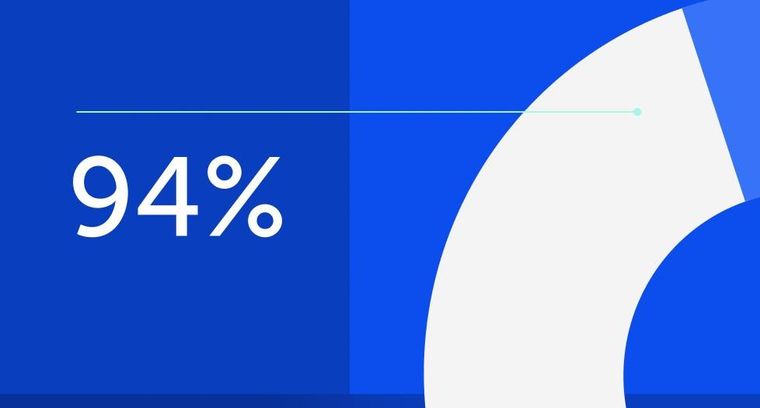
94% of researchers rate our articles as excellent or good
Learn more about the work of our research integrity team to safeguard the quality of each article we publish.
Find out more
ORIGINAL RESEARCH article
Front. Built Environ., 22 October 2019
Sec. Indoor Environment
Volume 5 - 2019 | https://doi.org/10.3389/fbuil.2019.00125
This article is part of the Research TopicRecent Advances in Seismic Risk Assessment and its ApplicationsView all 7 articles
Italian historical buildings do not respect often standard requirements established by National regulations, so that they need of an energy requalification. In addition, commonly the adopted provisions do not belong to an integral design approach in which different aspects, such as architectonic, energetic, and structural should be together considered for obtaining a total building rehabilitation. This work mainly addresses to the application of non-invasive methods for energy retrofitting of historical buildings in Italy. In particular, an energy audit protocol is studied and applied to a case study, consisting on an ancient masonry building located within the historical center of “Sassi of Matera,” recognized as UNESCO site since 1993. In the paper some energy efficiency measures are considered, by examining also alternative interventions with respect to the classical ones, by applying innovative and sustainable materials, fashionable as well from a structural point of view. This proposal, to be investigated more in detail in future, responds to the criteria of non-invasiveness for preserving the cultural heritage identity, and simultaneously improving the energy and the structural response.
The main policies and measures promoted by the UNFCCC (1997) are addressed to improve the energy efficiency in relevant sectors of the national economy and research, promoting, and developing a larger use of new renewable energy forms. As known, in Europe buildings are responsible for 68% of the energy consumption (Fredrizzi and Dipasquale, 2015), due to heating, cooling, hot water, cooking and appliances (BPIE, 2011). The improvement of energy efficiency entails benefits for the whole European economy and, even more, for local development. It is estimated that the direct benefits of energy savings, should achieve the 20% reduction target in 2020, corresponding to an amount saved of € 220 billion per year. To this it must be added that the energy efficiency, in accordance to the Direttiva 2002/91/CE (2002), has to be calculated using different methodologies at regional level considering, in addition to insulation, a series of other factors playing an increasingly important role, such as: the type of heating system, the use of renewable energy sources and the architectural features of a building.
In this framework, nowadays the energy retrofit of historic and traditionally constructed buildings is particularly important. These constructions, although not responding to the current standards, are still in use having also, very often, an artistical value such that they are classified and protected as cultural heritage (Andrus and Shrimpton, 1997; McClelland, 1997; English Heritage, 2008; Mazzarella, 2015). The importance of improving the energy efficiency of historic buildings has been also introduced by the Italian Ministry of Cultural Heritage and Activities and Tourism (MIBACT) with “Guidelines for the improvement of energy efficiency in cultural heritage” (MIBACT, 2015). This document is aimed to provide indications for assessing and improving the energy performance of the protected cultural heritage, with reference to Italian standards on energy saving and efficiency. Recently, these guidelines are also analyzed in detail in Negro et al. (2016) to verify their compatibility with the energy interventions realized in the ancient Matera Cathedral, belonging to the historic center of Matera, named “Sassi of Matera.” These guidelines are useful for designers because they provide a tool for assessing the energy performance of historic buildings in the existing conditions, as well as provide operational criteria for designing of any energy intervention.
It is important to remark that until some year ago, designers were able to design the energy system of a building through the steady-state regime referred to the conventional “external design temperature” for heating and air conditioning. The calculation of the energy requirement was always referred to monthly average conditions that, however, did not allow of realistically simulating the behavior of the energy system. On the contrary, as recently demonstrated dynamic simulation is an extremely advanced calculation method allowing to evaluate the energy performance of a building taking into consideration the inertial effects of building envelope and system (Filippi and Fabrizio, 2012; Rospi et al., 2017a). It should be remarked that in these cases the thermal regime is dynamic because of:
• the climatic parameters (temperature, relative humidity, solar radiation, wind speed, etc.), characterizing the territorial context in which the building surveyed is included, vary with hourly and sub-hourly intervals;
• all the microclimatic parameters and the internal systems (such temperature, relative humidity, system response, etc.) are time-variable dependent responding to instantaneous modifications of the external environmental parameters.
The objective of the dynamic calculation is to study, by varying the time, the real thermo-energetic behavior of a building determining, differently from the classical stationary calculation, the energy needs through detailed simulation methods. The thermal evolution of buildings is characterized by the behavior of external walls in conditions of thermal transient. From an operative point of view, the best approach is given by of the critical integration of different detection methods, both direct and indirect (photogrammetry, stereo photogrammetry, laser scanning). In historical buildings it is important to pay attention to the evaluation of the walls thickness, floors, vaults, and roofing. Likewise, it becomes essential to register, with particular attention, the presence of false vaults, false ceilings, overhangs, cavities, flues, niches, and discontinuities, either through direct surveys, or with indirect non-destructive investigations (e.g., thermography, ground-penetrating radar, sonic investigations) or weakly destructive (such as endoscopies, essays, sampling, etc.).
In this paper, some methods for assessing the energy performance of existing buildings are examined. Then, they are applied to a case study, an ancient masonry building placed within the historical center of Matera, recognized as UNESCO site since 1993. The procedure applied follows the indications reported in UNI ISO 9869-1 (2015), UNI EN ISO 6946 (2018), UNI EN ISO 10077-1 (2018) for energy assessment of the building investigated. The case study results interesting since it is still in service, and to date an experimental measuring campaign addressed to retrofit its energy performance results in progress. In addition, starting from the obtained results at first some interventions are considered in order to retrofit the building from an energetic point of view. Among these, innovative applications with sustainable materials are also discussed, analyzing the possibility of being applied also as structural interventions for upgrading as well the seismic capacity. As known, the latter aspect is ruled by Directive of the Italian Prime Minister1 and discussed, for instance, in Formisano et al. (2017). In this way an integrated approach for buildings rehabilitation may be achieved capable, through the application of innovative materials, of simultaneously upgrading both thermal and structural performances. In the future the new approach may be largely applied by substituting classical intervention strategies, usually independently conceived by each other and resulting, very often, also expensive. Moreover, due to the use of innovative materials, the proposed approach results environmentally and architecturally sustainable, too.
In this section, the methodology and the related tests applied for estimating the energy performance are illustrated. It is noted that a significant problem, especially for historic buildings, is the lack of data on plant and elevation, to which the lack of information on materials and their stratigraphy has to be added.
The transmittance values of opaque and transparent components of an existing building are not always known in advance. Their evaluation is not a simple operation because it depends on the difficulty of data acquisition and/or on the impossibility to directly verify the individual layers. Therefore, very often in situ measurements are required.
In general, it is possible to calculate building components transmittance by invasive and non-invasive processes. In particular:
• Invasive processes: among these endoscopy and extractions with cores should be mentioned. Their purpose is to identify the type of material and the thickness, and to calculate the thermal transmittance with the UNI EN ISO 6946 (2018). Critical point is represented by the actual thermal conductivity of the identified individual materials.
• Non-invasive processes: the transmittance of the structure is measured on site in accordance with UNI ISO 9869-1 (2015), by the means of a thermal flux-meter measuring the actual heat flow from which the real transmittance of the structure is obtained (Figure 1A).
Precisely, the thermal flux-meter is an instrument permitting to measure the thermal flux passing through a component in a non-invasive way. Energy survey with thermal flux-meter is particularly used to evaluate the transmittance of existing components, on the basis of redevelopment or energy refurbishment, or to verify for new buildings the actual performance. The instrumentation for this type of survey consists of an instrument able to measure and store data (data logger), a flow-meter plate (which will produce the thermal flow through the building component being investigated) and four contact probes (two internal and two external) for the wall temperatures measurement. The acquired measurements, after at least 72 h (UNI ISO9869-1, 2015), are then downloaded onto the operator's computer and processed using a dedicated software that will perform the direct calculation of the K coefficient, and the parameters related to thermal insulation using the “moving averages” method or the “black box” method.
The thermal flux-meter test, carried out after a thermographic inspection, is the most reliable test to evaluate the real thermal insulation of a component of the building envelope. The detection with the thermo-flowmeter can take place only when there is a certain temperature gradient (ΔT) between the two sides of the wall or of the structure in general (about and at least 8 ≈ 10°C). Therefore, it should be carried out preferably during the winter season when outside temperatures are sufficiently low, and in the interior much higher due to the presence of heating in operation. Moreover, measurements on walls directly exposed to solar irradiation must be avoided, as it disturbs the acquisition of data, creating interpretative difficulties of the recording curves which are subjected to anomalous peaks in the time bands from the increased irradiation. The determination of the thermal flow passing through the building envelope should be carried out on a portion of the wall as “average” as possible, i.e., equidistant from other construction elements (such as doors, windows, corner nodes, pillars, beams, projections, etc.). Furthermore, each detection must be carried out avoiding the correspondence with thermal bridges and their zones of influence, which can be determined through an infrared thermographic examination preliminary to the thermal flux-meter survey. A formally correct survey, carried out in accordance with UNI ISO 9869-1 (2015), requires that the instruments must be left in place for a variable period of time depending on the climatic conditions and the type of structure investigated. UNI ISO 9869-1 (2015) indicates that the minimum time frame for an effective and realistic survey is of 3 days, i.e., 72 h. Thanks to the data logger, all the thermal data measured by the individual sensors are recorded at specific time intervals. The collected data will then be downloaded to a PC and processed using a special software to obtain the transmittance value U (W/m2 K).
The calculation of transmittance can be done through two methods (Rospi et al., 2010):
• The moving average method which consists in calculating the conductance using, at all times, instead of the instantaneous values of flow and temperature, the average values calculated on all previous instants. Indicating with Qj the thermal flow passing through the considered element for j-th measurement, and Tsi and Tse the internal and external surface temperature, the conductance C may be obtained as follows:
• The “Black-box” method is a method of identification (Figure 1B), so called because it does not assume the knowledge of the physical system under examination (in our case the wall), but only of time series of input data (internal and external temperature) and outgoing ones (flow). From the data going backward, with a statistical method, it is possible to estimate the physical characteristics of the wall, such as the conductance and, by knowing the internal and external surface thermal resistances, the considered element transmittance.
It is specified that in this study the thermal conductance is calculated by the means of average method. It allows of calculating C by using the mean values obtained on all previous instants, instead of taking into account just the instantaneous values of temperatures and heat flux. To measure the in situ thermal transmittance, it was necessary to measure three parameters: the thermal flow crossing the wall, the internal surface temperature, and the external surface temperature. To this scope the same methods reported in Rospi et al. (2010) were applied. In particular, the heat flux sensor and the probe for measuring the temperature of the internal surface were positioned under a window on the wall north-facing, avoiding direct sources of heat. Then, from the values of thermal flow and surface temperatures, the value of the instantaneous conductance was calculated in according to the Equation (1).
The analysis of thermal bridges and insulation of buildings can be performed using thermography, regulated by the UNI EN 13187 (2000). This type of analysis is used in order to investigate mold and condensation problems or excessive thermal dispersion. Through a thermographic inspection performed on a casing it is possible to determine if and where thermal anomalies (thermal bridges) are localized. The thermography is part of the non-destructive diagnostic tests, carried out through the acquisition of images in the infrared field, measuring the energy emitted by bodies at different temperature from absolute zero by appropriate sensors, and correlating it to the surface temperature of the body itself.
The perception of the overall comfort of the occupants of an environment depends on various aspects such as: thermal comfort, indoor air quality, acoustic quality, and visual comfort. Although the feeling of comfort is subjective, it can be assessed through the measurement of a series of environmental parameters, as well as the development of appropriate indicators. Since the 1960's, many studies have been directed to the analysis of thermal comfort in confined environments, and to the possibility of defining indicators, linked to environmental parameters, that were able to express a quantitative estimate of the thermal sensation perceived by the occupants (Fanger, 1970).
These indexes, based on the theory of the thermal balance of the human body and supported by statistical studies, represent a consolidated approach based on the main technical regulations on the subject such as the UNI EN ISO 7726 (2002), UNI EN ISO 7730 (2006), UNI EN 15251 (2008). For the assessment of global comfort, it is used the Predicted Mean Vote (PMV) index and the Percentage Predicted Dissatisfied (PPD) index. The condition of well-being of an individual depends on the psychophysiological state deriving from the interaction of the organism and its sensory channels with the surrounding physical environment, with particular reference to the thermo-acoustic, lighting and air quality aspects. They together constitute the so-called quality of the internal environment, also called IEQ, Indoor Environmental Quality. The PMV index provides an estimate of the thermal sensation expressed by a certain number of people exposed to the same environment. Whereas, the PPD index expresses the perceived thermal discomfort, estimating the percentage of users that in a certain environment manifest dissatisfaction for too hot or too cold. The PPD is clearly related to the value assumed by the PMV.
The case study chosen in this work is “Sedile” building, an historical school building situated in the ancient downtown of Matera (Italy), recognized as UNESCO Cultural Heritage since 1993. In the past, this building was the old Municipal Parliament present in all free towns of the Naples's Kingdom, while nowadays it is reconverted and used as public conservatory. It was built in 1540 with calcarenite masonry consisting of lime and local sedimentary rock, improperly named “Tufo.” The bearing walls have a thickness variable between 80 to 100 cm for external walls, and between 40 and 60 cm for internal ones, while the two floors were realized by the means of vaulted structures having different heights (Rospi et al., 2017b). In Figure 2, some images of the considered building are reported. In detail, the mezzanine floor, composed by seven rooms (secretariat, direction, bursar's office, reception, and toilets), has a shape similar to a pentagon and an atrium connects the rooms on the ground floor with those on the first floor. The surface in plan is of about 345 m2, while height varies from 2.40 to 5.05 m due to different cross and barrel vaults. Similarly, the same situation is found on the first floor made up of 13 environments divided into classrooms and services. Its surface is about 590 m2 and the heights vary from 2.60 up to 6.70 m. The rooms with flat floors have a height of 3.00 m. On the mezzanine floor one can to clearly (see Figure 3) that the structural body exposed to the west has different characteristics from the central body of the building. The two parts (named Block A and Block B), in fact, were made in different periods, with different types of construction. Waterproofing on the roof is made of tar and bricks in walkways and shingles in sloped areas.
Table 1 shows the parameters found during the inspections, while Table 2 reports the stratigraphy of the opaque elements, the characteristics of the transparent elements and the relative limits imposed by the Italian Law. As for the external walls, their thickness and transmittance vary according to the analyzed plane. External wall transmittance has been calculated using UNI ISO 9869-1 (2015), while opaque covering envelope transmittance has been calculated using UNI EN ISO 6946 (2018), and transparent envelope calculated using UNI EN ISO 10077-1 (2018). In this work for the analyzed case study, it has been also calculated dynamic thermal parameters, that are the periodic thermal transmittance Yie, the internal thermal capacity Ki and the phase displacement ϕ, reported in Table 3. As it is easy to observe, the parameters found are very high, in accordance with the tendency that the environments belonging to the old body are characterized by a greater thermal inertia.
As far as the comfort conditions are concerned, they have been calculated as previously described. In particular, temperature measurements and relative humidity have been conducted through both the BABUC/A and the FLIR MR77 moisture meter. As recommended, the microclimate variables have been measured at to 0.6 m for sitting persons and at 1.1 m for standing people.
To detect air temperature and relative humidity data a psychometric wet bulb sensor with forced ventilation has been applied (Figure 4A). LSI LASTEM BSU102 probe, produced in conformity with the UNI EN ISO 7726 (2002), is a psychrometer for measurement of air temperature and relative humidity and other related quantities. It is composed of two temperature sensors: the first is a thermometer for measuring the air's dry-bulb temperature (Ta), while the second is a thermometer covered with an absorbent sheath and immersed in a tank of distilled water for measuring the forced-ventilation, wet-bulb temperature (Tw). The two sensors are housed in a duct protecting them from sun rays and similar. Air is force-fed into the duct by a fan at a speed of approximately 4 m/sec. The probe air temperature measurements related to dry-bulb and wet-bulb are analyzed by the BABUC unit to calculate the relative and absolute humidity and other related quantities (such as dew-point temperature, enthalpy of air, etc.). For completeness, in the following all the technical features are reported. Environmental limits: 0 ÷ 60°C (with water) and −10 ÷ +60°C (without water). Range of measure: for dry temp.: −25 ÷ +150°C, for wet temp.: 0 ÷ +60°C. Dew point: −20 ÷ +60°C. Temperature accuracy: ±0.15°C at 0°C, ±0.19°C at 20°C, ±0.23°C at 40°C. UR% accuracy (Temperature: 15 ÷ 25°C): 70 ÷ 98%: 0.5%; 40 ÷ 70%: 1%; 15 ÷ 40%: 2%. Resolution is 0.1% UR and the maximum difference the two bulbs (without water) is 0.1°C.
Figure 4. (A) Psychometric wet bulb sensor, (B) Thermometers globe, (C) Hot wire anemometer sensor, (D) 11 inputs multiple data acquisition device- BABUC/A.
The mean radiant temperature has been measured directly using a radiant temperature sensor black globe (thermometers globe) (Figure 4B). LSI LASTEM BST131 globe thermometer probe is composed of a hollow, black metal globe with a temperature sensor mounted at its center. This probe is manufactured in accordance with UNI EN ISO 7726 (2002) standards. Temperature readings (Tg) for a known dry temperature and air speed, allow BABUC unit to calculate the mean radiant temperature (Tr). The globe, also known as a Vernon globe, has a very high emission level (0.98) and very low reflection (2% ASTM 97-55), effectively making it a black body with respect to infrared radiation. With this probe, it is possible to evaluate and to check radiant thermal loads, which are extremely important for analyzing both temperate and hot environments. In particular, this probe is indispensable for determining the operating temperature, the PMV and PPD indices and the WBGT index, to cite just the more important standards. It has an environmental limit of −5 ÷ 60°C, measurement range is −10 ÷ +100°C. Accuracy is ±0.15°C at 0°C, ±0.19°C at 20°C, and ±0.23°C at 40°C.
Whereas, for measuring the air velocity a hot wire anemometer sensor has been used (Figure 4C). LSI LASTEM BSV105 sensor is a portable anemometric probe with omnidirectional hot wire for measurement of average air speed (va) and turbulence intensity (TU) in compliance with UNI EN ISO 7726 (2002). Due to its very fast acquisition rate (1 value/100 ms), the sensor measures air speed calculates the average (va) and the standard deviation over a period of 2 sec. measurements, and divides these two quantities, directly obtaining the turbulence intensity (TU) expressed as a percentage. The turbulence intensity is an important quantity in order to evaluate air drafts that could cause discomfort to the people; the TU value is used in the following:
• as a factor inside the DR index formula (Draft rating—ISO7730). The DR index shows the risk of drafts, that is the percentage of dissatisfied due to air drafts. This parameter is directly calculated by BABUC (Vers. 0 > 5.02) as derived calculation;
• as value for studying the dynamics of air flow in environments, inside ducts and in ventilation outlets, that could result in a build-up of air or gas turbulence which could affect the efficiency and effectiveness of the HVAC system.
Environmental limits are −30 + 200°C sensitive element −30 + 70°C electronic circuitry. Average air speed range is 0 ÷ 20 m/s and turbulence intensity range is 0 ÷ 100%. Accuracy (Temperature: 10 ÷ 30°C) is: 0 ÷ 0.5 m/s: ±5 cm/s; 0.5 ÷ 1.5 m/s: ±10 cm/s; > 1.5 m/s: ±4%. Resolution 0.01 m/s; 1%.
All the sensors listed above have been connected to a control unit, BABUC/A, a 11 inputs multiple data acquisition device that can be connected to sensors of different sizes and with different physical principles (Figure 4D).
To detect the values of temperature and relative humidity on the outside of the buildings a FLIR MR77 device has been used (Figure 5), a non-penetrating psychrometer incorporating an infrared thermometer. IR Temperature has a range −4 to 392°F, −20 to 200°C/Basic with an accuracy of ±3.5%. Relative Humidity has a range of 0–99% RH/Basic with an accuracy of ±2.5% RH. Vapor Pressure has a range of 0–20.0 kPA/Basic with an accuracy of ±2%.
For the case study eight rooms have been monitored six times per day, calculating the PMV, PPD and operative temperature “Top” for each measure. The measurements have been performed with plant on and off, and by considering a metabolic rate “M” of 1.46 met and a clothing resistance “Icl” of 0.8. The measurement campaign has been conducted in three different periods, specifically, summer, intermediate season, and winter. The obtained measurements are plotted in Figures 6, 7. In particular, Figure 6 show as in summer and winter season PMV index is neutral and its value is, respectively, 0.53 and 0.55, while PPD index results, respectively, 10.82 and 11.36. In the intermediate season PMV and PPD values are 0.01 and 5.10, respectively. Thanks to high thermal inertia of this structure, the internal comfort is neutral.
Whereas, in Figure 7 indoor comfort results to be neutral in intermediate and winter season. Because of different wall structure in summer season we have a slightly warm condition.
Another analysis useful to energy assessment is the thermography which was conducted using Flir E75 thermo-camera. FLIR E75 camera offers the superior resolution and range performance needed to quickly identify hot spots and discover potential points of failure in electrical distribution and mechanical systems. With up to 161.472 pixels resolution and a larger, more vibrant LCD screen than any other pistol-grip thermal camera, the Exx-Series makes it easier than ever to diagnose problems—even at a certain distance. IR Resolution is 320 × 240 with object temperature range of −20 to 120°C (−4 to 248°F) 0 to 650°C (32 to 1,200°F). Accuracy is of ±2°C (±3.6°F) or ±2% of reading for ambient temperature 15–35°C (59 to 95°F) and object temperature above 0°C (32°F). The images reported in Figure 8 show some problem found in this building contributing to heat loss through the enclosure.
Figure 8. (A) Thermography of new building, (B) Infiltration of water from the cover, (C) Infiltration of water from wall against earth, (D) Thermal bridge walls-windows.
All non-invasive methods applied in the previous paragraph are useful to hypothesize energy improvement linked to seismic retrofit. In a previous work (Rospi et al., 2017b) a building model was implemented in Design Builder software (dynamic method based on Energy Plus Code) identifying interventions necessary to reduce the energy consumption. In this work the authors calibrated energy model using Inequality Coefficient IC based on differences of measured temperature [Dexp,t = (Tint,t−Text,t)exp] and simulated temperature [Dsim,t = (Tint,t−Text,t)sim].
IC can range between 0 and 1 (value of 0 indicates a perfect match, while 1 indicates no match). This coefficient was calculated for different seasons: in summer season IC value is 0.24; in intermediate season IC value is 0.2; in winter season IC value is 0.14.
To improve energy performance of the studied historical building it is possible to proceed by considering the envelope or in plant by verifying environmental and architectural sustainability due to historical and architectural restriction.
As result of the numerical simulation, in Figure 9 fabric and ventilation losses are reported. By analyzing these data, it is possible to note that the heat losses through the vertical envelope are greater than other contributions. Therefore, at first it is considered necessary to intervene on the vertical envelope and cover plan.
Nowadays, there are available different modern techniques in order to improve the energy performance of the existing buildings. Among these, it should be possible to use those that may provide also an upgrading from a seismic point of view for the structure. Discussion on the seismic response of masonry structures may be found, by referring to ancient churches in Caprili et al. (2017), Chiumiento and Formisano (2019), D'Amato et al. (2018), D'Amato et al. (2019), Díaz Fuentes et al. (2019), Formisano and Marzo (2017), Fuentes et al. (2019), Lopez et al. (2019), Ramirez et al. (2019), and by considering ancient masonry bridges in D'Amato et al. (2017), Laterza et al. (2017a,b). In this approach, it also important to pay attention to significance of in-situ tests, as reported in Krstevska et al. (2010), Formisano et al. (2018), and in Luchin et al. (2018).
A classical intervention is represented by the thermal coat application consisting, as known, of a particular coat added very often on the two faces (internal and external) of a wall, such that to create a “sandwich.” In this way, the added layers increase significantly the energy performance of the walls, and consequently, the building one. In reality, if the so-applied “second skin” is properly made and connected to the primary walls also a seismic upgrading may be obtained. It is worthy of mentioning the thermal coat composed by a simple superimposition of two insulating layers containing an additional core of reinforced concrete (Elsaid et al., 2011). Moreover, the concrete core very often is reinforced by a steel web, i.e., horizontal and vertical steel reinforcement. This application may be realized on one or both the wall faces, and for providing a seismic performance improvement the thermal coat should be properly connected to the floors, through dedicated connection (e.g., dowels), and transversally with steel ties, or similar, passing through the wall thickness. In this way, the applied layers ensure the transmission of the internal forces acting in the additional panels to vertical elements of the structure, down to the foundation plan. It is clear, however, that this solution in the case of existing buildings with historical value may result problematic, not only for its invasiveness. In fact, in these buildings the thermal advantage is that the walls are generally massive, usually made of stone or solid brick. In winter they work as thermal insulation and containment of dispersions. In the summer, however, the dissipation of excess heat takes place thanks to the excellent capacity of the casing to store and re-emit heat with a certain temporal phase shift. To this, is should be added that an additional insulator can have negative effects on the hygrometric behavior of the walls, with possible formation of interstitial condensation. In effects, in traditional buildings the presence of high porosity materials is commonly found, together with constructive elements generally without vapor barrier or waterproofing. This determines a higher level of humidity which, thanks to the characteristics of these walls, is usually dispersed through an easy evaporation of the building internal humidity. For these reasons, the choice of thermal insulation must be compatible with the permeability and breathability of the existing building system. Discussion of this aspect may be found in Ramesh (2016).
Another typical intervention, much more compatible from a thermal point of view, is represented by natural organic fibrous materials such as hemp fiber, sheep's wool, cellulose fibers. These insulators derive from materials possessing an open cell structure, with a large number of elongated fibers. They constitute a network capable of retaining hot air and limiting collisions between gaseous molecules, reducing heat transmission by convection and radiation. Furthermore, this structure makes them breathable and hygroscopic, that is, capable of absorbing, transmitting, and emitting steam and air humidity. This characteristic brings their thermos-hygrometric behavior closer to that of traditional masonry, moist, and transpiring, supporting the natural permeability of the envelope. This determines a higher level of humidity which, thanks to the characteristics of these walls, is usually disposed of through an easy evaporation of the internal humidity of the building. Looking at our case study, the first intervention is for example the insulation of walls with inner coat with 5 cm of kenaf plate (λ = 0.038 W/mK). The choice of an inner coat is necessary because of, due to historical constraints, the external faces of walls have to be preserved. Kenaf is a material with excellent insulating properties both from a thermal and acoustic point of view (Table 4). It is breathable, hygroscopic and able to regulate the internal humidity of an environment throughout the year, thus ensuring a healthy and comfortable climate. It also presents a normal (class B2) fire resistance, in accordance with UNI EN 13501-1 (2009). By applying this panel the thermal transmittance varies from 0.88 to 0.31 W/m2K. This allows the reduction of the energy consumption of 4% as reported in Table 5. Instead, the second intervention is a cover insulation with 9 cm of Kenaf plate (λ = 0.038 W/mK, U = 0.25 W/m2K) that, as results from the Table 5, needs to analyze overloads.
Alternatively, in this paper it is also discussed the basic idea to continue in applying Kenaf, but in the form of bio-composites material. Recently, the depletion of petroleum resources and the increase of environmental awareness has led to the development of composites based natural resources. These new materials are directly derived from the agriculture and are sustainable, eco-friendly, eco-efficient, and biodegradable. In detail, polymers applied forming the matrix consist of renewable raw materials or easily biodegradable and disposable in composting plants. Among these, there are the polymers from renewable resources (indicated as PFRR), such as the Poly-Lactic Acid (PLA), derived from corn, wheat, and beet, that are rich in natural sugar (dextrose; Henton et al., 2005). These natural polymers may be used as resins similarly to plastics, reinforced with kenaf fibers. For instance, for producing 1 kg of PLA are required about 2.5 kg of corn. Few experimental results are available in the published literature on composites material such as Kenaf/PLA. For instance, in Ochi (2008) are reported experimental results about Kenaf/PLA specimens. More in detail, in this study it has been found that unidirectional biodegradable composites fabricated using an emulsion-type PLA resin and kenaf fibers at a fiber content of 70% have shown high tensile strength of 223 MPa and flexural strength of 254 MPa, respectively. In addition, by analyzing its biodegradability, after composting for 4 weeks tensile strength decreases of 91% while weight decreases of 38%, respectively. Therefore, since the mechanical properties are really relevant, it would be useful to applicate these composite material in the structural rehabilitation as wall strengthening. In particular, the idea would be of applying a layer of kenaf/PLA as internal plaster, or a coat on the two faces (internal and external) of a wall of opportune thickness. The thickness will be designed in according to structural and thermal criteria, so that the designed intervention will respond to integrated design requirements. This intervention may substitute the classical intervention of spritz-beton, usually applied in existing masonry buildings for quickly spraying the grout on a wall face in addition to a welded mesh as reinforcement. As example, in Figure 10 it is reported the structural response of primary walls belonging to the case study in terms of push-over curve. The capacity model here adopted is indicated in the Instructions for the application of the Ministerial Decree (M.D. 17/01/2018)2. In accordance to this model, and by considering the most likely failure mechanism due to the diagonal cracking for walls having a masonry regular texture, in the Figure 10 it is reported the actual response (without intervention) and the response would result by applying the kenaf/PLA composite. Two different applications are considered: one referred to an internal wall where an internal and an external layer are considered; while the second one is supposed to be applied to an external wall only on the internal face. This applicability conditions are typical of historic buildings where it has to be preserved only the external aspect of the building, while internally the intervention application is permitted. In both cases the layer of kenaf/PLA composite has a width of at least 5 cm. As it is easy to note, the intervention with the considered material produces, not only a benefit from an energetic point of view but also an improvement in the structural response. Of course, this aspect should deserve to be experimentally investigated in detail, mainly to properly understand the superficial interaction among the composite and the masonry support due to the debonding phenomena. The proposal of an integrated approach combining the energetic and structural performances would make the design intervention much more convenient and also competitive in terms of sustainability.
Figure 10. Pushover curves of two walls belonging to the case study without and with the application of the kenaf/PLA composite.
Thanks to non-invasive method it is possible to investigate historical building from energy point of view without compromising their identity. Often historical building, especially buildings in calcareous stone, as the case study considered, are affected by moisture due to a high rate of voids that characterize the calcareous rocks, which increases the ability to retain water, when the rock itself comes into contact with this fluid mass.
The moisture due to infiltration, condensation or capillary rise is often linked to many thermal bridges. This phenomenon can be investigated with thermal mapping, thermo-hygrometer and knowing the physical characteristic of materials. Because of few openings to the outside to avoid both excessive radiation in summer and excessive heat loss in winter, the repaired surfaces are subject to the night condensation with the development of lichens which caused a degradation of the finishing surface layers.
In this paper, it has been also discussed the basic idea of an integrated approach for buildings rehabilitation from thermal and structural point of view. In particular, it has been proposed an innovative application of a bio-composite material, easy to apply capable of improving the energy and seismic performance of the elements, respecting the architectural requirements. Its efficiency will be demonstrated with dedicated laboratory tests, where the applications will be mainly addressed to the type of masonry typical of ancient historical structures. The required thickness will satisfy to structural and thermal criteria, so that the designed intervention will respond to integrated design requirements.
All datasets generated for this study are included in the manuscript/supplementary files.
EN took care of the part concerned of methodology for estimating the energy performance, energy audit, and discussion of results. NC took care of the part concerned introduction. MD'A took care of the part concerned the discussion of main results. All authors took part in the same way in writing the paper.
The authors declare that the research was conducted in the absence of any commercial or financial relationships that could be construed as a potential conflict of interest.
Q, thermal flow [W/m2]; Tsi, internal surface temperature [°C]; Tse, external surface temperature [°C]; C, conductance [W/m2 K]; PMV, predicted mean vote [dimensionless]; PPD, percentage predicted dissatisfied [%]; s, thickness [m]; U, thermal transmittance [W/m2K]; Yie, periodic thermal transmittance [W/m2K]; Φ, phase displacement [h]; Ki, internal thermal capacity [kJ/m2K]; Top, operative temperature [°C]; Icl, clothing resistance; ρ, densità [kg/m3]; c, specific heat [J/kg*K]; λ, conducibilità termica [W/mK]; m, surface mass [kg/m2].
1. ^G.U. n. 47. (February 26th, 2011). Directive of the Prime Minister dated on 09/02/2011. Assessment and mitigation of seismic risk of cultural heritage with reference to the technical code for the design of construction, issued by D. M. 14/01/2008 (in Italian).
2. ^Instructions for the application of the Ministerial Decree M.D. 17/01/2018. Circolare 21 Febbraio 2019, n. 7 – Istruzioni per l'applicazione dell'Aggiornamento delle Nuove Norme Tecniche per le Costruzioni di cui al D.M. 17 Gennaio 2018, S.O. n. 8 of the Official Gazette of the Italian Republic published on 20/02/2018, n. 42. In Italian.
Andrus, P. W., and Shrimpton, R. H. (1997). Section II: National Register Criteria for Evaluation. National register bulletin 15: How to Apply the National Register Criteria for Evaluation, 4th Edn. Washington, DC: U.S. Department of the Interior.
BPIE (2011). Europe's Buildings Under the Microscope. Brussel: Buildings Performance Institute Europe.
Caprili, S., Mangini, F., Salvatore, W., Bevilacqua, M. G., Karwacka, C, odini, E., et al. (2017). A knowledge-based approach for the structural assessment of cultural heritage, a case study: La Sapienza Palace in Pisa. B. Earthq. Eng. 15, 4851–4886. doi: 10.1007/s10518-017-0158-y.
Chiumiento, G., and Formisano, A. (2019). Simplified and refined analyses for seismic investigation of historical masonry clusters: Comparison of results and influence of the structural units position. Front. Built Environ. 5:84. doi: 10.3389/fbuil.2019.00084
D'Amato, M., Gigliotti, R., and Laguardia, R. (2019). Comparative seismic assessment of ancient masonry churches. Front. Built Environ. 5:56. doi: 10.3389/fbuil.2019.00056
D'Amato, M., Laterza, M., and Casamassima, V. M. (2017). Seismic performance evaluation of a multi-span existing masonry arch bridge. Open Construct. Build. Technol. J. 11(Suppl.-5), 1191–1207. doi: 10.2174/1874149501711011191
D'Amato, M., Laterza, M., and Dìaz Fuentes, D. (2018). Simplified Seismic analyses of ancient churches in Matera's landscape. Int. J. Architect. Heritage. doi: 10.1080/15583058.2018.1511000. [Epub ahead of print].
Díaz Fuentes, D., Baquedano Julià, P. A., D'Amato, M, and Laterza, M. (2019). Preliminary seismic damage assessment of Mexican churches after September 2017 earthquakes. Int. J. Architect. Heritage. doi: 10.1080/15583058.2019.1628323
Elsaid, A., Dawood, M., Seracino, R., and Bobko, C. (2011). Mechanical properties of kenaf fibre reinforced concrete. Constr. Build. Mater. 25, 1991–2001. doi: 10.1016/j.conbuildmat.2010.11.052
Fanger, P. O. (1970). Thermal Comfort-Analysis and Applications in Environmental Engineering. Copenhagen: Danish Technical Press.
Filippi, M., and Fabrizio, E. (2012). Introduction to the Dynamic Termoenergetic Simulation of Buildings, AiCARR Guidelines. Milan: Editoriale Delfino.
Formisano, A., Chiumiento, G., Fabbrocino, F., and Landolfo, R. (2017). “Comparative seismic evaluation between numerical analysis and Italian guidelines on cultural heritage applied to the case study of a masonry building compound,” in Proceedings of the 14th International Conference of Numerical Analysis and Applied Mathematics–ICNAAM 2016, Vol. 1863 of AIP Conference Proceedings, eds T. Simos and C. Tsitouras (Rhodes).
Formisano, A., and Marzo, A. (2017). Simplified and refined methods for seismic vulnerability assessment and retrofitting of an Italian cultural heritage masonry building. Comput. Struct. 180, 13–26. doi: 10.1016/j.compstruc.2016.07.005
Formisano, A., Vaiano, G., Fabbrocino, F., and Milani, G. (2018). Seismic vulnerability of Italian masonry churches: the case of the nativity of blessed virgin Mary in Stellata of Bondeno. J. Build. Eng. 20, 179–200. doi: 10.1016/j.jobe.2018
Fredrizzi, R., and Dipasquale, C. (2015). Fabbisogni energetici: case e uffici sotto la lente, Casaeclima n. 54 (In Italian).
Fuentes, D. D., Laterza, M., and D'Amato, M. (2019). “Seismic vulnerability and risk assessment of historic constructions: the case of masonry and adobe Churches in Italy and Chile,” in Proceedings of SAHC 2018, 11th International Conference on Structural Analysis of Historical Constructions, RILEM Bookseries 18 (Cusco), 1127–1137.
Henton, D. E., Gruber, P., Lunt, J., and Randall, J. (2005). Polylactic acid technology. Nat. Fiber Biopol. Biocompos. 16, 527–577. doi: 10.1201/9780203508206.ch16
Krstevska, L., Tashkov, L., Naumovski, N., Florio, G., Formisano, A., Fornaro, A., et al. (2010). “In-situ experimental testing of four historical buildings damaged during the 2009 L'Aquila earthquake,” in COST ACTION C26: Urban Habitat Constructions under Catastrophic Events - Proceedings of the Final Conference (Naples), 427–432.
Laterza, M., D'Amato, M., and Casamassima, V. M. (2017a). “Seismic performance evaluation of multi-span existing masonry arch bridge,” in Proceedings of the 14th International Conference of Numerical Analysis and Applied Mathematics - ICNAAM 2016, Vol. 1863 of AIP Conference Proceedings, eds T. Simos and C. Tsitouras (Rhodes).
Laterza, M., D'amato, M., and Casamassima, V. M. (2017b). Stress-life curves method for fatigue assessment of ancient brick arch bridges. Int. J. Architect. Heritage 11, 843–858. doi: 10.1080/15583058.2017.1315621
Lopez, S., D'Amato, M., Ramos, L., Laterza, M., and Lourenço, P. B. (2019). Simplified formulation for estimating the main frequencies of ancient masonry churches. Front. Built Environ. 5:18. doi: 10.3389/fbuil.2019.00018
Luchin, G., Ramos, L. F., and D'Amato, M. (2018). Sonic tomography for masonry walls characterization. Int. J. Architect. Heritage. doi: 10.1080/15583058.2018.1554723. [Epub ahead of print].
Mazzarella, L. (2015). Energy retrofit of historic and existing buildings. The legislative and regulatory point of view. Energy Build. 95, 23–31. doi: 10.1016/j.enbuild.2014.10.073.
McClelland, L. F. (1997). Appendix IV: Glossary of National Register Terms. National Register Bulletin 16A: How to Complete the National Register Registraton Form, 4th Edn. Washington, DC: U.S. Department of the Interior.
MIBACT (2015). Guidelines for the Improvement of Energy Efficiency in Cultural Heritage. Rome: MIBACT
Negro, E., Cardinale, T., Cardinale, N., and Rospi, G. (2016). Italian guidelines for energy performance of cultural heritage and historical buildings: the case study of the Sassi of Matera. Energy Proc. 97, 7–14. doi: 10.1016/j.egypro.2016.10.008
Ochi, S. (2008). Mechanical properties of kenaf fibers and kenaf/PLA composites. Mech. Mater. 40, 446–452. doi: 10.1016/j.mechmat.2007.10.006
Ramesh, M. (2016). Kenaf (Hibiscus cannabinus L.) fibre based bio-materials: a review on processing and properties. Progr. Mater. Sci. 78–79, 1–92. doi: 10.1016/j.pmatsci.2015.11.001
Ramirez, E., Lourenco, P. B., and D'Amato, M. (2019). “Seismic assessment of the Matera cathedral,” in Proceedings of SAHC 2018, 11th International Conference on Structural Analysis of Historical Constructions, RILEM Bookseries 18 (Cusco), 1346–1354.
Rospi, G., Cardinale, N., Intini, F., and Negro, E. (2017a). Analysis of the energy performance strategies of school buildings site in the Mediterranean climate: a case study the schools of Matera city. Energy Build. 152, 52–60. doi: 10.1016/j.enbuild.2017.07.018
Rospi, G., Cardinale, N., and Negro, E. (2017b). Energy performance and economic feasibility study of historical building in the city of Matera, Southern Italy. Energies 10:2009. doi: 10.3390/en10122009
Rospi, G., Cardinale, N., Stefanizzi, P., and Augenti, V. (2010). Thermal performance of mobile home with light envelope. Build. Simul. 3, 331–338. doi: 10.1007/s12273-010-0017-0
UNI EN 13187 (2000). Thermal Performance of Buildings - Qualitative Detection of Thermal Irregularities in Building Envelopes - Infrared Method.
UNI EN 13501-1 (2009). Fire Classification of Construction Products and Building Elements - Part 1: Classification Using Data From Reaction to Fire Tests.
UNI EN 15251 (2008). Indoor Environmental Input Parameters for Design and Assessment of Energy Performance of Buildings Addressing Indoor Air Quality, Thermal Environment, Lighting and Acoustics.
UNI EN ISO 10077-1 (2018). Thermal Performance of Windows, Doors and SHUTTERS - CALCULATION of Thermal Transmittance - Part 1: General
UNI EN ISO 6946 (2018). Building Components and Building Elements - Thermal Resistance and Thermal Transmittance - Calculation Methods.
UNI EN ISO 7726 (2002). Ergonomics of the Thermal Environment - Instruments for Measuring Physical Quantities.
Keywords: energy audit, kenaf fiber, indoor comfort, thermography, seismic upgrading
Citation: Negro E, D'Amato M and Cardinale N (2019) Non-invasive Methods for Energy and Seismic Retrofit in Historical Building in Italy. Front. Built Environ. 5:125. doi: 10.3389/fbuil.2019.00125
Received: 26 April 2019; Accepted: 03 October 2019;
Published: 22 October 2019.
Edited by:
Hasim Altan, Final International University, CyprusReviewed by:
Michele Dassisti, Politecnico di Bari, ItalyCopyright © 2019 Negro, D'Amato and Cardinale. This is an open-access article distributed under the terms of the Creative Commons Attribution License (CC BY). The use, distribution or reproduction in other forums is permitted, provided the original author(s) and the copyright owner(s) are credited and that the original publication in this journal is cited, in accordance with accepted academic practice. No use, distribution or reproduction is permitted which does not comply with these terms.
*Correspondence: Elisabetta Negro, aW5nLm5lZ3JvZWxpc2FiZXR0YUBnbWFpbC5jb20=
Disclaimer: All claims expressed in this article are solely those of the authors and do not necessarily represent those of their affiliated organizations, or those of the publisher, the editors and the reviewers. Any product that may be evaluated in this article or claim that may be made by its manufacturer is not guaranteed or endorsed by the publisher.
Research integrity at Frontiers
Learn more about the work of our research integrity team to safeguard the quality of each article we publish.