepted: 05 June 2020;
Published: 16 July 2020.
Edited by:
Sakdirat Kaewunruen, University of Birmingham, United KingdomReviewed by:
Jianhua Shao, Jiangsu University of Science and Technology, ChinaCopyright © 2020 Ahmed, Hulme, Fountas, Eker, Benedyk, Still and Anastasopoulos. This is an open-access article distributed under the terms of the Creative Commons Attribution License (CC BY). The use, distribution or reproduction in other forums is permitted, provided the original author(s) and the copyright owner(s) are credited and that the original publication in this journal is cited, in accordance with accepted academic practice. No use, distribution or reproduction is permitted which does not comply with these terms.
*Correspondence: Panagiotis Ch. Anastasopoulos, cGFuYXN0YXNAYnVmZmFsby5lZHU=
Disclaimer: All claims expressed in this article are solely those of the authors and do not necessarily represent those of their affiliated organizations, or those of the publisher, the editors and the reviewers. Any product that may be evaluated in this article or claim that may be made by its manufacturer is not guaranteed or endorsed by the publisher.
Research integrity at Frontiers
Learn more about the work of our research integrity team to safeguard the quality of each article we publish.