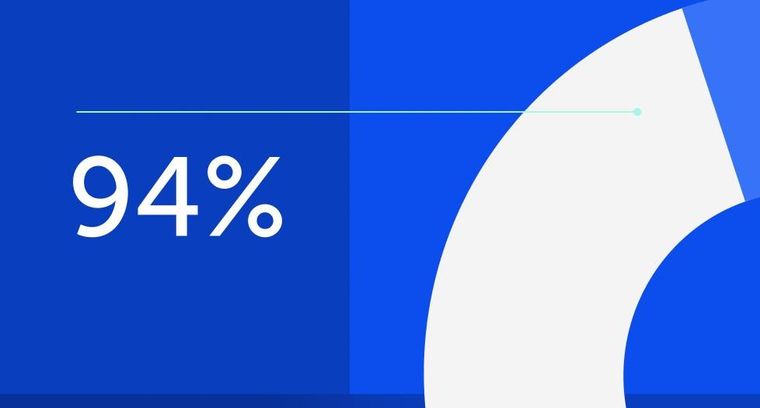
94% of researchers rate our articles as excellent or good
Learn more about the work of our research integrity team to safeguard the quality of each article we publish.
Find out more
REVIEW article
Front. Biomater. Sci., 27 March 2024
Sec. Biomaterials Science for Regenerative Therapies
Volume 3 - 2024 | https://doi.org/10.3389/fbiom.2024.1347324
Cancer affects tens of millions of the world’s population each year with a stark mortality rate. It is well established that in order to be effective in treating solid tumor cancers, the current treatment methods used often sacrifice surrounding healthy tissue and cause damage at the site of treatment, inducing changes to the surrounding microenvironment. These changes to the microenvironment can lead to adverse side effects as well as long-term damage which continues to have a detrimental impact on the patient’s quality of life, even after remission. It is believed that by modulating the tumor microenvironment (TME) post-treatment, not only may the efficacy of current treatments be improved, but such associated negative side effects, as well as further complications arising from treatment, including metastasis, have the potential to be reduced. Mediating the microenvironment is also considered to aid in repairing the damaged site post-treatment, subsequently making the conditions more favourable for promoting regenerative processes. This review provides a brief overview of the alterations in the TME resulting from the three main cancer treatments–chemotherapy, radiation therapy and surgery–and the most common tissue engineering methods currently used in an attempt to mediate the TME post-cancer therapy. Furthermore, it investigates new emerging technologies within this field and the progress of such methods in terms of reaching the clinical setting.
Cancer is a worldwide public health issue and, based on the most recent data collected by the Global Cancer Observatory in 2020, just under 20 million new cases and 10 million deaths were attributed to cancer worldwide (Chhikara and Parang, 2023). The three most common treatment options for solid tumors are chemotherapy, radiation therapy and surgical resection. The treatment regime that a patient subsequently receives is dependent on numerous factors, including the anatomical location of the tumor(s) and whether metastasis has occurred, as well as taking into consideration the age and general health of the patient. Whilst the three aforementioned treatment options are often largely effective in treating cancer, by causing adverse effects to the tumor and tumor microenvironment (TME), they can all cause damage to the surrounding healthy tissue, which can negatively impact a patient’s quality of life. Importantly, these adverse effects can be experienced long after treatment has finished and the cancer has been cleared, leaving patients with long-lasting impairments, in some cases for the rest of their lives. This typically arises as a result of the treatment influencing surrounding healthy tissue; and whether as a direct or indirect result of the treatment, the tissue microenvironment surrounding the original cancer site becomes damaged. Not only can this altered, harsh microenvironment lead to adverse side effects, but it can also hinder the repair and subsequent regeneration of the surrounding tissue post-treatment. Indirect damage to the microenvironment is not limited to the area targeted by the treatment, and surrounding tissue is often also affected. Acute signs of damage to the TME include elevated levels of reactive oxygen species (ROS), inflammation, and cell death (Landskron et al., 2014; Barker et al., 2015; Yang et al., 2018), while chronic signs include fibrosis (Chandler et al., 2019). Developing a therapeutic strategy to mediate the TME during and after cancer treatment is a viable approach to improve the patient’s quality of life. However, the treatment regime will also play a role in how the TME is altered, which will have a subsequent effect on the method in which the microenvironment can be mediated.
Current cancer treatments focus on clearing the cancer cells, with an emphasis on preventing recurrence. However, the damage caused to the surrounding microenvironment remains after treatment has ceased, even as the patient is in remission, and can cause life-long adverse effects. These post-remission effects are not often taken into consideration prior to and during treatment and there are limited treatment options currently available to target this severe consequence. However, there is some evidence that mediating the microenvironment to a state that closely resembles that of homeostasis could potentially improve the repair and regeneration processes within this ‘damaged site’ (Yao et al., 2019). In particular, focus on gaining an understanding of the changes that occur at the level of the tissue microenvironment will be crucial in designing successful interventions to benefit the patient.
This review aims to introduce cancer and the TME, and to examine current cancer treatments for solid tumors, the effects that these treatments subsequently have on the surrounding microenvironment and methods in which these effects can be remedied. Various methods have been investigated in attempts to overcome the harsh microenvironment that arises from cancer treatments, primarily with the aim of preventing reoccurrence and regenerating functional tissue. Such methods include tissue engineering; primarily using biomaterials such as electrospun mats and hydrogel systems which can incorporate and introduce cofactors, drugs, or other small molecules into the microenvironment. An overview of the main methods described within the review, which both initiate damage to the TME and those currently used to mediate the TME is shown in Figure 1. A summary of the methods used post-cancer for microenvironmental reversal can be found in the Supplementary Material S1. It is worthy to note that there is a plethora of literature that delves into alternative routes to both treat cancer and also mediate the TME, such as immunotherapy and nanomedicine, respectively, as well as neoadjuvant approaches (Aboulkheyr Es et al., 2018; Mendes et al., 2021; Gugenheim et al., 2022; Li et al., 2022; Sahu et al., 2022; Yan et al., 2022; Ou et al., 2023; Yang et al., 2023); however the purpose of this review is to evaluate, and give an overview of, the most common types of treatment (radiation, chemotherapy and surgery) and specifically tissue engineering approaches to mediate the damage of these treatments inflicted upon the TME.
Figure 1. Overview of the methods that initiate damage to the TME and some tissue engineered methods that are currently employed to mediate the damaged TME. Common administration routes for each tissue engineering method are also provided (teal), where implant and topical application are directly applied to the exposed surgical site, and wound dressing refers to skin surface applications. A more detailed description of the TME is provided in Figure 2. Created using BioRender.com.
Furthermore, for the purpose of this review, environmental change refers to any process in which an environment is altered, either as a direct result of, or indirectly from, the treatment.
There are over 277 types of cancer, generally classified by the abnormal proliferation of immortal cells, by which the reproduction of normal cells becomes uncontrolled (Sarkar et al., 2013; Hassanpour and Dehghani, 2017). During the normal cell cycle, any cell which becomes damaged or “old” dies and is replaced by a newly generated cell. When this controlled process is disrupted, the aged or damaged cells are not replaced and instead they continue to survive and divide whilst new cells are still generated. This leads to a mass of cells which can promote the formation of a tumor.
The TME refers to the microenvironment around the tumor and all it encompasses (Figure 2), from the blood vessels to stroma, cancer stem cells (CSCs), epithelial cells, fibroblasts, immune cells (including macrophages and T cells) and other factors including growth factors, cytokines, the extracellular matrix (ECM), cell-matrix interactions, pH and oxygen concentration (Yang and Gao, 2017; Arneth, 2020; Bhattacharya et al., 2020; Blache et al., 2020). Compared to a healthy microenvironment, characteristics of a tumor microenvironment can include reduced oxygen concentration which ultimately leads to hypoxia, more acidic pH, remodelling of the ECM and the presence of a plethora of cells which activate inflammatory and immune responses, promote angiogenesis, and support the structure of the solid tumor (Fernando et al., 2021; Suwa et al., 2021). The distance between blood vessels and cells, which is maintained in a normal microenvironment, becomes disrupted within the TME. Generally, this distance expands to a point where regions become deprived of a sufficient blood supply, meaning there is a lack of oxygen and nutrients delivered to these areas. It is well known that the TME is also influenced by external factors, such as age, as discussed in a recent review (Fane and Weeraratna, 2020). Until recently, cancer research has focused on cancer cells alone, however more recent studies have found that tumors are intricately related to the TME, with tumor cells interacting closely in regard to their development, function and their response to treatments (Giussani et al., 2015; Hirata and Sahai, 2017). These constant interactions mean that the structure and growth of the tumor are continuously evolving (Hass, 2020). With the newfound understanding that the TME is directly involved in events such as tumor progression and metastasis, and therefore plays a role in the efficacy of various treatments, it is important to consider not only the traits of cancer cells themselves but also the TME that they are found in, and whether it can be activated and used as a restorative agent (Hanahan and Weinberg, 2011; Barker et al., 2015).
Figure 2. A simplified schematic of the TME depicting the ECM, blood vessels and typical cells found within the microenvironment, including cancer cells, cancer-associated fibroblasts (CAFs), cytotoxic T cells, mesenchymal stem cells (MSCs), lymphocytes, dendritic cells, and tumor associated macrophages (TAMs). Also highlighted are some of the influential changes associated with the TME. Created using BioRender.com.
Given the close proximity of the TME to cancer cells targeted by treatment, the TME is invertedly changed during cancer treatment. These changes, and other factors regarding the TME, such as the cell types present, need to be taken into consideration when designing a platform for its mediation. Some of the major influences of the TME are detailed in Figure 2 and described below.
Within the TME, tumor vasculature is altered and becomes geometrically abnormal. The shapes of the blood vessels change and become more permeable due to the development of an irregular endothelial cell lining and a decrease in smooth muscle cells (Graham and Unger, 2018). These changes to the structure of blood vessels have a negative impact on their function, hindering blood flow and subsequently reducing the delivery of oxygen to the area.
Regulation of oxygen levels is essential for homeostasis, therefore any deviation from this within the TME, particularly a reduction, can indicate phenomena such as hypoxia. Hypoxia within the tumor and its surrounding microenvironment is one of the hallmarks of cancer and has been found to be significantly influential in the effectiveness of treatment, likely due to its involvement in affecting multiple molecular pathways including glycolysis, cell proliferation and angiogenesis (Hanahan and Weinberg, 2000; Sørensen and Horsman, 2020). It is found within all solid tumors and influences a patient’s response to all treatments, with the exception of surgical resection. Consequently, hypoxia is the most researched parameter within the TME and is a major target in improving cancer treatments. There are multiple mechanisms involved in the development of a hypoxic microenvironment, and even within the same tumor the regions and levels of hypoxia are not always uniform (Graham and Unger, 2018). Hypoxia has been associated with an increased risk of mortality, enabling tumor cells to survive in typically unfavourable conditions such as low oxygen concentration, as well as increasing their resistance to radiation (Bhattacharya et al., 2020; Mughees et al., 2021).
Initial events following radiotherapy and chemotherapy treatment that occur at the damaged site include double strand DNA breaks, production of reactive oxygen species (ROS), subsequently leading to an overall increase in ROS levels, and general stress responses. These factors induce damage to the cells, which can lead to a number of detrimental outcomes, including apoptosis, senescence and mitotic catastrophe, as well as inducing inflammatory responses and fibrosis at the tissue level (Gao et al., 2018). Following surgical resection of a tumor and its associated microenvironment, a number of wound healing responses are activated, including COX-2 enzyme (inflammatory response) and transforming growth factors (Predina et al., 2012). However, research has found that these factors can actually promote the survival of any remaining cancer cells, causing subsequent recurrence (Predina et al., 2012).
Through interaction with pro-inflammatory cells, such as macrophages, neutrophils, and lymphocytes, as well as non-cellular components, cancer cells aid in the production of the inflammatory TME. This results in chronic inflammation which is a key factor in cancer development, as well as promoting tumorigenesis, as detailed in other literature (Greten and Grivennikov, 2019; Wen et al., 2022).
Under healthy tissue conditions, the function of fibroblasts is to inhibit tumor growth and maintain homeostasis within the tissue. However, when in close proximity to tumorigenic cells, the healthy fibroblasts are influenced by the signals emitted from these cells and undergo irreversible morphological changes, producing cancer-associated fibroblasts (CAFs). A detailed description of CAFs and a comparison to normal fibroblasts, as well as how the interact with the TME has previously been published (Liu et al., 2019). CAFs have been shown to promote tumor progression, metastasis and importantly, CAFs are known to reduce the efficacy of the naturally occurring tumor immunity found within the microenvironment, subsequently reducing the efficacy of existing cancer treatments, in particular, chemotherapy (Farhood et al., 2019; Joshi et al., 2021).
One particular aspect of the TME worthy of note is the presence of cancer stem cells (CSCs) and their plasticity–a property that enables cells to change their phenotypic expression as a result of environmental cues, without causing any genetic mutation. CSCs have been found to exhibit enhanced self-renewal, differentiation and tumorigenesis in multiple cancer types (Kruyt, 2023; Salem and Salo, 2023). For these reasons, complete obliteration of CSCs is a major factor that should be considered when looking for effective therapy methods.
Not only does the TME influence the evolution of the tumor, but it also has an effect on its response to cancer therapeutics (Hirata and Sahai, 2017). This can be utilised to provide new avenues for therapeutic treatments, including improving the results of currently available treatments as well as novel treatment methods. The most significant challenges to tackle when developing new therapeutics in cancer treatment is tumor recurrence and metastasis (Zhao and Cui, 2020). Even in cases where chemotherapy and/or radiotherapy are given post-operatively following surgical resection of solid tumors, recurrence occurs in up to 40% of patients (Predina et al., 2013). Additionally, the unspecific targeting nature of current treatments, such as chemotherapy, often leads to reduced efficacy, resistance to treatment and subsequent cancer recurrence. The immunosuppressive nature of the TME is another influencing factor that appears to act as a protective barrier, defending the tumor from therapeutics and allowing it to thrive (Jin and Jin, 2020; Parayath et al., 2020). However, methods of overcoming such issues are currently being studied in the area of tissue engineering. One of the drawbacks in using tissue engineering to repair, and ultimately restore and regenerate the microenvironment, is that many of the factors used to promote these processes can also facilitate tumor survival, growth and metastasis, such as is the case with factors targeting improving angiogenesis (as reviewed in Zimmerlin et al., 2013).
Such changes to the TME and the microenvironments of surrounding healthy tissues and organs are attributed to the unpleasant side effects, notoriously associated with current cancer therapies. These side effects range from short term effects experienced during treatment to long term side effects which are experienced for a prolonged period of time post-treatment, ranging anywhere from months or years after treatment to the rest of the patient’s life. Most of these side effects have enough of an impact to affect the person’s day-to-day activities, leading to poor quality of life, as well as impacting their health and wellbeing. Currently, there are limited treatments available for such impairments, and most options only offer palliative, surface level care. If a detailed understanding of how the TME responds to the various cancer treatments can be established then interventions can be developed to overcome these post-treatment side effects, and thus improve the patient’s quality of life after cancer.
Tissue engineering is an emerging technology concept discussed in detail throughout literature which primarily utilises specifically engineered constructs to aid the repair of damaged tissue, using combinations of biomaterials, cells and biochemical cues to mimic the healthy normal tissue (Yoo et al., 2014; Lombaert et al., 2017; Micek et al., 2020; Unnikrishnan et al., 2021). A variety of tissue engineering methods have been applied to cancer research, not only to support repair but also to mimic the native TME for in vitro modelling, including using patient-derived cells to obtain more clinically relevant data (Unnikrishnan et al., 2021), drug screening and toxicity studies for radiotherapy and chemotherapy (Xu et al., 2014).
Biomaterials have commonly been used to support the repair, and in some cases regeneration, of damaged sites. This is due to the similarities that can be observed within their structure and composition to the natural extracellular matrix. As a result, biomaterials can mimic the natural cellular environment, creating new microenvironments capable of enabling cell attachment, growth, and proliferation, by providing mechanical support to control factors such as cell phenotype and behaviour. Electrospun scaffolds in particular show great promise as therapeutic agents as they are used to mimic the native ECM, with high drug loading capabilities which makes them suitable for both drug delivery systems for local cancer therapy, which can further be tuned to release the drug in a stimuli-responsive manner, and also to influence tissue regeneration (Hinderer et al., 2016; Liu et al., 2018; 2021; Blache et al., 2020). Electrospinning is the process of applying a high voltage electric field to a polymer solution to create a fibrous mat, known as an electrospun scaffold. Numerous parameters, such as temperature and humidity, voltage, solvent used to dissolve the polymer, and parameters within the electrospinning setup itself, are all vital in controlling the fabrication process. Altering any one, or combination, of these parameters enables control over many characteristics of the produced fibre scaffold, including fibre diameter, surface topography, porosity and fibre alignment. All of these subsequently affect the relationship between the cells and the scaffold, and can therefore be tailored to the desired application of the scaffold. One feature of electrospun scaffolds suggested to be beneficial in cancer therapeutics is an appropriately designed electrospun mat with well-interconnected porosity, which can have the potential to trap cancer cells, preventing them from cell migration and thus reducing metastasis (Samadzadeh et al., 2021). Electrospun fibres in particular are advantageous in that they have a large surface area to volume ratio which aids drug release, and they are also able to be physically implanted during surgical resection procedures. Studies have also found that electrospun scaffolds can be used to promote angiogenesis, which is essential for successful implantation and acceptance of the graft in the body (Montero et al., 2012; Gigliobianco et al., 2015). However, promotion of angiogenesis should be avoided until it is certain that there are no remaining cancer cells present. Similarly, hydrogels are another form of biomaterial that can be employed. Hydrogels are cross-linked polymer networks that are prepared and then surgically implanted, and have the potential to be used in a variety of applications, including repair and regeneration, as cell or drug delivery systems, attributing their biomimetic properties and multifunctionality (Zhang et al., 2016). Over fibrous scaffolds, hydrogels have the advantage of the ability to create the material in a liquid form that can respond to environmental stimuli to form a gel in situ. This allows a non-invasive delivery method for the precise delivery of either drugs or cells, which reduces the risk of post-surgical infection, as well as reducing trauma to surrounding tissue (Zhang et al., 2016). Modification of the synthesis of the polymer allows properties of the hydrogel, including water content, mechanical properties and drug release profiles, to be tailored for each individual application. Additionally, hybrid scaffolds of electrospun scaffolds and hydrogels can also be fabricated. Recently, there has been an increase in hybrid scaffolds, where electrospun fibres and hydrogels, such as PCL and alginate, respectively, are used to promote the effects seen by each individual material to yield improved results (Chen M. et al., 2019; Koch et al., 2022).
A list of polymers commonly used to fabricate biomaterials is provided in Table 1. As shown, most polymers are used in the fabrication of both electrospun scaffolds and hydrogels; however, PCL and PTFA are two synthetic fibres that have only been used in the electrospinning process and only exist in hydrogels when used as a polymer backbone to support a gel in hybrid systems. There are many reasons for selecting particular biomaterials, including their indented applications and for their functions within the TME. The advantage of using biomaterials is that, whilst there are some more suited to other functionalities than others, they can be tailored to suit both the purpose and application of their intended use. For example, PLGA, a synthetic polymer, can be fabricated into both electrospun fibres and also hydrogel systems. The same holds true for natural biopolymers such as alginate, collagen and gelatin (Xue et al., 2019). These can be used alone or in combination with other biomaterials–combinations are commonly used to enhance the properties of the existing biomaterials. For example, studies have found that coating alginate fibres with molecules such as glutaraldehyde or chitosan improves the mechanical properties of the alginate fibres (Najafiasl et al., 2020). Alginate is already a popular choice of biomaterial due to its easy gel formation and its ability to perform as an effective carrier and delivery system for drug and biomolecule compounds, including genes. Using combinations to improve other properties of the material, such as chitosan to improve tensile properties, makes an appealing biomaterial choice even more attractive. Hyaluronic acid (HA) is another popular biomaterial most commonly used post-operatively, particularly in the form of a hydrogel, and can be combined with both natural molecules as well as synthetic polymers (Abatangelo et al., 2020). However, care must be taken when using HA, as it has been shown to regulate the TME and promote the malignant phenotype of cancer cells, and lead to multidrug resistance (Markowska et al., 2023). So, whilst HA is beneficial in applications such as wound repair, studies have shown that it can also effectively feed the cancer, causing it to grow and spread.
Table 1. Common polymers used in biomaterials, their biomaterial forms, and compound types commonly added to the biomaterial.
Biomaterial scaffolds can also be combined with other therapies, such as stem cell and immune therapy to heighten or produce multiple simultaneous/subsequent effects (Zhao and Cui, 2020). This can also be achieved by incorporation of additional compounds, such as drugs or growth factors, to elicit various beneficial responses within the TME. For example, Quercetin is one commonly used compound that is often incorporated within both electrospun fibres and hydrogel systems. Quercetin has been shown to display multiple beneficial properties: as an antioxidant to suppress oxidative stress through free radical scavenging; inhibiting inflammatory enzymes such as COX to act as an anti-inflammatory agent; reduces fibrogenesis; and also acts as an anticancer by decreasing expression of CSCs and myofibroblast markers, suppressing proliferation and mitosis and has also been shown to reduce drug resistance (Ajmal et al., 2019; Asgharian et al., 2021; Reyes-Avendaño et al., 2022). Growth factors are also commonly added to scaffolds as a means to repair and restore the environment back to normal–VEGF is one example which has been incorporated into different scaffold systems and has been shown to act on both the vasculature of the local environment but also directly with cells and other molecular components of the surrounding environment. Studies have suggested that using polymer scaffolds as a means of growth factor delivery can play a vital role in aiding regeneration in where the vasculature of the environment has been damaged through means including enhancing angiogenesis, increasing blood vessel density and vascular perfusion, as well as displaying capacity to increase bone formation (Kaigler et al., 2006).
Another method is stem cell therapy, which uses a wide variety of techniques, often in conjunction with the aforementioned biomaterials, to improve the efficacy of the biomaterial construct or to tackle multiple concerns simultaneously. Stem cells are extremely versatile and have several properties which make them special, including self-renewal, differentiation, migration and tissue repair (Dalerba et al., 2020). Stem cell therapy is most commonly used for regenerative purposes and has significant potential and a large amount of interest in terms of cancer treatment. Specifically, due to their involvement in several processes which aid the development of tumors, targeting CSCs could improve the efficacy of cancer treatment (Duan et al., 2021).
Whilst not specifically a tissue engineered approach, drugs are often incorporated into such constructs and used in the remodelling of the TME (Ye et al., 2019). Biomaterials are commonly used as drug delivery systems and to improve properties such as bioavailability and solubility in anticancer drugs which on their own, perform poorly, with the additional benefit of being able to cleverly control the release kinetics. By designing systems with features such as release only under certain conditions means that greater control and more specific targeting can be achieved. This approach is extremely common, as will be shown throughout the review where tissue engineering approaches are used to create biomaterials-based modalities for delivering treatment without causing the extensive damage caused via traditional treatment options.
Chemotherapy is typically used for advanced cancers, particularly when metastasis has occurred. It can be used as a curative measure, both by itself and in combination with other treatments such as radiotherapy or surgery (both pre and post), as well as for palliative treatment. Chemotherapy works by using cytotoxic drugs, including paclitaxel, doxorubicin, or fluorouracil, to inhibit the growth of and destroy cancerous cells; however, it is not selective and as such, also targets healthy cells.
Most conventional chemotherapy drugs are administered as part of a ‘cocktail mix’ of drugs, typically delivered intravenously. The administration periods and cycles vary on an individual basis. Recent research has looked at alternative methods of delivering chemotherapy agents, including tissue engineered biomaterial scaffolds, hydrogel systems and other alternatives, and in some cases novel, drug delivery systems. These alternative methods of administration have the advantage of being able to incorporate additional agents to deliver to the site, which can provide benefits such as mediating the TME. Chemotherapy is often combined with surgical resection of tumors; therefore, research has looked at ways in which chemotherapeutics can be inserted into the tumor resection site. This is often achieved using biomaterials such as electrospun scaffolds or hydrogel systems. It is commonly seen in polymer fibres loaded with a drug that there is an initial burst release of the drug followed by a period of sustained release which can range from 14 days to 80 days (Ranganath and Wang, 2008; Samadzadeh et al., 2021). With typical drug administration routes, it is common for drugs to only become effective once they have reached therapeutic concentrations within the body, which in some cases can take up to several months. Such results indicate that having the ability to directly implant the desired dose of drug into the target site could offer the possible benefit of reducing the time taken for the drug to produce its therapeutic effect. Another advantage of using a biomaterial scaffold as opposed to traditional administration routes such as IV is that the drug delivery is more localized, meaning that there is less accumulation of the drug in areas other than directly at the tumor site, constituting less damage to healthy tissue and thus reducing adverse effects (Chen et al., 2020).
Chemotherapy drugs target many aspects of cell physiology, including DNA replication, RNA synthesis, cell structure and metabolism. The cytotoxic nature of chemotherapeutic agents can lead to numerous side effects including nausea, anemia, fatigue, immunosuppression, and alopecia. In long-term studies, researchers discovered that children who had received chemotherapy also suffered from long lasting side effects, including fertility issues, organ disfunction and cognitive impairment (West et al., 2009; Demaria et al., 2017; Goossens et al., 2020). The wide range of side effects is the result of chemotherapeutics targeting a wide range of cell-cycle dependent mechanisms, and often specifically rapidly dividing cells. This includes cells found in hair follicles, bone marrow, the digestive tract and reproductive system (Prieto-Callejero et al., 2020). Cellular senescence inhibits cell pathways and halts cell proliferation, therefore suppressing cancer. During chemotherapy, the action of the drugs can induce cellular senescence, creating therapy-induced senescence cells, which remain in the microenvironment and can trigger inflammation. A study by Demaria et al. revealed that there was a direct relationship between the removal of these therapy-induced senescent cells and a reduction in several side effects (Demaria et al., 2017). The results of the study indicated that the presence of these cells contribute to the side effects observed with chemotherapy, thereby making them a desirable target in improving anticancer treatments (Demaria et al., 2017). Senolytics have been developed to selectively target senescent cells as an alternative therapeutic approach, and have been found to successfully clear senescent cells whilst also reducing cancer relapse and improving the treatment-damaged environment (Demaria et al., 2017; Barazzuol et al., 2020). However the removal of senescent cells also impacts regeneration in a number of tissues so a fine balance will be necessary in order to be beneficial (Elder and Emmerson, 2020).
The efficacy of chemotherapy can be affected by many factors, such as vasculature of the tumor, which impacts drug delivery. Chemotherapy drugs are designed to damage the rapidly dividing cancer cells, inducing tissue damage through mechanisms such DNA damage and ROS production, but other surrounding healthy cells also succumb to the same damage (Gao et al., 2018). This can potentially lead to activation of pathways/events which can protect tumor cells from DNA damage, but also protect cancer cells against the cytotoxic action of chemotherapy drugs. For example, research has shown that chemotherapy agents can promote hypoxia in the TME, which drives the activation of hypoxia-inducible factors (HIFs), in particular HIF-α. This in turn, through a series of events, can influence not only growth and progression of cancer, but also metastasis and chemo-resistance (Li et al., 2016; Budi and Farhood, 2023).
New approaches to delivering chemotherapy agents can bring forth many new concepts, such as delivering agents capable of mediating the TME, including growth factors, which not only better the efficacy of chemotherapy but also provide these additional benefits. Methods which have been used to reverse damage to the TME following chemotherapy are outlined in Table 2, which highlights whether the approach aims to modify the environment, or initiate or aid regeneration. Both studies provided used mesenchymal stem cells to promote ovarian function restoration post-chemotherapy. One example is a combined chemo-photodynamic therapy which is directly injected into the tumor to trigger a series of anti-tumor immune responses. However, during their studies, G. Yang et al. also discovered that this injection was able to relieve hypoxia and unexpectedly also found that, when used in combination with checkpoint blockade therapy, tumors at distant sites were reduced, meaning that this treatment also has promising potential for tumor metastasis (Yang et al., 2017). Chemotherapy drugs are often regarded as a double-edged sword, as whilst they are cytotoxic to cells, they can also activate reaction pathways and other processes which subsequently result in off-target effects. Another important consideration regarding chemotherapeutics is that the cells can quickly become resistant to the cytotoxic effects. However, some studies found that using a synergistic combination of chemotherapy drugs and other small molecules can reduce the accumulation of tumor promoting activity and enhance the tumor response to the chemotherapeutic (Kemp et al., 2016).
As shown in Table 2, there is currently a limited amount of investigation and proposed tissue engineered treatment approaches in mediating the microenvironment post-chemotherapy. While this is not a comprehensive overview of all studies it acts to highlight the most clinically relevant avenues which are showing good potential in post-chemotherapy care.
Radiation therapy is one of the key treatment options for cancer, with approximately 50% of all cancer patients receiving radiotherapy as either a stand-alone treatment or in combination with surgery or chemotherapy (Barazzuol et al., 2020). Depending on the type and stage of the cancer, radiation therapy can be used as a cure for cancer, prevent recurrence, halt its growth or spread, as well as for palliative reasons to ease symptoms. It is predominantly used when the cancer is in its early stages and can be delivered in many different ways, with its main purpose being inducing DNA damage within the cancer cells. The most well-known method of administration is external radiotherapy, where a dose of radiation is beamed directly onto the target area and passes through the body. Alternatives include radiotherapy implants or injections where a radioactive material is administered into the body where it remains for anywhere from minutes to days, with some small implants being permanently placed. The treatment is usually given as fractionated doses, where the total amount of radiation to be delivered is divided into small amounts over a number of days. Fractionated radiation not only maximizes the effect that the radiation has on the cancer cells, but also to reduce the amount of damage caused to healthy cells, with some studies showing that tumor cells are more susceptible to fractionated radiation than healthy cells (Baskar et al., 2014; Wang J.song et al., 2018). The dose given is specifically calculated for each patient.
Radiotherapy damages cancer cells by using radiation to damage the DNA within the cells, hindering their growth and causing cell death. This does not happen immediately; therefore, a course of radiation treatment is provided to give enough radiation to initiate this process and to continue destroying cancer cells for a duration of time after. Due to its reduced blood supply, the TME can impair the efficacy of radiotherapy as this reduces the accessibility of radio-sensitizers to the area (Koukourakis and Giatromanolaki, 2020; Sørensen and Horsman, 2020).
Penetrating radiation causes cellular stress as a result of several responses including the activation of the damage repair cascade in normal tissues. This begins with DNA damage, the production of ROS, subcellular organelle damage (incorporating the mitochondria and endoplasmic reticulum) and autophagy (Kim et al., 2019). Whilst these responses are all actively involved in cancer cell death, the activation of damage-repair and survival signaling responses can be activated in a small number of the cancer cells present, ultimately resulting in cancer cell survival and tumor resistance to radiation (Kim et al., 2019). This can then lead to tumor regrowth and/or recurrence, hindering the overall effectiveness of radiotherapy. Following exposure to irradiation, there is an increase in the repair and protect mechanisms as well as the level of molecules associated with them, such as superoxide dismutase and glutathione to name a few (Tarnuzzer et al., 2005). The molecular pathways and mechanisms arising from irradiation therapy-related adverse TME conditions have been described in detail in several reviews (Lara et al., 2015; Morgan and Sowa, 2015; Sprung et al., 2015)
Whilst radiation is effective in initiating damage in cancer cells, it also targets local healthy cells. Not only do some patients get recurrence or metastasis of the cancer from instances where not all cancer cells are depleted following treatment, but the large majority of patients also experience unpleasant side effects. These can vary depending on the region targeted by the radiation beam. For example, a common side effect incurred during radiotherapy for head and neck cancer is xerostomia, more commonly known as dry mouth (Nelson et al., 2013; Holmberg and Hoffman, 2014). This can continue long after the treatment is complete, leaving the patient with life-long conditions. Many of these ailments that arise as a side effect of a particular treatment are only managed using surface-level treatments.
An overview of the methods used to mediate the TME post-irradiation therapy is provided in Table 3. One of the challenges in finding effective methods to overcome damage to normal tissue is the fact that whilst normal tissues will have close to identical cellular processes, there are several independent, localized parameters, such as the loss of stem cells, that can cause different organs to react in a completely different manner. This is covered in detail in a review conducted by Barazzuol et al., which discusses both the short- and long-term effects of radiation on the salivary glands, cardiopulmonary system and brain (Barazzuol et al., 2020).
Recent work has delved into how altering the precision of the radiation dose given can improve treatment by reducing exposure of otherwise healthy tissue. One such method that has been investigated for its beneficial non-invasiveness of non-cancer tissue is intensity-modulated radiotherapy (IMRT), which precisely delivers the target dose of radiation to the tumor or specific areas within the tumor (Taylor and Powell, 2004). A similarly interesting concept, currently in preclinical development, is microbeam radiation therapy (MRT), which delivers one large dose of fractionated radiation across the tumor in a grid pattern, meaning that some areas of the tumor are hit with radiation whilst other areas are spared (Fontanella et al., 2015).
A number of methods focus on reoxygenation of the microenvironment (Siemann and Horsman, 2015; Yang et al., 2021). It is well established that oxygen deficiency, leading to a hypoxic microenvironment, in the TME limits the efficacy of radiotherapy. In an attempt to overcome this, as number of methods have looked at ways in which oxygen levels can be replenished, and what makes the use of biomaterials in such applications particularly exciting is the ability to manipulate the biomaterials to gain different results. For example, Yang et al. developed a hydrogel for the purpose of increasing oxygen delivery to the hypoxic TME. But, what makes this design particularly interesting is its exploitation of the thermosensitive property of polymers. The delivery of a sufficient amount of oxygen to the hypoxic site without leaking any oxygen into blood circulation had proven difficult, but hydrogels offered one route that the exogenous oxygen could be delivered in a suitable dose, directly to the target site, and activated to trigger the release of oxygen, reducing the aforementioned issues previously encountered. When heated post-injection, the hydrogel released exogenous oxygen to the hypoxic site. When tested on a mouse model, the increase in oxygen was found to not only improve the efficacy of radiotherapy by relieving tumor hypoxia, it also induced downregulation of HIF-α, significantly reducing suppressing tumor growth and subsequently prolonging survival (Yang et al., 2021).
However, not all methods of correction have been successful. In a study performed by Tarnuzzer et al. evaluating the radio-protective effect of cerium oxide nanoparticles in normal and tumor cells, the treatment was almost 99% effective in protecting against radiotherapy in a normal human breast cell line but showed no significant effectiveness against a human breast tumor cell line (Tarnuzzer et al., 2005). Kaigler et al. found that by simply incorporating VEGF into a poly (lactide-co-glycolide) (PLGA) scaffold enabled better angiogenesis/neovascularization/blood vessel formation and function, as well as bone formation/regeneration following radiation (Kaigler et al., 2006).
Surgical resection of solid tumors is another common method of treatment and is the most obvious method of treatment for localized solid tumors. However, when removing the tumor, it is difficult for the surgeon to completely remove the cancerous mass in all of its entirety without touching the surrounding healthy tissue. The aim of surgery is to completely remove the tumor but leave surgical margins of healthy tissue to reduce the chance of the cancer returning. However, depending on the complexity of the tumor this is not always possible and there is no official guideline on what size this margin should be. Studies have shown that the type of cancer affects the conditions within the surrounding TME in different amounts and to different extents; therefore the resection margin differs accordingly and is usually determined on a patient by patient basis (Cha et al., 2020). In such cases, the surgeon will remove as much of the tumor as possible to reduce the tumor mass and then chemotherapy or radiation treatment will be given to clear any remaining cancer cells. This is then routinely confirmed by pathological inspection of the margins, and advice is given on whether further surgery is required. Surgery can also be used as a diagnostic tool in identifying if masses are malignant and to determine if and to what extent the cancer has spread. The surgical methods used in diagnostic cases, namely, biopsies, can induce the same response as tumor removal.
Whilst tumor resection does not introduce any drugs to the TME like chemotherapy, or directly induce cell damage and death at the target site in the same manner as radiation therapy, surgical resection of tumors also has a substantial impact on the TME, not just at the primary resection site but also at distant, metastasis sites (Alieva et al., 2018). Evidence that suggests that despite being the current ‘gold standard’ method of removing solid tumors, even minor and minimally invasive surgical procedures such as biopsies can cause a response from many different pathological processes, which can not only lead to tumor recurrence but also metastasis (Wang et al., 2017; Alieva et al., 2018; Hu et al., 2021). Even in patients that previously showed no metastatic indicators, there have been numerous instances where the cancer has returned and is metastatic following surgical resection (Chen Z. et al., 2019). Surgical resection can cause direct alterations within the TME that ultimately leads to a number of unfavorable changes including an increase in the number of cancer cells which circulate in the blood and suppression of anti-tumor immunity (Tohme et al., 2017b).
One of the major concerns following surgical resection specifically is local cancer recurrence and is a common problem in several types of cancer often caused by inadequate resection margins, which allows any remnant cancer cells to continue to proliferate. Complete resection with no residual cancer cells is thought to be key in not only preventing metastasis, but also in reducing the likelihood of chemoresistance, which often arises due to chemo-resistant and senescent cells (Bilbao et al., 2021).
The physical act of surgery and cutting through tissue also influences injury-induced inflammation within the TME; this is one of the main causative factors related to surgical resection that contributes to an adverse TME. There is an abundance of evidence regarding inflammation post-surgery and inflammatory cells influencing cancer progression (Forget et al., 2013; Tohme et al., 2017a; Singh et al., 2019; Kinoshita and Goto, 2021; Zhao et al., 2021). An additional aspect that needs to be taken into consideration, specifically when performing surgical resection, is the loss of function within the organ. This is important with organs such as the lung and liver, where organ function reduces quite drastically following resection, particularly in cases where large margins are required (Cha et al., 2020). For example, a lobectomy for lung cancer can reduce the function of the lung by 25%, in addition to its already hindered performance due to the cancer. Whilst a wedge resection is not as drastic, the likelihood of local cancer recurrence increases up to two-fold (Samadzadeh et al., 2021).
As shown in Table 4, the vast majority of interventions used as a reparative approach post-surgery are hydrogel systems placed in the surgical incision site following tumor removal. A recent review by Feng et al. gives a fantastically detailed insight into hydrogel-based biomaterials used postoperatively and is recommended by the authors (Feng et al., 2023). One exciting approach developed by Chen et al. incorporates a bio-responsive immunotherapeutic gel in spray form that can be directly sprayed onto the tumor resection cavity (Chen Q. et al., 2019). The gel contained anti-CD47 antibody, which increases phagocytosis of cancer cells by macrophages. Furthermore, through H+ scavenging via calcium carbonate nanoparticles, which impairs initial T cell responses that in turn control tumor growth, the gel was also able to facilitate the reversal of the immunosuppressive TME and induce systemic immunological responses to prevent both local recurrence in the tumor cavity and also any potential metastatic spread (Chen Q. et al., 2019).
One of the benefits of the various tissue engineering approaches, such as those discussed within this review, is the ability to incorporate components which provide additional benefits. This is highlighted in a method by Wang et al. where they developed an injectable hydrogel containing the chemotherapy drug gemcitabine and anti-PD-L1 blocking antibody (aPDL1) (Wang C. et al., 2018). Whilst the hydrogel locally delivers the chemotherapeutic to promote an immunogenic tumor phenotype within the TME, the release of aPDL1 increases antitumor responses, leading to immune-mediated rejection of the tumor and also preventing tumor recurrence after the tumor has been surgically removed (Wang C. et al., 2018). A similar concept was used by Hu et al. whereby a hydrogel system was used to deliver combination immunotherapy to the surgical resection site following tumor resection to prevent the recurrence of cancer (Hu et al., 2021). Naturally occurring compounds can also be used to exploit their benefits. In a study by Jain et al., (derived from black pepper) was incorporated into an electrospun fibre, and was shown to display anticancer properties (Jain et al., 2016). They found that the inclusion of piperine generated ROS and induced cell cycle arrest, both leading to the death of cancer cells, with no deleterious impact on healthy cells.
As with any field, there are constantly exciting new and emerging technologies being developed with regards to mediating the damaged microenvironment following cancer. Tissue engineering is one discipline which has been explored to counteract these problems. Such emerging approaches have been summarized in Table 5.
Table 5. An overview of some of the emerging technologies within the field, including the treatment provided, the reparative approach used and its target.
In recent years, there has been an increase in employing the use of biomaterial scaffolds as engineered pre-metastatic niches (Aguado et al., 2018). These sites, conditioned as a subsequent effect of systemic responses to the primary tumor, are found in distant organs and are suited to the growth and survival of tumor cells before the cells are even present in the site (Aguado et al., 2017; 2018; Peinado et al., 2017). It has been found that the introduction of such a scaffold elicits a foreign body response effect when implanted, meaning that immune cells and/or including metastatic cells are drawn to the scaffold (Dondossola et al., 2017). Biodegradable polymers are a good choice of material as once implanted, they safely degrade within the body whilst reinstating the microenvironment, with many polymers, such as polycaprolactone, already having Food and Drug Administration (FDA) approval. It is believed that the main reason for the failure of tissue-engineered constructs in vivo is due to incompatibility and the inability to integrate the graft with the patient’s own tissues. This is primarily due to poor neovascularization, meaning that the graft is unable to support cell survival and ultimately cannot survive long-term (Gigliobianco et al., 2015; Sun F. et al., 2021). For this reason, there are a number of emerging biomaterials which can incorporate and release growth factors to promote the formation of new blood vessels. Vascular endothelial growth factor (VEGF) is one particularly popular growth factor that is incorporated into scaffolds, as it is believed that even on its own, VEGF is capable of inducing angiogenesis (Gigliobianco et al., 2015; Campbell et al., 2017; Seah et al., 2020; Sun H. et al., 2021; Kim et al., 2021). However, inducing angiogenesis should be undertaken with caution, as inducing the formation of new blood vessels in areas where cancer cells are still present can facilitate tumor growth and could allow the cells to enter the systemic blood flow, with the possibility of inducing metastasis (Muz et al., 2015).
One of the major drawbacks to current chemotherapy treatments is that an ineffective amount of drug reaches the tumor using typical administrative routes. One prime example of this is that when administered intravenously, less than 0.5% of the total dose of paclitaxel given reaches the tumor (Chua et al., 2020). This is difficult to overcome by simply altering the dose as factors such as the drug properties and pharmacokinetics/pharmacodynamics, as well as toxicity, need to be considered. Researchers are currently looking at new drug delivery systems that will enable the drug to be delivered directly to the target site. One popular method is incorporating the drug into biomaterials such as electrospun mats or hydrogel systems. Scaffolds are commonly being used as a local drug delivery method in cancer treatment, as they have the potential to cover multiple avenues, including stimuli-responsive drug release and can be used in combination with other treatment methods such as stem cell treatment and immunotherapy (Zhao and Cui, 2020). One group developed a drug-eluting hydrogel system which, when implanted in a surgical resection site, has the benefits of delivering high doses of the drug directly to the target site, reducing systemic side effects, with continuous and sustained release (unlike intermittent doses with other drug delivery methods) (Brudno et al., 2018). Such qualities are ideal for cancer treatments, as it bypasses systemic administration, meaning less healthy cells are in contact with the chemotherapeutic thus reducing side effects arising from damage to healthy cells, and is overall less invasive and disruptive of the patient’s day to day life.
As well as incorporating growth factors and drug compounds into biomaterials, more recent studies are beginning to introduce other compounds such as antibiotics and antioxidants (Kim et al., 2004; Romanò et al., 2016; Celebioglu and Uyar, 2020). Such applications have been seen in relation to non-cancerous diseases, but a consideration of the effects of antioxidants in relation to cancer has gained traction in recent years. This approach allows the scaffolds to be produced to target other events and processes/pathways, potentially providing alternative methods of treatment. To date, such compounds are commonly used in wound-healing applications, such as the incorporation of antibiotics in hydrogels to reduce early post-surgical infection (Romanò et al., 2016). This approach is being evaluated in a number of different applications, where bioactive ingredients are being employed to scavenge free radicals, from using vitamin E in intravenous micelle formulations to scaffolds (Banshoya et al., 2021).
As previously mentioned, techniques are more commonly being used in combination to exploit the advantages of each individual technique and maximise the overall potential, as well as the ability to produce more than one therapeutic response. There are several published examples of such combinations, including incorporating anticancer chemotherapy agents with ROS responsive compounds to mediate the microenvironment, some of which are discussed below. An in situ, injectable bio-responsive hydrogel scaffold designed by Wang et al. is one example of this technique (Wang C. et al., 2018). In this study, the scaffold is suitable for localized chemotherapy through the incorporation of gemcitabine and aPDL1 into a ROS-degradable hydrogel which not only promotes tumor regression but also prevents tumor recurrence after primary resection (Wang C. et al., 2018). Another combined approach study delved into intraperitoneal chemotherapy using hydrogel systems to deliver chemotherapeutics directly into the abdomen as opposed to intravenously, in application for ovarian cancer (Sun et al., 2016). Such a targeted-delivery approach would be favourable over typical IV administration, which affects the entire body.
The field of nanotechnology and the use of nanoparticles (NPs) is a key interest of researchers in both repair and regeneration tissue engineering aspects, as well as in cancer diagnostics and treatments. There are numerous articles detailing the benefits of nanoparticles in cancer treatments, specifically in modulating the TME and drug delivery (Prabaharan et al., 2009; Li et al., 2018; Zhang Y. et al., 2020). They are commonly used in drug delivery systems as they provide numerous benefits including improving drug properties such as biocompatibility and solubility, which typically hinder the effectiveness of a drug (Yang and Gao, 2017). One advantage of NPs is that their surface chemistry can be modified and tailored to suit the desired outcome. This feature has been utilised for numerous applications in cancer therapeutics, including in diagnostics and also as a direct drug delivery system straight to the tumor. However, less than 1% of the NPs actually reach the tumor, with the majority remaining in circulation, again causing an onset of additional side effects alongside those of the drug itself (Chua et al., 2020). Most commonly, various different NPs are incorporated into a scaffold or gel. These are then employed as post-surgical treatments where they are implanted into the tumor resection site (Qiu et al., 2013). A particularly exciting avenue of cancer treatment investigates the practicality of structurally altering a particular drug (to form a pro-drug), in order to render it inactive, whereupon it is activated when in close contact with a catalyst such as gold or palladium. This allows for specific placing of catalyst “beads” in the precise area of treatment, and the systemic administration of the pro-drug, without the commonly associated side-effects (Adam et al., 2018). This approach has been demonstrated as a practical and efficient approach for chemotherapy delivery (Sancho-albero et al., 2019; Pérez-López et al., 2020; Rubio-Ruiz et al., 2021; Adam et al., 2022). More recently, the approach has also been used to deliver a neuroactive agent into the central nervous system, thus demonstrating its potential for wider ranging applications involved in cancer treatment and recovery (Ortega-Liebana et al., 2022).
In addition to the three aforementioned treatments, immunotherapy is another modality which targets the TME and acts by activating or suppressing the patient’s immune responses through modulating their immune system. Whilst it is not as well-established as the other treatments mentioned in this review, primarily due to its inconsistent efficacy, immunotherapy methods have become increasingly popular avenues for exploration and have even had some clinical success (Bates et al., 2018; Bhattacharya et al., 2020). This includes techniques such as reprogramming of immune cells and checkpoint inhibition. Checkpoint inhibition therapy uses immune checkpoint inhibitors to block the pathways which control immune response and block signalling responses, which under normal conditions can help cancer cells avoid cell death (Brahmer et al., 2018). In tissue engineering approaches, immunotherapy methods are often used in combination with other approaches such as scaffolds or hydrogels, and also in combination with other agents such as chemotherapeutics. The challenges and countermeasures of immunotherapy are similar to those for techniques such as chemotherapy, however, these combination approaches are advantageous in that they are able to be specifically designed, enabling them to counteract known side effects associated with treatment types. For example, whilst immune checkpoint blockade has been shown to have a marked effect in treating against some cancers, it is known to cause adverse side effects in healthy organs. However, these effects were seen to a lesser extent in patients who had also received chemotherapy, improving therapeutic responses and reducing side effects such as chemoresistance. Therefore, studies have looked into ways in which chemotherapy and immunotherapy can be combined, for example, in a hydrogel, in order to get the best possible outcome (Wang C. et al., 2018). Importantly, once a course of radiotherapy has been completed, immune cells play an important role in clearing any remaining cancer cells if the irradiated tumor equilibrium is correctly modulated (Koukourakis and Giatromanolaki, 2020). One particular example that utilises this combination approach is discussed in a paper from Mei et al., where they developed a hybrid hydrogel to be used for photothermal therapy (PTT) and immunotherapy (Mei et al., 2019). In this case, the gel encapsulated both a photothermal drug and an immunological agent. Overall, the gel was found to be capable of delivering the therapeutics in a localised and prolonged manner. Excitingly the authors discovered that the initial PTT cleared the primary tumors whilst producing tumor-associated antigens which worked in combination with the immunotherapeutic to protect against recurrence and metastasis (Mei et al., 2019).
Modulating the microenvironment via cell therapy has been successfully performed to treat other non-cancer related injuries, in ways that could be translatable to treating the damaged microenvironment post-cancer treatment and/or to aid with the regeneration process following such damage. One interesting example of this comes from Li et al., using a hydrogel implant following surgery as a means of transplanting mesenchymal stem cells (MSC) (Li L. et al., 2019). Here, MSCs were used to induce regeneration of the central nervous spinal cord tissue in order to restore motor function in a rat following spinal cord injury, alongside magnesium oxide (MnO2) nanoparticles, which not only act to alleviate the oxidative microenvironment through regulation of ROS but also improve the viability of the implanted MSC (Li L. et al., 2019). This dual approach is an interesting concept with the ability to not only successfully mediate the harsh microenvironment, but also induce regenerative repair to the damaged site.
Another approach worthy of mention is immune cell therapy. Chimeric antigen receptor (CAR) T cells are one example of an immunotherapeutic approach, with increasing popularity. Since the first CAR T cell treatment was approved by the FDA in 2017, there have been five further approved treatments to date (Hamieh et al., 2023). CAR T cells specifically target tumor associated, or tumor-specific antigens to selectively kill the cancer (target) cells (Johnson et al., 2022). This property can allow for TME mediation by, for example, eliminating tumor associated macrophages which in turn delays tumor progression and prolonging survival (Rodriguez-Garcia et al., 2021). For example, in a study by O’Conner et al, it was found that administration of T-cell infusion post-chemotherapy treatment led to improved tumor-free survival (O’Connor et al., 2012). Such results suggest that the combination of chemotherapy with T-cell infusion can modify the TME in such a manner to enhance anti-tumor effects. It is also important to note that administration of CAR T cells pre-treatment can also be used to condition the TME in such a manner to improve the effectiveness of treatment (Rodriguez-Garcia et al., 2021). The ability to engineer CAR T cells, which includes the ability to remove them from the patient and modify them ex-vivo to give personalised treatment, is discussed in detail in numerous reviews (Li D. et al., 2019; Hamieh et al., 2023). Whilst immunotherapy shows great promise, and can result in longer survival post-treatment, the side effects can be extreme–for example, kidney failure. It is also known to be most effective in advanced cancers, particularly where the number of genetic mutations is high. Between this and only limited success on various phase 3 trials, only a minority of patients are currently selected to receive such treatments. This is discussed in more detail by Semstein et al. (Samstein et al., 2019).
In addition to the aforementioned emerging techniques, there are a number of other interesting techniques that are being introduced to target the TME post-cancer. One particularly exciting avenue is that of exosome-based approaches. Exosomes are secreted by cancer cells and have a profound effect on the TME, impacting both environmental conditions and inflammatory and immune reactions (Jin et al., 2022). Naturally used to encapsulate substances such as proteins and nucleic acids and transport them to target cells, this property of exosomes has been exploited to be used as a drug delivery system, with advantages such as improved biocompatibility and stability, improved targeting, and reduced toxicity. A review by Koh et al. discusses the future direction of exosome-based approaches; their transition into a clinical setting and their advantages and limitations (Koh et al., 2023).
Whilst such interventions have proven to be relatively successful in vitro, and in some cases in vivo, there are still major difficulties in translating these tissue engineering approaches from the laboratory to human clinical trials. Issues arise with biocompatibility and the inability to accurately mimic the complex and patient-distinctive forms of cancer and its microenvironment in one animal model.
In spite of the issues in translating results from lab to clinic, there are some studies which have shown promise (Table 6). One method that successfully completed clinical trials is Lipsod (Delanian et al., 1994). The concept of this study is that liposomal Cu/Zn superoxide dismutase (Lipsod) is an antioxidant and anti-inflammatory agent that has the potential to reduce radiation-induced fibrosis of the skin and underlying tissue. The drug was administered via intermuscular injection twice a week for a total of 3 weeks, and a reduction in fibrotic tissue was seen in all patients. However, despite the initial success of the clinical trial, there are still several unanswered questions regarding the Lipsod treatment with respect to its mechanism of action.
Table 6. Clinical Trials using tissue engineering approaches. A combination of data collected from research papers accessed using PubMed and from clinicaltrials.gov (clinical trial ID number (NCT#) provided if known).
As previously mentioned, hypoxia is heavily involved in the reduced efficacy of both chemotherapy and radiotherapy. Hypoxic cells have a limited lifespan but exist in equilibrium with their proliferation and migration in the TME matching the rate of cell death in hypoxic regions. Radiotherapy creates free ROS radicals, a mechanism reliant on the presence of oxygen, to exert its toxic effect, meaning that hypoxic cells are resistant to it; and chemotherapy drugs fail to reach tumor cells that are in these hypoxic areas due to poor blood perfusion (Saggar and Tannock, 2015; Graham and Unger, 2018). Both treatments are delivered as multiple individual doses to provide as much protection for healthy cells as possible and to allow for components such as bone marrow to repopulate and repair. However, this simultaneously allows time for hypoxic cells to reoxygenate and any remaining tumor cells to proliferate, reducing the clinical outcome of both treatments (Saggar and Tannock, 2015). Hypoxia-activated pro-drugs (HAPs) have been a research focus for several decades. More recently, TH-302 was used to deliver a cytotoxic warhead to the hypoxic region of the tumor without inducing significant systemic toxicity (Liu et al., 2012). TH-302 research had been both extensive and promising, with the treatment reaching clinic but failing in the stage three trials (Li et al., 2021). Despite this, researchers are still studying the effects of TH-302 as a promising therapeutic option.
Whilst there are a limited number of treatments/therapeutics in clinical trial stages specifically for post-cancer treatments, the techniques discussed in this review have been successful in other applications. There is potential crossover and application in the repair and/or regeneration of the damaged microenvironment post cancer treatment, where the approach contains certain components that is capable of triggering certain responses which can create a microenvironment favorable for regeneration. One application in which extensive research has looked at using both electrospun fiber mat and hydrogel scaffolds is wound repair and skin regeneration (Zahid et al., 2019). Such scaffolds are used in multiple applications from surgical wounds to major burn victims (Dimatteo et al., 2018; Asiri et al., 2021). Another common application is following surgery for spinal cord injury, where there have been a range of techniques applied in this field to aid repair. The first use of a bioresorbable polymer scaffold for human spinal cord injury was administered to a patient following a spinal fracture and was directly implanted into the spinal cord (Theodore et al., 2016). During the period of study, improvement to the injury was observed, along with no complications or issues arising from the implanted scaffold.
Some of the most common tissue engineering approaches that reach clinical trial stages cannot be easily applied to cancer settings. For example, decellularized ECM (dECM) is commonly incorporated into biomaterials and used for tissue regeneration, however challenges arise when using dECM for cancer research as the ECM can differ significantly in terms of composition and microstructure between individual patients (Hoshiba, 2019).
Based on the focus of the current emerging technologies and those currently in clinical trial, it is clear that the concept of using biomaterials, predominantly hydrogels, to deliver local chemotherapy directly to the target site following surgical resection is where the majority of research is currently focused. This is most likely due to ease of administration via the surgical site without inducing any further stress on the body. Future work into how such therapeutics can be translated for alternative approaches to the delivery of treatments such as chemotherapy and irradiation therapy will further improve the efficacy of cancer treatments whilst reducing systemic side effects.
The cancer death rate has fallen consistently since its peak in 1991, reaching a total decline of 31% in 2018; and this is partially due to improvements in terms of treatment, as well as other factors including better awareness and improved health and lifestyle choices (Siegel et al., 2021). Whilst this reflects the significant improvement in treating cancer, this subsequently means that there are more patients suffering from the, in some cases debilitating, long-term effects caused by damage to the microenvironment during treatment. This highlights the need for mediation treatments capable of restoring the microenvironment with subsequent potential regeneration and restoration of tissue function, improving the patient’s quality of life post-cancer remission.
Whilst there are limited options available to patients to alleviate some of the long-term side effects caused by cancer therapeutics, these are often medication-dependent approaches that, with long term use, will eventually lead to damage of other organs, such as the kidneys and liver (Ricart, 2017; Perazella and Shirali, 2018). Tissue engineering approaches offer a fresh alternative to the current route of heavy medication and lifelong dependence.
Each of the current treatment approaches have their positive and negative attributes in terms of the impact that they have on the microenvironment, but on top of this, there are considerations including the location and accessibility of the TME which all play a role in how the damaged microenvironment can be reversed. As discussed, ways to alleviate this include biomaterials and tissue engineering, in addition to new therapies. Based on the location and accessibility of the tumor, and the initial treatment given, this leads on to possible post-cancer treatments in the microenvironment, with the ultimate goal for post-cancer treatment being the development of a method in which the damaged microenvironment can be mediated following initial treatment, with the conclusive aim of initiating the regeneration process.
In terms of biomaterials used, some trends can be seen. Stem cell therapy has been typically used alone in post-chemotherapy methods of remediation, whereas when used following radiotherapy and surgery, stem cell therapy has been combined with a polymer material in either an electrospun scaffold or within a hydrogel system. Hydrogels are more commonly used for surgery, but within this, different administration routes were used, ranging from implants to injections to sprays. The uses and forms of the most common biomaterials encountered during this review have been summarised in Table 7. The most popular biomaterial used in the selection of studies discussed within this review is PLGA, whether that be alone or in combination with other polymers and biomaterials. Approved for clinical use by the US FDA, PLGA is a popular choice for biomaterials for many reasons, including its biocompatibility and degradation properties, which can be tailored based on its molecular weight and, if used in combination with another polymer, its copolymer ratio (Gentile et al., 2014; Patel et al., 2021). It also has the added beneficial properties of favourable drug encapsulation and sustained drug release (Patel et al., 2021). This follows true for the other popular polymers used, such as polycaprolactone (PCL) and polyethylene glycol (PEG). PLGA has great potential as a biomaterial in particular as research has found that PLGA coatings have shown the capability to tune properties such as biocompatibility and degradability, but also mechanical characteristics such as tensile and compressive properties (Maadani et al., 2023). This is key in providing an environment that can mimic that of the natural tissue, including the ability to support the survival and proliferation of cells. For post-surgical repair methods, inclusion of a drug compound, particularly anticancer drugs such as paclitaxel, is also common. There are several additional reasons that polymer-based drug delivery systems are increasing in popularity, some of which include non-immunogenicity, long blood circulation time and high drug loading capacity (Fazal et al., 2023). Such properties are highly beneficial when it comes to designing a drug delivery system, as this would overcome several of the commonly known issues that hinder the efficacy of drug treatments. For further detail, the review by Khan et al. provides an extensive and detailed exploration of the current realm of polymer based biomaterials in tissue engineering (Khan et al., 2023). Furthermore, these polymers can be utilised in different forms and tailored for specific uses. For example, as seen in the studies explored within this review, electrospun scaffolds can be adhered to the tissue itself through a simple stitching; whereas hydrogels can be designed to be injected, implanted, or sprayed topically.
Table 7. A summary of the most common biomaterials used post-radiotherapy and -surgical resection (≥3) and their form, taken from the data collected and provided in the Supplementary Material S1 and organized from most common to least.
The vast majority of emerging technology is still focused on the idea of containing any further development or recurrence of cancer rather than microenvironmental reversal and what can be done to improve quality of life post-cancer. Whilst this current area of research is of high importance, the significant increase in the number of cancer survivors means that the need for more than palliative-level treatment for the long-term damaged caused by cancer treatment is now greater than ever. There is still a major lack of investigation into whether the microenvironment can be mediated and returned to its normal state of homeostasis, with the potential of promoting regeneration and return of function to damaged tissue. Additionally, in order to understand the complex relationship between cancer cells and the TME, more research needs to focus on the cancer cells themselves, including looking at patient-derived multi-cell populations which, due to the heterogeneity of cancers, would be necessary to obtain more clinically relevant data. Additionally, as this area is still in its infancy in terms of developing post-cancer treatments, future reviews providing insight into mechanistic action associated with the biomaterials will be important to consider once these technologies have matured further.
Whilst there is still considerable research required with regards to the effects that current cancer treatments have on the TME, it is clear that they do impact said microenvironment in a negative manner and finding a way to combat this issue has the potential to not only improve the efficacy of treatment, but also reduce associated side effects. Tissue engineering approaches have shown promise in the laboratory setting, however translating these results into human clinical studies will be the next major breakthrough. Whilst all have been considered individually, the findings within this review indicate that a treatment option that can not only suppress the cancer but also, whether it be simultaneously or subsequently, neutralize the microenvironment and promote regeneration of healthy tissue is highly desirable and could benefit the lives of patients post-cancer remission.
LW: Writing–review and editing, Writing–original draft, Investigation, Conceptualization. IN: Writing–review and editing. EE: Writing–review and editing, Investigation. AC: Writing–review and editing, Conceptualization.
The author(s) declare that financial support was received for the research, authorship, and/or publication of this article. This study was supported by the Engineering and Physical Sciences Research Council grant EP/T517884/1.
The authors declare that the research was conducted in the absence of any commercial or financial relationships that could be construed as a potential conflict of interest.
All claims expressed in this article are solely those of the authors and do not necessarily represent those of their affiliated organizations, or those of the publisher, the editors and the reviewers. Any product that may be evaluated in this article, or claim that may be made by its manufacturer, is not guaranteed or endorsed by the publisher.
The Supplementary Material for this article can be found online at: https://www.frontiersin.org/articles/10.3389/fbiom.2024.1347324/full#supplementary-material
Abatangelo, G., Vindigni, V., Avruscio, G., Pandis, L., and Brun, P. (2020). Hyaluronic acid: redefining its role. Cells 9, 1743–1819. doi:10.3390/cells9071743
Aboulkheyr Es, H., Montazeri, L., Aref, A. R., Vosough, M., and Baharvand, H. (2018). Personalized cancer medicine: an organoid approach. Trends Biotechnol. 36, 358–371. doi:10.1016/j.tibtech.2017.12.005
Adam, C., Bray, T. L., Pérez-López, A. M., Tan, E. H., Rubio-Ruiz, B., Baillache, D. J., et al. (2022). A 5-FU precursor designed to evade anabolic and catabolic drug pathways and activated by Pd chemistry in vitro and in vivo. J. Med. Chem. 65, 552–561. doi:10.1021/acs.jmedchem.1c01733
Adam, C., Pérez-López, A. M., Hamilton, L., Rubio-Ruiz, B., Bray, T. L., Sieger, D., et al. (2018). Bioorthogonal uncaging of the active metabolite of irinotecan by palladium-functionalized microdevices. Chem. - A Eur. J. 24, 16783–16790. doi:10.1002/chem.201803725
Aguado, B. A., Bushnell, G. G., Rao, S. S., Jeruss, J. S., and Shea, L. D. (2017). Engineering the pre-metastatic niche. Nat. Biomed. Eng. 1, 0077. doi:10.1038/s41551-017-0077
Aguado, B. A., Hartfield, R. M., Bushnell, G. G., Decker, J. T., Azarin, S. M., Nanavati, D., et al. (2018). Biomaterial scaffolds as pre-metastatic niche mimics systemically alter the primary tumor and tumor microenvironment. Adv. Healthc. Mat. 7, e1700903–e1700911. doi:10.1002/adhm.201700903
Ajmal, G., Bonde, G. V., Mittal, P., Khan, G., Pandey, V. K., Bakade, B. V., et al. (2019). Biomimetic PCL-gelatin based nanofibers loaded with ciprofloxacin hydrochloride and quercetin: a potential antibacterial and anti-oxidant dressing material for accelerated healing of a full thickness wound. Int. J. Pharm. 567, 118480. doi:10.1016/j.ijpharm.2019.118480
Alieva, M., van Rheenen, J., and Broekman, M. L. D. (2018). Potential impact of invasive surgical procedures on primary tumor growth and metastasis. Clin. Exp. Metastasis 35, 319–331. doi:10.1007/s10585-018-9896-8
Al Kayal, T., Losi, P., Pierozzi, S., and Soldani, G. (2020). A new method for fibrin-based electrospun/sprayed scaffold fabrication. Sci. Rep. 10, 5111. doi:10.1038/s41598-020-61933-z
Anisiei, A., Oancea, F., and Marin, L. (2023). Electrospinning of chitosan-based nanofibers: from design to prospective applications. Rev. Chem. Eng. 39, 31–70. doi:10.1515/revce-2021-0003
Antaby, E., Klinkhammer, K., and Sabantina, L. (2021). Electrospinning of chitosan for antibacterial applications—current trends. Appl. Sci. 11, 11937. doi:10.3390/app112411937
Asgharian, P., Tazehkand, A. P., Soofiyani, S. R., Hosseini, K., Martorell, M., Tarhriz, V., et al. (2021). Quercetin impact in pancreatic cancer: an overview on its therapeutic effects. Oxid. Med. Cell. Longev. 2021, 1–13. doi:10.1155/2021/4393266
Asiri, A., Saidin, S., Sani, M. H., and Al-Ashwal, R. H. (2021). Epidermal and fibroblast growth factors incorporated polyvinyl alcohol electrospun nanofibers as biological dressing scaffold. Sci. Rep. 11, 5634–5714. doi:10.1038/s41598-021-85149-x
Bagó, J. R., Pegna, G. J., Okolie, O., and Hingtgen, S. D. (2016). Fibrin matrices enhance the transplant and efficacy of cytotoxic stem cell therapy for post-surgical cancer. Biomaterials 84, 42–53. doi:10.1016/j.biomaterials.2016.01.007
Banshoya, K., Nakamura, T., Tanaka, T., and Kaneo, Y. (2021). Development of α-tocopherol nanomicellar formulation using polyethylene glycol monostearate for the oxidative stress-related disease. J. Drug Deliv. Sci. Technol. 61, 102310. doi:10.1016/j.jddst.2020.102310
Barazzuol, L., Coppes, R. P., and van Luijk, P. (2020). Prevention and treatment of radiotherapy-induced side effects. Mol. Oncol. 14, 1538–1554. doi:10.1002/1878-0261.12750
Barker, H. E., Paget, J. T. E., Khan, A. A., and Harrington, K. J. (2015). The tumour microenvironment after radiotherapy: mechanisms of resistance and recurrence. Nat. Rev. Cancer 15, 409–425. doi:10.1038/nrc3958
Baskar, R., Dai, J., Wenlong, N., Yeo, R., and Yeoh, K. W. (2014). Biological response of cancer cells to radiation treatment. Front. Mol. Biosci. 1, 24–29. doi:10.3389/fmolb.2014.00024
Bates, J. P., Derakhshandeh, R., Jones, L., and Webb, T. J. (2018). Mechanisms of immune evasion in breast cancer. BMC Cancer 18, 556–614. doi:10.1186/s12885-018-4441-3
Beikzadeh, S., Hosseini, S. M., Mofid, V., Ramezani, S., Ghorbani, M., Ehsani, A., et al. (2021). Electrospun ethyl cellulose/poly caprolactone/gelatin nanofibers: the investigation of mechanical, antioxidant, and antifungal properties for food packaging. Int. J. Biol. Macromol. 191, 457–464. doi:10.1016/j.ijbiomac.2021.09.065
Bhattacharya, S., Calar, K., Evans, C., Petrasko, M., and de la Puente, P. (2020). Bioengineering the oxygen-deprived tumor microenvironment within a three-dimensional platform for studying tumor-immune interactions. Front. Bioeng. Biotechnol. 8, 1040–1120. doi:10.3389/fbioe.2020.01040
Bilbao, M., Aikins, J. K., and Ostrovsky, O. (2021). Is routine omentectomy of grossly normal omentum helpful in surgery for ovarian cancer? A look at the tumor microenvironment and its clinical implications. Gynecol. Oncol. 161, 78–82. doi:10.1016/j.ygyno.2020.12.033
Blache, U., Stevens, M. M., and Gentleman, E. (2020). Harnessing the secreted extracellular matrix to engineer tissues. Nat. Biomed. Eng. 4, 357–363. doi:10.1038/s41551-019-0500-6
Blanquer, A., Kostakova, E. K., Filova, E., Lisnenko, M., Broz, A., Mullerova, J., et al. (2024). A novel bifunctional multilayered nanofibrous membrane combining polycaprolactone and poly (vinyl alcohol) enriched with platelet lysate for skin wound healing. Nanoscale 16, 1924–1941. doi:10.1039/d3nr04705a
Brahmer, J., Lacchetti, C., Atkins, M. B., Brassil, K. J., Chau, I., Ernstoff, M. S., et al. (2018). Management of immune-related adverse events in patients treated with immune checkpoint inhibitor therapy: American society of clinical oncology clinical practice guideline. J. Clin. Oncol. 36, 1714–1768. doi:10.1200/jco.2017.77.6385
Brudno, Y., Pezone, M. J., Snyder, T. K., Uzun, O., Moody, C. T., Aizenberg, M., et al. (2018). Replenishable drug depot to combat post-resection cancer recurrence. Biomaterials 178, 373–382. doi:10.1016/j.biomaterials.2018.05.005
Budi, H. S., and Farhood, B. (2023). Targeting oral tumor microenvironment for effective therapy. Cancer Cell Int. 23, 101–127. doi:10.1186/s12935-023-02943-5
Campbell, K. T., Hadley, D. J., Kukis, D. L., and Silva, E. A. (2017). Alginate hydrogels allow for bioactive and sustained release of VEGF-C and VEGF-D for lymphangiogenic therapeutic applications. PLoS One 12, 01814844–e181515. doi:10.1371/journal.pone.0181484
Castro, K. C., Campos, M. G. N., and Mei, L. H. I. (2021). Hyaluronic acid electrospinning: challenges, applications in wound dressings and new perspectives. Int. J. Biol. Macromol. 173, 251–266. doi:10.1016/j.ijbiomac.2021.01.100
Celebioglu, A., and Uyar, T. (2020). Design of polymer-free Vitamin-A acetate/cyclodextrin nanofibrous webs: antioxidant and fast-dissolving properties. Food Funct. 11, 7626–7637. doi:10.1039/d0fo01776k
Cha, S. W., Sohn, J. H., Kim, S. H., Kim, Y. T., Kang, S. H., Cho, M. Y., et al. (2020). Interaction between the tumor microenvironment and resection margin in different gross types of hepatocellular carcinoma. J. Gastroenterol. Hepatol. 35, 648–653. doi:10.1111/jgh.14848
Chandler, C., Liu, T., Buckanovich, R., and Coffman, L. (2019). The double edge sword of fibrosis in cancer. Transl. Res. 209, 55–67. doi:10.1016/j.trsl.2019.02.006
Chen, H., Shi, Y., Sun, L., and Ni, S. (2020). Electrospun composite nanofibers with all-trans retinoic acid and MWCNTs-OH against cancer stem cells. Life Sci. 258, 118152–118212. doi:10.1016/j.lfs.2020.118152
Chen, M., Feng, Z., Guo, W., Yang, D., Gao, S., Li, Y., et al. (2019a). PCL-MECM-Based hydrogel hybrid scaffolds and meniscal fibrochondrocytes promote whole meniscus regeneration in a rabbit meniscectomy model. ACS Appl. Mat. Interfaces 11, 41626–41639. doi:10.1021/acsami.9b13611
Chen, Q., Wang, C., Zhang, X., Chen, G., Hu, Q., Li, H., et al. (2019b). In situ sprayed bioresponsive immunotherapeutic gel for post-surgical cancer treatment. Nat. Nanotechnol. 14, 89–97. doi:10.1038/s41565-018-0319-4
Chen, X., Wang, Q., Li, X., Wang, Q., Xie, J., and Fu, X. (2018). Heat shock pretreatment of mesenchymal stem cells for inhibiting the apoptosis of ovarian granulosa cells enhanced the repair effect on chemotherapy-induced premature ovarian failure. Stem Cell Res. Ther. 9, 240–311. doi:10.1186/s13287-018-0964-4
Chen, Z., Zhang, P., Xu, Y., Yan, J., Liu, Z., Lau, W. B., et al. (2019c). Surgical stress and cancer progression: the twisted tango. Mol. Cancer 18, 132–211. doi:10.1186/s12943-019-1058-3
Chhikara, B. S., and Parang, K. (2023). Global Cancer Statistics 2022: the trends projection analysis. Chem. Biol. Lett. 10, 1–16.
Cho, H., and Kwon, G. S. (2014). Thermosensitive poly-(d,l-lactide-co-glycolide)-block-poly(ethylene glycol)-block-poly-(d,l-lactide-co-glycolide) hydrogels for multi-drug delivery. J. Drug Target. 22, 669–677. doi:10.3109/1061186X.2014.931406
Chua, C. Y. X., Ho, J., Demaria, S., Ferrari, M., and Grattoni, A. (2020). Emerging technologies for local cancer treatment. Adv. Ther. 3, 2000027–2000118. doi:10.1002/adtp.202000027
ClinicalTrials.gov (2021a). Coronary microcirculatory and bioresorbable vascular scaffolds (EMPIRE-BVS). Available at: https://clinicaltrials.gov/ct2/show/NCT03076476?term=coronary+microcirculatory+and+bioresorbable+vascular+scaffolds&draw=2&rank=1 (Accessed September 28, 2021).
ClinicalTrials.gov (2021b). The effect of RadiaAce gel in the prevention and treatment of radiation dermatitis in breast cancer patients. Available at: https://clinicaltrials.gov/ct2/show/NCT04481802 (Accessed September 29, 2021).
ClinicalTrials.gov (2021c). Efficacy of MUCIPLIQ on the incidence of radio-chemotherapy-induced mucositis in patients suffering from oral cancer (MUCIPLIQ). Available at: https://clinicaltrials.gov/ct2/show/study/NCT01840436?term=tumor+environment+repair+and+regeneration&draw=2&rank=1 (Accessed September 28, 2021).
ClinicalTrials.gov (2021d). EktoTherix regenerative tissue scaffold for repair of surgical excision wounds. Available at: https://clinicaltrials.gov/ct2/show/study/NCT02409628?term=polymer+scaffolds&draw=3&rank=12 (Accessed September 28, 2021).
ClinicalTrials.gov (2021e). Impact of applying a bandage skin hydrogel on the pains of the head and neck in patients with cancer of head and neck treated with radiotherapy (IPAC). Available at: https://clinicaltrials.gov/ct2/show/NCT01520701 (Accessed August 29, 2021).
ClinicalTrials.gov (2021f). The inspire study: probable benefit of the neuro-spinal scaffold for treatment of ais A thoracic acute spinal cord injury. Available at: https://clinicaltrials.gov/ct2/show/study/NCT02138110?term=scaffold&cond=neuro-spinal&draw=2&rank=1 (Accessed September 28, 2021).
ClinicalTrials.gov (2021g). NeoVas bioresorbable coronary scaffold. Available at: https://clinicaltrials.gov/ct2/show/results/NCT02195414?term=polymer+scaffolds&draw=3&rank=1&view=results (Accessed September 28, 2021).
ClinicalTrials.gov (2021h). Phase I-ii clinical trial with autologous bone marrow derived mesenchymal stem cells for the therapy of multiple sclerosis. Available at: https://clinicaltrials.gov/ct2/show/NCT02035514 (Accessed September 29, 2021).
ClinicalTrials.gov (2021i). Safety and efficacy of doxorubicin-eluting-bead embolization in patients with advanced hepatocellular carcinoma. Available at: https://clinicaltrials.gov/ct2/show/NCT02525380 (Accessed September 29, 2021).
ClinicalTrials.gov (2021j). Use of stem cells cultured on a scaffold for the treatment of aneurysmal bone cysts (ABC). Available at: https://clinicaltrials.gov/ct2/show/NCT03066245?term=polymer+scaffolds&cond=cancer&draw=2&rank=2 (Accessed September 29, 2021).
Dalerba, P., Diehn, M., Weissman, I. L., and Clarke, M. F. (2020). Stem cells, cell differentiation, and cancer. Sixth edit. Elsevier Inc. doi:10.1016/b978-0-323-47674-4.00007-4
Delanian, S., Baillet, F., Huart, J., Lefaix, J. L., Maulard, C., and Housset, M. (1994). Successful treatment of radiation-induced fibrosis using liposomal superoxide dismutase: clinical trial. Radiother. Oncol. 32, 12–20. doi:10.1016/0167-8140(94)90444-8
Demaria, M., O’Leary, M. N., Chang, J., Shao, L., Liu, S., Alimirah, F., et al. (2017). Cellular senescence promotes adverse effects of chemotherapy and cancer relapse. Cancer Discov. 7, 165–176. doi:10.1158/2159-8290.CD-16-0241
Deng, H., Dong, A., Song, J., and Chen, X. (2019). Injectable thermosensitive hydrogel systems based on functional PEG/PCL block polymer for local drug delivery. J. Control. Release 297, 60–70. doi:10.1016/j.jconrel.2019.01.026
Dimatteo, R., Darling, N. J., and Segura, T. (2018). In situ forming injectable hydrogels for drug delivery and wound repair. Adv. Drug Deliv. Rev. 127, 167–184. doi:10.1016/j.addr.2018.03.007
Ding, Y. W., Zhang, X. W., Mi, C. H., Qi, X. Y., Zhou, J., and Wei, D. X. (2023). Recent advances in hyaluronic acid-based hydrogels for 3D bioprinting in tissue engineering applications. Smart Mat. Med. 4, 59–68. doi:10.1016/j.smaim.2022.07.003
Dondossola, E., Holzapfel, B. M., Alexander, S., Filippini, S., Hutmacher, D. W., and Friedl, P. (2017). Examination of the foreign body response to biomaterials by nonlinear intravital microscopy. Nat. Biomed. Eng. 1, 0007–0010. doi:10.1038/s41551-016-0007
Dong, J., Sakai, K., Koma, Y., Watanabe, J., Liu, K., Maruyama, H., et al. (2021). Dental pulp stem cell-derived small extracellular vesicle in irradiation-induced senescence. Biochem. Biophys. Res. Commun. 575, 28–35. doi:10.1016/j.bbrc.2021.08.046
Duan, H., Liu, Y., Gao, Z., and Huang, W. (2021). Recent advances in drug delivery systems for targeting cancer stem cells. Acta Pharm. Sin. B 11, 55–70. doi:10.1016/j.apsb.2020.09.016
Elder, S. S., and Emmerson, E. (2020). Senescent cells and macrophages: key players for regeneration? senescent cells and macrophages. Open Biol. 10, 200309. doi:10.1098/rsob.200309
Fane, M., and Weeraratna, A. (2020). How the ageing microenvironment influences tumour progression. Nat. Rev. Cancer 20, 89–106. doi:10.1038/s41568-019-0222-9
Farhood, B., Najafi, M., and Mortezaee, K. (2019). Cancer-associated fibroblasts: secretions, interactions, and therapy. J. Cell. Biochem. 120, 2791–2800. doi:10.1002/jcb.27703
Fazal, T., Murtaza, B. N., Shah, M., Iqbal, S., Rehman, M. U., Jaber, F., et al. (2023). Recent developments in natural biopolymer based drug delivery systems. RSC Adv. 13, 23087–23121. doi:10.1039/d3ra03369d
Feng, Y., Zhang, Z., Tang, W., and Dai, Y. (2023). Gel/hydrogel-based in situ biomaterial platforms for cancer postoperative treatment and recovery. Exploration 3, 20220173. doi:10.1002/EXP.20220173
Fernando, K., Kwang, L. G., Lim, J. T. C., and Fong, E. L. S. (2021). Hydrogels to engineer tumor microenvironments: in vitro. Biomater. Sci. 9, 2362–2383. doi:10.1039/d0bm01943g
Fontanella, A. N., Boss, M. K., Hadsell, M., Zhang, J., Schroeder, T., Berman, K. G., et al. (2015). Effects of high-dose microbeam irradiation on tumor microvascular function and angiogenesis. Radiat. Res. 183, 147–158. doi:10.1667/RR13712.1
Forget, P., Simonet, O., and De Kock, M. (2013). Cancer surgery induces inflammation, immunosuppression and neo-angiogenesis, but is it influenced by analgesics? F1000Research 2, 102. doi:10.12688/f1000research.2-102.v1
Gao, Q., Zhou, G., Lin, S.-J., Paus, R., and Yue, Z. (2018). How chemotherapy and radiotherapy damage the tissue: comparative biology lessons from feather and hair models. Exp. Dermatol. 28, 413–418. doi:10.1111/exd.13846
Gentile, P., Chiono, V., Carmagnola, I., and Hatton, P. V. (2014). An overview of poly(lactic-co-glycolic) Acid (PLGA)-based biomaterials for bone tissue engineering. Int. J. Mol. Sci. 15, 3640–3659. doi:10.3390/ijms15033640
Gigliobianco, G., Chong, C. K., and MacNeil, S. (2015). Simple surface coating of electrospun poly-L-lactic acid scaffolds to induce angiogenesis. J. Biomater. Appl. 30, 50–60. doi:10.1177/0885328215569891
Giussani, M., Merlino, G., Cappelletti, V., Tagliabue, E., and Daidone, M. G. (2015). Tumor-extracellular matrix interactions: identification of tools associated with breast cancer progression. Semin. Cancer Biol. 35, 3–10. doi:10.1016/j.semcancer.2015.09.012
Goossens, E., Jahnukainen, K., Mitchell, R., van Pelt, A., Pennings, G., Rives, N., et al. (2020). Fertility preservation in boys: recent developments and new insights. Hum. Reprod. Open 2020, hoaa016–18. doi:10.1093/hropen/hoaa016
Graham, K., and Unger, E. (2018). Overcoming tumor hypoxia as a barrier to radiotherapy, chemotherapy and immunotherapy in cancer treatment. Int. J. Nanomedicine 13, 6049–6058. doi:10.2147/IJN.S140462
Greten, F. R., and Grivennikov, S. I. (2019). Inflammation and cancer: triggers, mechanisms, and consequences. Immu 51, 27–41. doi:10.1016/j.immuni.2019.06.025
Gugenheim, J., Crovetto, A., and Petrucciani, N. (2022). Neoadjuvant therapy for pancreatic cancer. Updat. Surg. 74, 35–42. doi:10.1007/s13304-021-01186-1
Hamieh, M., Mansilla-Soto, J., Rivière, I., and Sadelain, M. (2023). Programming CAR T cell tumor recognition: tuned antigen sensing and logic gating. Cancer Discov. 13, 829–843. doi:10.1158/2159-8290.CD-23-0101
Han, X., Zhao, M., Xu, R., Zou, Y., Wang, Y., Liang, J., et al. (2023). Electrospun hyaluronan nanofiber membrane immobilizing aromatic doxorubicin as therapeutic and regenerative biomaterial. Int. J. Mol. Sci. 24, 7023. doi:10.3390/ijms24087023
Hanahan, D., and Weinberg, R. A. (2000). The hallmarks of cancer. Cell Press 100, 57–70. doi:10.1016/s0092-8674(00)81683-9
Hanahan, D., and Weinberg, R. A. (2011). Hallmarks of cancer: the next generation. Cell 144, 646–674. doi:10.1016/j.cell.2011.02.013
Hass, R. (2020). Role of msc in the tumor microenvironment. Cancers (Basel) 12, 2107–2117. doi:10.3390/cancers12082107
Hassanpour, S. H., and Dehghani, M. (2017). Review of cancer from perspective of molecular. J. Cancer Res. Pract. 4, 127–129. doi:10.1016/j.jcrpr.2017.07.001
Hernández-Rangel, A., and Martin-Martinez, E. S. (2021). Collagen based electrospun materials for skin wounds treatment. J. Biomed. Mat. Res. - Part A 109, 1751–1764. doi:10.1002/jbm.a.37154
Hinderer, S., Layland, S. L., and Schenke-Layland, K. (2016). ECM and ECM-like materials - biomaterials for applications in regenerative medicine and cancer therapy. Adv. Drug Deliv. Rev. 97, 260–269. doi:10.1016/j.addr.2015.11.019
Hirata, E., and Sahai, E. (2017). Tumor microenvironment and differential responses to therapy. Cold Spring Harb. Perspect. Med. 7, 0267811–a26814. doi:10.1101/cshperspect.a026781
Holmberg, K. V., and Hoffman, M. P. (2014). Anatomy, biogenesis and regeneration of salivary glands. Monogr. Oral Sci. 24, 1–13. doi:10.1159/000358776
Hoshiba, T. (2019). Decellularized extracellular matrix for cancer research. Mater. (Basel) 12, 1311–1327. doi:10.3390/ma12081311
Hu, Q., Li, H., Archibong, E., Chen, Q., Ruan, H., Ahn, S., et al. (2021). Inhibition of post-surgery tumour recurrence via a hydrogel releasing CAR-T cells and anti-PDL1-conjugated platelets. Nat. Biomed. Eng. 5, 1038–1047. doi:10.1038/s41551-021-00712-1
Jain, S., Meka, S. R. K., and Chatterjee, K. (2016). Engineering a piperine eluting nanofibrous patch for cancer treatment. ACS Biomater. Sci. Eng. 2, 1376–1385. doi:10.1021/acsbiomaterials.6b00297
Jin, M. Z., and Jin, W. L. (2020). The updated landscape of tumor microenvironment and drug repurposing. Signal Transduct. Target. Ther. 5, 166. doi:10.1038/s41392-020-00280-x
Jin, Y., Xing, J., Xu, K., Liu, D., and Zhuo, Y. (2022). Exosomes in the tumor microenvironment: promoting cancer progression. Front. Immunol. 13, 1025218. doi:10.3389/fimmu.2022.1025218
Johnson, A., Townsend, M., and O’Neill, K. (2022). Tumor microenvironment immunosuppression: a roadblock to CAR T-cell advancement in solid tumors. Cells 11, 3626. doi:10.3390/cells11223626
Joshi, R. S., Kanugula, S. S., Sudhir, S., Pereira, M. P., Jain, S., and Aghi, M. K. (2021). The role of cancer-associated fibroblasts in tumor progression. Cancers (Basel) 13, 1399–1427. doi:10.3390/cancers13061399
Kaigler, D., Wang, Z., Horger, K., Mooney, D. J., and Krebsbach, P. H. (2006). VEGF scaffolds enhance angiogenesis and bone regeneration in irradiated osseous defects. J. Bone Min. Res. 21, 735–744. doi:10.1359/jbmr.060120
Kalantary, S., Golbabaei, F., Latifi, M., Shokrgozar, M. A., and Yaseri, M. (2020). Assessment of electrospinning antioxidant nanofibers in skin exposure to oxidative stress. J. Maz. Univ. Med. Sci. 30, 68–79.
Karsh, L. I., Gross, E. T., Pieczonka, C. M., Aliotta, P. J., Skomra, C. J., Ponsky, L. E., et al. (2018). Absorbable hydrogel spacer use in prostate radiotherapy: a comprehensive review of phase 3 clinical trial published data. Urology 115, 39–44. doi:10.1016/j.urology.2017.11.016
Kemp, J. A., Shim, M. S., Heo, C. Y., and Kwon, Y. J. (2016). “Combo” nanomedicine: Co-delivery of multi-modal therapeutics for efficient, targeted, and safe cancer therapy. Adv. Drug Deliv. Rev. 98, 3–18. doi:10.1016/j.addr.2015.10.019
Khalf, A., and Madihally, S. V. (2017). Modeling the permeability of multiaxial electrospun poly(ε-caprolactone)-gelatin hybrid fibers for controlled doxycycline release. Mat. Sci. Eng. C 76, 161–170. doi:10.1016/j.msec.2017.03.093
Khan, M. U. A., Aslam, M. A., Bin Abdullah, M. F., Hasan, A., Shah, S. A., and Stojanović, G. M. (2023). Recent perspective of polymeric biomaterial in tissue engineering– a review. Mat. Today Chem. 34, 101818. doi:10.1016/j.mtchem.2023.101818
Kim, H. Y., Park, J. H., Kim, M. J., Lee, J. H., Oh, S. H., and Byun, J. H. (2021). The effects of VEGF-centered biomimetic delivery of growth factors on bone regeneration. Biomater. Sci. 9, 3675–3691. doi:10.1039/d1bm00245g
Kim, K., Luu, Y. K., Chang, C., Fang, D., Hsiao, B. S., Chu, B., et al. (2004). Incorporation and controlled release of a hydrophilic antibiotic using poly(lactide-co-glycolide)-based electrospun nanofibrous scaffolds. J. Control. Release 98, 47–56. doi:10.1016/j.jconrel.2004.04.009
Kim, W., Lee, S., Seo, D., Kim, D., Kim, K., Kim, E. G., et al. (2019). Cellular stress responses in radiotherapy. Cells 8, 1105–1118. doi:10.3390/cells8091105
Kinoshita, T., and Goto, T. (2021). Links between inflammation and postoperative cancer recurrence. J. Clin. Med. 10, 228–318. doi:10.3390/jcm10020228
Koch, F., Thaden, O., Conrad, S., Tröndle, K., Finkenzeller, G., Zengerle, R., et al. (2022). Mechanical properties of polycaprolactone (PCL) scaffolds for hybrid 3D-bioprinting with alginate-gelatin hydrogel. J. Mech. Behav. Biomed. Mat. 130, 105219. doi:10.1016/j.jmbbm.2022.105219
Koh, H. B., Kim, H. J., Kang, S. W., and Yoo, T. H. (2023). Exosome-based drug delivery: translation from bench to clinic. Pharmaceutics 15, 2042. doi:10.3390/pharmaceutics15082042
Kolesnik, I., Tverdokhlebova, T., Danilenko, N., Plotnikov, E., Kulbakin, D., Zheravin, A., et al. (2021). Characterization and determination of the biocompatibility of porous polytetrafluoroethylene membranes fabricated via electrospinning. J. Fluor. Chem. 246, 109798. doi:10.1016/j.jfluchem.2021.109798
Koukourakis, M. I., and Giatromanolaki, A. (2020). Tumor microenvironment, immune response and post-radiotherapy tumor clearance. Clin. Transl. Oncol. 22, 2196–2205. doi:10.1007/s12094-020-02378-8
Kruyt, F. A. E. (2023). Cancer stem cells and cellular plasticity: a preface to the special issue “Advances in understanding cancer stem cell biology and perspectives for targeted therapy.”. Biochem. Pharmacol. 214, 115670. doi:10.1016/j.bcp.2023.115670
Landskron, G., De La Fuente, M., Thuwajit, P., Thuwajit, C., and Hermoso, M. A. (2014). Chronic inflammation and cytokines in the tumor microenvironment. J. Immunol. Res. 2014, 1–19. doi:10.1155/2014/149185
Lara, P. C., López-Peñalver, J. J., Farias, V. de A., Ruiz-Ruiz, M. C., Oliver, F. J., and Ruiz de Almodóvar, J. M. (2015). Direct and bystander radiation effects: a biophysical model and clinical perspectives. Cancer Lett. 356, 5–16. doi:10.1016/j.canlet.2013.09.006
Li, D., Li, X., Zhou, W. L., Huang, Y., Liang, X., Jiang, L., et al. (2019a). Genetically engineered t cells for cancer immunotherapy. Signal Transduct. Target. Ther. 4, 35. doi:10.1038/s41392-019-0070-9
Li, L., Xiao, B., Mu, J., Zhang, Y., Zhang, C., Cao, H., et al. (2019b). A MnO2 nanoparticle-dotted hydrogel promotes spinal cord repair via regulating reactive oxygen species microenvironment and synergizing with mesenchymal stem cells. ACS Nano 13, 14283–14293. doi:10.1021/acsnano.9b07598
Li, M., Li, M., Yin, T., Shi, H., Wen, Y., Zhang, B., et al. (2016). Targeting of cancer-associated fibroblasts enhances the efficacy of cancer chemotherapy by regulating the tumor microenvironment. Mol. Med. Rep. 13, 2476–2484. doi:10.3892/mmr.2016.4868
Li, M., Zhang, F., Su, Y., Zhou, J., and Wang, W. (2018). Nanoparticles designed to regulate tumor microenvironment for cancer therapy. Life Sci. 201, 37–44. doi:10.1016/j.lfs.2018.03.044
Li, Y., Ni, K., Chan, C., Guo, N., Luo, T., Han, W., et al. (2022). Dimethylaminomicheliolide sensitizes cancer cells to radiotherapy for synergistic combination with immune checkpoint blockade. Adv. Ther. 5, 2100160–2100169. doi:10.1002/adtp.202100160
Li, Y., Zhao, L., and Li, X. F. (2021). The hypoxia-activated prodrug TH-302: exploiting hypoxia in cancer therapy. Front. Pharmacol. 12, 636892–636899. doi:10.3389/fphar.2021.636892
Liu, C., Wang, Z., Wei, X., Chen, B., and Luo, Y. (2021). 3D printed hydrogel/PCL core/shell fiber scaffolds with NIR-triggered drug release for cancer therapy and wound healing. Acta Biomater. 131, 314–325. doi:10.1016/j.actbio.2021.07.011
Liu, Q., Sun, J. D., Wang, J., Ahluwalia, D., Baker, A. F., Cranmer, L. D., et al. (2012). TH-302, a hypoxia-activated prodrug with broad in vivo preclinical combination therapy efficacy: optimization of dosing regimens and schedules. Cancer Chemother. Pharmacol. 69, 1487–1498. doi:10.1007/s00280-012-1852-8
Liu, T., Zhou, L., Li, D., Andl, T., and Zhang, Y. (2019). Cancer-associated fibroblasts build and secure the tumor microenvironment. Front. Cell Dev. Biol. 7, 60. doi:10.3389/fcell.2019.00060
Liu, X., Zhang, H., Cheng, R., Gu, Y., Yin, Y., Sun, Z., et al. (2018). An immunological electrospun scaffold for tumor cell killing and healthy tissue regeneration. Mat. Horizons 5, 1082–1091. doi:10.1039/c8mh00704g
Lombaert, I., Movahednia, M. M., Adine, C., and Ferreira, J. N. (2017). Concise review: salivary gland regeneration: therapeutic approaches from stem cells to tissue organoids. Stem Cells 35, 97–105. doi:10.1002/stem.2455
Lu, H., Butler, J. A., Britten, N. S., Venkatraman, P. D., and Rahatekar, S. S. (2021). Natural antimicrobial nano composite fibres manufactured from a combination of alginate and oregano essential oil. Nanomaterials 11, 2062. doi:10.3390/nano11082062
Maadani, A. M., Davoodian, F., and Salahinejad, E. (2023). Effects of PLGA coating on biological and mechanical behaviors of tissue engineering scaffolds. Prog. Org. Coatings 176, 107406. doi:10.1016/j.porgcoat.2023.107406
Majumder, S., Matin, M. A., Sharif, A., and Arafat, M. T. (2020). Electrospinning of antibacterial cellulose acetate/polyethylene glycol fiber with in situ reduced silver particles. J. Polym. Res. 27, 381. doi:10.1007/s10965-020-02356-2
Markowska, A., Antoszczak, M., Markowska, J., and Huczyński, A. (2023). Role of hyaluronic acid in selected malignant neoplasms in women. Biomedicines 11, 304. doi:10.3390/biomedicines11020304
Mei, E., Li, S., Song, J., Xing, R., Li, Z., and Yan, X. (2019). Self-assembling Collagen/Alginate hybrid hydrogels for combinatorial photothermal and immuno tumor therapy. Colloids Surfaces A Physicochem. Eng. Asp. 577, 570–575. doi:10.1016/j.colsurfa.2019.06.023
Mendes, B. B., Sousa, D. P., Conniot, J., and Conde, J. (2021). Nanomedicine-based strategies to target and modulate the tumor microenvironment. Trends Cancer 7, 847–862. doi:10.1016/j.trecan.2021.05.001
Micek, H. M., Visetsouk, M. R., Masters, K. S., and Kreeger, P. K. (2020). Engineering the extracellular matrix to model the evolving tumor microenvironment. iScience 23, 101742. doi:10.1016/j.isci.2020.101742
Mokhena, T. C., and Luyt, A. S. (2017). Electrospun alginate nanofibres impregnated with silver nanoparticles: preparation, morphology and antibacterial properties. Carbohydr. Polym. 165, 304–312. doi:10.1016/j.carbpol.2017.02.068
Montero, R. B., Vial, X., Nguyen, D. T., Farhand, S., Reardon, M., Pham, S. M., et al. (2012). BFGF-containing electrospun gelatin scaffolds with controlled nano-architectural features for directed angiogenesis. Acta Biomater. 8, 1778–1791. doi:10.1016/j.actbio.2011.12.008
Morgan, W. F., and Sowa, M. B. (2015). Non-targeted effects induced by ionizing radiation: mechanisms and potential impact on radiation induced health effects. Cancer Lett. 356, 17–21. doi:10.1016/j.canlet.2013.09.009
Moussa, L., Demarquay, C., Réthoré, G., Benadjaoud, M. A., Siñeriz, F., Pattapa, G., et al. (2019). Heparan sulfate mimetics: a new way to optimize therapeutic effects of hydrogel-embedded mesenchymal stromal cells in colonic radiation-induced damage. Sci. Rep. 9, 164–214. doi:10.1038/s41598-018-36631-6
Mughees, M., Sengupta, A., Khowal, S., and Wajid, S. (2021). Mechanism of tumour microenvironment in the progression and development of oral cancer. Mol. Biol. Rep. 48, 1773–1786. doi:10.1007/s11033-020-06054-6
Muz, B., de la Puente, P., Azab, F., and Azab, A. K. (2015). The role of hypoxia in cancer progression, angiogenesis, metastasis, and resistance to therapy. Hypoxia 3, 83–92. doi:10.2147/hp.s93413
Najafiasl, M., Osfouri, S., Azin, R., and Zaeri, S. (2020). Alginate-based electrospun core/shell nanofibers containing dexpanthenol: a good candidate for wound dressing. J. Drug Deliv. Sci. Technol. 57, 101708. doi:10.1016/j.jddst.2020.101708
Nelson, D. W., and Gilbert, R. J. (2021). Extracellular matrix-mimetic hydrogels for treating neural tissue injury: a focus on fibrin, hyaluronic acid, and elastin-like polypeptide hydrogels. Adv. Healthc. Mat. 10, e2101329. doi:10.1002/adhm.202101329
Nelson, J., Manzella, K., and Baker, O. J. (2013). Current cell models for bioengineering a salivary gland: a mini-review of emerging technologies. Oral Dis. 19, 236–244. doi:10.1111/j.1601-0825.2012.01958.x
O’Connor, C. M., Sheppard, S., Hartline, C. A., Huls, H., Johnson, M., Palla, S. L., et al. (2012). Adoptive T-cell therapy improves treatment of canine non-Hodgkin lymphoma post chemotherapy. Sci. Rep. 2, 249–312. doi:10.1038/srep00249
O’Meara, C. H., Nguyen, T. V., Jafri, Z., Boyer, M., Shonka, D. C., and Khachigian, L. M. (2023). Personalised medicine and the potential role of electrospinning for targeted immunotherapeutics in head and neck cancer. Nanomaterials 14, 6. doi:10.3390/nano14010006
Ortega-Liebana, M. C., Porter, N. J., Adam, C., Valero, T., Hamilton, L., Sieger, D., et al. (2022). Truly-biocompatible gold catalysis enables vivo-orthogonal intra-CNS release of anxiolytics. Angew. Chem. - Int. Ed. 61, e202111461–e202111465. doi:10.1002/anie.202111461
Ou, W., Stewart, S., White, A., Kwizera, E. A., Xu, J., Fang, Y., et al. (2023). In-situ cryo-immune engineering of tumor microenvironment with cold-responsive nanotechnology for cancer immunotherapy. Nat. Commun. 14, 392–420. doi:10.1038/s41467-023-36045-7
Parayath, N., Padmakumar, S., Nair, S. V., Menon, D., and Amiji, M. M. (2020). Strategies for targeting cancer immunotherapy through modulation of the tumor microenvironment. Regen. Eng. Transl. Med. 6, 29–49. doi:10.1007/s40883-019-00113-6
Patel, M., Jha, A., and Patel, R. (2021). Potential application of PLGA microsphere for tissue engineering. J. Polym. Res. 28, 214. doi:10.1007/s10965-021-02562-6
Peinado, H., Zhang, H., Matei, I. R., Costa-Silva, B., Hoshino, A., Rodrigues, G., et al. (2017). Pre-metastatic niches: organ-specific homes for metastases. Nat. Rev. Cancer 17, 302–317. doi:10.1038/nrc.2017.6
Perazella, M. A., and Shirali, A. C. (2018). Nephrotoxicity of cancer immunotherapies: past, present and future. J. Am. Soc. Nephrol. 29, 2039–2052. doi:10.1681/ASN.2018050488
Pereira, A. O., Lopes, I. M. I., Silva, T. R., Corrêa, T. Q., Paschoalin, R. T., Inada, N. M., et al. (2021). Bacterial photoinactivation using PLGA electrospun scaffolds. ACS Appl. Mat. Interfaces. 13, 31406–31417. doi:10.1021/acsami.1c02686
Pérez-López, A. M., Rubio-Ruiz, B., Valero, T., Contreras-Montoya, R., Álvarez De Cienfuegos, L., Sebastián, V., et al. (2020). Bioorthogonal uncaging of cytotoxic paclitaxel through Pd nanosheet-hydrogel frameworks. J. Med. Chem. 63, 9650–9659. doi:10.1021/acs.jmedchem.0c00781
Pinzón-García, A. D., Sinisterra, R., Cortes, M., Mesa, F., and Ramírez-Clavijo, S. (2021). Polycaprolactone nanofibers as an adjuvant strategy for Tamoxifen release and their cytotoxicity on breast cancer cells. PeerJ 9, 121244–e12221. doi:10.7717/peerj.12124
Prabaharan, M., Grailer, J. J., Pilla, S., Steeber, D. A., and Gong, S. (2009). Gold nanoparticles with a monolayer of doxorubicin-conjugated amphiphilic block copolymer for tumor-targeted drug delivery. Biomaterials 30, 6065–6075. doi:10.1016/j.biomaterials.2009.07.048
Predina, J., Eruslanov, E., Judy, B., Kapoor, V., Cheng, G., Wang, L. C., et al. (2013). Changes in the local tumor microenvironment in recurrent cancers may explain the failure of vaccines after surgery. Proc. Natl. Acad. Sci. U. S. A. 110, E415–E424. doi:10.1073/pnas.1211850110
Predina, J. D., Judy, B., Fridlender, Z. G., Aliperti, L. A., Madajewski, B., Kapoor, V., et al. (2012). A positive-margin resection model recreates the postsurgical tumor microenvironment and is a reliable model for adjuvant therapy evaluation. Cancer Biol. Ther. 13, 745–755. doi:10.4161/cbt.20557
Prieto-Callejero, B., Rivera, F., Fagundo-Rivera, J., Romero, A., Romero-Martín, M., Gómez-Salgado, J., et al. (2020). Relationship between chemotherapy-induced adverse reactions and health-related quality of life in patients with breast cancer. Med. Baltim. 99, e21695. doi:10.1097/MD.0000000000021695
Qiu, K., He, C., Feng, W., Wang, W., Zhou, X., Yin, Z., et al. (2013). Doxorubicin-loaded electrospun poly(l-lactic acid)/mesoporous silica nanoparticles composite nanofibers for potential postsurgical cancer treatment. J. Mat. Chem. B 1, 4601–4611. doi:10.1039/c3tb20636j
Ranganath, S. H., and Wang, C. H. (2008). Biodegradable microfiber implants delivering paclitaxel for post-surgical chemotherapy against malignant glioma. Biomaterials 29, 2996–3003. doi:10.1016/j.biomaterials.2008.04.002
Rao, A. D., Coquia, S., De Jong, R., Gourin, C., Page, B., Latronico, D., et al. (2018). Effects of biodegradable hydrogel spacer injection on contralateral submandibular gland sparing in radiotherapy for head and neck cancers. Radiother. Oncol. 126, 96–99. doi:10.1016/j.radonc.2017.09.017
Ren, X., Wang, N., Zhou, Y., Song, A., Jin, G., Li, Z., et al. (2021). An injectable hydrogel using an immunomodulating gelator for amplified tumor immunotherapy by blocking the arginase pathway. Acta Biomater. 124, 179–190. doi:10.1016/j.actbio.2021.01.041
Reyes-Avendaño, I., Reyes-Jiménez, E., González-García, K., Pérez-Figueroa, D. C., Baltiérrez-Hoyos, R., Tapia-Pastrana, G., et al. (2022). Quercetin regulates key components of the cellular microenvironment during early hepatocarcinogenesis. Antioxidants 11, 358. doi:10.3390/antiox11020358
Ricart, A. D. (2017). Drug-induced liver injury in Oncology. Ann. Oncol. 28, 2013–2020. doi:10.1093/annonc/mdx158
Rodriguez-Garcia, A., Lynn, R. C., Poussin, M., Eiva, M. A., Shaw, L. C., O’Connor, R. S., et al. (2021). CAR-T cell-mediated depletion of immunosuppressive tumor-associated macrophages promotes endogenous antitumor immunity and augments adoptive immunotherapy. Nat. Commun. 12, 877–917. doi:10.1038/s41467-021-20893-2
Romanò, C. L., Malizos, K., Capuano, N., Mezzoprete, R., D’Arienzo, M., Der, C. V., et al. (2016). Does an antibiotic-loaded hydrogel coating reduce early post-surgical infection after joint arthroplasty? J. Bone Jt. Infect. 1, 34–41. doi:10.7150/jbji.15986
Rubio-Ruiz, B., Pérez-López, A. M., Sebastián, V., and Unciti-Broceta, A. (2021). A minimally-masked inactive prodrug of panobinostat that is bioorthogonally activated by gold chemistry. Bioorg. Med. Chem. 41, 116217–116226. doi:10.1016/j.bmc.2021.116217
Saggar, J. K., and Tannock, I. F. (2015). Chemotherapy rescues hypoxic tumor cells and induces their reoxygenation and repopulation - an effect that is inhibited by the hypoxia-activated prodrug TH-302. Clin. Cancer Res. 21, 2107–2114. doi:10.1158/1078-0432.CCR-14-2298
Sahu, A., Kose, K., Kraehenbuehl, L., Byers, C., Holland, A., Tembo, T., et al. (2022). In vivo tumor immune microenvironment phenotypes correlate with inflammation and vasculature to predict immunotherapy response. Nat. Commun. 13, 5312. doi:10.1038/s41467-022-32738-7
Salem, A., and Salo, T. (2023). Identity matters: cancer stem cells and tumour plasticity in head and neck squamous cell carcinoma. Expert Rev. Mol. Med. 25, e8. doi:10.1017/erm.2023.4
Samadzadeh, S., Mousazadeh, H., Ghareghomi, S., Dadashpour, M., Babazadeh, M., and Zarghami, N. (2021). In vitro anticancer efficacy of Metformin-loaded PLGA nanofibers towards the post-surgical therapy of lung cancer. J. Drug Deliv. Sci. Technol. 61, 102318–8. doi:10.1016/j.jddst.2020.102318
Samstein, R. M., Lee, C. H., Shoushtari, A. N., Hellmann, M. D., Shen, R., Janjigian, Y. Y., et al. (2019). Tumor mutational load predicts survival after immunotherapy across multiple cancer types. Nat. Genet. 51, 202–206. doi:10.1038/s41588-018-0312-8
Sancho-albero, M., Rubio-ruiz, B., Pérez-lópez, A. M., Sebastián, V., Martín-Duque, P., Arruebo, M., et al. (2019). Cancer-derived exosomes loaded with ultrathin palladium nanosheets for targeted bioorthogonal catalysis. Nat. Catal. 2, 864–872. doi:10.1038/s41929-019-0333-4
Sarkar, N., and Bose, S. (2020). Controlled release of soy isoflavones from multifunctional 3D printed bone tissue engineering scaffolds. Acta Biomater. 114, 407–420. doi:10.1016/j.actbio.2020.07.006
Sarkar, S., Horn, G., Moulton, K., Oza, A., Byler, S., Kokolus, S., et al. (2013). Cancer development, progression, and therapy: an epigenetic overview. Int. J. Mol. Sci. 14, 21087–21113. doi:10.3390/ijms141021087
Seah, I., Zhao, X., Lin, Q., Liu, Z., Su, S. Z. Z., Yuen, Y. S., et al. (2020). Use of biomaterials for sustained delivery of anti-VEGF to treat retinal diseases. Eye 34, 1341–1356. doi:10.1038/s41433-020-0770-y
Sheets, K. T., Ewend, M. G., Mohiti-Asli, M., Tuin, S. A., Loboa, E. G., Aboody, K. S., et al. (2020). Developing implantable scaffolds to enhance neural stem cell therapy for post-operative glioblastoma. Mol. Ther. 28, 1056–1067. doi:10.1016/j.ymthe.2020.02.008
Shen, J., Cao, D., and Sun, J.-L. (2020). Ability of human umbilical cord mesenchymal stem cells to repair chemotherapy-induced premature ovarian failure. World J. Stem Cells 12, 277–287. doi:10.4252/wjsc.v12.i4.277
Shi, X., Cheng, Y., Wang, J., Chen, H., Wang, X., Li, X., et al. (2020). 3D printed intelligent scaffold prevents recurrence and distal metastasis of breast cancer. Theranostics 10, 10652–10664. doi:10.7150/thno.47933
Shin, K., Koo, K. H., Jeong, J., Park, S. J., Choi, D. J., Ko, Y.-G., et al. (2019). Three-dimensional culture of salivary gland stem cell in orthotropic decellularized extracellular matrix hydrogels. Tissue Eng. - Part A 25, 1396–1403. doi:10.1089/ten.tea.2018.0308
Siegel, R. L., Miller, K. D., Fuchs, H. E., and Jemal, A. (2021). Cancer statistics, 2021. Ca. Cancer J. Clin. 71, 7–33. doi:10.3322/caac.21654
Siemann, D. W., and Horsman, M. R. (2015). Modulation of the tumor vasculature and oxygenation to improve therapy. Pharmacol. Ther. 153, 107–124. doi:10.1016/j.pharmthera.2015.06.006
Singh, N., Deepack, B., Rajguru, J. P., Patil, P. B., Thankannavar, S. S., and Pujari, V. B. (2019). Inflammation and cancer. Ann. Afr. Med. 18, 121–126. doi:10.4103/aam.aam_56_18
Song, D. Y., Herfarth, K. K., Uhl, M., Eble, M. J., Pinkawa, M., Van Triest, B., et al. (2013). A multi-institutional clinical trial of rectal dose reduction via injected polyethylene-glycol hydrogel during intensity modulated radiation therapy for prostate cancer: analysis of dosimetric outcomes. Int. J. Radiat. Oncol. Biol. Phys. 87, 81–87. doi:10.1016/j.ijrobp.2012.12.019
Song, Y., Sharipol, A., Uchida, H., Ingalls, M. H., Piraino, L., Mereness, J. A., et al. (2022). Encapsulation of primary salivary gland acinar cell clusters and intercalated ducts (AIDUCs) within matrix metalloproteinase (MMP)-Degradable hydrogels to maintain tissue structure and function. Adv. Healthc. Mat. 11, 2101948–e2102014. doi:10.1002/adhm.202101948
Sørensen, B. S., and Horsman, M. R. (2020). Tumor hypoxia: impact on radiation therapy and molecular pathways. Front. Oncol. 10, 562–611. doi:10.3389/fonc.2020.00562
Sprung, C. N., Ivashkevich, A., Forrester, H. B., Redon, C. E., Georgakilas, A., and Martin, O. A. (2015). Oxidative DNA damage caused by inflammation may link to stress-induced non-targeted effects. Cancer Lett. 356, 72–81. doi:10.1016/j.canlet.2013.09.008
Sun, B., Taha, M. S., Ramsey, B., Torregrosa-Allen, S., Elzey, B. D., and Yeo, Y. (2016). Intraperitoneal chemotherapy of ovarian cancer by hydrogel depot of paclitaxel nanocrystals. J. Control. Release 235, 91–98. doi:10.1016/j.jconrel.2016.05.056
Sun, F., Lu, Y., Wang, Z., and Shi, H. (2021a). Vascularization strategies for tissue engineering for tracheal reconstruction. Regen. Med. 16, 549–566. doi:10.2217/rme-2020-0091
Sun, H., Dong, J., Wang, Y., Shen, S., Shi, Y., Zhang, L., et al. (2021b). Polydopamine-coated poly(l -lactide) nanofibers with controlled release of VEGF and BMP-2 as a regenerative periosteum. ACS Biomater. Sci. Eng. 7, 4883–4897. doi:10.1021/acsbiomaterials.1c00246
Suwa, T., Kobayashi, M., Nam, J. M., and Harada, H. (2021). Tumor microenvironment and radioresistance. Exp. Mol. Med. 53, 1029–1035. doi:10.1038/s12276-021-00640-9
Taemeh, M. A., Shiravandi, A., Korayem, M. A., and Daemi, H. (2020). Fabrication challenges and trends in biomedical applications of alginate electrospun nanofibers. Carbohydr. Polym. 228, 115419. doi:10.1016/j.carbpol.2019.115419
Talukder, M. E., Hasan, K. M. F., Wang, J., Yao, J., Li, C., and Song, H. (2021). Novel fibrin functionalized multilayered electrospun nanofiber membrane for burn wound treatment. J. Mat. Sci. 56, 12814–12834. doi:10.1007/s10853-021-06123-6
Tang, Y., Chen, L., Zhao, K., Wu, Z., Wang, Y., and Tan, Q. (2016). Fabrication of PLGA/HA (core)-collagen/amoxicillin (shell) nanofiber membranes through coaxial electrospinning for guided tissue regeneration. Compos. Sci. Technol. 125, 100–107. doi:10.1016/j.compscitech.2016.02.005
Tarnuzzer, R. W., Colon, J., Patil, S., and Seal, S. (2005). Vacancy engineered ceria nanostructures for protection from radiation-induced cellular damage. Nano Lett. 5, 2573–2577. doi:10.1021/nl052024f
Taylor, A., and Powell, M. E. B. (2004). Intensity-modulated radiotherapy - what is it? Cancer Imaging 4, 68–73. doi:10.1102/1470-7330.2004.0003
Theodore, N., Hlubek, R., Danielson, J., Neff, K., Vaickus, L., Ulich, T. R., et al. (2016). First human implantation of a bioresorbable polymer scaffold for acute traumatic spinal cord injury: a clinical pilot study for safety and feasibility. Neurosurgery 79, E305–E312. doi:10.1227/NEU.0000000000001283
Tohme, S., Simmons, R. L., and Tsung, A. (2017a). Surgery for cancer: a trigger for metastases. Cancer Res. 77, 1548–1552. doi:10.1158/0008-5472.CAN-16-1536
Tohme, S., Simmons, R. L., and Tsung, A. (2017b). Surgery for cancer: a trigger for metastases. Cancer Res. 77, 1548–1552. doi:10.1158/0008-5472.can-16-1536
Tsekoura, E. K., Pankongadisak, P., Graf, D., Boluk, Y., Uludağ, H., and Uludağ, H. (2021). Delivery of bioactive gene particles via gelatin-collagen-peg-based electrospun matrices. Pharmaceuticals 14, 666. doi:10.3390/ph14070666
Unnikrishnan, K., Thomas, L. V., and Ram Kumar, R. M. (2021). Advancement of scaffold-based 3D cellular models in cancer tissue engineering: an update. Front. Oncol. 11, 733652–733711. doi:10.3389/fonc.2021.733652
Wang, C., Sun, W., Ye, Y., Hu, Q., Bomba, H. N., and Gu, Z. (2017). In situ activation of platelets with checkpoint inhibitors for post-surgical cancer immunotherapy. Nat. Biomed. Eng. 1, 0011. doi:10.1038/s41551-016-0011
Wang, C., Wang, J., Zhang, X., Yu, S., Wen, D., Hu, Q., et al. (2018a). In situ formed reactive oxygen species-responsive scaffold with gemcitabine and checkpoint inhibitor for combination therapy. Sci. Transl. Med. 10, eaan3682–12. doi:10.1126/scitranslmed.aan3682
Wang, J., Wang, H.-J., and Qian, H. (2018b). Biological effects of radiation on cancer cells. Mil. Med. Res. 5, 20–10. doi:10.1186/s40779-018-0167-4
Wen, Y., Zhu, Y., Zhang, C., Yang, X., Gao, Y., Li, M., et al. (2022). Chronic inflammation, cancer development and immunotherapy. Front. Pharmacol. 13, 1040163–1040216. doi:10.3389/fphar.2022.1040163
West, E. R., Zelinski, M. B., Kondapalli, L. A., Gracia, C., Chang, J., Coutifaris, C., et al. (2009). Preserving female fertility following cancer treatment: current options and future possibilities. Pediatr. Blood Cancer 53, 289–295. doi:10.1002/pbc.21999
Westphal, M., Hilt, D. C., Bortey, E., Delavault, P., Olivares, R., Warnke, P. C., et al. (2004). A phase 3 trial of local chemotherapy with biodegradable carmustine (BCNU) wafers (Gliadel wafers) in patients with primary malignant glioma. Neuro. Oncol. 5, 79–88. doi:10.1215/s1522851702000236
Westwood, L., Emmerson, E., and Callanan, A. (2023). Fabrication of polycaprolactone electrospun fibres with retinyl acetate for antioxidant delivery in a ROS-mimicking environment. Front. Bioeng. Biotechnol. 11, 1233801–1233814. doi:10.3389/fbioe.2023.1233801
Wolf, M. T., Ganguly, S., Wang, T. L., Anderson, C. W., Sadtler, K., Narain, R., et al. (2019). A biologic scaffold-associated type 2 immune microenvironment inhibits tumor formation and synergizes with checkpoint immunotherapy. Sci. Transl. Med. 11, eaat7973. doi:10.1126/scitranslmed.aat7973
Wu, D., Witt, R. L., Harrington, D. A., and Farach-Carson, M. C. (2019). Dynamic assembly of human salivary stem/progenitor microstructures requires coordinated α1β1 integrin-mediated motility. Front. Cell Dev. Biol. 7, 224–315. doi:10.3389/fcell.2019.00224
Wu, X., Wang, Y., Zhu, C., Tong, X., Yang, M., Yang, L., et al. (2016). Preclinical animal study and human clinical trial data of co-electrospun poly(L-lactide-co-caprolactone) and fibrinogen mesh for anterior pelvic floor reconstruction. Int. J. Nanomedicine 11, 389–397. doi:10.2147/IJN.S88803
Xie, Z., Shen, J., Sun, H., Li, J., and Wang, X. (2021). Polymer-based hydrogels with local drug release for cancer immunotherapy. Biomed. Pharmacother. 137, 111333. doi:10.1016/j.biopha.2021.111333
Xu, W., Hu, X., and Pan, W. (2014). Tissue engineering concept in the research of the tumor biology. Technol. Cancer Res. Treat. 13, 149–159. doi:10.7785/tcrt.2012.500363
Xue, C. C., Li, M. H., Sutrisno, L., Yan, B. B., Zhao, Y., Hu, Y., et al. (2021). Bioresorbable scaffolds with biocatalytic chemotherapy and in situ microenvironment modulation for postoperative tissue repair. Adv. Funct. Mat. 31, 1–18. doi:10.1002/adfm.202008732
Xue, J., Wu, T., Dai, Y., and Xia, Y. (2019). Electrospinning and electrospun nanofibers: methods, materials, and applications. Chem. Rev. 119, 5298–5415. doi:10.1021/acs.chemrev.8b00593
Yan, W. L., LangYuan, T. W., Yin, Q., and Li, Y. P. (2022). Nanosized drug delivery systems modulate the immunosuppressive microenvironment to improve cancer immunotherapy. Acta Pharmacol. Sin. 43, 3045–3054. doi:10.1038/s41401-022-00976-6
Yang, G., Tian, J., Chen, C., Jiang, D., Xue, Y., Wang, C., et al. (2019). An oxygen self-sufficient NIR-responsive nanosystem for enhanced PDT and chemotherapy against hypoxic tumors. Chem. Sci. 10, 5766–5772. doi:10.1039/c9sc00985j
Yang, G., Xu, L., Chao, Y., Xu, J., Sun, X., Wu, Y., et al. (2017). Hollow MnO2 as a tumor-microenvironment-responsive biodegradable nano-platform for combination therapy favoring antitumor immune responses. Nat. Commun. 8, 902–913. doi:10.1038/s41467-017-01050-0
Yang, H., Villani, R. M., Wang, H., Simpson, M. J., Roberts, M. S., Tang, M., et al. (2018). The role of cellular reactive oxygen species in cancer chemotherapy. J. Exp. Clin. Cancer Res. 37, 266–310. doi:10.1186/s13046-018-0909-x
Yang, S., and Gao, H. (2017). Nanoparticles for modulating tumor microenvironment to improve drug delivery and tumor therapy. Pharmacol. Res. 126, 97–108. doi:10.1016/j.phrs.2017.05.004
Yang, X., Wang, Y., Mao, T., Wang, Y., Liu, R., Yu, L., et al. (2021). An oxygen-enriched thermosensitive hydrogel for the relief of a hypoxic tumor microenvironment and enhancement of radiotherapy. Biomater. Sci. 9, 7471–7482. doi:10.1039/d1bm01280k
Yang, Y., Chen, L., Zheng, B., and Zhou, S. (2023). Metabolic hallmarks of natural killer cells in the tumor microenvironment and implications in cancer immunotherapy. Oncogene 42, 1–10. doi:10.1038/s41388-022-02562-w
Yao, Y., Zhang, H., Wang, Z., Ding, J., Wang, S., Huang, B., et al. (2019). Reactive oxygen species (ROS)-responsive biomaterials mediate tissue microenvironments and tissue regeneration. J. Mat. Chem. B 7, 5019–5037. doi:10.1039/c9tb00847k
Ye, K., Kuang, H., You, Z., Morsi, Y., and Mo, X. (2019). Electrospun nanofibers for tissue engineering with drug loading and release. Pharmaceutics 11, 182–217. doi:10.3390/pharmaceutics11040182
Yoo, C., Vines, J. B., Alexander, G., Murdock, K., Hwang, P., and Jun, H. W. (2014). Adult stem cells and tissue engineering strategies for salivary gland regeneration: a review. Biomater. Res. 18, 9–12. doi:10.1186/2055-7124-18-9
Yu, Z., Yu, S., Laijun, L., Wenjing, L., Chaojing, L., Hong, J., et al. (2022). Construction of ultrasmooth PTFE membrane for preventing bacterial adhesion and cholestasis. Colloids Surfaces B Biointerfaces 213, 112332. doi:10.1016/j.colsurfb.2022.112332
Zahid, S., Khalid, H., Ikram, F., Iqbal, H., Samie, M., Shahzadi, L., et al. (2019). Bi-layered α-tocopherol acetate loaded membranes for potential wound healing and skin regeneration. Mat. Sci. Eng. C 101, 438–447. doi:10.1016/j.msec.2019.03.080
Zhan, Q., Shen, B., Deng, X., Chen, H., Jin, J., Zhang, X., et al. (2013). Drug-eluting scaffold to deliver chemotherapeutic medication for management of pancreatic cancer after surgery. Int. J. Nanomedicine 8, 2465–2472. doi:10.2147/ijn.s47666
Zhang, G., Cheng, W., Du, L., Xu, C., and Li, J. (2021). Synergy of hypoxia relief and heat shock protein inhibition for phototherapy enhancement. J. Nanobiotechnology 19, 9–13. doi:10.1186/s12951-020-00749-5
Zhang, J., Muirhead, B., Dodd, M., Liu, L., Xu, F., Mangiacotte, N., et al. (2016). An injectable hydrogel prepared using a PEG/vitamin E copolymer facilitating aqueous-driven gelation. Biomacromolecules 17, 3648–3658. doi:10.1021/acs.biomac.6b01148
Zhang, L., Dong, Y., Liu, Y., Liu, X., Wang, Z., Wan, J., et al. (2023a). Multifunctional hydrogel/platelet-rich fibrin/nanofibers scaffolds with cell barrier and osteogenesis for guided tissue regeneration/guided bone regeneration applications. Int. J. Biol. Macromol. 253, 126960. doi:10.1016/j.ijbiomac.2023.126960
Zhang, L., Zhou, J., Hu, L., Han, X., Zou, X., Chen, Q., et al. (2020a). Cellulose II aerogel-based triboelectric nanogenerator. Adv. Funct. Mat. 30, 2001763. doi:10.1002/adfm.202001763
Zhang, M., Chen, X., Yang, K., Dong, Q., Yang, H., Gu, S., et al. (2023b). Dual-crosslinked hyaluronic acid hydrogel with self-healing capacity and enhanced mechanical properties. Carbohydr. Polym. 301, 120372. doi:10.1016/j.carbpol.2022.120372
Zhang, Y., Ho, S. H., Li, B., Nie, G., and Li, S. (2020b). Modulating the tumor microenvironment with new therapeutic nanoparticles: a promising paradigm for tumor treatment. Med. Res. Rev. 40, 1084–1102. doi:10.1002/med.21644
Zhao, H., Wu, L., Yan, G., Chen, Y., Zhou, M., Wu, Y., et al. (2021). Inflammation and tumor progression: signaling pathways and targeted intervention. Signal Transduct. Target. Ther. 6, 263. doi:10.1038/s41392-021-00658-5
Zhao, J., and Cui, W. (2020). Functional electrospun fibers for local therapy of cancer. Adv. Fiber Mat. 2, 229–245. doi:10.1007/s42765-020-00053-9
Keywords: cancer treatment, tumor microenvironment, chemotherapy, radiotherapy, surgery, tissue engineering, repair, regeneration
Citation: Westwood L, Nixon IJ, Emmerson E and Callanan A (2024) The road after cancer: biomaterials and tissue engineering approaches to mediate the tumor microenvironment post-cancer treatment. Front. Front. Biomater. Sci. 3:1347324. doi: 10.3389/fbiom.2024.1347324
Received: 30 November 2023; Accepted: 14 March 2024;
Published: 27 March 2024.
Edited by:
Iman Yazdi, Moderna Therapeutics, United StatesReviewed by:
Changlu Xu, University of California, Los Angeles, United StatesCopyright © 2024 Westwood, Nixon, Emmerson and Callanan. This is an open-access article distributed under the terms of the Creative Commons Attribution License (CC BY). The use, distribution or reproduction in other forums is permitted, provided the original author(s) and the copyright owner(s) are credited and that the original publication in this journal is cited, in accordance with accepted academic practice. No use, distribution or reproduction is permitted which does not comply with these terms.
*Correspondence: Anthony Callanan, YW50aG9ueS5jYWxsYW5hbkBlZC5hYy51aw==
Disclaimer: All claims expressed in this article are solely those of the authors and do not necessarily represent those of their affiliated organizations, or those of the publisher, the editors and the reviewers. Any product that may be evaluated in this article or claim that may be made by its manufacturer is not guaranteed or endorsed by the publisher.
Research integrity at Frontiers
Learn more about the work of our research integrity team to safeguard the quality of each article we publish.