- 1Louvain Institute of Biomolecular Sicence and Technology, Université Catholique de Louvain, Ottignies-Louvain-la-Neuve, Belgium
- 2Walloon Excellence in Life Sciences and Biotechnology (WELBIO), Wavre, Belgium
Introduction
The field of biophysics has its roots in the late 19th and early 20th centuries. The term biophysics was first introduced by Karl Pearson in 1892 justified by the need to describe our perception of living organisms through a branch of science dealing with the application of the laws of inorganic or physical phenomena to complex organic forms (Pearson, 1892). This field strives to show how fundamental underpinnings of biology are, in fact, special cases of general physics. While this field did not make much progress at the time of its foundation, it was immediately predicted to have a bright future. However, the formal establishment of biophysics as a distinct scientific discipline is often credited to Max Delbrück and others, who established the first biophysics research groups in the 1940s. Since then, the field of biophysics has developed rapidly, growing exponentially over the past three decades (Figure 1), with the field now encompassing a wide range of disciplines and techniques, generating important contributions to our understanding of biological systems.
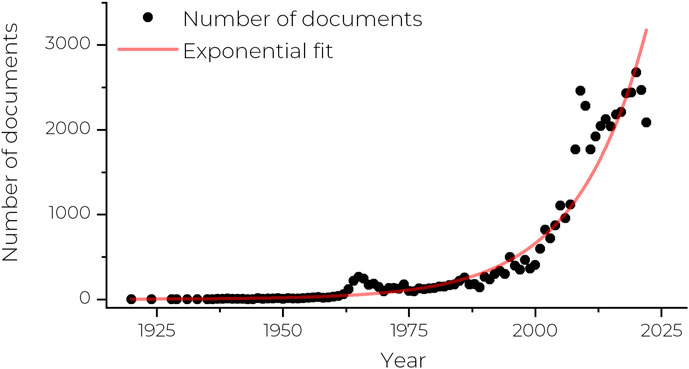
FIGURE 1. Graph showing the number of research articles mentioning the term biophysics in the abstract or keywords between 1920 and 2022. Data extracted from Scopus. Trend fitted with an exponential fit (red line).
But what is exactly biophysics? Where is the boundary between physics and biology? Is biophysics really physics or just the application of methods from physics to the problems of biology? Nowadays, biophysics is perceived as a multidisciplinary field that combines physics, biology, chemistry, and mathematics to study the physical and chemical processes that occur in living organisms. Biophysics undoubtedly emerges from a desire to reconcile different visions of the same phenomenon. Faced with the same observation, physicists and biologists will approach the question in very different ways, because their understanding of the phenomena is based on different definitions. By basing themselves on the paradigms resulting from historical discoveries in biology, biologists lose contact with the more physical vision which is based on deep theoretical knowledge, namely, that there should be a physics of life and not only the physics of this particular system. While in biology the principle of a phenomenon will be elucidated by experimental discoveries, in physics underlying theory and experimentation are more on an equal footing. A set of interconnected theories and principles will define what is possible and will be tested against a series of quantitative experiments. Biophysics aims to reconcile the physicist’s desire to unify theoretical principles with the obvious diversity of the mechanisms of life as perceived by biologists. This field covers many aspects of the understanding of biological phenomena at all levels, including, among others, the structure and dynamics of individual molecules, the assembly and mechanics of (macro) molecules, the study of physiological processes such as mechanotransduction or signaling at the level of cells and tissues, the understanding of interactions with the direct environment from the individual cell to the whole organism, etc. In recent years, advances in biophysics, which are often intrinsically linked to technological developments, have not only led to a better understanding of biological systems but have also allowed researchers to develop new diagnostic techniques and make advances in the treatment of certain diseases.
The solving of molecular structures, the characterization of molecular properties, and the understanding of cellular behavior, among others, represent tremendous challenges for biophysicists. To get access to those properties, a plethora of (bio-) physical techniques have been developed over the last decades with the general aim of pushing further the limits of characterization methods whether in terms of resolution, dynamics, properties, or analysis conditions.
For instance, biophysical approaches have helped to elucidate the structures of complex biological molecules such as proteins, nucleic acids, and lipids. Techniques such as X-ray crystallography, NMR spectroscopy, and cryo-electron microscopy have allowed researchers to determine the three-dimensional structures of these molecules, which has in turn led to insights into their function (Rosenbaum et al, 2009).
Biophysics has also enabled a better-detailed understanding of the physical and chemical mechanisms underlying biological processes such as enzyme catalysis (Agarwal, 2019), protein folding (Fisher et al, 1999), and molecular recognition (Moy et al, 1994). For instance, studies of the mechanical properties of proteins and DNA have shed light on their roles in cellular processes such as DNA replication and protein synthesis (Bustamante et al, 2000a; Bryant et al, 2003). These advances have been made possible by the development of new methods that allow the manipulation of single molecules, making it possible to directly measure the forces that generate biochemical reactions and even to exert external forces to alter the course of these reactions (Bustamante et al, 2000b).
Biophysicists also contributed to a better understanding of the properties and functions of biological membranes and their molecular machinery, which are essential for cell survival. More than two decades ago, a new aspect of cell membrane organization was introduced, based on the dynamic clustering of sphingolipids and cholesterol to form rafts that move within the fluid bilayer (Simons and Ikonen, 1997). This concept is based on the use of multiple biophysical techniques that independently demonstrate the association of sphingolipids and cholesterol, including freeze-etch immune-electron microscopy, fluorescence microscopy, differential scanning calorimetry, spin-label electron resonance spectroscopy, or nuclear magnetic resonance spectroscopy. Besides rafts, direct lines of evidence for larger and more stable domains, enriched or not in cholesterol and sphingolipids, were reported in artificial membranes, fixed cells and more recently in living cells (Baumgart et al, 2007; Frisz et al, 2013; Dumitru et al, 2018). Lipid asymmetry refers to the differential distribution of lipids between the inner and outer leaflets of the membrane, and results in different biophysical properties among leaflets. The maintenance of this lipid asymmetry between the leaflets, assured by specific lipid transporters and flippases, has a high energy cost but is necessary for certain cellular functions, such as cell protection or signal transduction (Lorent et al, 2020). The lateral organization of lipids into rafts or microdomains posseses a significant impact on the functional states of membrane proteins (Laude and Prior, 2004). For example, the asymmetric distribution of phosphatidylserine on the inner leaflet of the plasma membrane is recognized by certain proteins involved in the clearance of apoptotic cells and some lipids, such as PIP2, are known to regulate the conformational dynamics of some G protein-coupled receptors (GPCRs). Techniques such as fluorescence microscopy/spectroscopy and electron microscopy have been used to study membrane structure and dynamics, the structure and dynamics of membrane proteins, as well as the transport of ions and molecules across membranes. Beside in vitro and in vivo experiments, in silico approaches appears a highly complementary approach, providing a detailed view of the molecular interactions that cannot be directly observed through experimental methods. For example, simulations are of great help to study how a protein interacts with a drug molecule (Kitchen et al, 2004) or how water molecules move around a protein (Papoian et al, 2004). In addition, simulations could help to understand the effects of mutations and predict how these variations may affect protein function (McCoy et al, 2021).
By applying the principles of physics to biological systems, biophysics has provided tools and techniques to detect and measure biological molecules, cells, and tissues with high sensitivity and specificity. The development of various imaging techniques that allow visualization of biological structures and processes. For example, X-ray, magnetic resonance imaging, positron emission tomography, and ultrasound imaging are all based on physical principles of waves and their interactions with biological tissues. These techniques are widely used in clinical diagnosis and monitoring of diseases. Biophysics has also contributed to the development of biosensors that can detect biological molecules such as proteins, DNA, and RNA with high sensitivity and specificity. Biosensors are based on physical phenomena such as surface plasmon resonance, fluorescence, and electrochemistry. They are used for early diagnosis and monitoring of diseases, as well as in research applications.
The future of biophysics
Biological phenomena span a broad range of temporal and spatial scales, encompassing events that range from femtosecond molecular motions to processes that occur over minutes, hours, and even days, such as protein folding, cell cycle regulation and biodistribution. At the same time, biological systems of interest can range from small molecules to multi-protein complexes, to cells, tissues, and entire populations. Consequently, understanding the emergence of collective phenomena from the interaction and conformational changes of molecular species at the smallest scales poses a significant challenge. To meet this, one of the big challenges facing the field of biophysics in the future is not only to integrate the various technologies and approaches used to study biological systems, but also to develop new tools and techniques to study biological systems at different levels of organization, from the molecular to the organismal level. This will require the development of new cross-disciplinary collaborations and a better understanding of the underlying principles of biological systems. To meet this challenge, they will also need to use their expertise in modeling complex systems based on mathematical and computational tools. For example, researchers will need to develop new approaches for integrating the information obtained from molecular imaging, computational simulations, and experimental measurements, in order to gain a comprehensive understanding of biological systems.
Another challenge facing the field of biophysics in the future is the application of knowledge gained from biophysics research in order to improve human health. This will require the development of new therapeutic strategies based on understanding the physical and chemical processes that underlie the onset of disease and determining how these processes can be interfered with to restore the healthy conditions.
In conclusion, biophysics is a rapidly evolving field that plays a critical role in our understanding of biological systems. The development of new technologies and approaches, as well as the integration of different disciplines, will be essential for addressing the big challenges facing biophysics in the future. Through continued research and collaboration, biophysicists will continue to make important contributions to our understanding of living organisms and the development of new treatments for diseases.
Summary
In summary, the area of Biophysics embraces the study of the physical principles underlying all processes of living systems, and is further linked to other disciplines such as mathematics and chemistry. The journal Frontiers in Biophysics will publish high quality original papers as well as review articles that use physics-based approaches or methods to better understand biological systems and their functions. This journal is part of the Frontiers publishing structure that offers biophysicists a new opportunity to publish their work in an open access journal using a transparent peer review process.
Author contributions
The author confirms being the sole contributor of this work and has approved it for publication.
Conflict of interest
The author DA declared that they were an editorial board member of Frontiers, at the time of submission. This had no impact on the peer review process and the final decision.
Publisher’s note
All claims expressed in this article are solely those of the authors and do not necessarily represent those of their affiliated organizations, or those of the publisher, the editors and the reviewers. Any product that may be evaluated in this article, or claim that may be made by its manufacturer, is not guaranteed or endorsed by the publisher.
References
Agarwal, P. K. (2019). A biophysical perspective on enzyme catalysis. Biochemistry 58 (6), 438–449. doi:10.1021/acs.biochem.8b01004
Baumgart, T., Hammond, A. T., Sengupta, P., Hess, S. T., Holowka, D. A., Baird, B. A., et al. (2007). Large-scale fluid/fluid phase separation of proteins and lipids in giant plasma membrane vesicles. Proc. Natl. Acad. Sci. U. S. A. 104 (9), 3165–3170. doi:10.1073/pnas.0611357104
Bryant, Z., Stone, M. D., Gore, J., Smith, S. B., Cozzarelli, N. R., and Bustamante, C. (2003). Structural transitions and elasticity from torque measurements on DNA. Nature 424 (6946), 338–341. doi:10.1038/nature01810
Bustamante, C., et al. (2000). Single-molecule studies of DNA mechanics. Curr. Opin. Struct. Biol. 10 (3), 279–285. doi:10.1016/s0959-440x(00)00085-3
Dumitru, A., Poncin, M., Conrard, L., Dufrene, Y., Tyteca, D., and Alsteens, D. (2018). Nanoscale membrane architecture of healthy and pathological red blood cells. Nanoscale Horiz. 3. doi:10.1039/C7NH00187H
Fisher, T. E., Oberhauser, A. F., Carrion-Vazquez, M., Marszalek, P. E., and Fernandez, J. M. (1999). The study of protein mechanics with the atomic force microscope. Trends biochem. Sci. 24 (10), 379–384.
Frisz, J. F., Lou, K., Klitzing, H. A., Hanafin, W. P., Lizunov, V., Wilson, R. L., et al. (2013). Direct chemical evidence for sphingolipid domains in the plasma membranes of fibroblasts. Proc. Natl. Acad. Sci. U. S. A. 110 (8), E613–E622.
Kitchen, D. B., Decornez, H., Furr, J. R., and Bajorath, J. (2004). Docking and scoring in virtual screening for drug discovery: Methods and applications. Nat. Rev. Drug Discov. 3 (11), 935–949. doi:10.1038/nrd1549
Laude, A. J., and Prior, I. A. (2004). Plasma membrane microdomains: Organization, function and trafficking (Review). Mol. Membr. Biol. 21 (3), 193–205.
Lorent, J. H., Levental, K. R., Ganesan, L., Rivera-Longsworth, G., Sezgin, E., Doktorova, M., et al. (2020). Plasma membranes are asymmetric in lipid unsaturation, packing and protein shape. Nat. Chem. Biol. 16 (6), 644–652.
McCoy, M. D., Hamre, J., Klimov, D. K., and Jafri, M. S. (2021). Predicting genetic variation severity using machine learning to interpret molecular simulations. Biophys. J. 120 (2), 189–204.
Moy, V. T., Florin, E. L., and Gaub, H. E. (1994). Intermolecular forces and energies between ligands and receptors. Science 266 (5183), 257–259. doi:10.1126/science.7939660
Papoian, G. A., Ulander, J., Eastwood, M. P., Luthey-Schulten, Z., and Wolynes, P. G. (2004). Water in protein structure prediction. Proc. Natl. Acad. Sci. U. S. A. 101 (10), 3352–3357.
Rosenbaum, D. M., Rasmussen, S. G., and Kobilka, B. K. (2009). The structure and function of G-protein-coupled receptors. Nature 459 (7245), 356–363. doi:10.1038/nature08144
Keywords: biophysics, X-ray crystallography, NMR spectroscopy, cryo-electron microscopy (cryo- EM), biophysical techniques
Citation: Alsteens D (2023) Grand challenges in biophysics. Front. Biophys. 1:1215594. doi: 10.3389/frbis.2023.1215594
Received: 02 May 2023; Accepted: 18 May 2023;
Published: 26 May 2023.
Edited and reviewed by:
Jody Rosenblatt, King’s College London, United KingdomCopyright © 2023 Alsteens. This is an open-access article distributed under the terms of the Creative Commons Attribution License (CC BY). The use, distribution or reproduction in other forums is permitted, provided the original author(s) and the copyright owner(s) are credited and that the original publication in this journal is cited, in accordance with accepted academic practice. No use, distribution or reproduction is permitted which does not comply with these terms.
*Correspondence: David Alsteens, ZGF2aWQuYWxzdGVlbnNAdWNsb3V2YWluLmJl