- 1Department of Biology, Texas A&M University, College Station, TX, United States
- 2Department of Biochemistry & Molecular Biology, The University of Chicago, Chicago, IL, United States
- 3Department of Medicine, Section of Genetic Medicine, The University of Chicago, Chicago, IL, United States
Biomolecular condensation allows for the dynamic organization of molecules in time and space. Condensate formation is regulated through many mechanisms including the action of molecular chaperones. While molecular chaperones have long been viewed through the lens of their roles in protein folding, misfolding, and quality control, their ability to manipulate protein-protein interactions is increasingly recognized to play a major role in the precise control of condensate biology. In this review we highlight recent studies investigating the roles of canonical and non-canonical chaperones in regulating condensate formation, material state, and dispersal. We discuss the broadening of longstanding conceptions of chaperone functions to include condensate regulation, and the discovery of previously unappreciated chaperone activities in well-known proteins. We close by considering the biological activities being uncovered during the ongoing upheaval at the boundary between chaperone biology and biomolecular condensation.
Introduction
The regulation of reactions in cells requires the dynamic organization of macromolecules. In addition to being segregated by membrane-bound compartments, numerous processes in cells are organized into biomolecular condensates—membraneless, non-stoichiometric ensembles of macromolecules (Banani et al., 2017; Sabari et al., 2020; Lyon et al., 2021; Glauninger et al., 2022). Some of these condensates, such as the nucleolus, are constitutive features of cells which help organize basic housekeeping functions of the cell (Lafontaine et al., 2021). Others, such as stress granules, form transiently in response to an external signal (Glauninger et al., 2022).
Remarkable progress has been made in understanding the biophysical forces underlying condensation in cells and even reconstituting in vitro many condensates, both simple and complex. These studies have shown that the condensation behavior of many proteins is encoded directly in their amino acid sequences, even including the ability to modulate condensation based on environmental signals—often conceived of as stresses—such as heat, pH, and desiccation (Riback et al., 2017; Yoo et al., 2019; Iserman et al., 2020; Dorone et al., 2021). Early studies which helped jumpstart the ongoing revolution in condensate biology routinely showed not just that proteins, along with other biological molecules, formed condensates upon a change in conditions—concentration, ionic strength, pH, temperature, and so on—but also that condensation was spontaneously reversible when conditions returned to the prior state (Molliex et al., 2015; Nott et al., 2015). The loss of spontaneously reversible interactions was highlighted as a key step in the transition to pathological states, such as amyloid (Molliex et al., 2015).
Over time, the accumulation of results in multiple systems has modulated this picture considerably. Spontaneous reversal of nonpathological condensates gave way to regulated dispersal by posttranslational modification or the action of molecular chaperones (Yoo et al., 2022). The latter, one aspect of the chaperone-mediated regulation of biomolecular condensates which is the subject of this review, remains relatively new, and may be surprising, given entrenched models describing the nature of chaperone action and of chaperone substrates. Chaperones have long been conceived of as protein quality control systems, acting to combat toxic protein misfolding (proteotoxic stress) and restore protein homeostasis (proteostasis) when it is disrupted (Morimoto, 2008; Vabulas et al., 2010; Zhou et al., 2014; Ungelenk et al., 2016; Hua et al., 2022). It is abundantly clear that chaperones possess these activities, and express them when confronted with foreign misfolded aggregation-prone substrates. However, the endogenous substrates of chaperones remain remarkably ill-defined.
Chaperones and condensates: beyond protein quality control
The present review is intended to promote reconsideration of some of these ideas in light of biomolecular condensation. In the simplest terms, the model in which proteins form toxic aggregates that can be cleaned up by chaperones must be expanded—and in some circumstances replaced—by a model in which proteins form adaptive condensates which are regulated at every step by chaperones.
A recent study serves to illustrate how the proteotoxicity and protein quality control perspective has become paradigmatic, in the sense of being an implicit frame which profoundly shapes interpretations, and yet is potentially misleading. In work focusing on the sequestrase activity of Btn2 (reviewed extensively here), ethanol-treated cells show similar insoluble protein levels to cells heat-shocked at 42°C, leading to the conclusion that “severe ethanol stress … causes the denaturation and aggregation of proteins in yeast cells” (Kato et al., 2019). One can see the paradigm at the edges of this framing. Yet it is increasingly clear that many stress-triggered condensates are adaptive, evolved, and unlike denatured aggregates. Specifically, 42°C heat shock has not been shown to cause either denaturation or proteotoxic aggregation of mature proteins; instead, dozens of proteins form reversible condensates which variously retain enzymatic activity, promote growth and translation during stress, form by phase separation driven by limited conformational changes which are conserved across species rather than denaturation and aggregation, and so on (Wallace et al., 2015; Riback et al., 2017; Iserman et al., 2020; Keyport Kik et al., 2024; Chen et al., 2024). To the subject of this review, chaperones interactions with thermally induced misfolded aggregates turn out to differ markedly from their interactions with thermally triggered condensates (Yoo et al., 2022).
In light of these developments, we suggest the reader keep an open mind. Where prior work has implied aggregation is toxic under conditions in which endogenous substrates are largely or completely unknown, and where key results have relied on the use of foreign aggregating reporters, small-molecule inhibitors, overexpression, or deletions, the door to an alternative adaptive condensation interpretation should remain open.
We also extend this open mind to the term biomolecular condensate itself, which we here use in the most broad sense, referring to any membrane-less cluster which concentrates specific macromolecules. This includes structures such as amyloids, in which high-affinity specific interactions link molecules together into a chain, or effectively a one-dimensional condensate. While amyloids are traditionally associated with toxic aggregation, there are now a plethora of examples of functional, adaptive, and reversible amyloids (Boke et al., 2016; Chuang et al., 2018; Woodruff et al., 2018; Cereghetti et al., 2021). That said, we will still attempt to distinguish between aggregates (inert, non-adaptive and misfolded assemblies of proteins) and condensates whose clustering we generally assume to be an evolved or at least adaptive trait.
With that in mind, here we will focus on regulation of condensation, broadly conceived, by molecular chaperones. The concept of a molecular chaperone has evolved and expanded over time, and we adopt an inclusive view. Chaperones were originally described as “proteins whose function is to ensure that the folding of certain other polypeptide chains and their assembly into oligomeric structures occur correctly” (Ellis, 1987). Although this initial definition also included a provision that chaperones not form part of the final assembly, subsequent work has relaxed this requirement to include chaperones, including small heat-shock proteins, which remain associated with larger-scale structures, potentially to alter their physical characteristics, and perhaps as a consequence of directing their formation. Indeed, the recognition that heat-shock proteins—literally, at the outset, proteins produced in response to heat shock—were rife with what soon came to be called molecular chaperones has led to use of these terms somewhat interchangeably and imprecisely.
The recent recognition that some intracellular aggregates are in fact biomolecular condensates, even in the case of heat shock itself, further justifies the stance we adopt here: chaperone activities include not just the prevention of oligomeric assemblies—even today synonymous with “chaperone activity”—but also promotion, modulation, and dispersal of oligomeric assemblies, of which condensates are an increasingly important example. This stance leaves out related activities, such as the posttranslational modification of molecules to regulate their condensation, while preserving the spirit of the chaperone concept. We will also leave out nucleic acid chaperones, such as RNA helicases, which play an important role in regulating biomolecular condensates containing both nucleic acids and proteins by regulating RNA-RNA (or DNA-DNA) and nucleic acid-protein interactions (Ripin and Parker, 2022). In Table 1, we have included a list of chaperones discussed in this review and the condensates that they regulate.
We begin with a brief overview of the biophysics of condensate formation, highlighting the thermodynamic and kinetic forces which enable the complex dynamics and structures of condensates. Chaperones are able to finely tune these forces to regulate all stages of the condensate lifecycle, and we organize our review accordingly, highlighting the ways in which chaperones promote and direct the formation of condensates; regulate condensate size, dynamics, and internal structure; and promote condensate dispersal. We close with discussion and review of the functional consequences of condensate regulation by chaperones, highlighting open challenges in understanding the biology of these still-enigmatic processes.
Integrating chaperones into a conceptual framework of condensation
While biomolecular condensate formation is often compared to simple systems such as the phase separation of oil and water, the underlying molecular forces governing complex biological polymers are much more complex. We will not attempt a comprehensive review of the biophysics underlying condensates, instead referring readers to many excellent and detailed reviews on the subject (Brangwynne et al., 2015; Banani et al., 2017; Choi et al., 2020; Mittag and Pappu, 2022). As a very brief intro, biomolecular condensates generally require multivalent interactions in order to form a three dimensional network (Li et al., 2012; Brangwynne et al., 2015; Choi et al., 2020). One useful model for these multivalent interactions is that interacting “stickers” are connected by a flexible “spacer” (Choi et al., 2019). This model can be adapted to interaction surfaces as small as individual amino acids and as big as entire proteins. The propensity of a system to phase separate is determined by the relative strength of the interactions between the stickers, spacers and solvent. Stronger sticker-sticker interactions and weaker sticker-solvent interactions will promote condensation. The strength of these interactions is not just an intrinsic property of the molecule, but is dependent on a variety of environmental factors, including salt concentrations, temperature and pH.
Importantly these interactions also set a critical concentration of the condensing molecules themselves. Condensation is only energetically favorable above this concentration. Because the critical concentrations reflect the current cellular environment, any changes to that environment can change the transition threshold, causing condensates to form or dissolve without changes in the concentration of the molecules. These cellular changes could include direct environmental signals such as heat or osmolarity changes, the appearance of a new species such as dsRNA from a virus or changes in the concentration of a secondary messenger such as cAMP(Riback et al., 2017; Jalihal et al., 2019; Iserman et al., 2020; Zhang et al., 2020; Dorone et al., 2021; Wen and Ma, 2022; Watson et al., 2023). Chaperones can also change this critical concentration by competing for those same intramolecular interactions that drive condensation. This is then thermodynamic control of condensates, in which chaperones change the underlying energy landscape to favor or disfavor condensation (Figure 1).
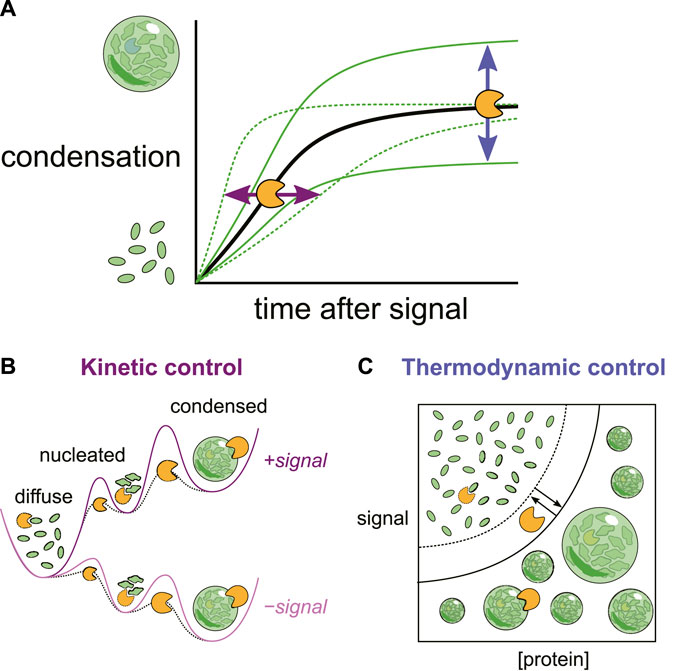
Figure 1. (A) Changes in the cellular environment can trigger biomolecular condensation. Chaperones can reshape both the (B) kinetics (rates) and (C) thermodynamics (levels) of condensation by interactions at multiple stages.
While phase transitions can happen quickly, they are not instantaneous. Even in an energetic landscape where condensation is favorable, there is a kinetic barrier to converting soluble molecules to condensates and vice versa. Here too chaperones play a key role. In fact, modulating barriers between intermediate states is the principle mechanism by which chaperones accomplish their canonical task of encouraging proteins to reach their most energetically favorable form (Hartl et al., 2011). By modulating the kinetic barrier between soluble and condensed states, chaperones can accelerate or inhibit condensate formation and dispersal, which in turn can have important functional implications for cellular growth and adaptation (Figure 1).
Chaperones promote and direct biomolecular condensation
Alongside the well-known roles of chaperones in preventing aggregation, dispersing aggregates, and assisting with folding, a wide range of studies have uncovered roles for chaperones—primarily small heat-shock proteins and functional homologs—in promoting and directing localization of condensation. The overwhelming majority of work on such activities of chaperones, which are termed sequestrases, aggregases, or condensases in this context (Kedersha et al., 2016; Ho et al., 2019; Mogk et al., 2019; Weis, 2021; Shrivastava et al., 2022; Carter et al., 2023), has focused on formation of aggregates or deposits of misfolded, denatured proteins en route to degradation, with sequestration thought to serve a cytoprotective function (Shrivastava et al., 2022). As described in the Introduction, we adopt the perspective that adaptive condensation coexists with such quality control activities.
Our knowledge of sequestrase activity has come overwhelmingly from studies in budding yeast, where, after considerable efforts, multiple groups have converged on a common high-level understanding of the structures and players involved (Kaganovich et al., 2008; Malinovska et al., 2012; Miller et al., 2015). Yeast forms three major quality control structures apparent as foci by microscopy: a cytosolic quality control compartment called CytoQ, an intranuclear quality control compartment called INQ, and a perivacuolar insoluble protein deposit called IPOD (Kaganovich et al., 2008; Miller et al., 2015; Miller et al., 2015). Although these structures are commonly referred to as “compartments,” the nature of their boundaries remains unclear, apart from their lack of a membrane.
How precisely these proteostatic compartments form, and how the activity of sequestrase molecules promotes the appearance of microscopically visible structures, is not yet fully known. However, their appearance is regulated by two key chaperone proteins, the major sequestrases in budding yeast: CytoQ by the small heat-shock protein Hsp42, and INQ by the small heat-shock protein Btn2.
The small heat shock protein (sHsp) class of chaperones (Figure 2) consist of a structured α-crystallin domain (ACD) flanked by unstructured regions on the 5’ (NTD) and 3’ (CTD) ends (Haslbeck and Vierling, 2015). Interactions between these disordered regions and the ACD enable sHSP oligomerization, forming a range of species from dimers to 40-mers (Clouser et al., 2019; Mühlhofer et al., 2021). The exact mechanism of substrate binding varies between clients, but can involve binding sites in either the unstructured termini or the structured domain (Reinle et al., 2022). Unlike many other molecular chaperones, sHSPs do not hydrolyze ATP. Instead it is thought that they form a shell around misfolded proteins, thus preventing the substrate from forming aggregates (Friedrich et al., 2004; Shi et al., 2013; Żwirowski et al., 2017; Johnston et al., 2021; Mühlhofer et al., 2021). While sHSPs can form large oligomers, it is actually thought that oligomerization can inhibit sHSP activity by sequestering substrate-binding sites (Franzmann et al., 2008; Peschek et al., 2013; Jovcevski et al., 2015; Freilich et al., 2018).
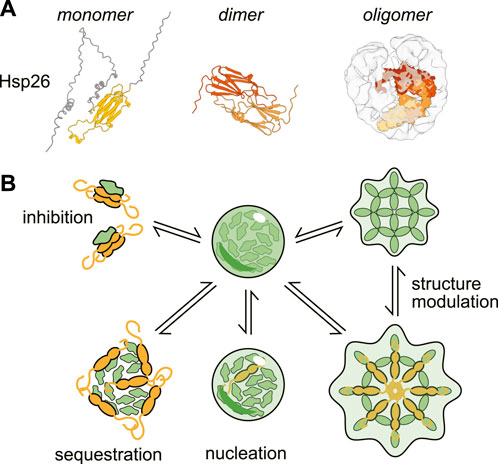
Figure 2. Structure and activities of a canonical small heat-shock protein (sHSP). (A) Structures of the small heat shock protein Hsp26 as a monomer (AlphaFold prediction (Jumper et al., 2021; Varadi et al., 2022)), dimer (only structured α-crystallin domain shown, ColabFold prediction (Mirdita et al., 2022) and oligomer (cryo-EM structure with modeled monomers of Hsp26 (Mühlhofer et al., 2021)). (B) Examples of the ways small heat shock proteins can modulate condensation.
Like many small heat-shock proteins, Btn2 forms large oligomers in the absence of stress (Ho et al., 2019). Although the precise structure of these oligomers remains unclear, Btn2 may behave similarly to other sHsps, with partial oligomer breakdown creating interaction sites for substrates (Haslbeck et al., 2019). In certain models of complete oligomers, such as polyhedral structures, the intrinsic multivalence of such incomplete oligomers could provide a scaffold for recruiting multiple substrates, driving sequestration, perhaps through nucleation. In addition to its role in INQ formation, Btn2 sequesters prion-associated Ure2p amyloid filaments in mother cells, such that after cell division, some daughter cells are cured of the prion (Kryndushkin et al., 2008; Son and Wickner, 2022).
An important clue to Btn2’s structure and function comes from its paralog in Saccharomyces cerevisiae, Cur1, which shares the Btn2 ACD but lacks Btn2’s long, disordered C-terminal domain (CTD) (Miller et al., 2015). Deletion of Btn2 disrupts INQ and CytoQ formation, and overexpression of Btn2 promotes formation of both structures, whereas deletion of Cur1 has a minimal effect whether deleted or overexpressed (Malinovska et al., 2012). Together, these results suggest that it is Btn2’s disordered CTD that is crucial for its sequestrase activity. A similar result holds for Hsp42, whose N-terminal prion-like disordered domain is required for CytoQ formation and aggregase activity in vitro (Grousl et al., 2018).
Hsp42 is the primary sequestrase active in the yeast cytoplasm, driving CytoQ formation—earlier called peripheral aggregates or Q-bodies—in response to proteotoxic conditions (Specht et al., 2011; Malinovska et al., 2012; Grousl et al., 2018). Like other sHSPs, it forms oligomers, with the most-populated wild-type state consistent with a 10-mer (Grousl et al., 2018). Unlike other sHSPs, these oligomers remain intact even upon heat stress (Haslbeck et al., 2004). Hsp42 and Btn2 both help to suppress the aggregation and toxicity of stress-induced aggregating proteins (Carter et al., 2023), although their endogenous targets outside of prion proteins remain obscure. We now turn our attention toward the roles of both proteins in regulating condensation and the focus-forming compartments CytoQ and INQ.
What is the relationship between condensation promoted by sequestrase action and the appearance of cellular foci? The naive expectation—that they are two expressions of the same underlying phenomenon—may not hold, as it does not hold in other related systems. Cycloheximide (CHX) inhibits CytoQ formation (Grousl et al., 2018), interpreted to mean that newly synthesized polypeptides make up the bulk of CytoQ substrates (Boczek and Alberti, 2018). However, disruption by CHX is a hallmark of other structures, notably P bodies (Sheth and Parker, 2003; Cougot et al., 2004; Kshirsagar and Parker, 2004) and stress granules (Mazroui et al., 2002; Mollet et al., 2008; Zhou et al., 2014; Wallace et al., 2015), with a different interpretation: that these structures depend on ribosome-free RNA for their formation. A key result is that translation inhibition with cycloheximide or emetine, which lock ribosomes on mRNAs, prevents granule formation, whereas inhibition with puromycin, which releases ribosomes from mRNAs, promotes granule formation (Glauninger et al., 2022). To our knowledge, a comparable experiment for either CytoQ or INQ has not been performed. It may be useful to consider the possibility that these compartments are the result of elaborate cell-biological processes, and that the in vitro aggregation experiments probe only early stages of subassembly formation, as has been found for stress granules (Wallace et al., 2015; Glauninger et al., 2022).
Questions regarding the seeding of condensed structures naturally lead to the core concept of nucleation. In the above examples, mRNA may serve as a nucleus (formally) or a scaffold (less formally) on which other biomolecules condense. sHsps, in their role as sequestrases, may use nucleation to spatially direct condensation; a simple cellular strategy would be to direct sequestrases to the compartments in which they should promote condensation. Indeed, deletion of Btn2, compromising INQ, can be rescued by expressing Hsp42-NLS, a construct which drives cytosolic Hsp42 into the nucleus using a nuclear localization sequence (NLS) (Ho et al., 2019).
Nucleation is likely to be the principal kinetic barrier to formation of many intracellular condensates (Martin et al., 2021; Shimobayashi et al., 2021). Condensation without a nucleation barrier can occur under conditions where nuclei form spontaneously and, as a result, condensation proceeds immediately everywhere in the solution—spatial control of where condensates form is lost. Thus, biological systems in which spatial control is beneficial will tend to have nucleation barriers. And similar to many familiar examples, from snowflakes nucleated by dust grains to steam bubbles nucleated by boiling chips, nucleation sites can be provided by auxiliary and often lower-abundance components present with the condensing species. This separation of roles creates a key niche for chaperones to act as sequestrases.
Condensates can be directed to specific locations not just by nucleation (directing formation), but also by tethering. Here, the subcellular tethering of age-associated aggregates by the Hsp40 chaperone Ydj1 provides an illuminating example. Aging yeast cells gradually accumulate protein aggregates consistent with CytoQ bodies (e.g., marked by Hsp104) (Saarikangas and Barral, 2015). Rejuvenation of new daughter cells depends on asymmetric retention of these aggregates in the aging mother cell. Retention depends on diffusion barriers (Clay et al., 2014) and also on Ydj1, with a twist: although abundant in the cytosol, Ydj1 has a subpopulation which is farnesylated and tethered to the cytoplasmic side of the ER membrane. Disruption of this tethered Ydj1 population compromises asymmetric large-aggregate retention, perhaps by client-mode binding of smaller aggregate precursors (Saarikangas et al., 2017).
Less well-studied relative to quality-control structures are foci—putative condensates—formed by the protein Std1 which form under glucose-replete conditions and disassemble when cells switch to respiration (Simpson-Lavy et al., 2017). Here, remarkably, the disaggregase Hsp104 acts as a sequestrase: Δhsp104 cells cannot form Std1 foci, inhibiting Hsp104 with low levels of guanadinium hydrochloride reduces focus formation, and Hsp104 is recruited to, perhaps integral to, these foci (Simpson-Lavy et al., 2017). Hsp40 (Sis1) and Hsp70 (Ssa1) chaperones also contribute to focus formation and associated phenotypes, as do Btn2 and Cur1, suggesting the involvement of multiple aspects of the sequestrase and disaggregase systems in regulating these structures. That Std1 foci are present under what in most other studies is a non-stress, wild type condition, and that Std1 appears to be an endogenous substrate rather than a primary regulatory factor for these foci, makes this system particularly tantalizing. Further work, particularly at the biochemical level, is needed to clarify how apparently opposing functions from proteins best known for dispersal activities contribute to condensate assembly in this case.
Chaperones regulate the material state of condensates
In addition to their role in promoting the formation of condensates, chaperones also regulate the internal structure, dynamics, and other in situ features of condensates, which we broadly refer to as the material state. Most commonly, a single feature of condensate material state, dynamics, is measured using techniques such as fluorescence recovery after photobleaching (FRAP) and single particle tracking (Alshareedah et al., 2021; Wang et al., 2022). While such measurements do report on the dynamics of molecules in the condensates, they provide only limited insight into the underlying molecular structure of the condensate. For instance, solid-like materials can either be gels, which are characterized by cross-linked networks of polymers swollen with solvent, or as glasses, which are disordered materials with a high barrier to reorganization (Jawerth et al., 2020; Mittag and Pappu, 2022). Furthermore, even simple two-component systems can form complex structures with multiple distinct phases coexisting (Garaizar et al., 2022). Cellular biological condensate structures can contain hundreds of different components, and can have correspondingly complex internal structures and organizations. Furthermore, they may be composites of multiple types of condensates. Much research remains to be done linking these material states with the adaptive role of condensates in cells, but we will highlight some examples below in which chaperones have been shown to play an important role in modulating condensate structure and we will discuss the possible functional implications of this regulation.
A number of recent results have shown that chaperones can alter the material state of condensates by ‘fluidizing’ the structure. That is, chaperones increase the rate at which the condensate can internally reorganize. In multiple instances this fluidization appears to be important for keeping condensates in a material state that is easily dispersible. This is analogous to the long known function of small heat shock proteins in maintaining protein aggregates in a more easily re-solubilized state (Kampinga et al., 1994; Ehrnsperger et al., 1997; Lee et al., 1997; Cashikar et al., 2005; Ungelenk et al., 2016; Yoo et al., 2022).
For instance, molecular chaperones help maintain a liquid-like state for peri-nucleolar condensate-containing orphan ribosomal protein subunits (Ali et al., 2023). These condensates form in response to heat shock in both yeast and humans and they recruit molecular chaperones including the Hsp40 Sis1/DNAJB6. Hsp40s are an ATP-independent class of molecular chaperones that are thought to be primarily substrate recognizing adapters for the ATP-dependent Hsp70 chaperone (Kampinga et al., 2019). Ali et al. were able to study the condensates in a lysate system, where either adding a chemical inhibitor of Hsp70 or depleting ATP slows down the dynamics of the condensate. Depleting Sis1/DNAJB6 from the nucleus delays the dispersal of the ribosomal protein condensates during recovery from stress, matching a report that inhibition of Hsp70 delays resolubilization of ribosome biogenesis proteins after heat shock in human cells (Sui et al., 2022). Importantly this delayed dispersal affects the fitness of yeast, delaying the resumption of cell growth after stress (Ali et al., 2023).
A similar interplay between chaperones and condensates contributes to the resumption of growth for yeast spores after exiting dormancy (Plante et al., 2023). More than 100 proteins condense during spore dormancy and are then resolubilized upon the resumption of growth. Deletion of the small heat shock protein Hsp42 delays the dispersal of these condensates. While its mechanism of action is still unknown, Hsp42 may be acting to maintain proteins in a state that is more easily solubilized during recovery.
Condensates again regulate the material state of the selective autophagy receptor p62/SQSTM1, which forms condensates around damaged lysosomes in order to initiate their degradation via lysophagy (Gallagher and Holzbaur, 2023). Hsp27 is recruited into these condensates and when Hsp27 is depleted the condensates are less liquid-like (as measured by FRAP) and initiate lysophagy more slowly. This fits with results from a slightly different system showing that maintaining liquidity of condensates formed by autophagy cargo is important for efficient selective autophagy (Yamasaki et al., 2020).
Another chaperone-regulated condensate is the purinosome, a multienzyme condensate which forms upon changes in growth conditions which upregulate purine biosynthesis (An et al., 2008; French et al., 2013; Kyoung et al., 2015; Pedley et al., 2018; 2022). Two components of purinosomes, PPAT and FGAMS, directly interact with Hsp90 (Pedley et al., 2018). Furthermore, inhibiting Hsp70 or Hsp90, another ATP-dependent molecular chaperone, prevents the formation of intact purinosomes and instead triggers the formation of condensates containing FGAMS alone (French et al., 2013). These FGAMS condensates are more solid-like than normal purinosomes, as assayed by their reduced sphericity (Pedley et al., 2022). Because the FGAMS condensates do not contain the entire suite of enzymes necessary for purine biosynthesis, Hsp90 is likely playing an important role in maintaining functional purinosome condensates. However, as is the case with the ribosomal protein condensates above, the mechanism by which chaperones tune the condensate properties of the purinosome remains to be uncovered.
Chaperones have also been shown to play a key role in modulating the material state of multiple condensing proteins involved in neurodegenerative disease, including FUS and TDP-43. FUS and TDP-43 are normally nuclear proteins involved in RNA metabolism, but are found in cytoplasmic inclusions in degenerating neurons that are key hallmarks of the neurodegenerative diseases amyotrophic lateral sclerosis (ALS) and frontotemporal dementia (FTD) (Harrison and Shorter, 2017). Mutations in FUS which alter its phase-separating properties are linked to ALS and FTD, suggesting that the regulation of FUS condensate structure is important for maintaining healthy neurons (Murakami et al., 2015; Patel et al., 2015; Harrison and Shorter, 2017; Murray et al., 2017; Portz et al., 2021). While reconstituted FUS and TDP-43 form condensates that are initially liquid-like, they harden and develop into amyloid-like fibrils over time (Kato et al., 2012; Molliex et al., 2015; Patel et al., 2015; Gasset-Rosa et al., 2019). This tendency could be due to the high protein concentrations experienced in liquid condensates which promote oligomer formation. Due to their importance for neurodegenerative diseases, a number of studies have looked at chaperone involvement in preventing and dispersing aggregates and condensates of amyloid-prone RNA-binding proteins including FUS, TDP-43 and Ataxin-2 (Ciechanover and Kwon, 2017). We will not cover the large body of literature examining the role of chaperones in preventing and dispersing misfolded aggregates and amyloid fibers of these and other proteins (Wentink et al., 2019), but will rather focus on the interaction between chaperones and the more liquid-like condensates of these proteins.
A number of chaperones have been shown to help maintain this liquid-like state of FUS and TDP-43 and prevent amyloid fiber formation. For FUS, this includes ATP-independent chaperones like the Hsp40 chaperone DNAJB1 and the small heat shock proteins Hsp27 and HspB8 (Gu et al., 2020; Liu et al., 2020; Boczek et al., 2021; Li et al., 2022). The ATP-dependent Hsp70 chaperones HSPA1A and HSPA8 are also reported to prevent amyloid formation of FUS condensates (Li et al., 2022). Similarly, HspB1, another small heat shock protein, helps maintain TDP-43 condensates in a liquid-like state (Lu et al., 2022). In cells, depleting HspB1 delayed the dispersal of TDP-43 condensates, again emphasizing the potential role of material state in regulating the dispersal kinetics of condensates (Lu et al., 2022).
In general, the ATP-independent chaperones help to maintain liquid-like states by first partitioning into condensates and then interfering with the protein-protein interactions that drive oligomer formation and the transition to a more solid-like state. For instance, both Hsp27 and HspB8 use their unstructured N-terminal domains (NTDs) to interact with FUS condensates (Liu et al., 2020; Boczek et al., 2021). For Hsp27 this interaction is tuned by stress-induced phosphorylation in the NTD of Hsp27(Liu et al., 2020). While unphosphorylated Hsp27 completely prevents FUS condensation in vitro, a phosphomimetic variant of Hsp27 rather co-partitions with the FUS condensate. Meanwhile, HspB8 does not prevent FUS condensation, but does partition into them (Boczek et al., 2021). Removing the CTD largely prevented multimerization of Hsp27, but did not abolish chaperone activity, consistent with previous studies showing that oligomerization of Hsp27 actually inhibits chaperone activity (Franzmann et al., 2008; Peschek et al., 2013; Jovcevski et al., 2015; Freilich et al., 2018; Mühlhofer et al., 2021). Intriguingly, it was recently reported that purified Hsp40 proteins can form condensates themselves (Gu et al., 2020). In addition, the same disordered region of the protein that enables homotypic condensates also promotes co-condensation of Hsp40 with FUS, while a different domain is required to prevent FUS fibrilization. Similarly, while the NTDs of Hsp27 and HspB8 are crucial for controlling the interaction of the chaperone with the FUS condensate, it is actually the α-crystallin-like domain (ACD) which is required to modulate the material state of the condensate (Liu et al., 2020; Boczek et al., 2021). The ACD interacts with the RNA recognition motif (RRM) domain of FUS, which is not normally involved in FUS condensation and may prevent the local RRM unfolding which has been hypothesized to drive fibril formation (Boczek et al., 2021).
As regards TDP-43, HspB1 binds to a region which is thought to form a transient helix involved in phase separation, suggesting that it may be acting to regulate the material state by directly weakening the interactions involved in promoting condensation. This same region of TDP-43 is bound by a variety of chaperones in vitro including Hsp40, Hsp70 and Hsp90 type chaperones (Carrasco et al., 2023). HspB1 also binds to the RRM domain of TDP-43, which, as it is for FUS, appears to be important for amyloid fibril formation (Chen et al., 2019; French et al., 2019). This is also reminiscent of the condensation mechanism of poly(A)-binding protein (Pab1), in which interactions between locally unfolded RRM domains drives assembly (Chen et al., 2024). These results highlight that it is not just interactions between chaperones and disordered regions that regulate condensation, but that interactions with seemingly folded domains can also be critical for modulating condensation formation and structure.
While cytoplasmic TDP-43 condensates are enriched for HspB1, TDP-43 also forms nuclear condensates which are associated with Hsp70s rather than small HSPs(Udan-Johns et al., 2014; Gu et al., 2021; Yu et al., 2021; François-Moutal et al., 2022). Yu et al. found that RNA-binding deficient TDP-43 forms condensates with a remarkable internal structure, which they termed an “anisosome” (Yu et al., 2021). The TDP-43 anisosome contains a liquid-like core of Hsp70 and is surrounded by a shell of ordered (and hence anisotropic), but liquid-like TDP-43. This structure is dependent on the ATPase activity of the Hsp70 chaperone, as inhibiting the chaperone in vivo causes the structure to become a well-mixed liquid that ages over time to a more solid-like state (Gu et al., 2021). The anisome structure is not unique to TDP-43, as Zhu et al. recently identified a similar structure with a core of Hsp70 and a shell of the nuclear protein REV-ERBα (Zhu et al., 2023). REV-ERBα is involved in the repression of the circadian clock in mouse livers, and the anisosome condensates appear to be important for this repressive function. Further research is needed to identify other proteins which may form chaperone-associated anisomes, and to determine both the molecular mechanisms of how they form and what the functional consequences of this intricate structure is.
Chaperones inhibit and dissolve condensates
The best-studied function of molecular chaperones is to prevent and disperse aggregates of misfolded proteins. Chaperones accomplish this task by disrupting unwanted protein-protein interactions and untangling networks of protein polymers (Hartl et al., 2011). These same functions make chaperones ideal regulators of biomolecular condensation, which similarly involve a multitude of protein-protein interactions and potentially interwoven networks of biological polymers. In fact, as discussed below, chaperones appear to be much more efficient at clearing biomolecular condensates than at dispersing aggregates of misfolded proteins (Yoo et al., 2022). While there are some constitutive condensates in cells, such as the nucleolus, many condensates form in response to an environmental signal. Once the signal has dissipated or the cell has regained homeostasis, the induced condensate will then be dissolved.
Stress granules are a key example of a signal-induced condensate which is known to be dispersed by molecular chaperones. Stress granules are assemblies of proteins and RNA that form in the cytosol in response to cellular stresses such as hypoxia, starvation, heat shock, oxidative stress and viral infection (Glauninger et al., 2022). They are often defined by the visible appearance of foci containing mRNA and key marker proteins such as G3BP1/2, poly(A)-binding protein (Pab1) and other translation initiation factors such as the eIF3 complex. However, their composition is complex and stress-dependent, making a precise definition of stress granules difficult. Reflecting this ambiguity, the function of stress granules is an active area of research with proposed functions ranging from regulating translation and RNA metabolism to serving as a platform for innate immune signaling (White and Lloyd, 2012; Reineke et al., 2015; Deater et al., 2022; Mateju and Chao, 2022). Recently they have even been shown to play a key role in maintaining the integrity of endolysosomal membranes (Bussi et al., 2023).
Stress granules are thought to assemble in stages, driven by the condensation of multiple different proteins with RNA. Many of these proteins, including G3BP1, PABP, and Ded1/DDX3, condense in a test tube in response to physiological triggers (e.g., heat shock or long ribosome-free RNA) (Riback et al., 2017; Kroschwald et al., 2018; Guillén-Boixet et al., 2020; Iserman et al., 2020; Yang et al., 2020). For instance, purified Pab1 forms condensates when exposed to heat shock in a test tube (Riback et al., 2017). The condensation of Pab1 is not driven by its intrinsically disordered regions, though they do play an important role in modulating the condensation behavior. Rather the assembly process involves interactions between locally unfolded regions of Pab1’s RRM domains (Chen et al., 2024). These condensates are initially liquid-like, but quickly mature into a more solid-like state which does not dissolve after the protein has been returned to room temperature. This is similar to the in vitro behavior of Ded1, another stress granule component which condenses in response to elevated temperature (Iserman et al., 2020).
Stress granules also do not immediately resolve upon the cessation of the stress. Thus both stress granules in cells and stress granule proteins in vitro demonstrate hysteresis, where the state of the system is influenced not only by its current conditions but also by past conditions. As we will discuss below, this property provides a useful regulatory handle by which chaperones can control the adaptation process of cells (Figure 3). Mechanistically, it could reflect a strong kinetic barrier to dissolution (Figure 1).
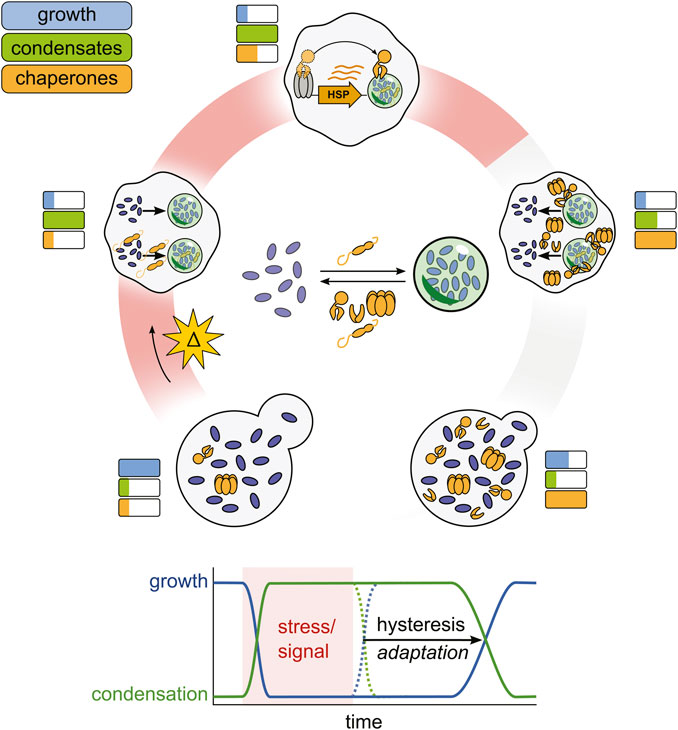
Figure 3. A proposed chaperone-enabled adaptive cycle of condensation. Stressful changes in cellular environments arrest cellular growth and trigger the formation of condensates, nucleated by small heat shock proteins. Stress-induced condensates then help to upregulate chaperone production, which begin to antagonize condensate formation. When stress ends, condensates do not spontaneously dissolve, but are dispersed by stress-induced chaperones, restoring growth. This lag (hysteresis) is adaptive, enabling cells to prepare for successive stresses and ensuring a successful response to stress before growth restarts.
Stress granules are dissolved through a combination of at least two pathways: clearance and degradation by autophagy and resolubilization of the components by molecular chaperones (Buchan et al., 2013; Ganassi et al., 2016; Gwon et al., 2021). In yeast, condensation of proteins into stress-induced assemblies after a brief 42°C heat shock is completely reversible, suggesting that, at least for such short-term stresses, autophagy is not required for dissolution. Instead, dissolution is driven by the Hsp40/Hsp70/Hsp104/Hsp110 molecular chaperone pathway (Cherkasov et al., 2013; Kroschwald et al., 2015; Walters et al., 2015; Kroschwald et al., 2018; Yoo et al., 2022; To et al., 2023). In this pathway, the chaperones Hsp70, together with its co-chaperones Hsp40 and Hsp110, deliver substrates to the ring-shaped AAA+ unfoldase Hsp104, which uses energy from ATP hydrolysis to thread proteins through its central pore and resolubilize its substrates (Hartl et al., 2011).
Yoo et al. recently reconstituted this process in vitro using Pab1 condensates as a model substrate (Yoo et al., 2022). The full complement of chaperones from the pathway are required for maximal efficiency, but a combination of Hsp40, Hsp70 and either Hsp104 or Hsp110 is sufficient to reconstitute dispersal activity, with Hsp104-driven dispersal being significantly faster than that driven by Hsp110. While type I (Ydj1 in yeast) and type II (Sis1) Hsp40 proteins act synergistically in the disassembly of luciferase aggregates, only the type II Hsp40 is able to disperse Pab1 condensates (Nillegoda et al., 2015; Nillegoda et al., 2017). Another key point is that Hsp70, despite being able to catalytically hydrolyze ATP, needs to be present in super-stoichiometric abundance in order to disperse the condensates. This fits with other studies showing that multiple Hsp70s need to bind to a substrate in order to activate Hsp104 (Seyffer et al., 2012; Carroni et al., 2014). Similarly, even in the absence of Hsp104, multiple Hsp70s cluster together in order to disperse amyloid substrates (Wentink et al., 2020). In order to understand the regulation of stress granules and other condensates, more research is needed to identify the mechanisms enabling particular chaperones to disperse particular substrates.
A surprising finding was that dispersal of Pab1 condensates is orders of magnitude faster than dispersal of aggregates of luciferase, a commonly used model substrate, formed under similar conditions (Yoo et al., 2022). Computational modeling suggests that the difference in efficiency might be attributable to a higher dispersal rate of the substrate, and/or to the fact that, in contrast to luciferase, Pab1 remains monomeric after solubilization and does not reaggregate or reenter the condensate. Supporting contributions of the second mechanism, intrinsically disordered regions aid in refoldability of proteins, and condensing proteins in budding yeast are particularly adept at reforming their native structure after being unfolded (To et al., 2023). This suggests that proteins in condensates may have evolved properties such as fast refolding which allow them to be easily dispersed by molecular unfoldases like Hsp104.
While stress granule dispersal in budding yeast requires Hsp104, metazoa have lost this chaperone and yet are still able to disperse stress granules independently of autophagy (Erives and Fassler, 2015; Mateju et al., 2017; Wang et al., 2019; Gwon et al., 2021). In humans, this dispersal is enabled by VCP, a AAA+ ring-shaped unfoldase that is structurally quite similar to Hsp104 (Gwon et al., 2021). VCP is recruited to stress granules through its adaptor FAF2, which binds to ubiquitinated G3BP1. This is analogous to the recruitment of Hsp104 to stress granules by Hsp40/Hsp70 and suggests that VCP may have adopted some of the functions in regulating condensates performed by Hsp104 in fungi and other eukaryotes.
The Hsp70 system still plays an important role in regulating stress granules in humans, however, as it helps to maintain stress granules in a dispersible state (Ganassi et al., 2016; Mateju et al., 2017). Depletion of the Hsp70 HSPA1A, its co-chaperone BAG3, and the small heat shock protein HspB8 all lead to the accumulation of defective ribosomal products (prematurely terminated polypeptides) in stress granules, which in turn changes their material state and delays their dispersal (Ganassi et al., 2016). Similarly, overexpression of misfolded proteins, such as ALS-associated variants of the protein SOD1 leads to aberrant stress granules which are particularly dependent on chaperones for their dispersal (Mateju et al., 2017). Thus chaperones act across the life-cycle of stress granules to maintain their proper structural state, enabling them to be efficiently dispersed once the stress is relieved.
In addition to direct dispersal, chaperones can also control stress granules via indirectly modulating stress granule regulators. In particular, the active state of DYRK3, a kinase which regulates stress granule dissolution, is maintained by the ATP-dependent chaperone Hsp90 (Wippich et al., 2013; Mediani et al., 2021). Upon stress, DYRK3 is inactivated, disassociates from Hsp90 and is targeted to stress granules. During recovery, Hsp90 re-engages with DRYK3, reactivating it and further promoting stress granule dissolution. This system thus acts as a feed-forward system, sharpening the transitions between stress granule formation and dissolution. It also further ties chaperone activity to stress granules.
Stress granules are not the only condensate dispersed by the Hsp40/Hsp70 system. Another example of such condensates are those formed by RIα, a cAMP-dependent protein kinase (PKA) which aids in the regulation of cAMP signaling (Zhang et al., 2020). In turn, cAMP signaling regulates numerous pathways in cells. In liver cancer fibrolamellar carcinoma RIα is fused to the Hsp40 DNAJB1, which recruits Hsp70 and leads to the dispersal of RIα condensates. Similarly, direct targeting of Hsp70 to model condensates of RIα or EML4-Alk via de novo designed Hsp40 mimics also led to condensate dispersal (Zhang et al., 2023). This result opens up the exciting possibility of using chaperones as designer tools for modulating specific condensates in cells. Such tools would be similar to a number of variants of Hsp104 which have been specifically designed to disperse toxic aggregate associated with neurodegenerative diseases (Jackrel et al., 2014; Mack et al., 2023).
While the chaperones discussed above are general protein chaperones or unfoldases, a number of studies have highlighted the unexpected chaperone activities of other condensate binding proteins such as nuclear/cytoplasmic transport machinery (Guo et al., 2018; Hofweber et al., 2018; Qamar et al., 2018; Yoshizawa et al., 2018; Guo et al., 2019; Bourgeois et al., 2020; Hutten et al., 2020; Niaki et al., 2020; Baade et al., 2021; Khalil et al., 2022; Odeh et al., 2022; Rhine et al., 2022; Boeynaems et al., 2023; Thomas et al., 2023). Many of the RNA-binding proteins whose aberrant condensation is associated with neurodegenerative diseases, including FUS, TDP-43 and hnRNPA1, are trafficked into the nucleus via importins like Karyopherin-β2 (Kapβ2 or transportin 1) and KPNB1. Importins bind to proteins containing a nuclear localization sequence, and then use the energy from a concentration gradient of their regulator RAN to facilitate directional travel across the nuclear pore. Surprisingly, importin binding to FUS, TDP-43, hnRNPA1 and cold-inducible RNA-binding protein (CIRBP) inhibits their condensation and fibrilization both in vivo and in vitro (Guo et al., 2018; Hofweber et al., 2018; Qamar et al., 2018; Yoshizawa et al., 2018; Bourgeois et al., 2020; Hutten et al., 2020; Niaki et al., 2020; Baade et al., 2021; Khalil et al., 2022; Rhine et al., 2022). Even more impressive, despite having no ATP hydrolysis activity, Kapβ2 can dissolve preformed fibrils of FUS and hnRNPA1 in vitro (Guo et al., 2018; Fare et al., 2023). It accomplishes this dispersal by interacting with the substrate not just at the NLS but at multiple sites within the protein and thereby competing with the interactions that drive condensation and fibrilization (Guo et al., 2018; Yoshizawa et al., 2018; Fare et al., 2023). Similarly, the exportin CRM1 inhibits the formation of condensates of FG repeat-containing nucleoporins that appear when the nucleoporins are expressed at high levels (Milles et al., 2013; Thomas et al., 2023).
In a different example of unexpected chaperoning activity, the stress granule marker Pab1 (or PABPC in humans) also serves as a chaperone to prevent spontaneous condensation of ataxin-2 and to direct its localization to stress granules during cellular stress (Boeynaems et al., 2023). PABPC binds to a conserved short linear motif (SLiM) in ataxin-2, and is proposed to function by bringing ataxin-2 to RNA, which then competes with the multivalent interactions that otherwise lead to spontaneous condensation. In the context of stress, PABPC then acts as an emulsifier to allow ataxin-2 mixing with other stress granule components. In the absence of the SLiM, and thus PABPC binding, ataxin-2 does not mix with stress granules but rather forms condensates that dot the outside of stress granules. Together with the nuclear transport results, these findings force us to expand our definition of condensate chaperone beyond the traditional heat shock proteins.
Biology enabled by chaperone-regulated condensation
The functions of chaperones in regulating condensates are, unsurprisingly, both as varied and as poorly understood as the functions of condensates themselves—determination of which is currently (and properly) considered a central aim of condensate biology.
We begin by noting and nodding to the standard protein quality control literature, extensively cited above, which has illuminated the many ways in which chaperones may act in the context of condensates to reduce the cellular impact of misfolded proteins. Prevention and dispersal of toxic condensates/aggregates, formation of quality control compartments such as the INQ and CytoQ, retention of similar bodies in aging cells, and alteration of the dispersability of aggregates by sHSPs represent the major activities attributable to chaperones and reviewed extensively above and elsewhere (Miller et al., 2015; Sontag et al., 2017; Haslbeck et al., 2019; Kumar et al., 2022). From the perspective of condensate biology, the key principles underlying the chaperone-regulated features of these systems are the sequestration of potentially toxic species into defined structures where their processing can be controlled and toxicity limited. A major open question is the degree to which these same structures and strategies apply to endogenous, adaptive condensing proteins—where, for example, sequestration of an activity which is deleterious in certain circumstances may follow the same logic as sequestration of toxicity.
Another relatively well-understood functional role of chaperones in regulating condensates is chaperone-mediated propagation of prions (Chakrabortee et al., 2016; Chakravarty et al., 2020; Itakura et al., 2020; Garcia et al., 2021; Saad and Jarosz, 2021). Prions are proteins which are able to propagate a particular conformational state, usually that of a multimeric assembly, across generations. These inherited states can have important functional consequences for cells, such as balancing a trade-off between proliferation and lifespan (Garcia et al., 2021). Many prions are deleterious, and chaperones form a key part of antiprion systems, in part by sHSP-mediated sequestration of potentially toxic prion assemblies to prevent their passage to younger cells (Kryndushkin et al., 2008; Son and Wickner, 2022). In other cases, chaperones break up prion assemblies which otherwise could not be transmitted, creating transmissible seeds which template future assembly growth (Shorter and Lindquist, 2004). The degree to which prions and condensates can and should be considered related phenomena remains somewhat hazy, but the understanding of condensation has been profoundly shaped by studies of prions, prion-like domains, sequestrases in a prion context, etc., enough to warrant substantial further attention.
Moving beyond quality control and toxicity, yet still in the realm of stress biology, two general functions of chaperones interacting with condensates have become clear in recent studies: regulating the timing of events following stress, and a crucial role in regulating responses to stress.
First, a common theme among stress-induced condensates is that their dissolution corresponds temporally to resumptions in the cellular processes which were suppressed during the stress. By regulating the timing of this dissolution, chaperones are playing a vital role in gatekeeping the return to normal cellular homeostasis. For instance, resumption of translation and the cell cycle after heat shock corresponds well with the dissolution of stress granules in yeast (Cherkasov et al., 2013; Kroschwald et al., 2018). It was also recently shown that in budding yeast availability of the chaperone Ydj1 regulates the cell-cycle (Moreno et al., 2019). Sequestration of Ydj1 into stress granules may thus directly delay resumption of the cell-cycle (Walters et al., 2015). Stress also triggers condensation of the ATP-producing enzyme Cdc19 into reversible amyloids which inhibit its function and must be disassembled before growth can restart (Cereghetti et al., 2021). Delaying the resolution of stress granules, on the other hand, delays the resumption of translation (Wippich et al., 2013; Maxwell et al., 2021). Similarly, delaying the resolution of orphan ribosomal protein condensates delays resumption of growth after heat shock (Ali et al., 2023). Another example comes from restarting growth in budding yeast spores, which corresponds with a large-scale resolubilization of proteins which had condensed during dormancy (Plante et al., 2023).
Second, chaperones appear to play a central role in transducing stress sensed by condensing proteins. Condensation and the more specific phenomenon of phase transitions represent an effective strategy to convert small changes in a relevant parameter (temperature, pH, etc.) into large-scale changes in intracellular organization (Yoo et al., 2019). However, to trigger downstream behavior, such sensory condensation must be read out, directly or indirectly. In the case of activation of Hsf1, the primary eukaryotic transcriptional regulator of the heat shock response, a mechanism for direct readout has already been described (Pincus, 2020). Under physiological growth conditions, Hsf1 is bound and repressed by the chaperone Hsp70(Zheng et al., 2016; Krakowiak et al., 2018). As new Hsp70 substrates emerge during stress, they titrate Hsp70 away from Hsf1, thus removing the inhibitory block and inducing chaperone induction. What are these substrates? One major source of new substrates for Hsp70 are nascent polypeptides which may misfold during cellular stress (Xu et al., 2016; Masser et al., 2019). However, even when translation is inhibited, stress leads to robust induction of the heat shock response (Triandafillou et al., 2020), suggesting that there is another source of Hsp70 substrates induced by stress. Indeed, poly(A)-binding protein (Pab1) both autonomously condenses in vivo and in vitro in response to temperature and pH changes (Riback et al., 2017), and when condensed is an endogenous substrate of the classic disaggregase system, including Hsp70 (Yoo et al., 2022). We hypothesize that many such stress-induced condensates, which are both abundant and interact strongly with chaperones during stress, also function as strong activators of Hsf1. Further supporting this adaptive role in regulating the stress response, we have recently shown that proteome-wide condensation in fungi is well-tuned to their evolutionary niches, with the temperature onset of condensation closely following the thermal niche of cryophilic, mesophilic and thermotolerant budding yeast species (Keyport Kik et al., 2024).
Temperature and pH trigger Pab1 condensation and, remarkably, similar structural rearrangements promoting condensation (Chen et al., 2024). Intracellular pH drops accompany multiple cellular stresses (Munder et al., 2016; Triandafillou et al., 2020), and have been proposed to be a second messenger for stress (Dechant et al., 2010). Generalized, these results suggest a relatively simple mechanism by which condensing proteins can respond to a diverse array of stresses, yet, via recruitment of chaperones acting as inhibitory factors on response proteins such as Hsf1, trigger convergent responses.
Given that chaperones appear to both be induced by stress-induced condensates and aid in their dispersal, we propose that chaperones and condensates have evolved as an adaptive monitoring system similar to negative feedback loops that govern many other signaling pathways (Figure 3) (Lake et al., 2016). To articulate the full picture, in this model stress triggers an arrest of growth and cellular processes such as translation, and also the induction of condensates, a process which itself is regulated by constitutive chaperones like small heat shock proteins (Rowley et al., 1993; Grousl et al., 2009). The condensates then aid in maintaining the repressed state by sequestering mRNA, translation initiation factors, and other proteins required for normal cellular growth (Riback et al., 2017; Bresson et al., 2020; Iserman et al., 2020). Condensates begin to recruit molecular chaperones away from Hsf1, relieving Hsf1 inhibition and triggering the induction of more molecular chaperones (Keyport Kik et al., 2024). This process continues until an equilibrium is established between condensate formation, condensate induction, and condensate dispersal. Autonomous stress-triggered condensation but facilitated dispersal dependent on limited chaperones produces hysteresis: condensates which form rapidly upon stress do not dissolve rapidly upon cessation of the triggering stress. Rather, condensates persist, generating a lag phase until sufficient chaperone activity exists to fully dissolve condensates. During this lag, the cell persists in a stress-specific state, with alterations in activities due to condensation then able to remodel the cell via selective transcription and translation to contend with or react appropriately to stress. Eventual condensate dissolution, in turn, corresponds with the resumption of the cell cycle and high-level translational activity (Cherkasov et al., 2013; Kroschwald et al., 2018; Moreno et al., 2019). This hysteretic system, in short, provides a simple mechanism by which the cell can generate specific responses and automatically detect a successful response, rather than merely the cessation of stress.
All of these functions, in their various states of illumination and mechanistic detail, provide merely a glimpse into the potential functions which may be executed by chaperones acting as regulators of condensation. We anticipate that the frame will continue to shift and expand to accommodate more adaptive mechanisms, more surprising reconsiderations of well-established chaperone activities, and a deeper understanding of condensate biology in the context of conserved regulatory machinery.
Author contributions
JB: Writing–review and editing, Writing–original draft, Visualization, Funding acquisition, Conceptualization. DD: Writing–review and editing, Writing–original draft, Visualization, Supervision, Funding acquisition, Conceptualization.
Funding
The author(s) declare that financial support was received for the research, authorship, and/or publication of this article. JB acknowledges support from the Helen Hay Whitney Foundation. DD acknowledges support from NIH (award GM144278). These funding agencies provided general support for research during which this review was written.
Conflict of interest
The authors declare that the research was conducted in the absence of any commercial or financial relationships that could be construed as a potential conflict of interest.
Publisher’s note
All claims expressed in this article are solely those of the authors and do not necessarily represent those of their affiliated organizations, or those of the publisher, the editors and the reviewers. Any product that may be evaluated in this article, or claim that may be made by its manufacturer, is not guaranteed or endorsed by the publisher.
References
Ali, A., Garde, R., Schaffer, O. C., Bard, J. A. M., Husain, K., Kik, S. K., et al. (2023). Adaptive preservation of orphan ribosomal proteins in chaperone-dispersed condensates. Nat. Cell Biol. 25, 1691–1703. doi:10.1038/s41556-023-01253-2
Alshareedah, I., Kaur, T., and Banerjee, P. R. (2021). Methods for characterizing the material properties of biomolecular condensates. Methods Enzym. 646, 143–183. doi:10.1016/bs.mie.2020.06.009
An, S., Kumar, R., Sheets, E. D., and Benkovic, S. J. (2008). Reversible compartmentalization of de novo purine biosynthetic complexes in living cells. Science 320, 103–106. doi:10.1126/science.1152241
Baade, I., Hutten, S., Sternburg, E. L., Pörschke, M., Hofweber, M., Dormann, D., et al. (2021). The RNA-binding protein FUS is chaperoned and imported into the nucleus by a network of import receptors. J. Biol. Chem. 296, 100659. doi:10.1016/j.jbc.2021.100659
Banani, S. F., Lee, H. O., Hyman, A. A., and Rosen, M. K. (2017). Biomolecular condensates: organizers of cellular biochemistry. Nat. Rev. Mol. Cell Biol. 18, 285–298. doi:10.1038/nrm.2017.7
Boczek, E. E., and Alberti, S. (2018). One domain fits all: using disordered regions to sequester misfolded proteins. J. Cell Biol. 217, 1173–1175. doi:10.1083/jcb.201803015
Boczek, E. E., Fürsch, J., Niedermeier, M. L., Jawerth, L., Jahnel, M., Ruer-Gruß, M., et al. (2021). HspB8 prevents aberrant phase transitions of FUS by chaperoning its folded RNA-binding domain. Elife 10, e69377. doi:10.7554/eLife.69377
Boeynaems, S., Dorone, Y., Zhuang, Y., Shabardina, V., Huang, G., Marian, A., et al. (2023). Poly(A)-binding protein is an ataxin-2 chaperone that regulates biomolecular condensates. Mol. Cell 83, 2020–2034.e6. doi:10.1016/j.molcel.2023.05.025
Boke, E., Ruer, M., Wühr, M., Coughlin, M., Lemaitre, R., Gygi, S. P., et al. (2016). Amyloid-like self-assembly of a cellular compartment. Cell 166, 637–650. doi:10.1016/j.cell.2016.06.051
Bourgeois, B., Hutten, S., Gottschalk, B., Hofweber, M., Richter, G., Sternat, J., et al. (2020). Nonclassical nuclear localization signals mediate nuclear import of CIRBP. Proc. Natl. Acad. Sci. U. S. A. 117, 8503–8514. doi:10.1073/pnas.1918944117
Brangwynne, C. P., Tompa, P., and Pappu, R. V. (2015). Polymer physics of intracellular phase transitions. Nat. Phys. 11, 899–904. doi:10.1038/nphys3532
Bresson, S., Shchepachev, V., Spanos, C., Turowski, T. W., Rappsilber, J., and Tollervey, D. (2020). Stress-induced translation inhibition through rapid displacement of scanning initiation factors. Mol. Cell 80, 470–484.e8. e8. doi:10.1016/j.molcel.2020.09.021
Buchan, J. R., Kolaitis, R.-M., Taylor, J. P., and Parker, R. (2013). Eukaryotic stress granules are cleared by autophagy and Cdc48/VCP function. Cell 153, 1461–1474. doi:10.1016/j.cell.2013.05.037
Bussi, C., Mangiarotti, A., Vanhille-Campos, C., Aylan, B., Pellegrino, E., Athanasiadi, N., et al. (2023). Stress granules plug and stabilize damaged endolysosomal membranes. Nature 623, 1062–1069. doi:10.1038/s41586-023-06726-w
Carrasco, J., Antón, R., Valbuena, A., Pantoja-Uceda, D., Mukhi, M., Hervás, R., et al. (2023). Metamorphism in TDP-43 prion-like domain determines chaperone recognition. Nat. Commun. 14, 466. doi:10.1038/s41467-023-36023-z
Carroni, M., Kummer, E., Oguchi, Y., Wendler, P., Clare, D. K., Sinning, I., et al. (2014). Head-to-tail interactions of the coiled-coil domains regulate ClpB activity and cooperation with Hsp70 in protein disaggregation. Elife 3, e02481. doi:10.7554/elife.02481
Carter, Z., Creamer, D., Kouvidi, K., and Grant, C. M. (2023). Sequestrase chaperones protect against oxidative stress-induced protein aggregation and [PSI+] prion formation. bioRxiv 10 (18), 562867. doi:10.1101/2023.10.18.562867
Cashikar, A. G., Duennwald, M., and Lindquist, S. L. (2005). A chaperone pathway in protein disaggregation: HSP26 alters the nature of protein aggregates to facilitate reactivation by HSP104. J. Biol. Chem. 280, 23869–23875. doi:10.1074/jbc.m502854200
Cereghetti, G., Wilson-Zbinden, C., Kissling, V. M., Diether, M., Arm, A., Yoo, H., et al. (2021). Reversible amyloids of pyruvate kinase couple cell metabolism and stress granule disassembly. Nat. Cell Biol. 23, 1085–1094. doi:10.1038/s41556-021-00760-4
Chakrabortee, S., Byers, J. S., Jones, S., Garcia, D. M., Bhullar, B., Chang, A., et al. (2016). Intrinsically disordered proteins drive emergence and inheritance of biological traits. Cell 167, 369–381.e12. doi:10.1016/j.cell.2016.09.017
Chakravarty, A. K., Smejkal, T., Itakura, A. K., Garcia, D. M., and Jarosz, D. F. (2020). A non-amyloid prion particle that activates a heritable gene expression program. Mol. Cell 77, 251–265.e9. e9. doi:10.1016/j.molcel.2019.10.028
Chen, H.-J., Topp, S. D., Hui, H. S., Zacco, E., Katarya, M., McLoughlin, C., et al. (2019). RRM adjacent TARDBP mutations disrupt RNA binding and enhance TDP-43 proteinopathy. Brain 142, 3753–3770. doi:10.1093/brain/awz313
Chen, R., Glauninger, H., Kahan, D. N., Shangguan, J., Sachleben, J. R., Riback, J. A., et al. (2024). HDX-MS finds that partial unfolding with sequential domain activation controls condensation of a cellular stress marker. Proc. Natl. Acad. Sci. U. S. A. 121, e2321606121. doi:10.1073/pnas.2321606121
Cherkasov, V., Hofmann, S., Druffel-Augustin, S., Mogk, A., Tyedmers, J., Stoecklin, G., et al. (2013). Coordination of translational control and protein homeostasis during severe heat stress. Curr. Biol. 23, 2452–2462. doi:10.1016/j.cub.2013.09.058
Choi, J.-M., Dar, F., and Pappu, R. V. (2019). LASSI: a lattice model for simulating phase transitions of multivalent proteins. PLoS Comput. Biol. 15, e1007028. doi:10.1371/journal.pcbi.1007028
Choi, J.-M., Holehouse, A. S., and Pappu, R. V. (2020). Physical principles underlying the complex biology of intracellular phase transitions. Annu. Rev. Biophys. 49, 107–133. doi:10.1146/annurev-biophys-121219-081629
Chuang, E., Hori, A. M., Hesketh, C. D., and Shorter, J. (2018). Amyloid assembly and disassembly. J. Cell Sci. 131, jcs189928. doi:10.1242/jcs.189928
Ciechanover, A., and Kwon, Y. T. (2017). Protein quality control by molecular chaperones in neurodegeneration. Front. Neurosci. 11, 185. doi:10.3389/fnins.2017.00185
Clay, L., Caudron, F., Denoth-Lippuner, A., Boettcher, B., Buvelot Frei, S., Snapp, E. L., et al. (2014). A sphingolipid-dependent diffusion barrier confines ER stress to the yeast mother cell. Elife 3, e01883. doi:10.7554/elife.01883
Clouser, A. F., Baughman, H. E., Basanta, B., Guttman, M., Nath, A., and Klevit, R. E. (2019). Interplay of disordered and ordered regions of a human small heat shock protein yields an ensemble of “quasi-ordered” states. Elife 8, e50259. doi:10.7554/eLife.50259
Cougot, N., Babajko, S., and Seraphin, B. (2004). Cytoplasmic foci are sites of mRNA decay in human cells. J. Cell Biol. 165, 31–40. doi:10.1083/jcb.200309008
Deater, M., Tamhankar, M., and Lloyd, R. E. (2022). TDRD3 is an antiviral restriction factor that promotes IFN signaling with G3BP1. PLoS Pathog. 18, e1010249. doi:10.1371/journal.ppat.1010249
Dechant, R., Binda, M., Lee, S. S., Pelet, S., Winderickx, J., and Peter, M. (2010). Cytosolic pH is a second messenger for glucose and regulates the PKA pathway through V-ATPase. EMBO J. 29, 2515–2526. doi:10.1038/emboj.2010.138
Dorone, Y., Boeynaems, S., Flores, E., Jin, B., Hateley, S., Bossi, F., et al. (2021). A prion-like protein regulator of seed germination undergoes hydration-dependent phase separation. Cell 184, 4284–4298.e27. e27. doi:10.1016/j.cell.2021.06.009
Ehrnsperger, M., Gräber, S., Gaestel, M., and Buchner, J. (1997). Binding of non-native protein to Hsp25 during heat shock creates a reservoir of folding intermediates for reactivation. EMBO J. 16, 221–229. doi:10.1093/emboj/16.2.221
Erives, A. J., and Fassler, J. S. (2015). Metabolic and chaperone gene loss marks the origin of animals: evidence for Hsp104 and Hsp78 chaperones sharing mitochondrial enzymes as clients. PLoS One 10, e0117192. doi:10.1371/journal.pone.0117192
Fare, C. M., Rhine, K., Lam, A., Myong, S., and Shorter, J. (2023). A minimal construct of nuclear-import receptor Karyopherin-β2 defines the regions critical for chaperone and disaggregation activity. J. Biol. Chem. 299, 102806. doi:10.1016/j.jbc.2022.102806
François-Moutal, L., Scott, D. D., Ambrose, A. J., Zerio, C. J., Rodriguez-Sanchez, M., Dissanayake, K., et al. (2022). Heat shock protein Grp78/BiP/HspA5 binds directly to TDP-43 and mitigates toxicity associated with disease pathology. Sci. Rep. 12, 8140. doi:10.1038/s41598-022-12191-8
Franzmann, T. M., Menhorn, P., Walter, S., and Buchner, J. (2008). Activation of the chaperone Hsp26 is controlled by the rearrangement of its thermosensor domain. Mol. Cell 29, 207–216. doi:10.1016/j.molcel.2007.11.025
Freilich, R., Betegon, M., Tse, E., Mok, S.-A., Julien, O., Agard, D. A., et al. (2018). Competing protein-protein interactions regulate binding of Hsp27 to its client protein tau. Nat. Commun. 9, 4563. doi:10.1038/s41467-018-07012-4
French, J. B., Zhao, H., An, S., Niessen, S., Deng, Y., Cravatt, B. F., et al. (2013). Hsp70/Hsp90 chaperone machinery is involved in the assembly of the purinosome. Proc. Natl. Acad. Sci. U. S. A. 110, 2528–2533. doi:10.1073/pnas.1300173110
French, R. L., Grese, Z. R., Aligireddy, H., Dhavale, D. D., Reeb, A. N., Kedia, N., et al. (2019). Detection of TAR DNA-binding protein 43 (TDP-43) oligomers as initial intermediate species during aggregate formation. J. Biol. Chem. 294, 6696–6709. doi:10.1074/jbc.ra118.005889
Friedrich, K. L., Giese, K. C., Buan, N. R., and Vierling, E. (2004). Interactions between small heat shock protein subunits and substrate in small heat shock protein-substrate complexes. J. Biol. Chem. 279, 1080–1089. doi:10.1074/jbc.m311104200
Gallagher, E. R., and Holzbaur, E. L. F. (2023). The selective autophagy adaptor p62/SQSTM1 forms phase condensates regulated by HSP27 that facilitate the clearance of damaged lysosomes via lysophagy. Cell Rep. 42, 112037. doi:10.1016/j.celrep.2023.112037
Ganassi, M., Mateju, D., Bigi, I., Mediani, L., Poser, I., Lee, H. O., et al. (2016). A surveillance function of the HSPB8-BAG3-HSP70 chaperone complex ensures stress granule integrity and dynamism. Mol. Cell 63, 796–810. doi:10.1016/j.molcel.2016.07.021
Garaizar, A., Espinosa, J. R., Joseph, J. A., Krainer, G., Shen, Y., Knowles, T. P. J., et al. (2022). Aging can transform single-component protein condensates into multiphase architectures. Proc. Natl. Acad. Sci. U. S. A. 119, e2119800119. doi:10.1073/pnas.2119800119
Garcia, D. M., Campbell, E. A., Jakobson, C. M., Tsuchiya, M., Shaw, E. A., DiNardo, A. L., et al. (2021). A prion accelerates proliferation at the expense of lifespan. Elife 10, e60917. doi:10.7554/eLife.60917
Gasset-Rosa, F., Lu, S., Yu, H., Chen, C., Melamed, Z., Guo, L., et al. (2019). Cytoplasmic TDP-43 de-mixing independent of stress granules drives inhibition of nuclear import, loss of nuclear TDP-43, and cell death. Neuron 102, 339–357.e7. doi:10.1016/j.neuron.2019.02.038
Glauninger, H., Wong Hickernell, C. J., Bard, J. A. M., and Drummond, D. A. (2022). Stressful steps: progress and challenges in understanding stress-induced mRNA condensation and accumulation in stress granules. Mol. Cell 82, 2544–2556. doi:10.1016/j.molcel.2022.05.014
Grousl, T., Ivanov, P., Frýdlová, I., Vasicová, P., Janda, F., Vojtová, J., et al. (2009). Robust heat shock induces eIF2alpha-phosphorylation-independent assembly of stress granules containing eIF3 and 40S ribosomal subunits in budding yeast, Saccharomyces cerevisiae. J. Cell Sci. 122, 2078–2088. doi:10.1242/jcs.045104
Grousl, T., Ungelenk, S., Miller, S., Ho, C.-T., Khokhrina, M., Mayer, M. P., et al. (2018). A prion-like domain in Hsp42 drives chaperone-facilitated aggregation of misfolded proteins. J. Cell Biol. 217, 1269–1285. doi:10.1083/jcb.201708116
Gu, J., Liu, Z., Zhang, S., Li, Y., Xia, W., Wang, C., et al. (2020). Hsp40 proteins phase separate to chaperone the assembly and maintenance of membraneless organelles. Proc. Natl. Acad. Sci. U. S. A. 117, 31123–31133. doi:10.1073/pnas.2002437117
Gu, J., Wang, C., Hu, R., Li, Y., Zhang, S., Sun, Y., et al. (2021). Hsp70 chaperones TDP-43 in dynamic, liquid-like phase and prevents it from amyloid aggregation. Cell Res. 31, 1024–1027. doi:10.1038/s41422-021-00526-5
Guillén-Boixet, J., Kopach, A., Holehouse, A. S., Wittmann, S., Jahnel, M., Schlüßler, R., et al. (2020). RNA-induced conformational switching and clustering of G3BP drive stress granule assembly by condensation. Cell 181, 346–361.e17. doi:10.1016/j.cell.2020.03.049
Guo, L., Fare, C. M., and Shorter, J. (2019). Therapeutic dissolution of aberrant phases by nuclear-import receptors. Trends Cell Biol. 29, 308–322. doi:10.1016/j.tcb.2018.12.004
Guo, L., Kim, H. J., Wang, H., Monaghan, J., Freyermuth, F., Sung, J. C., et al. (2018). Nuclear-Import receptors reverse aberrant phase transitions of RNA-binding proteins with prion-like domains. Cell 173, 677–692.e20. e20. doi:10.1016/j.cell.2018.03.002
Gwon, Y., Maxwell, B. A., Kolaitis, R.-M., Zhang, P., Kim, H. J., and Taylor, J. P. (2021). Ubiquitination of G3BP1 mediates stress granule disassembly in a context-specific manner. Science 372, eabf6548. doi:10.1126/science.abf6548
Harrison, A. F., and Shorter, J. (2017). RNA-binding proteins with prion-like domains in health and disease. Biochem. J. 474, 1417–1438. doi:10.1042/bcj20160499
Hartl, F. U., Bracher, A., and Hayer-Hartl, M. (2011). Molecular chaperones in protein folding and proteostasis. Nature 475, 324–332. doi:10.1038/nature10317
Haslbeck, M., Braun, N., Stromer, T., Richter, B., Model, N., Weinkauf, S., et al. (2004). Hsp42 is the general small heat shock protein in the cytosol of Saccharomyces cerevisiae. EMBO J. 23, 638–649. doi:10.1038/sj.emboj.7600080
Haslbeck, M., and Vierling, E. (2015). A first line of stress defense: small heat shock proteins and their function in protein homeostasis. J. Mol. Biol. 427, 1537–1548. doi:10.1016/j.jmb.2015.02.002
Haslbeck, M., Weinkauf, S., and Buchner, J. (2019). Small heat shock proteins: simplicity meets complexity. J. Biol. Chem. 294, 2121–2132. doi:10.1074/jbc.rev118.002809
Ho, C.-T., Grousl, T., Shatz, O., Jawed, A., Ruger-Herreros, C., Semmelink, M., et al. (2019). Cellular sequestrases maintain basal Hsp70 capacity ensuring balanced proteostasis. Nat. Commun. 10, 4851. doi:10.1038/s41467-019-12868-1
Hofweber, M., Hutten, S., Bourgeois, B., Spreitzer, E., Niedner-Boblenz, A., Schifferer, M., et al. (2018). Phase separation of FUS is suppressed by its nuclear import receptor and arginine methylation. Cell 173, 706–719.e13. doi:10.1016/j.cell.2018.03.004
Hua, S., Kłosowska, A., Rodrigues, J. I., Petelski, G., Esquembre, L. A., Lorentzon, E., et al. (2022). Differential contributions of the proteasome, autophagy, and chaperones to the clearance of arsenite-induced protein aggregates in yeast. J. Biol. Chem. 298, 102680. doi:10.1016/j.jbc.2022.102680
Hutten, S., Usluer, S., Bourgeois, B., Simonetti, F., Odeh, H. M., Fare, C. M., et al. (2020). Nuclear import receptors directly bind to arginine-rich dipeptide repeat proteins and suppress their pathological interactions. Cell Rep. 33, 108538. doi:10.1016/j.celrep.2020.108538
Iserman, C., Desroches Altamirano, C., Jegers, C., Friedrich, U., Zarin, T., Fritsch, A. W., et al. (2020). Condensation of Ded1p promotes a translational switch from housekeeping to stress protein production. Cell 181, 818–831.e19. doi:10.1016/j.cell.2020.04.009
Itakura, A. K., Chakravarty, A. K., Jakobson, C. M., and Jarosz, D. F. (2020). Widespread prion-based control of growth and differentiation strategies in Saccharomyces cerevisiae. Mol. Cell 77, 266–278.e6. doi:10.1016/j.molcel.2019.10.027
Jackrel, M. E., DeSantis, M. E., Martinez, B. A., Castellano, L. M., Stewart, R. M., Caldwell, K. A., et al. (2014). Potentiated Hsp104 variants antagonize diverse proteotoxic misfolding events. Cell 156, 170–182. doi:10.1016/j.cell.2013.11.047
Jalihal, A. P., Pitchiaya, S., Xiao, L., Bawa, P., Jiang, X., Bedi, K., et al. (2019). Multivalent proteins rapidly and reversibly phase-separate upon osmotic cell volume change. bioRxiv 748293. doi:10.1101/748293
Jawerth, L., Fischer-Friedrich, E., Saha, S., Wang, J., Franzmann, T., Zhang, X., et al. (2020). Protein condensates as aging Maxwell fluids. Science 370, 1317–1323. doi:10.1126/science.aaw4951
Johnston, C. L., Marzano, N. R., Paudel, B. P., Wright, G., Benesch, J. L. P., van Oijen, A. M., et al. (2021). Single-molecule fluorescence-based approach reveals novel mechanistic insights into human small heat shock protein chaperone function. J. Biol. Chem. 296, 100161. doi:10.1074/jbc.ra120.015419
Jovcevski, B., Kelly, M. A., Rote, A. P., Berg, T., Gastall, H. Y., Benesch, J. L. P., et al. (2015). Phosphomimics destabilize Hsp27 oligomeric assemblies and enhance chaperone activity. Chem. Biol. 22, 186–195. doi:10.1016/j.chembiol.2015.01.001
Jumper, J., Evans, R., Pritzel, A., Green, T., Figurnov, M., Ronneberger, O., et al. (2021). Highly accurate protein structure prediction with AlphaFold. Nature. doi:10.1038/s41586-021-03819-2
Kaganovich, D., Kopito, R., and Frydman, J. (2008). Misfolded proteins partition between two distinct quality control compartments. Nature 454, 1088–1095. doi:10.1038/nature07195
Kampinga, H. H., Andreasson, C., Barducci, A., Cheetham, M. E., Cyr, D., Emanuelsson, C., et al. (2019). Function, evolution, and structure of J-domain proteins. Cell Stress Chaperones 24, 7–15. doi:10.1007/s12192-018-0948-4
Kampinga, H. H., Brunsting, J. F., Stege, G. J., Konings, A. W., and Landry, J. (1994). Cells overexpressing Hsp27 show accelerated recovery from heat-induced nuclear protein aggregation. Biochem. Biophysical Res. Commun. 204, 1170–1177. doi:10.1006/bbrc.1994.2586
Kato, M., Han, T. W., Xie, S., Shi, K., Du, X., Wu, L. C., et al. (2012). Cell-free formation of RNA granules: low complexity sequence domains form dynamic fibers within hydrogels. Cell 149, 753–767. doi:10.1016/j.cell.2012.04.017
Kato, S., Yoshida, M., and Izawa, S. (2019). Btn2 is involved in the clearance of denatured proteins caused by severe ethanol stress in Saccharomyces cerevisiae. FEMS Yeast Res. 19, foz079. doi:10.1093/femsyr/foz079
Kedersha, N., Panas, M. D., Achorn, C. A., Lyons, S., Tisdale, S., Hickman, T., et al. (2016). G3BP-Caprin1-USP10 complexes mediate stress granule condensation and associate with 40S subunits. J. Cell Biol. 212, 845–860. doi:10.1083/jcb.201508028
Khalil, B., Chhangani, D., Wren, M. C., Smith, C. L., Lee, J. H., Li, X., et al. (2022). Nuclear import receptors are recruited by FG-nucleoporins to rescue hallmarks of TDP-43 proteinopathy. Mol. Neurodegener. 17, 80. doi:10.1186/s13024-022-00585-1
Keyport Kik, S., Christopher, D., Glauninger, H., Hickernell, C. W., Bard, J. A. M., Lin, K. M., et al. (2024). An adaptive biomolecular condensation response is conserved across environmentally divergent species. Nat. Commun. 15, 1–17. doi:10.1038/s41467-024-47355-9
Krakowiak, J., Zheng, X., Patel, N., Feder, Z. A., Anandhakumar, J., Valerius, K., et al. (2018). Hsf1 and Hsp70 constitute a two-component feedback loop that regulates the yeast heat shock response. Elife 7, e31668. doi:10.7554/elife.31668
Kroschwald, S., Maharana, S., Mateju, D., Malinovska, L., Nüske, E., Poser, I., et al. (2015). Promiscuous interactions and protein disaggregases determine the material state of stress-inducible RNP granules. Elife 4, e06807. doi:10.7554/elife.06807
Kroschwald, S., Munder, M. C., Maharana, S., Franzmann, T. M., Richter, D., Ruer, M., et al. (2018). Different material states of Pub1 condensates define distinct modes of stress adaptation and recovery. Cell Rep. 23, 3327–3339. doi:10.1016/j.celrep.2018.05.041
Kryndushkin, D. S., Shewmaker, F., and Wickner, R. B. (2008). Curing of the [URE3] prion by Btn2p, a Batten disease-related protein. EMBO J. 27, 2725–2735. doi:10.1038/emboj.2008.198
Kshirsagar, M., and Parker, R. (2004). Identification of Edc3p as an enhancer of mRNA decapping in Saccharomyces cerevisiae. Genetics 166, 729–739. doi:10.1093/genetics/166.2.729
Kumar, A., Mathew, V., and Stirling, P. C. (2022). Nuclear protein quality control in yeast: the latest INQuiries. J. Biol. Chem. 298, 102199. doi:10.1016/j.jbc.2022.102199
Kyoung, M., Russell, S. J., Kohnhorst, C. L., Esemoto, N. N., and An, S. (2015). Dynamic architecture of the purinosome involved in human de novo purine biosynthesis. Biochemistry 54, 870–880. doi:10.1021/bi501480d
Lafontaine, D. L. J., Riback, J. A., Bascetin, R., and Brangwynne, C. P. (2021). The nucleolus as a multiphase liquid condensate. Nat. Rev. Mol. Cell Biol. 22, 165–182. doi:10.1038/s41580-020-0272-6
Lake, D., Corrêa, S. A. L., and Müller, J. (2016). Negative feedback regulation of the ERK1/2 MAPK pathway. Cell. Mol. Life Sci. 73, 4397–4413. doi:10.1007/s00018-016-2297-8
Lee, G. J., Roseman, A. M., Saibil, H. R., and Vierling, E. (1997). A small heat shock protein stably binds heat-denatured model substrates and can maintain a substrate in a folding-competent state. EMBO J. 16, 659–671. doi:10.1093/emboj/16.3.659
Li, P., Banjade, S., Cheng, H. C., Kim, S., Chen, B., Guo, L., et al. (2012). Phase transitions in the assembly of multivalent signalling proteins. Nature 483, 336–340. doi:10.1038/nature10879
Li, Y., Gu, J., Wang, C., Hu, J., Zhang, S., Liu, C., et al. (2022). Hsp70 exhibits a liquid-liquid phase separation ability and chaperones condensed FUS against amyloid aggregation. iScience 25, 104356. doi:10.1016/j.isci.2022.104356
Liu, Z., Zhang, S., Gu, J., Tong, Y., Li, Y., Gui, X., et al. (2020). Hsp27 chaperones FUS phase separation under the modulation of stress-induced phosphorylation. Nat. Struct. Mol. Biol. 27, 363–372. doi:10.1038/s41594-020-0399-3
Lu, S., Hu, J., Arogundade, O. A., Goginashvili, A., Vazquez-Sanchez, S., Diedrich, J. K., et al. (2022). Heat-shock chaperone HSPB1 regulates cytoplasmic TDP-43 phase separation and liquid-to-gel transition. Nat. Cell Biol. 24, 1378–1393. doi:10.1038/s41556-022-00988-8
Lyon, A. S., Peeples, W. B., and Rosen, M. K. (2021). A framework for understanding the functions of biomolecular condensates across scales. Nat. Rev. Mol. Cell Biol. 22, 215–235. doi:10.1038/s41580-020-00303-z
Mack, K. L., Kim, H., Barbieri, E. M., Lin, J., Braganza, S., Jackrel, M. E., et al. (2023). Tuning Hsp104 specificity to selectively detoxify α-synuclein. Mol. Cell 83, 3314–3332.e9. e9. doi:10.1016/j.molcel.2023.07.029
Malinovska, L., Kroschwald, S., Munder, M. C., Richter, D., and Alberti, S. (2012). Molecular chaperones and stress-inducible protein-sorting factors coordinate the spatiotemporal distribution of protein aggregates. Mol. Biol. Cell 23, 3041–3056. doi:10.1091/mbc.e12-03-0194
Martin, E. W., Harmon, T. S., Hopkins, J. B., Chakravarthy, S., Incicco, J. J., Schuck, P., et al. (2021). A multi-step nucleation process determines the kinetics of prion-like domain phase separation. Nat. Commun. 12, 4513. doi:10.1038/s41467-021-24727-z
Masser, A. E., Kang, W., Roy, J., Mohanakrishnan Kaimal, J., Quintana-Cordero, J., Friedländer, M. R., et al. (2019). Cytoplasmic protein misfolding titrates Hsp70 to activate nuclear Hsf1. Elife 8, e47791. doi:10.7554/eLife.47791
Mateju, D., and Chao, J. A. (2022). Stress granules: regulators or by-products? FEBS J. 289, 363–373. doi:10.1111/febs.15821
Mateju, D., Franzmann, T. M., Patel, A., Kopach, A., Boczek, E. E., Maharana, S., et al. (2017). An aberrant phase transition of stress granules triggered by misfolded protein and prevented by chaperone function. EMBO J. 36, 1669–1687. doi:10.15252/embj.201695957
Maxwell, B. A., Gwon, Y., Mishra, A., Peng, J., Nakamura, H., Zhang, K., et al. (2021). Ubiquitination is essential for recovery of cellular activities after heat shock. Science 372, eabc3593. doi:10.1126/science.abc3593
Mazroui, R., Huot, M.-E., Tremblay, S., Filion, C., Labelle, Y., and Khandjian, E. W. (2002). Trapping of messenger RNA by Fragile X Mental Retardation protein into cytoplasmic granules induces translation repression. Hum. Mol. Genet. 11, 3007–3017. doi:10.1093/hmg/11.24.3007
Mediani, L., Antoniani, F., Galli, V., Vinet, J., Carrà, A. D., Bigi, I., et al. (2021). Hsp90-mediated regulation of DYRK3 couples stress granule disassembly and growth via mTORC1 signaling. EMBO Rep. 22, e51740. doi:10.15252/embr.202051740
Miller, S. B., Ho, C.-T., Winkler, J., Khokhrina, M., Neuner, A., Mohamed, M. Y., et al. (2015a). Compartment-specific aggregases direct distinct nuclear and cytoplasmic aggregate deposition. EMBO J., e201489524. doi:10.15252/embj.201489524
Miller, S. B. M., Mogk, A., and Bukau, B. (2015b). Spatially organized aggregation of misfolded proteins as cellular stress defense strategy. J. Mol. Biol. 427, 1564–1574. doi:10.1016/j.jmb.2015.02.006
Milles, S., Huy Bui, K., Koehler, C., Eltsov, M., Beck, M., and Lemke, E. A. (2013). Facilitated aggregation of FG nucleoporins under molecular crowding conditions. EMBO Rep. 14, 178–183. doi:10.1038/embor.2012.204
Mirdita, M., Schütze, K., Moriwaki, Y., Heo, L., Ovchinnikov, S., and Steinegger, M. (2022). ColabFold: Making Protein folding accessible to all. Nat. Methods. doi:10.1038/s41592-022-01488-1
Mittag, T., and Pappu, R. V. (2022). A conceptual framework for understanding phase separation and addressing open questions and challenges. Mol. Cell 82, 2201–2214. doi:10.1016/j.molcel.2022.05.018
Mogk, A., Ruger-Herreros, C., and Bukau, B. (2019). Cellular functions and mechanisms of action of small heat shock proteins. Annu. Rev. Microbiol. 73, 89–110. doi:10.1146/annurev-micro-020518-115515
Mollet, S., Cougot, N., Wilczynska, A., Dautry, F., Kress, M., Bertrand, E., et al. (2008). Translationally repressed mRNA transiently cycles through stress granules during stress. Mol. Biol. Cell 19, 4469–4479. doi:10.1091/mbc.e08-05-0499
Molliex, A., Temirov, J., Lee, J., Coughlin, M., Kanagaraj, A. P., Kim, H. J., et al. (2015). Phase separation by low complexity domains promotes stress granule assembly and drives pathological fibrillization. Cell 163, 123–133. doi:10.1016/j.cell.2015.09.015
Moreno, D. F., Parisi, E., Yahya, G., Vaggi, F., Csikász-Nagy, A., and Aldea, M. (2019). Competition in the chaperone-client network subordinates cell-cycle entry to growth and stress. Life Sci. Alliance 2, e201800277. doi:10.26508/lsa.201800277
Morimoto, R. I. (2008). Proteotoxic stress and inducible chaperone networks in neurodegenerative disease and aging. Genes Dev. 22, 1427–1438. doi:10.1101/gad.1657108
Mühlhofer, M., Peters, C., Kriehuber, T., Kreuzeder, M., Kazman, P., Rodina, N., et al. (2021). Phosphorylation activates the yeast small heat shock protein Hsp26 by weakening domain contacts in the oligomer ensemble. Nat. Commun. 12, 6697. doi:10.1038/s41467-021-27036-7
Munder, M. C., Midtvedt, D., Franzmann, T., Nüske, E., Otto, O., Herbig, M., et al. (2016). A pH-driven transition of the cytoplasm from a fluid-to a solid-like state promotes entry into dormancy. eLife 5, e09347. doi:10.7554/elife.09347
Murakami, T., Qamar, S., Lin, J. Q., Schierle, G. S. K., Rees, E., Miyashita, A., et al. (2015). ALS/FTD mutation-induced phase transition of FUS liquid droplets and reversible hydrogels into irreversible hydrogels impairs RNP granule function. Neuron 88, 678–690. doi:10.1016/j.neuron.2015.10.030
Murray, D. T., Kato, M., Lin, Y., Thurber, K. R., Hung, I., McKnight, S. L., et al. (2017). Structure of FUS protein fibrils and its relevance to self-assembly and phase separation of low-complexity domains. Cell 171, 615–627.e16. doi:10.1016/j.cell.2017.08.048
Niaki, A. G., Sarkar, J., Cai, X., Rhine, K., Vidaurre, V., Guy, B., et al. (2020). Loss of dynamic RNA interaction and aberrant phase separation induced by two distinct types of ALS/FTD-Linked FUS mutations. Mol. Cell 77, 82–94.e4. doi:10.1016/j.molcel.2019.09.022
Nillegoda, N. B., Kirstein, J., Szlachcic, A., Berynskyy, M., Stank, A., Stengel, F., et al. (2015). Crucial HSP70 co-chaperone complex unlocks metazoan protein disaggregation. Nature 524, 247–251. doi:10.1038/nature14884
Nillegoda, N. B., Stank, A., Malinverni, D., Alberts, N., Szlachcic, A., Barducci, A., et al. (2017). Evolution of an intricate J-protein network driving protein disaggregation in eukaryotes. Elife 6, e24560. doi:10.7554/eLife.24560
Nott, T. J., Petsalaki, E., Farber, P., Jervis, D., Fussner, E., Plochowietz, A., et al. (2015). Phase transition of a disordered nuage protein generates environmentally responsive membraneless organelles. Mol. Cell 57, 936–947. doi:10.1016/j.molcel.2015.01.013
Odeh, H. M., Fare, C. M., and Shorter, J. (2022). Nuclear-Import receptors counter deleterious phase transitions in neurodegenerative disease. J. Mol. Biol. 434, 167220. doi:10.1016/j.jmb.2021.167220
Patel, A., Lee, H. O., Jawerth, L., Maharana, S., Jahnel, M., Hein, M. Y., et al. (2015). A liquid-to-solid phase transition of the ALS protein FUS accelerated by disease mutation. Cell 162, 1066–1077. doi:10.1016/j.cell.2015.07.047
Pedley, A. M., Boylan, J. P., Chan, C. Y., Kennedy, E. L., Kyoung, M., and Benkovic, S. J. (2022). Purine biosynthetic enzymes assemble into liquid-like condensates dependent on the activity of chaperone protein HSP90. J. Biol. Chem. 298, 101845. doi:10.1016/j.jbc.2022.101845
Pedley, A. M., Karras, G. I., Zhang, X., Lindquist, S., and Benkovic, S. J. (2018). Role of HSP90 in the regulation of de novo purine biosynthesis. Biochemistry 57, 3217–3221. doi:10.1021/acs.biochem.8b00140
Peschek, J., Braun, N., Rohrberg, J., Back, K. C., Kriehuber, T., Kastenmüller, A., et al. (2013). Regulated structural transitions unleash the chaperone activity of αB-crystallin. Proc. Natl. Acad. Sci. U. S. A. 110, E3780–E3789. doi:10.1073/pnas.1308898110
Pincus, D. (2020). “Regulation of Hsf1 and the heat shock response,” in HSF1 and molecular chaperones in biology and cancer. Editors M. L. Mendillo, D. Pincus, and R. Scherz-Shouval (Cham: Springer International Publishing), 41–50.
Plante, S., Moon, K.-M., Lemieux, P., Foster, L. J., and Landry, C. R. (2023). Breaking spore dormancy in budding yeast transforms the cytoplasm and the solubility of the proteome. PLoS Biol. 21, e3002042. doi:10.1371/journal.pbio.3002042
Portz, B., Lee, B. L., and Shorter, J. (2021). FUS and TDP-43 phases in health and disease. Trends Biochem. Sci. 46, 550–563. doi:10.1016/j.tibs.2020.12.005
Qamar, S., Wang, G., Randle, S. J., Ruggeri, F. S., Varela, J. A., Lin, J. Q., et al. (2018). FUS phase separation is modulated by a molecular chaperone and methylation of arginine cation-π interactions. Cell 173, 720–734.e15. doi:10.1016/j.cell.2018.03.056
Reineke, L. C., Kedersha, N., Langereis, M. A., van Kuppeveld, F. J. M., and Lloyd, R. E. (2015). Stress granules regulate double-stranded RNA-dependent protein kinase activation through a complex containing G3BP1 and Caprin1. MBio 6, e02486. doi:10.1128/mbio.02486-14
Reinle, K., Mogk, A., and Bukau, B. (2022). The diverse functions of small heat shock proteins in the proteostasis network. J. Mol. Biol. 434, 167157. doi:10.1016/j.jmb.2021.167157
Rhine, K., Dasovich, M., Yoniles, J., Badiee, M., Skanchy, S., Ganser, L. R., et al. (2022). Poly(ADP-ribose) drives condensation of FUS via a transient interaction. Mol. Cell 82, 969–985.e11. doi:10.1016/j.molcel.2022.01.018
Riback, J. A., Katanski, C. D., Kear-Scott, J. L., Pilipenko, E. V., Rojek, A. E., Sosnick, T. R., et al. (2017). Stress-triggered phase separation is an adaptive, evolutionarily tuned response. Cell 168, 1028–1040.e19. e19. doi:10.1016/j.cell.2017.02.027
Ripin, N., and Parker, R. (2022). Are stress granules the RNA analogs of misfolded protein aggregates? RNA 28, 67–75. doi:10.1261/rna.079000.121
Rowley, A., Johnston, G. C., Butler, B., Werner-Washburne, M., and Singer, R. A. (1993). Heat shock-mediated cell cycle blockage and G1 cyclin expression in the yeast Saccharomyces cerevisiae. Mol. Cell. Biol. 13, 1034–1041. doi:10.1128/mcb.13.2.1034
Saad, S., and Jarosz, D. F. (2021). Protein self-assembly: a new frontier in cell signaling. Curr. Opin. Cell Biol. 69, 62–69. doi:10.1016/j.ceb.2020.12.013
Saarikangas, J., and Barral, Y. (2015). Protein aggregates are associated with replicative aging without compromising protein quality control. Elife 4, e06197. doi:10.7554/eLife.06197
Saarikangas, J., Caudron, F., Prasad, R., Moreno, D. F., Bolognesi, A., Aldea, M., et al. (2017). Compartmentalization of ER-bound chaperone confines protein deposit formation to the aging yeast cell. Curr. Biol. 27, 773–783. doi:10.1016/j.cub.2017.01.069
Sabari, B. R., Dall’Agnese, A., and Young, R. A. (2020). Biomolecular condensates in the nucleus. Trends Biochem. Sci. 45, 961–977. doi:10.1016/j.tibs.2020.06.007
Seyffer, F., Kummer, E., Oguchi, Y., Winkler, J., Kumar, M., Zahn, R., et al. (2012). Hsp70 proteins bind Hsp100 regulatory M domains to activate AAA+ disaggregase at aggregate surfaces. Nat. Struct. Mol. Biol. 19, 1347–1355. doi:10.1038/nsmb.2442
Sheth, U., and Parker, R. (2003). Decapping and decay of messenger RNA occur in cytoplasmic processing bodies. Science 300, 805–808. doi:10.1126/science.1082320
Shi, J., Koteiche, H. A., McDonald, E. T., Fox, T. L., Stewart, P. L., and McHaourab, H. S. (2013). Cryoelectron microscopy analysis of small heat shock protein 16.5 (Hsp16.5) complexes with T4 lysozyme reveals the structural basis of multimode binding. J. Biol. Chem. 288, 4819–4830. doi:10.1074/jbc.m112.388132
Shimobayashi, S. F., Ronceray, P., Sanders, D. W., Haataja, M. P., and Brangwynne, C. P. (2021). Nucleation landscape of biomolecular condensates. Nature 599, 503–506. doi:10.1038/s41586-021-03905-5
Shorter, J., and Lindquist, S. (2004). Hsp104 catalyzes formation and elimination of self-replicating Sup35 prion conformers. Science 304, 1793–1797. doi:10.1126/science.1098007
Shrivastava, A., Sandhof, C. A., Reinle, K., Jawed, A., Ruger-Herreros, C., Schwarz, D., et al. (2022). The cytoprotective sequestration activity of small heat shock proteins is evolutionarily conserved. J. Cell Biol. 221, e202202149. doi:10.1083/jcb.202202149
Simpson-Lavy, K., Xu, T., Johnston, M., and Kupiec, M. (2017). The Std1 activator of the Snf1/AMPK kinase controls glucose response in yeast by a regulated protein aggregation. Mol. Cell 68, 1120–1133.e3. doi:10.1016/j.molcel.2017.11.016
Son, M., and Wickner, R. B. (2022). Antiprion systems in yeast cooperate to cure or prevent the generation of nearly all [PSI<sup>+</sup>] and [URE3] prions. Proc. Natl. Acad. Sci. U. S. A. 119, e2205500119. doi:10.1073/pnas.2205500119
Sontag, E. M., Samant, R. S., and Frydman, J. (2017). Mechanisms and functions of spatial protein quality control. Annu. Rev. Biochem. 86, 97–122. doi:10.1146/annurev-biochem-060815-014616
Specht, S., Miller, S. B. M., Mogk, A., and Bukau, B. (2011). Hsp42 is required for sequestration of protein aggregates into deposition sites in Saccharomyces cerevisiae. J. Cell Biol. 195, 617–629. doi:10.1083/jcb.201106037
Sui, X., Cox, D., Nie, S., Reid, G. E., and Hatters, D. M. (2022). A census of hsp70-mediated proteome solubility changes upon recovery from heat stress. J. Proteome Res. 21, 1251–1261. doi:10.1021/acs.jproteome.1c00920
Thomas, L., Taleb Ismail, B., Askjaer, P., and Seydoux, G. (2023). Nucleoporin foci are stress-sensitive condensates dispensable for C. elegans nuclear pore assembly. EMBO J. 42, e112987. doi:10.15252/embj.2022112987
To, P., Bhagwat, A. M., Tarbox, H. E., Ecer, A., Wendorff, H., Jamieson, Z., et al. (2023). Intrinsically disordered regions promote protein refoldability and facilitate retrieval from biomolecular condensates. bioRxiv 06 (25), 546465. doi:10.1101/2023.06.25.546465
Triandafillou, C. G., Katanski, C. D., Dinner, A. R., and Drummond, D. A. (2020). Transient intracellular acidification regulates the core transcriptional heat shock response. Elife 9, e54880. doi:10.7554/eLife.54880
Udan-Johns, M., Bengoechea, R., Bell, S., Shao, J., Diamond, M. I., True, H. L., et al. (2014). Prion-like nuclear aggregation of TDP-43 during heat shock is regulated by HSP40/70 chaperones. Hum. Mol. Genet. 23, 157–170. doi:10.1093/hmg/ddt408
Ungelenk, S., Moayed, F., Ho, C.-T., Grousl, T., Scharf, A., Mashaghi, A., et al. (2016). Small heat shock proteins sequester misfolding proteins in near-native conformation for cellular protection and efficient refolding. Nat. Commun. 7, 13673. doi:10.1038/ncomms13673
Vabulas, R. M., Raychaudhuri, S., Hayer-Hartl, M., and Hartl, F. U. (2010). Protein folding in the cytoplasm and the heat shock response. Cold Spring Harb. Perspect. Biol. 2, a004390. doi:10.1101/cshperspect.a004390
Varadi, M., Anyango, S., Deshpande, M., Nair, S., Natassia, C., Yordanova, G., et al. (2022). AlphaFold Protein Structure Database: Massively Expanding the Structural Coverage of Protein-Sequence Space with High-Accuracy Models. Nucleic Acids Res. 50 (D1): D439–D444.
Wallace, E. W. J., Kear-Scott, J. L., Pilipenko, E. V., Schwartz, M. H., Laskowski, P. R., Rojek, A. E., et al. (2015). Reversible, specific, active aggregates of endogenous proteins assemble upon heat stress. Cell 162, 1286–1298. doi:10.1016/j.cell.2015.08.041
Walters, R. W., Muhlrad, D., Garcia, J., and Parker, R. (2015). Differential effects of Ydj1 and Sis1 on Hsp70-mediated clearance of stress granules in Saccharomyces cerevisiae. RNA 21, 1660–1671. doi:10.1261/rna.053116.115
Wang, B., Maxwell, B. A., Joo, J. H., Gwon, Y., Messing, J., Mishra, A., et al. (2019). ULK1 and ULK2 regulate stress granule disassembly through phosphorylation and activation of VCP/p97. Mol. Cell 74, 742–757.e8. doi:10.1016/j.molcel.2019.03.027
Wang, Z., Lou, J., and Zhang, H. (2022). Essence determines phenomenon: assaying the material properties of biological condensates. J. Biol. Chem. 298, 101782. doi:10.1016/j.jbc.2022.101782
Watson, J. L., Seinkmane, E., Styles, C. T., Mihut, A., Krüger, L. K., McNally, K. E., et al. (2024). Author Correction: macromolecular condensation buffers intracellular water potential. Nature 1–11. doi:10.1038/s41586-024-07346-8
Weis, K. (2021). Dead or alive: DEAD-box ATPases as regulators of ribonucleoprotein complex condensation. Biol. Chem. 402, 653–661. doi:10.1515/hsz-2020-0381
Wen, Y., and Ma, J. (2022). Phase separation drives the formation of biomolecular condensates in the immune system. Front. Immunol. 13, 986589. doi:10.3389/fimmu.2022.986589
Wentink, A., Nussbaum-Krammer, C., and Bukau, B. (2019). Modulation of amyloid states by molecular chaperones. Cold Spring Harb. Perspect. Biol. 11, a033969. doi:10.1101/cshperspect.a033969
Wentink, A. S., Nillegoda, N. B., Feufel, J., Ubartaitė, G., Schneider, C. P., De Los Rios, P., et al. (2020). Molecular dissection of amyloid disaggregation by human HSP70. Nature 587, 483–488. doi:10.1038/s41586-020-2904-6
White, J. P., and Lloyd, R. E. (2012). Regulation of stress granules in virus systems. Trends Microbiol. 20, 175–183. doi:10.1016/j.tim.2012.02.001
Wippich, F., Bodenmiller, B., Trajkovska, M. G., Wanka, S., Aebersold, R., and Pelkmans, L. (2013). Dual specificity kinase DYRK3 couples stress granule condensation/dissolution to mTORC1 signaling. Cell 152, 791–805. doi:10.1016/j.cell.2013.01.033
Woodruff, J. B., Hyman, A. A., and Boke, E. (2018). Organization and function of non-dynamic biomolecular condensates. Trends Biochem. Sci. 43, 81–94. doi:10.1016/j.tibs.2017.11.005
Xu, G., Pattamatta, A., Hildago, R., Pace, M. C., Brown, H., and Borchelt, D. R. (2016). Vulnerability of newly synthesized proteins to proteostasis stress. J. Cell Sci. 129, 1892–1901. doi:10.1242/jcs.176479
Yamasaki, A., Alam, J. M., Noshiro, D., Hirata, E., Fujioka, Y., Suzuki, K., et al. (2020). Liquidity is a critical determinant for selective autophagy of protein condensates. Mol. Cell 77, 1163–1175.e9. e9. doi:10.1016/j.molcel.2019.12.026
Yang, P., Mathieu, C., Kolaitis, R.-M., Zhang, P., Messing, J., Yurtsever, U., et al. (2020). G3BP1 is a tunable switch that triggers phase separation to assemble stress granules. Cell 181, 325–345.e28. doi:10.1016/j.cell.2020.03.046
Yoo, H., Bard, J. A. M., Pilipenko, E. V., and Drummond, D. A. (2022). Chaperones directly and efficiently disperse stress-triggered biomolecular condensates. Mol. Cell 82, 741–755.e11. e11. doi:10.1016/j.molcel.2022.01.005
Yoo, H., Triandafillou, C., and Drummond, D. A. (2019). Cellular sensing by phase separation: using the process, not just the products. J. Biol. Chem. 294, 7151–7159. doi:10.1074/jbc.tm118.001191
Yoshizawa, T., Ali, R., Jiou, J., Fung, H. Y. J., Burke, K. A., Kim, S. J., et al. (2018). Nuclear import receptor inhibits phase separation of FUS through binding to multiple sites. Cell 173, 693–705.e22. doi:10.1016/j.cell.2018.03.003
Yu, H., Lu, S., Gasior, K., Singh, D., Vazquez-Sanchez, S., Tapia, O., et al. (2021). HSP70 chaperones RNA-free TDP-43 into anisotropic intranuclear liquid spherical shells. Science 371, eabb4309. doi:10.1126/science.abb4309
Zhang, J. Z., Greenwood, N., Hernandez, J., Cuperus, J. T., Huang, B., Ryder, B. D., et al. (2023). De novo designed Hsp70 activator dissolves intracellular condensates. bioRxiv 18. doi:10.1101/2023.09.18.558356
Zhang, J. Z., Lu, T.-W., Stolerman, L. M., Tenner, B., Yang, J. R., Zhang, J.-F., et al. (2020). Phase separation of a PKA regulatory subunit controls cAMP compartmentation and oncogenic signaling. Cell 182, 1531–1544.e15. doi:10.1016/j.cell.2020.07.043
Zheng, X., Krakowiak, J., Patel, N., Beyzavi, A., Ezike, J., Khalil, A. S., et al. (2016). Dynamic control of Hsf1 during heat shock by a chaperone switch and phosphorylation. Elife 5, e18638. doi:10.7554/eLife.18638
Zhou, C., Slaughter, B. D., Unruh, J. R., Guo, F., Yu, Z., Mickey, K., et al. (2014). Organelle-based aggregation and retention of damaged proteins in asymmetrically dividing cells. Cell 159, 530–542. doi:10.1016/j.cell.2014.09.026
Zhu, K., Celwyn, I. J., Guan, D., Xiao, Y., Wang, X., Hu, W., et al. (2023). An intrinsically disordered region controlling condensation of a circadian clock component and rhythmic transcription in the liver. Mol. Cell 83, 3457–3469.e7. e7. doi:10.1016/j.molcel.2023.09.010
Keywords: cell stress, adaptation, chaperones, biomolecular condensate, stress granules, proteostasis
Citation: Bard JAM and Drummond DA (2024) Chaperone regulation of biomolecular condensates. Front. Biophys. 2:1342506. doi: 10.3389/frbis.2024.1342506
Received: 21 November 2023; Accepted: 05 April 2024;
Published: 07 May 2024.
Edited by:
Kiersten Ruff, Washington University in St. Louis, United StatesReviewed by:
Kyota Yasuda, Hiroshima University, JapanLin Guo, Thomas Jefferson University, United States
Copyright © 2024 Bard and Drummond. This is an open-access article distributed under the terms of the Creative Commons Attribution License (CC BY). The use, distribution or reproduction in other forums is permitted, provided the original author(s) and the copyright owner(s) are credited and that the original publication in this journal is cited, in accordance with accepted academic practice. No use, distribution or reproduction is permitted which does not comply with these terms.
*Correspondence: D. Allan Drummond, ZGFkcnVtbW9uZEB1Y2hpY2Fnby5lZHU=