- 1School of Chemical Engineering, University of Birmingham, Birmingham, United Kingdom
- 2Healthcare Technologies Institute, University of Birmingham, Birmingham, United Kingdom
- 3Suzhou Microport Regenerative Medicine Technologies Co., Ltd., Suzhou, China
- 4Centre for Regenerative Medicine, Institute for Regeneration and Repair, University of Edinburgh, Edinburgh, United Kingdom
The mechanical performance of tissue-engineered grafts is crucial in determining their functional properties, integration with native tissue and long-term repair outcome post-implantation. To date, most approaches for testing the mechanical properties of tissue-engineered grafts are non-sterile and invasive. There is an urgent need to develop novel sterile approaches for online monitoring mechanical properties of engineered tissues in order to ensure these engineered products meet the desired mechanical strength prior to implantation. In this paper, we overview various approaches for mechanical testing of engineered tissues, which span from traditional methods to medical imaging concepts in magnetic resonance elastography, ultrasound elastography, and optical coherence elastography. We focused on the applicability of these methods to the manufacturing of tissue-engineered products online, e.g., if such approach provides a sterile monitoring capacity and is capable of defining mechanical heterogeneity in engineered tissues throughout their growth in vitro in real-time. The review delves into various imaging modalities that employ distinct methods for inducing displacement within the sample, utilizing either strain-based or shear wave-based approaches. This displacement can be achieved through external stimulation or by harnessing ambient vibrations. Subsequently, the imaging process captures and visualizes the resultant displacement. We specifically highlight the advantages of novel non-invasive imaging methods such as ultrasound elastography and optical coherence elastography to assess the mechanical properties of engineered tissues in vitro, as well as their potential applications in cancer study, drug screening and the in vivo evaluation of the engineered tissues.
1 Introduction
Tissue engineering strategies offer a promising approach for replacing diseased and damaged tissues in patients such as cartilage, bone, tendon and blood vessels (El et al., 2005; Sharma et al., 2005). Most of these tissues experience a highly challenging biomechanical environment in vivo. The mechanical properties of tissue-engineered grafts determine their functional performance, integration with native tissue as well as long-term repair outcomes post-implantation (Vijayavenkataraman et al., 2018). Therefore, monitoring their mechanical properties during culture and characterizing them prior to implantation is important for defining their ultimate quality and clinical outcomes.
Tissue engineered grafts, including tissue engineered skin and bone, are reaching clinical readiness, and manufacturing these grafts requires regulatory assurance (Trommelmans et al., 2008). Reproducibility, product metrics, guidelines and manufacturing confidence parameters are required for tissue-engineered or biofabricated products to be used in the clinic. To achieve this, manufacturing protocols that define parameters during production and the ability to monitor in vitro cultures are required. The main parameters that should be assessed are both the bulk and spatial mechanical properties of engineered tissues.
Current challenges in assessing the mechanical properties of tissue engineering products throughout their fabrication are multiple. First, conventional mechanical testing methodologies typically necessitate the direct interaction of the mechanical load with the sample, a process that is inherently non-sterile and destructive. Secondly, they provide only the bulk mechanical properties of the graft while most native organs and tissues are heterogeneous in nature with distinctive biomechanical and structural heterogeneity. Engineering tissues with such native-like biomechanical and structural heterogeneity is crucial to ensure function after implantation (Klein et al., 2009a; Klein et al., 2009b; Khoshgoftar et al., 2013).
Therefore, we believe that scaling up translational applications of tissue engineering will require the integration of technologies able to monitor bulk mechanical properties as well as the spatial mechanical heterogeneity in a contactless and non-destructive manner as tissues develop in bioreactors.
Here we briefly review and discuss potential candidates, based on imaging, to answer these challenges by providing a sterile monitoring capacity and access to the mechanical heterogeneity in engineered tissues in addition to bulk mechanical properties (Dalecki et al., 2016; Kim and Wagner, 2016; Kim et al., 2016; Larin and Sampson, 2017; Othman et al., 2015).
2 Conventional approaches
A common approach for mechanically testing biomaterials and engineered tissues involves compressing or stretching test samples while monitoring the corresponding the sample response to the induced changes. Typically, the force change is measured by a load cell while displacement is measured through a mechanical actuator or via video recording. Elastic modulus is then calculated from the stress-strain curve. Such approaches have been widely used for testing the mechanical properties of various tissue engineered grafts such as engineered skin (Sander et al., 2014), bladder (Rosario et al., 2008), cartilage (Luo et al., 2015), bone (Freeman et al., 2019) and tendon (Webb et al., 2013).
There are many commercially available pieces of equipment for conducting tests using these approaches. For example, the Bose ElectroForce from TA Instruments has been widely used for testing large-sized tissue engineered constructs (ranging from millimetre to centimetre scale) (Luo et al., 2015), whereas the Microtester from CellScale Biomaterials Testing has been mainly used for testing smaller-sized samples, such as cell spheroids and hydrogel microspheres (Cortes et al., 2019). A common limitation of these systems is that tests are usually conducted in a non-sterile manner leading to the termination of tissue culture. This implies that once tested they can no longer be used for implantation. Additionally, most measurements typically employ a 5%–10% strain, which can potentially damage the tissue’s structure and mechanical properties.
Recently, several instrument companies have started to produce systems suitable for online monitoring of mechanical properties of tissue engineered grafts. For example, the BioDynamic system developed by TA Instruments functions as both a mechanical stimulation bioreactor and a mechanical testing machine. In this system, the test is also conducted by measuring force and displacement, however, within a sterile chamber. Thus, samples can be returned to culture afterwards, enabling online monitoring of growing constructs. In addition to these commercial systems, researchers have also started to design their own bespoke systems for sterile online monitoring mechanical properties of engineered tissues. For example, Ahearne et al. developed an indentation system suitable for online monitoring viscoelastic properties of thin hydrogel-based constructs such as tissue engineered corneal (Figure 1) (Ahearne et al., 2005). Kortsmit et al. developed a perfusion bioreactor system that is capable of online monitoring mechanical properties of tissue engineered heart valves (Kortsmit et al., 2009a; Kortsmit et al., 2009b).
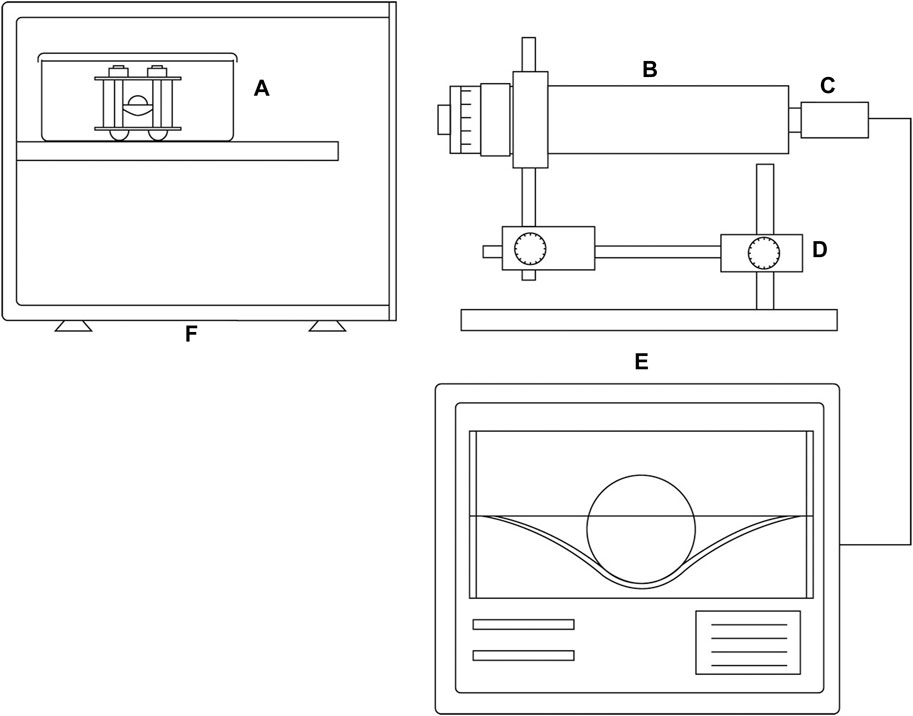
Figure 1. A schematic of the instrument system developed for sterile mechanical testing, designed to induce and measure deformation, as well as assess the creep behavior of membranes. (A) Sterile testing chamber consisting of a sample holder and an autoclavable stainless steel indentation ball. (B) long working distance microscope; (C) camera; (D) precision X–Y translation stage; (E) image analysis software; (F) incubator. Reproduced from Ahearne et al. with permission (Ahearne et al., 2005).
While promising, most new commercial and bespoke systems developed so far have limitations in the size or geometry of the test samples. For example, BioDynamic from TA Instruments is designed for testing large-sized tissue engineered grafts of millimetres and centimetres scale, not suitable for micro-tissues such as cell spheroids. Systems developed by Ahearne et al. and Kortsmit et al. have specific shape requirements, e.g., thin engineered tissues and engineered heart valves respectively (Ahearne et al., 2005; Kortsmit et al., 2009a). Furthermore, testing results derived from these systems only reflect bulk mechanical properties of the graft, gaining no insight into the spatial variation in mechanical properties throughout the 3D structure.
3 Elastography
Elastography is a medical imaging technique to map the mechanical properties of the test sample. It is conducted through applying either a force or mechanical wave onto the sample and then measuring the subsequent deformation or propagation of the shear wave within the sample (Kim et al., 2016). It is usually classified by imaging modalities (e.g., Magnetic resonance imaging (MRI), Ultrasound imaging (UI) and Optical coherence tomography (OCT)) and mechanical excitation methods (e.g., strain-based or shear wave-based). These methods will be discussed in detail in this review. Elastography offers a few advantages over the traditional approaches outlined above on measuring mechanical properties of tissue-engineered products. First, elastography offers details in spatial biomechanical and structural heterogeneity within the engineered tissue (Dalecki et al., 2016; Kim and Wagner, 2016; Larin and Sampson, 2017; Othman et al., 2015). Secondly, shear wave-based elastography induce micro to nanometre scale displacements, which is non-destructive and has minimum impact on the structure and biomechanical properties of the sample (Kim et al., 2016). Lastly and most importantly, the emergence of novel non-contact excitation methods in ultrasound elastography (Kim and Wagner, 2016) and optical coherence elastography (Kennedy et al., 2017; Larin and Sampson, 2017) have promoted new developments in sterile online monitoring of tissue engineered constructs.
3.1 Magnetic resonance elastography (MRE)
MRE uses MRI to map the stiffness of test samples. MRI is commonly used in clinics for medical diagnosis as it offers high-quality contrast between neighbouring soft tissues. Clinical MRI scanners offer a resolution at around 1mm, while customized scanners for research purpose can reach as low as 80 µm in resolution (Van Reeth et al., 2012). MRE has been clinically used to study pathological changes in tissue stiffness such as in tumour formation (McKnight et al., 2002; Bohte et al., 2018), liver fibrosis (Shire et al., 2011; Zhang et al., 2020) and osteoarthritis onset (Mariappan et al., 2010). However, only a few studies have investigated MRE for monitoring mechanical properties of tissue engineered grafts. Among these, two major methods have been used to map mechanical properties of engineered tissues: strain-based (Neu et al., 2009; Griebel et al., 2014) and shear wave-based (Curtis et al., 2012; Yin et al., 2014) MRE.
3.1.1 Strain-based MRE
Strain-based MRE maps the displacement and strain within the engineered tissue under compression using MRI. Neu et al. inserted an in vitro tissue engineered cartilage construct (agarose seeded with chondrocytes) into a native explant and compared the deformation of tissue engineered construct and the native cartilage under compression (Neu et al., 2009). MRI showed a significantly higher strain in the engineered construct compared to the native tissue, which correlates with the lower amount of proteoglycan in the engineered construct, demonstrating the potential of MRE in evaluating mechanical functions of tissue engineered constructs in cartilage defect model. In a follow-on study, they fabricated a bilayer acellular agarose construct (2% + 4% agarose) and tracked its deformation under compression. A significantly higher strain was observed in the softer 2% agarose layer (Figure 2), demonstrating that MRE can be used to map biomechanical heterogeneity in engineered tissues (Griebel et al., 2014). While promising, elastic modulus was not reported in these studies as the applied force was not quantified. Furthermore, sterile online monitoring was not achieved in these studies.
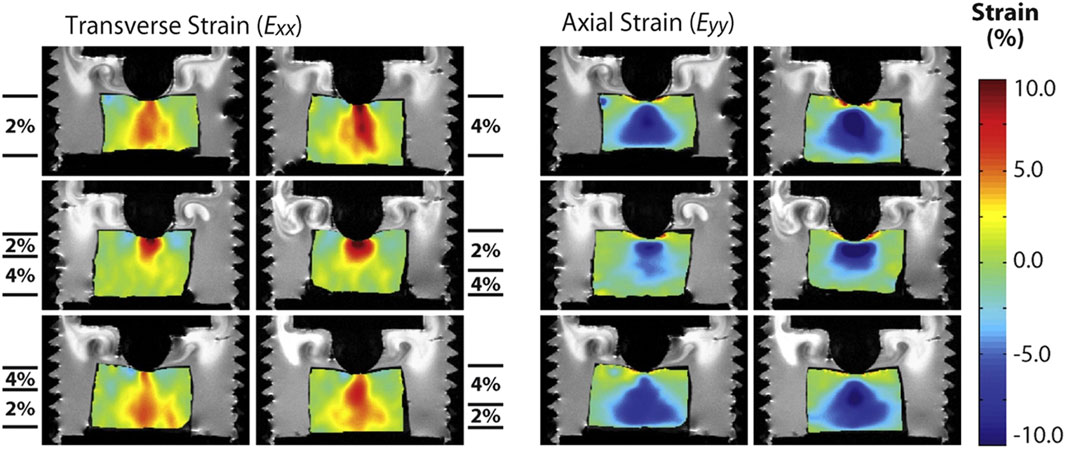
Figure 2. Transverse and axial images demonstrating two-dimensional internal strain fields measured by dualMRI. 2% agarose gel, 4% agarose gel and bilayer agarose gel with either 2% gel or 4% gel on the top were compressed using a custom-designed indentation system, and the deformation of samples was tracked with MRI. Transverse and axial strain fields were shown for each sample type. Adapted from Griebel et al. with permission (Griebel et al., 2014).
3.1.2 Shear wave-based MRE
Shear wave-based MRE involves applying a harmonic mechanical excitation onto samples and tracking the propagation of the shear wave in the sample using MRI to compute and map the stiffness of the sample (Kim et al., 2016). A harmonic mechanical excitation is generated using a piezo actuator resulting in the propagation of a shear wave into the samples (Curtis et al., 2012; Yin et al., 2014). Phase contrast MRI is then used to capture shear wave images in the sample and calculate its velocity propagating in the sample. Assuming the sample is isotropic, Young’s modulus can be calculated through the shear modulus calculations.
Yin et al. used shear wave-based MRE to evaluate the growth of chondrocyte pellets undergoing chondrogenic differentiation over 3 weeks culture and found that the shear stiffness of pellets increased from 6.4 kPa at day 1–16.4 kPa at day 21, which correlates with the increase in proteoglycan and collagen content in pellets (Figure 3) (Yin et al., 2014). Curtis et al. also used shear wave-based MRE to monitor mechanical properties of mesenchymal stem cell-seeded gelatine constructs undergoing adipogenic and osteogenic differentiation, respectively. The shear modulus of constructs cultured in adipogenic medium decreased upon culture time whereas it increased overtime for constructs cultured in osteogenic medium, in line with their corresponding differentiation pathways (Curtis et al., 2012). However, sterile online monitoring of construct stiffness using shear wave-based MRE with a piezoelectric actuator remains a challenge, as constructs will have to be embedded in agarose gel during cell culture. Furthermore, this technique is limited by sample size and stiffness. Using shear wave-based MRE to evaluate small and stiff tissue engineered constructs (e.g., bone construct) is challenging as it requires a high excitation frequency to shorten the wavelength so that it can be captured in the sample (Manduca et al., 2001). However, an increased excitation frequency is associated with an increased wave attenuation in the test sample (Lopez et al., 2007), making the shear wave not able to be attained (Othman et al., 2012), and this remains an obstacle for researchers to address (Othman et al., 2015).
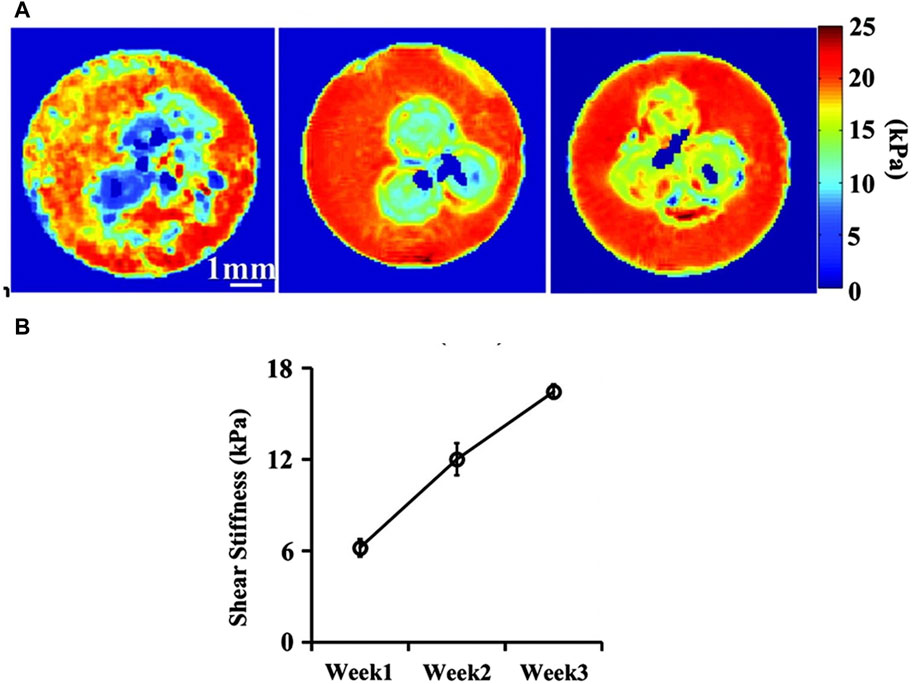
Figure 3. Shear wave-based MRE monitoring the stiffness of chondrocyte pellets over a 3-week chondrogenic differentiation period. (A) Shear stiffness maps of chondrocyte pellets (three spheres embedded at the centre of agarose gel) at week 1, two and 3; (B) Shear stiffness values of these pellets at week 1, 2 and 3. Adapted from Yin et al. with permission (Yin et al., 2014).
3.2 Ultrasound elastography (UE)
UE utilizes UI to map strain and elastic modulus of test samples. Clinical UI offers a resolution at around 100 μm, whereas research UI modalities that use higher ultrasound frequency than that of the clinics have also been developed recently and they can provide a resolution of 10–100 µm (Dalecki et al., 2016; Wang and Larin, 2015). UE has been widely used to measure the stiffness of tissues and organs such as skeletal muscle (Yanagisawa et al., 2011; Chino et al., 2012), tendon (Drakonaki et al., 2009; Chernak Slane and Thelen, 2014), breast tumour (Thitaikumar et al., 2008; Thittai et al., 2011) and vessel (de Korte et al., 2011; McCormick et al., 2012) in many preclinical and clinical studies. However, only a handful studies have explored this novel technique to measure the stiffness of engineered tissues. Similar to MRE, these researches mainly employed strain-based (Dutta et al., 2013; Chung et al., 2015; Van Kelle et al., 2017) or shear wave-based (Mercado et al., 2015) methods to map mechanical properties of tissue engineered constructs.
3.2.1 Strain-based UE
In the strain-based method, ultrasound signals are acquired using conventional B-mode UI before and after the application of a small strain through either compression or distension of test samples. Speckle tracking is then used to map out the displacement or strain in the engineered tissue. Van Kelle et al. developed a novel multimodal bioreactor, which enables a long-term culture of engineered cardiovascular tissues under mechanical stimulation and simultaneous mechanical testing of the developing tissue (Van Kelle et al., 2017). The stiffness of grafts was monitored weekly through a bulge test carried out within the pressure bioreactor and the displacement was tracked using UI in a sterile manner, thus enabling online monitoring of the stiffness of the engineered tissue. In a similar research, Chung et al. used UI to measure the deformation of tissue-engineered cartilage grafts in a compression test carried out within a sterile chamber (Chung et al., 2015). Higher strains were observed in the centre of engineered tissues compared to the periphery, demonstrating the feasibility of using UE to map the spatial mechanical heterogeneity of engineered tissues. However, one of the limitations of these two studies is that no elastic modulus but only strain was reported.
Dutta et al. successfully achieved online measurement of the elastic modulus of tissue engineered vessels using UE (Dutta et al., 2013). In this study, engineered vessels were cultured in a pulsatile flow bioreactor, the distension of grafts in response to the flow was monitored using UI in a sterile manner and the pressure of the flow that the tissue experienced was measured by a pressure transducer. Subsequently, Young’s modulus was calculated through the measured pressure and strain and these computed values were found to match well with those obtained by traditional mechanical testing methods. While these results are very encouraging, the bioreactor developed in this study is suitable for online monitoring of engineered vessel grafts with a tubular geometry only. New bioreactor designs are needed to align with online monitoring of other types of engineered tissues such as cartilage and bone grafts.
3.2.2 Shear wave-based UE
In shear wave-based methods, an ultrasound transducer emits a non-contact acoustic radiation force (ARF) to remotely manipulate a small region within test samples. This induces a shear wave through the recoil of the deformed region. The propagation of this wave through the sample is closely monitored using UI (W. Kim et al., 2016). Similar to shear wave-based MRE, the shear modulus of the sample is derived from the speed of the shear wave. Shear wave-based UE, including Supersonic shear imaging-based UE, have mainly been used for examining breast cancer (David O. Cosgrove, Berg, Doré, Skyba, Henry, Gay, Cohen-Bacrie, and the 2012), liver disease (Farmakis et al., 2019) and cornea disease (Nguyen et al., 2012) across numerous in vivo and clinical studies. To the author’s knowledge, to date, only one study has employed shear wave-based UE for online monitoring mechanical properties of tissue engineered constructs (Mercado et al., 2015). Within this study, fibroblast-seeded collagen gels, submerged in cell culture media—which facilitates the transfer of the ultrasound pulse from the transducer into the engineered tissues—were successfully monitored within a sterile system, and the shear modulus of the engineered tissue was effectively obtained.
3.3 Optical coherence elastography (OCE)
OCE leverages OCT to map the strain or elastic modulus of tested samples. OCT is a non-invasive imaging modality. It employs low coherence light to capture cross-sectional 2D or 3D images of an optical scattering sample (Huang et al., 1991). Recently, OCT has been explored within the field of tissue engineering as a non-invasive imaging modality to monitor cell proliferation, migration, cell-material interaction as well as structural changes in engineered tissues (Yang et al., 2005; Yang et al., 2006; Bagnaninchi et al., 2007; Liang et al., 2009; Holmes et al., 2015; Martucci et al., 2018). Offering a superior resolution of 5–15 μm, OCT is a good candidate for discerning the mechanical properties of engineered tissues when compared to MRI and UI (Kennedy et al., 2017; Wang and Larin, 2015). Both strain-based and shear wave-based OCE have been used to characterize the mechanical properties across a wide range of tissues including, but no limited to, breast (Kennedy et al., 2013), artery (Razani et al., 2014), liver, muscle (Ahmad et al., 2014), and tendon ex vivo (Guan et al., 2013), as well as skin (Kennedy et al., 2011) and cornea in vivo (Li et al., 2020; Zvietcovich et al., 2019). However, only a handful of studies have explored OCT for measuring mechanical properties of engineered tissues (Ko et al., 2006; Chhetri et al., 2010).
3.3.1 Strain-based OCE
Strain-based OCE leverage OCT to map sample deformation in response to an external force applied either by contact or remotely. Ko et al. employed OCT to monitor the stiffness of a cell-seeded collagen hydrogel over a 10-day culture period using a custom-designed compression system (Ko et al., 2006). Decreased strain in the engineered tissue was observed upon culture, indicating the increase in stiffness of the engineered tissue, which correlates with the increased matrix deposition in the engineered construct as shown with histological analysis. Although sterile online monitoring was not achieved, this study was the first to apply OCE to monitor stiffness of engineered tissues. In another study, Yang et al. developed an OCT-based microindentation technique for mechanical characterization of hydrogels (Yang et al., 2007). The test was carried out by placing a sterilized metal ball with known weight on top of the hydrogel and tracking its displacement with OCT. The Young’s modulus of the hydrogel obtained with this OCT-based microindentation method was found to match well with those obtained using microindentation test. Even though the study used only acellular hydrogel as a proof of concept, the method itself can also be used to online monitor mechanical properties of engineered tissues, since the test can be carried out in a sterile culture dish.
Aiming to use remotely applied force to induce the deformation in samples, Chhetri et al. used magnetomotive OCE (MMOCE) to monitor the stiffness of tissue engineered airway over a culture period of 32 h in a sterile manner (Chhetri et al., 2010). Human tracheobronchial epithelial cells were seeded onto an electrospun scaffold that was precoated with magnetic nanoparticles. The deformation of engineered tissue in response to a regulated external magnetic field was tracked using OCT as a MMOCE study. Albeit smaller strains were observed in the engineered tissue with augmented culture time, no elastic modulus was quantified in this study. More recently, air puff technology another method to remotely induce deformation, has been used with OCT to map the strain of ex vivo corneal tissue (Alonso-Caneiro et al., 2011; Dorronsoro et al., 2012), although its application within tissue engineering remains to be explored. This novel method together with magnetomotive OCE holds potential for the sterile online monitoring of engineered tissues stiffness.
3.3.2 Shear wave-based OCE
Shear wave-based OCE utilises OCT to measure the propagation of shear wave in the sample and deduce the elastic modulus of the sample. Studies have explored shear wave generated from various source such as piezoelectric actuators (Zvietcovich et al., 2019), laser impulse (Li et al., 2011), ultrasound (Zhou et al., 2018; Liu et al., 2021; Liu et al., 2023), air impulse (Han et al., 2016) and magnetic particles (Ahmad et al., 2014) to measure the elastic modulus of tissues ex vivo or in vivo. Li et al. used OCT to detect laser induced surface acoustic wave in tissue mimicking agar phantoms, facilitating Young’s modulus calculation via measured phase-velocity values (Li et al., 2011). Zhou et al. used OCT coupled with high intensity focused ultrasound to map the elastic modulus of agar phantoms and ex vivo porcine skin (Zhou et al., 2018). Han et al. used shear wave-based OCE induced with air impulse to quantify the Young’s modulus of chicken liver (Han et al., 2016). Ahmad et al. demonstrate the feasibility of using magnetic particles to induce shear waves in rat liver and measure its Young’s modulus through shear wave-based OCE (Ahmad et al., 2014). Despite their capacity for a non-contact mechanical testing environment, none of these shear wave-based OCE methods, to the author’s knowledge, have been deployed to explore the mechanical properties of tissue-engineered constructs.
Passive elastography is an alternative approach, which does not depend on external force or direct mechanical stimulation applied to the tissue. Rather, it utilises naturally occurring mechanical vibrations or movements within the environment. Nguyen et al. (Nguyen et al., 2016) extended the concept of passive elastography (Catheline et al., 2013) to OCT, which relies on naturally occurring broadband diffuse shear waves. Zvietcovich et al. adopted a closely related approach, measuring shear wavelength of reverberant waves to quantify individual corneal layers stiffness (Zvietcovich et al., 2019).
Passive elastography has a great potential for imaging tissue mechanical contrast with OCT and does not require any hardware modifications. This contactless technique can be easily executed in a sterile manner and could be integrated in a manufacturing workflow. Its ability to quantify the shear wavelength is however greatly biased by noise levels.
Recently Mason et al. introduced a debiased passive elastography technique that leverages ambient vibrations for real-time, non-invasive quantitative stiffness assessment (Mason et al., 2023). Using the technique, MechAscan, they evaluated the stiffness of gel constructs, tissue engineered bone and cartilage spheroids, bilayer cancer model, tissue repair model and single cells. The stiffness of engineered bone spheroids was investigated throughout differentiation and maturation in vitro in a sterile manner. MechAscan analysis showed an increase in sample stiffness with culture time. This finding was validated with histology and compression test applied to a subset of samples. Similarly, MechAscan stiffness analysis of engineered cartilage spheroids subjected to hydrostatic pressure was also compatible with biochemical analysis (Figure 4). The research group showcased employed method’s aptitude to map spatial mechanical heterogeneity in engineered tissues using a bilayer cancer model. This passive elastography method, which relies on ambient vibrations without necessitating an external stimulator, may present substantial advantages as a non-contact, sterile, online monitoring tool for the mechanical analysis of engineered constructs directly inside bioreactors.
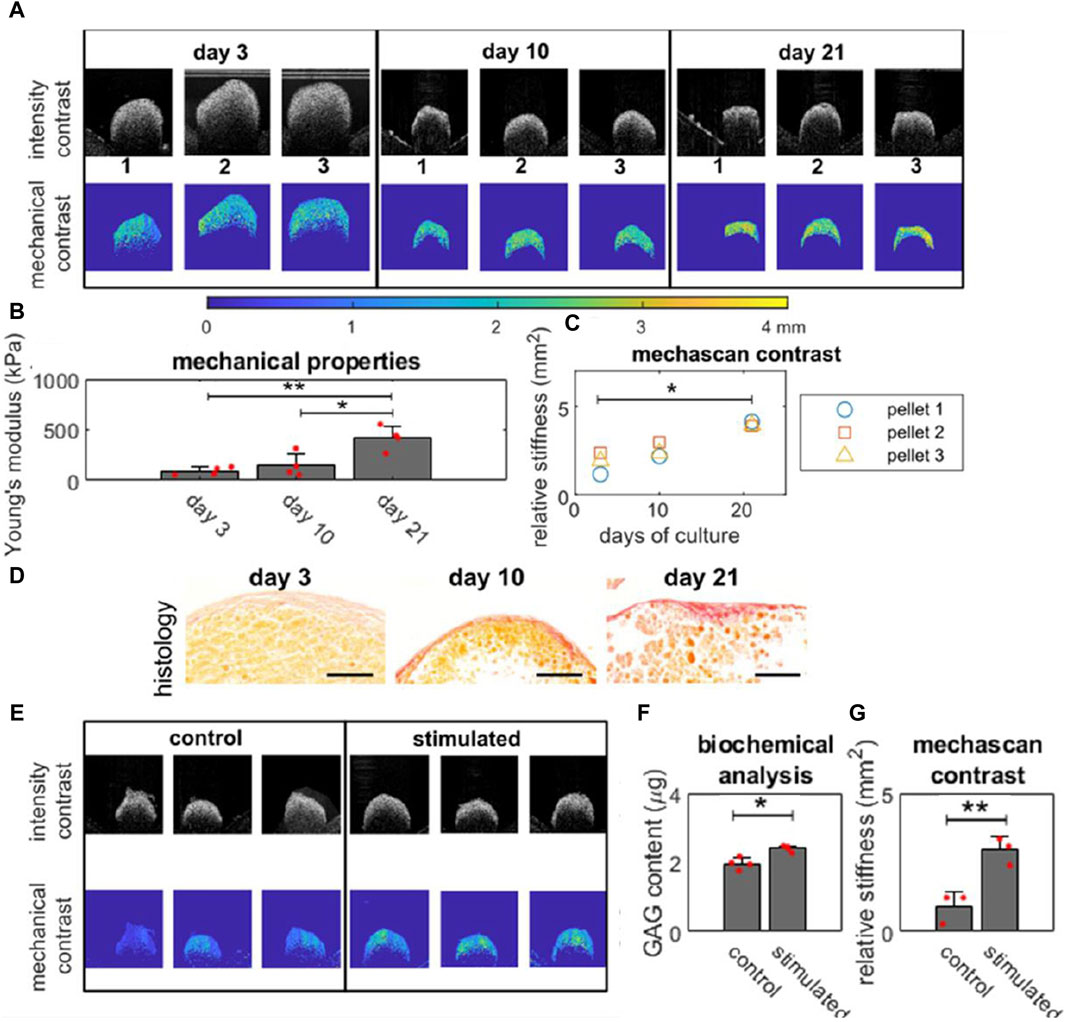
Figure 4. Online monitoring mechanical properties of engineered bones and cartilage tissues. (A) OCT intensity images and mechanical contrast map (generated using MechAscan) of engineered bone tissues (in triplicate) on day 3, 10, and 21; (B) Young’s modulus of the tissue (n = 3) obtained using a compression rig; (C) Mechanical stiffness data of each sample throughout culture, obtained by MechAscan; (D) Histology with picrosirius red staining for collagen content in osteo-genic pellets at day 3, 10 and 21. Orange and red staining represent cytoplasm and new synthesized collagen fibrils respectively with a 100 µm scale bar; (E) intensity images and mechanical contrast map (generated using MechAscan) of engineered cartilage tissues stimulated by hydro-static pressure for 21 days; (F) Glycosaminoglycans (GAG) content of samples and (G) the mechanical stiffness data (generated using MechAscan) of engineered cartilage tissues (n = 3). Reproduced from Mason et al. with permission (Mason et al., 2023).
4 Discussion and outlook
Tissue elasticity and mechanical properties are frequently evaluated in clinical settings. Non-invasive techniques such as OCE, UE, and MRE assist in diagnosing diverse conditions by offering significant insights into the elasticity or stiffness of tissues. MRE, utilizing MRI technology, generates images illustrating tissue stiffness variations, facilitating the noninvasive evaluation of viscoelastic characteristics in organs like the brain and liver (Klatt et al., 2007; Asbach et al., 2008; Green et al., 2008). Similarly, UE, employing ultrasound technology, measures tissue elasticity by assessing the speed of shear wave transmission, finding application across medical specialties such as evaluating pancreas, liver fibrosis (D. Cosgrove et al., 2013), and breast lesions (Cosgrove et al., 2012; Barr et al., 2015). Guidance for these studies has been well documented (D. Cosgrove et al., 2013; Miller et al., 2020). MRE and UE have proven valuable in clinical settings for elastographic imaging across various organs (e.g., breast, liver, brain). Their application to thin and small tissues like the cornea is limited due to relatively lower spatial resolution and decreased displacement sensitivities (Singh et al., 2017) when compared to OCE which has high resolution but low imaging depth. OCE, a non-contact, light-based imaging technique predominantly used in ophthalmology, assesses corneal tissue stiffness through non-contact examination methods like air-pulse systems (Wang et al., 2013; Twa et al., 2019). However, one typical drawback of these methods is the necessity to terminate tissue culture due to the non-sterile procedure, rendering the sample unsuitable for post-testing implantation.
This article presents an overview of various approaches for imaging the mechanical properties of tissue-engineered grafts, acellular biomaterials and ex vivo tissues. It highlights their distinct features, advantages and limitations as summarized in Table 1. Traditional approaches, commonly used for assessing mechanical properties, provide insights into stiffness and viscoelastic properties of samples (Cao et al., 2023). These techniques are applicable in the analysis of mechanical properties ranging from soft tissue mimicking hydrogels (Tejo-Otero et al., 2022) to bone (Gupta et al., 2021). However, one typical drawback of these methods is the necessity to terminate tissue culture due to the non-sterile procedure, rendering the sample unsuitable for post-testing implantation.
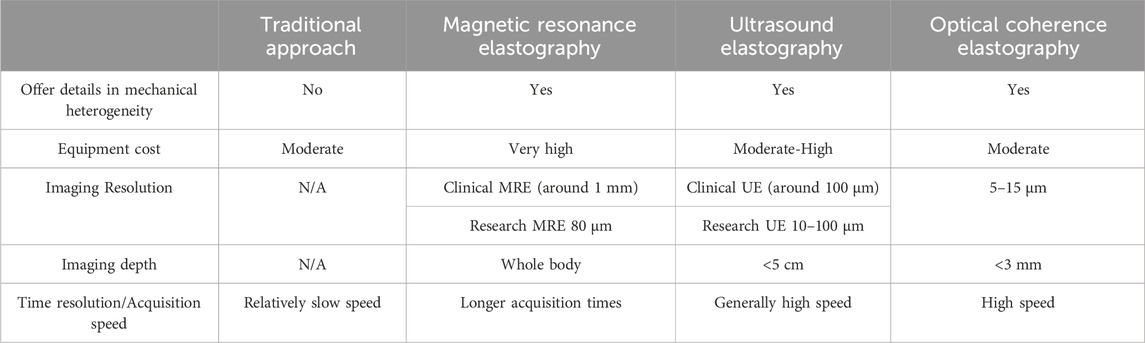
Table 1. Unique features of traditional approach, MRE, UE and OCE for measuring mechanical properties of tissue engineered grafts.
Furthermore, a 5%–10% strain is commonly used in measurements, could potentially compromise the tissue’s mechanical qualities and structure. To address this, systems like the BioDynamic system from TA Instruments have been designed to allow for online monitoring of the continuous growth of tissue grafts within sterile chambers. Additionally, some researchers have also developed bespoke systems that enable real-time monitoring of the mechanical properties of engineered tissue constructs (Ahearne et al., 2005; Kortsmit et al., 2009a). Despite their innovation, these systems have limitations, such as specific requirements for sample size or shape, and generally provide information on the bulk mechanical characteristics, lacking spatial heterogeneity throughout the three-dimensional structure.
Overall, imaging-based elastography possesses significant advantages over traditional approaches due to its capability to assess not only bulk mechanical properties but also the mechanical heterogeneity of engineered tissues (Table 2). The evaluation of such heterogeneity could serve as a crucial criterion for determining the readiness of a graft for implantation (Klein et al., 2009b; Vijayavenkataraman et al., 2018), since grafts lacking the native-like structural and mechanical heterogeneity may fail over long-term repair (Klein et al., 2009a; Khoshgoftar et al., 2013). Imaging methods can provide non-invasive assessment of the mechanical properties of engineered tissue constructs.
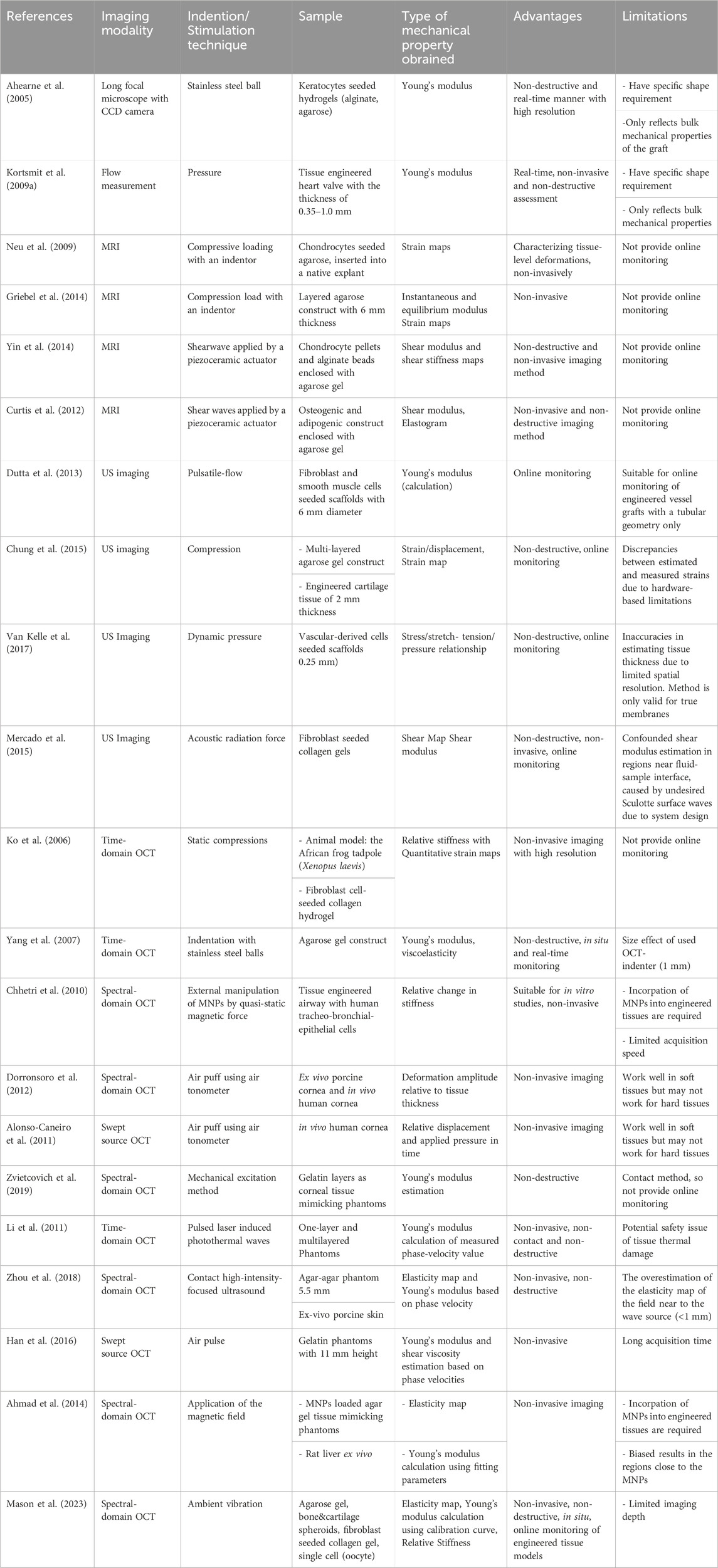
Table 2. Various approaches for online monitoring mechanical properties of tissue engineered grafts, acellular biomaterials and ex vivo tissues.
MRE itself is a nondestructive assessment method, and the excitation required for mechanical analysis can be induced by external stress or environmental vibrations. MRE has been successfully used to image shear viscoelastic properties of gel phantoms, tissue-engineered adipogenic and osteogenic (Othman et al., 2005) or chondrogenic constructs (Neu et al., 2009; Yin et al., 2014). While MRE or Microscopic MRE (μMRE) are effective, these methods may require embedding samples in hydrogels (Curtis et al., 2012; Yin et al., 2014) or direct contact with needles (Othman et al., 2005) for stress application. These requirements could limit their utility for continuous online imaging of tissue properties (Neu et al., 2009; Griebel et al., 2014).
UE systems providing online mechanical property assessment (Mercado et al., 2015) that can include viscoelastic properties analysis (Hong et al., 2016). This technique faces constraints in geometry (Dutta et al., 2013), hardware bringing on mismatches between measured and estimated strains (Chung et al., 2015) and limited spatial resolution (Van Kelle et al., 2017). While MRI and UE system are mainly used for medical purposes and are still expensive, OCT is commercially available at a moderate cost for researchers and industries (Ko et al., 2006), making it a more attractive option for tissue engineering applications. Furthermore, OCT offers higher resolutions than MRI and UI, at a few micrometres scale, making it a more suitable tool to monitor tissue and cellular mechanical properties. However, limited imaging depth (up to 3 mm in depth) remains a challenge for using OCE on large sized engineered tissues (Kennedy et al., 2017; Kim et al., 2016). Studies with OCE mostly cover mechanical property analysis of cornea or engineered tissue or tissue mimicking materials including viscoelasticity. The approach Han et al. developed has limitations to model based on assumptions about the sample such as sample shape (Han et al., 2017) or needed long acquisition time (Han et al., 2016). More efforts in extending the imaging depth and developing novel contactless excitation methods are needed to further advance the application of OCE in tissue engineering. To date, only a handful of studies have managed to build a sterile system for online monitoring mechanical properties of engineered tissues using either traditional or non-invasive imaging-based elastography approach. When designing a practical system for online monitoring stiffness of engineered tissues, one should consider the following four points: 1) The system should ensure the sterility of the culture, for example, using autoclavable parts or non-contact methods to induce mechanical deformation. 2) Ideally, it should also facilitate in situ measurement and avoiding manipulation of cultured samples, for example, avoiding moving samples in and out of culture dishes and/or incubators. 3) The system should be able to produce a quantitative readout of mechanical modulus, for example, Young’s modulus, instead of only strain values. 4) Finally, it should facilitate the measurement of spatial mechanical heterogeneity in addition to the bulk mechanical modulus.
In addition to the field of tissue engineering, non-contact UE and OCE may also present a useful tool for cancer study and in vitro drug screening. There has been an increasing emphasis in developing 3D tumour models for drug screening in pharmaceutical industries (Xu et al., 2014). It is well known that in diseased organs tumour tissues are stiffer than healthy tissues. Online monitoring of the stiffness of 3D tumour models may provide an insight to its growth and response to drug treatment. Furthermore, apart from monitoring in vitro cultures, imaging-based elastography may have a promising future for in vivo longitudinal assessment of engineered biomaterials and tissues (Park et al., 2014; Khalilzad-Sharghi et al., 2016). Traditionally, periodic animal sacrifices are needed for the longitudinal assessment of engineered grafts in repair site. The modalities discussed in this paper primarily focus on analyzing the mechanical properties of tissue-engineered grafts. However, it is crucial to note that the applicability of in vivo analysis is limited for these modalities due to various constraints. In the study conducted by Dorronsoro et al., deformation measurements were performed on the human cornea in vivo and on the porcine eye in vitro using OCT for imaging and an air puff for deformation (Dorronsoro et al., 2012). Meanwhile, Zhou et al. employed Surface Acoustic Waves for impulse generation and imaging on ex-vivo porcine skin, restricting the modality’s use to ex-vivo applications (Zhou et al., 2018). Dutta et al.'s system, designed for real-time imaging, has its own set of limitations. Its shape confines its suitability to monitoring artificial vascular grafts with tubular geometry exclusively. Furthermore, applying this modality to blood vessels in vivo proves challenging, as blood pressure is not precisely measured during imaging (Dutta et al., 2013). The non-invasive nature of MRE, UE and OCE may enable tissue engineers to online monitor the repair and changes in mechanical properties of implanted grafts and thus to reduce animal numbers needed for in vivo studies. For example, Yu et al. has used UE for non-invasive evaluation of the mechanical performance of polyurethane-based scaffolds in rat abdominal repair model over a 12-week period (Yu et al., 2013).
5 Conclusion
Real-time monitoring of the mechanical properties of engineered grafts is crucial for the field of tissue engineering. The benefits of imaging-based elastography over traditional techniques are emphasized in this article, along with its capacity to evaluate the mechanical heterogeneity of engineered tissues. The paper discusses several imaging modalities, emphasizing the advantages and disadvantages of OCE, UE, and MRE. Although MRE and UE systems provide good imaging, there may be issues with their cost, real-time capabilities, and spatial resolution. On the other hand, OCE offers an alternative because of its high resolution at the tissue and cell levels and its reasonably priced commercial availability. However, challenges such as limited imaging depth need to be addressed for larger tissue applications.
In conclusion, monitoring the mechanical properties of engineered grafts in real-time is a critical aspect of tissue engineering. This paper highlights the benefits of non-invasive, imaging-based elastography for this purpose. Additionally, it points out the potential applications of this technology in various areas, including cancer study, drug screening, and the in vivo assessment of tissue-engineered grafts.
Author contributions
LL: Writing–original draft. KO: Writing–original draft. PB: Writing–review and editing. AE: Writing–review and editing.
Funding
The author(s) declare that financial support was received for the research, authorship, and/or publication of this article. Funding was provided by Republic of Turkiye Ministry of National Education, EPSRC (EP/PO31137/1 and EP/PO31250/1), MRC UKRMP Hub: Engineered Cell Environment (# 623924) and H2020 ERC Advanced Grant: Dynaceutics (# 638836).
Acknowledgments
The authors gratefully acknowledge the generous support provided by our funders.
Conflict of interest
Author LL was employed by Suzhou Microport Regenerative Medicine Technologies Co., Ltd.
The remaining authors declare that the research was conducted in the absence of any commercial or financial relationships that could be construed as a potential conflict of interest.
Publisher’s note
All claims expressed in this article are solely those of the authors and do not necessarily represent those of their affiliated organizations, or those of the publisher, the editors and the reviewers. Any product that may be evaluated in this article, or claim that may be made by its manufacturer, is not guaranteed or endorsed by the publisher.
References
Ahearne, M., Yang, Y., El Haj, A. J., Then, K. Y., and Liu, K.-K. (2005). Characterizing the viscoelastic properties of thin hydrogel-based constructs for tissue engineering applications. J. R. Soc. Interface 2 (5), 455–463. doi:10.1098/rsif.2005.0065
Ahmad, A., Kim, J., Sobh, N. A., Shemonski, N. D., and Boppart, S. A. (2014). Magnetomotive optical coherence elastography using magnetic particles to induce mechanical waves. Biomed. Opt. Express 5 (7), 2349–2361. doi:10.1364/BOE.5.002349
Alonso-Caneiro, D., Karnowski, K., Kaluzny, B. J., Kowalczyk, A., and Wojtkowski, M. (2011). Assessment of corneal dynamics with high-speed swept source optical coherence tomography combined with an air puff system. Opt. Express 19 (15), 14188–14199. doi:10.1364/OE.19.014188
Asbach, P., Klatt, D., Hamhaber, U., Braun, J., Somasundaram, R., Hamm, B., et al. (2008). Assessment of liver viscoelasticity using multifrequency MR elastography. Magnetic Reson. Med. An Official J. Int. Soc. Magnetic Reson. Med. 60 (2), 373–379. doi:10.1002/mrm.21636
Bagnaninchi, P. O., Yang, Y., Zghoul, N., Maffulli, N., Wang, R. K., and El Haj, A. J. (2007). Chitosan microchannel scaffolds for tendon tissue engineering characterized using optical coherence tomography. Tissue Eng. 13 (2), 323–331. doi:10.1089/ten.2006.0168
Barr, R. G., Nakashima, K., Amy, D., Cosgrove, D., Farrokh, A., Schafer, F., et al. (2015). WFUMB guidelines and recommendations for clinical use of ultrasound elastography: Part 2: breast. Ultrasound Med. Biol. 41 (5), 1148–1160. doi:10.1016/j.ultrasmedbio.2015.03.008
Bohte, A. E., Nelissen, J. L., Runge, J. H., Holub, O., Lambert, S. A., de Graaf, L., et al. (2018). Breast magnetic resonance elastography: a review of clinical work and future perspectives. NMR Biomed. 31 (10), e3932. doi:10.1002/nbm.3932
Cao, H., Li, Z., Chen, Y., Zhu, J., Chen, M., Lei, H., et al. (2023). Viscoelasticity microenvironment constructed by self-crosslinking hyaluronan hybrid hydrogels regulates chondrogenic differentiation of mesenchymal stem cells. Compos. Part B Eng. 263, 110871. doi:10.1016/j.compositesb.2023.110871
Catheline, S., Souchon, R., Rupin, M., Brum, JDAH, Dinh, A. H., and Chapelon, J.-Y. (2013). Tomography from diffuse waves: passive shear wave imaging using low frame rate scanners. Appl. Phys. Lett. 103 (1). doi:10.1063/1.4812515
Chernak Slane, L., and Thelen, D. G. (2014). The use of 2D ultrasound elastography for measuring tendon motion and strain. J. Biomech. 47 (3), 750–754. doi:10.1016/j.jbiomech.2013.11.023
Chhetri, R. K., Carpenter, J., Superfine, R., Randell, S. H., and Oldenburg, A. L. (2010). Magnetomotive optical coherence elastography for relating lung structure and function in Cystic Fibrosis. Proc. SPIE Int. Soc. Opt. Eng. 7554, 755420. doi:10.1117/12.840592
Chino, K., Akagi, R., Dohi, M., Fukashiro, S., and Takahashi, H. (2012). Reliability and validity of quantifying absolute muscle hardness using ultrasound elastography. PLoS One 7 (9), e45764. doi:10.1371/journal.pone.0045764
Chung, C. Y., Heebner, J., Baskaran, H., Welter, J. F., and Mansour, J. M. (2015). Ultrasound elastography for estimation of regional strain of multilayered hydrogels and tissue-engineered cartilage. Ann. Biomed. Eng. 43 (12), 2991–3003. doi:10.1007/s10439-015-1356-x
Cortes, I., Matsui, R. A. M., Azevedo, M. S., Beatrici, A., Souza, K. L. A., Launay, G., et al. (2019). A scaffold- and serum-free method to mimic human stable cartilage validated by secretome. Tissue Eng. Part A 27, 311–327. doi:10.1089/ten.TEA.2018.0311
Cosgrove, D., Piscaglia, F., Bamber, J., Bojunga, J., Correas, J.-M., Gilja, O. H. al, et al. (2013). EFSUMB guidelines and recommendations on the clinical use of ultrasound elastography. Part 2: clinical applications. Ultraschall der Medizin-European J. Ultrasound 34 (03), 238–253. doi:10.1055/s-0033-1335375
Cosgrove, D. O., Berg, W. A., Doré, C. J., Skyba, D. M., Henry, J.-P., Gay, J., et al. (2012). Shear wave elastography for breast masses is highly reproducible. Eur. Radiol. 22 (5), 1023–1032. doi:10.1007/s00330-011-2340-y
Curtis, E. T., Zhang, S., Khalilzad-Sharghi, V., Boulet, T., and Othman, S. F. (2012). Magnetic resonance elastography methodology for the evaluation of tissue engineered construct growth. J. Vis. Exp. 60, 3618. doi:10.3791/3618
Dalecki, D., Mercado, K. P., and Hocking, D. C. (2016). Quantitative ultrasound for nondestructive characterization of engineered tissues and biomaterials. Ann. Biomed. Eng. 44 (3), 636–648. doi:10.1007/s10439-015-1515-0
de Korte, C. L., Hansen, H. H., and van der Steen, A. F. (2011). Vascular ultrasound for atherosclerosis imaging. Interface Focus 1 (4), 565–575. doi:10.1098/rsfs.2011.0024
Dorronsoro, C., Pascual, D., Perez-Merino, P., Kling, S., and Marcos, S. (2012). Dynamic OCT measurement of corneal deformation by an air puff in normal and cross-linked corneas. Biomed. Opt. Express 3 (3), 473–487. doi:10.1364/BOE.3.000473
Drakonaki, E. E., Allen, G. M., and Wilson, D. J. (2009). Real-time ultrasound elastography of the normal Achilles tendon: reproducibility and pattern description. Clin. Radiol. 64 (12), 1196–1202. doi:10.1016/j.crad.2009.08.006
Dutta, D., Lee, K. W., Allen, R. A., Wang, Y., Brigham, J. C., and Kim, K. (2013). Non-invasive assessment of elastic modulus of arterial constructs during cell culture using ultrasound elasticity imaging. Ultrasound Med. Biol. 39 (11), 2103–2115. doi:10.1016/j.ultrasmedbio.2013.04.023
El, H., Alicia, J., Wood, M. A., Thomas, P., and Yang, Y. (2005). Controlling cell biomechanics in orthopaedic tissue engineering and repair. Pathol. Biol. 53 (10), 581–589. doi:10.1016/j.patbio.2004.12.002
Farmakis, S. G., Buchanan, P. M., Guzman, M. A., Hardy, A. K., Jain, A. K., and Teckman, J. H. (2019). Shear wave elastography correlates with liver fibrosis scores in pediatric patients with liver disease. Pediatr. Radiol. 49 (13), 1742–1753. doi:10.1007/s00247-019-04493-3
Freeman, F. E., Browe, D. C., Nulty, J., Von Euw, S., Grayson, W. L., and Kelly, D. J. (2019). Biofabrication of multiscale bone extracellular matrix scaffolds for bone tissue engineering. Eur. Cell Mater 38, 168–187. doi:10.22203/eCM.v038a12
Green, M. A., Bilston, L. E., and Sinkus, R. (2008). In vivo brain viscoelastic properties measured by magnetic resonance elastography. NMR Biomed. an Int. J. Devoted Dev. Appl. Magnetic Reson. vivo 21 (7), 755–764. doi:10.1002/nbm.1254
Griebel, A. J., Khoshgoftar, M., Novak, T., van Donkelaar, C. C., and Neu, C. P. (2014). Direct noninvasive measurement and numerical modeling of depth-dependent strains in layered agarose constructs. J. Biomech. 47 (9), 2149–2156. doi:10.1016/j.jbiomech.2013.09.025
Guan, G., Li, C., Ling, Y., Yang, Y., Vorstius, J. B., Keatch, R. P., et al. (2013). Quantitative evaluation of degenerated tendon model using combined optical coherence elastography and acoustic radiation force method. J. Biomed. Opt. 18 (11), 111417. doi:10.1117/1.Jbo.18.11.111417
Gupta, S., Kumar Teotia, A., Qayoom, I., Ahmad Shiekh, P., Andrabi, S. M., and Kumar, A. (2021). Periosteum-mimicking tissue-engineered composite for treating periosteum damage in critical-sized bone defects. Biomacromolecules 22 (8), 3237–3250. doi:10.1021/acs.biomac.1c00319
Han, Z., Li, J., Singh, M., Wu, C., Liu, C.-hao, Raghunathan, R., et al. (2017). Optical coherence elastography assessment of corneal viscoelasticity with a modified Rayleigh-Lamb wave model. J. Mech. Behav. Biomed. Mater. 66, 87–94. doi:10.1016/j.jmbbm.2016.11.004
Han, Z., Singh, M., Aglyamov, S. R., Liu, C. H., Nair, A., Raghunathan, R., et al. (2016). Quantifying tissue viscoelasticity using optical coherence elastography and the Rayleigh wave model. J. Biomed. Opt. 21 (9), 090504. doi:10.1117/1.Jbo.21.9.090504
Holmes, C., Tabrizian, M., and Bagnaninchi, P. O. (2015). Motility imaging via optical coherence phase microscopy enables label-free monitoring of tissue growth and viability in 3D tissue-engineering scaffolds. J. tissue Eng. Regen. Med. 9 (5), 641–645. doi:10.1002/term.1687
Hong, X., Jan, P. S., and Deng, C. X. (2016). Microscale characterization of the viscoelastic properties of hydrogel biomaterials using dual-mode ultrasound elastography. Biomaterials 88, 12–24. doi:10.1016/j.biomaterials.2016.02.019
Huang, D., Swanson, E. A., Lin, C. P., Schuman, J. S., Stinson, W. G., Chang, W., et al. (1991). Optical coherence tomography. Sci. (New York, N.Y.) 254 (5035), 1178–1181. doi:10.1126/science.1957169
Kennedy, B. F., Liang, X., Adie, S. G., Gerstmann, D. K., Quirk, B. C., Boppart, S. A., et al. (2011). In vivo three-dimensional optical coherence elastography. Opt. Express 19 (7), 6623–6634. doi:10.1364/OE.19.006623
Kennedy, B. F., Wijesinghe, P., and Sampson, D. D. (2017). The emergence of optical elastography in biomedicine. Nat. Photonics 11 (4), 215–221. doi:10.1038/nphoton.2017.6
Kennedy, K. M., McLaughlin, R. A., Kennedy, B. F., Tien, A., Latham, B., Saunders, C. M., et al. (2013). Needle optical coherence elastography for the measurement of microscale mechanical contrast deep within human breast tissues. J. Biomed. Opt. 18 (12), 121510. doi:10.1117/1.JBO.18.12.121510
Khalilzad-Sharghi, V., Han, Z., Xu, H., and Othman, S. F. (2016). MR elastography for evaluating regeneration of tissue-engineered cartilage in an ectopic mouse model. Magn. Reson Med. 75 (3), 1209–1217. doi:10.1002/mrm.25745
Khoshgoftar, M., Wilson, W., Ito, K., and van Donkelaar, C. C. (2013). The effect of tissue-engineered cartilage biomechanical and biochemical properties on its post-implantation mechanical behavior. Biomechanics Model. Mechanobiol. 12 (1), 43–54. doi:10.1007/s10237-012-0380-0
Kim, K., and Wagner, W. R. (2016). Non-invasive and non-destructive characterization of tissue engineered constructs using ultrasound imaging technologies: a review. Ann. Biomed. Eng. 44 (3), 621–635. doi:10.1007/s10439-015-1495-0
Kim, W., Ferguson, V. L., Borden, M., and Neu, C. P. (2016). Application of elastography for the noninvasive assessment of biomechanics in engineered biomaterials and tissues. Ann. Biomed. Eng. 44 (3), 705–724. doi:10.1007/s10439-015-1542-x
Klatt, D., Hamhaber, U., Asbach, P., Braun, J., and Sack, I. (2007). Noninvasive assessment of the rheological behavior of human organs using multifrequency MR elastography: a study of brain and liver viscoelasticity. Phys. Med. Biol. 52 (24), 7281–7294. doi:10.1088/0031-9155/52/24/006
Klein, T. J., Malda, J., Sah, R. L., and Hutmacher, D. W. (2009a). Tissue engineering of articular cartilage with biomimetic zones. Tissue Eng. Part B Rev. 15 (2), 143–157. doi:10.1089/ten.TEB.2008.0563
Klein, T. J., Rizzi, S. C., Reichert, J. C., Georgi, N., Malda, J., Schuurman, W., et al. (2009b). Strategies for zonal cartilage repair using hydrogels. Macromol. Biosci. 9 (11), 1049–1058. doi:10.1002/mabi.200900176
Ko, H. J., Tan, W., Stack, R., and Boppart, S. A. (2006). Optical coherence elastography of engineered and developing tissue. Tissue Eng. 12 (1), 63–73. doi:10.1089/ten.2006.12.63
Kortsmit, J., Driessen, N. J., Rutten, M. C., and Baaijens, F. P. (2009a). Nondestructive and noninvasive assessment of mechanical properties in heart valve tissue engineering. Tissue Eng. Part A 15 (4), 797–806. doi:10.1089/ten.tea.2008.0197
Kortsmit, J., Rutten, M. C., Wijlaars, M. W., and Baaijens, F. P. (2009b). Deformation-controlled load application in heart valve tissue engineering. Tissue Eng. Part C Methods 15 (4), 707–716. doi:10.1089/ten.TEC.2008.0658
Larin, K. V., and Sampson, D. D. (2017). Optical coherence elastography - OCT at work in tissue biomechanics [Invited]. Biomed. Opt. Express 8 (2), 1172–1202. doi:10.1364/BOE.8.001172
Li, C., Huang, Z., and Wang, R. K. (2011). Elastic properties of soft tissue-mimicking phantoms assessed by combined use of laser ultrasonics and low coherence interferometry. Opt. Express 19 (11), 10153–10163. doi:10.1364/OE.19.010153
Li, Y., Moon, S., Chen, J. J., Zhu, Z., and Chen, Z. (2020). Ultrahigh-sensitive optical coherence elastography. Light Sci. Appl. 9 (1), 58. doi:10.1038/s41377-020-0297-9
Liang, X., Graf, B. W., and Boppart, S. A. (2009). Imaging engineered tissues using structural and functional optical coherence tomography. J. Biophot. 2 (11), 643–655. doi:10.1002/jbio.200910048
Liu, H.-C., Gaihre, B., Kijanka, P., Lu, L., and Urban, M. W. (2023). Acoustic force elastography microscopy. IEEE Trans. Biomed. Eng. 70 (3), 841–852. doi:10.1109/tbme.2022.3203435
Liu, H.-C., Kijanka, P., and Urban, M. W. (2021). Two-dimensional (2D) dynamic vibration optical coherence elastography (DV-OCE) for evaluating mechanical properties: a potential application in tissue engineering. Biomed. Opt. Express 12 (3), 1217–1235. doi:10.1364/boe.416661
Lopez, O., Amrami, K. K., Manduca, A., Rossman, P. J., and Ehman, R. L. (2007). Developments in dynamic MR elastography for in vitro biomechanical assessment of hyaline cartilage under high-frequency cyclical shear. J. Magn. Reson Imaging 25 (2), 310–320. doi:10.1002/jmri.20857
Luo, L., Thorpe, S. D., Buckley, C. T., and Kelly, D. J. (2015). The effects of dynamic compression on the development of cartilage grafts engineered using bone marrow and infrapatellar fat pad derived stem cells. Biomed. Mater 10 (5), 055011. doi:10.1088/1748-6041/10/5/055011
Manduca, A., Oliphant, T. E., Dresner, M. A., Mahowald, J. L., Kruse, S. A., Amromin, E., et al. (2001). Magnetic resonance elastography: non-invasive mapping of tissue elasticity. Med. Image Anal. 5 (4), 237–254. doi:10.1016/S1361-8415(00)00039-6
Mariappan, Y. K., Glaser, K. J., and Ehman, R. L. (2010). Magnetic resonance elastography: a review. Clin. Anat. 23 (5), 497–511. doi:10.1002/ca.21006
Martucci, N. J., Morgan, K., Anderson, G. W., Hayes, P. C., Plevris, J. N., Nelson, L. J., et al. (2018). Nondestructive optical toxicity assays of 3D liver spheroids with optical coherence tomography. Adv. Biosyst. 2 (3), 1700212. doi:10.1002/adbi.201700212
Mason, J. H., Lu, L., Reinwald, Y., Taffetani, M., Hallas-Potts, A., Simon Herrington, C., et al. (2023). Debiased ambient vibrations optical coherence elastography to profile cell, organoid and tissue mechanical properties. Commun. Biol. 6 (1), 543. doi:10.1038/s42003-023-04788-0
McCormick, M., Varghese, T., Wang, X., Mitchell, C., Kliewer, M. A., and Dempsey, R. J. (2012). Methods for robust in vivo strain estimation in the carotid artery. Phys. Med. Biol. 57 (22), 7329–7353. doi:10.1088/0031-9155/57/22/7329
McKnight, A. L., Kugel, J. L., Rossman, P. J., Manduca, A., Hartmann, L. C., and Ehman, R. L. (2002). MR elastography of breast cancer: preliminary results. AJR Am. J. Roentgenol. 178 (6), 1411–1417. doi:10.2214/ajr.178.6.1781411
Mercado, K. P., Langdon, J., Helguera, M., McAleavey, S. A., Hocking, D. C., and Dalecki, D. (2015). Scholte wave generation during single tracking location shear wave elasticity imaging of engineered tissues. J. Acoust. Soc. Am. 138 (2), EL138–44. doi:10.1121/1.4927633
Miller, D. L., Abo, A., Abramowicz, J. S., Bigelow, T. A., Dalecki, D., Dickman, E., et al. (2020). Diagnostic ultrasound safety review for point-of-care ultrasound practitioners. J. Ultrasound Med. 39 (6), 1069–1084. doi:10.1002/jum.15202
Neu, C. P., Arastu, H. F., Curtiss, S., and Reddi, A. H. (2009). Characterization of engineered tissue construct mechanical function by magnetic resonance imaging. J. Tissue Eng. Regen. Med. 3 (6), 477–485. doi:10.1002/term.188
Nguyen, T.-M., Ali, Z., Lescanne, M., Boccara, C., Fink, M., and Catheline, S. (2016). Diffuse shear wave imaging: toward passive elastography using low-frame rate spectral-domain optical coherence tomography. J. Biomed. Opt. 21 (12), 126013. doi:10.1117/1.jbo.21.12.126013
Nguyen, T.-M., Aubry, J.-F., Touboul, D., Fink, M., Gennisson, J.-L., Bercoff, J., et al. (2012). Monitoring of cornea elastic properties changes during UV-A/Riboflavin-Induced corneal collagen cross-linking using supersonic shear wave imaging: a pilot study. Investigative Ophthalmol. Vis. Sci. 53 (9), 5948–5954. doi:10.1167/iovs.11-9142
Othman, S. F., Curtis, E. T., Plautz, S. A., Pannier, A. K., Butler, S. D., and Xu, H. (2012). MR elastography monitoring of tissue-engineered constructs. NMR Biomed. 25 (3), 452–463. doi:10.1002/nbm.1663
Othman, S. F., Xu, H., and Mao, J. J. (2015). Future role of MR elastography in tissue engineering and regenerative medicine. J. Tissue Eng. Regen. Med. 9 (5), 481–487. doi:10.1002/term.1801
Othman, S. F., Xu, H., Royston, T. J., and Magin, R. L. (2005). Microscopic magnetic resonance elastography (μMRE). Magnetic Reson. Med. an official J. Int. Soc. Magnetic Reson. Med. 54 (3), 605–615. doi:10.1002/mrm.20584
Park, D. W., Ye, S. H., Jiang, H. B., Dutta, D., Nonaka, K., Wagner, W. R., et al. (2014). In vivo monitoring of structural and mechanical changes of tissue scaffolds by multi-modality imaging. Biomaterials 35 (27), 7851–7859. doi:10.1016/j.biomaterials.2014.05.088
Razani, M., Luk, T. W., Mariampillai, A., Siegler, P., Kiehl, T. R., Kolios, M. C., et al. (2014). Optical coherence tomography detection of shear wave propagation in inhomogeneous tissue equivalent phantoms and ex-vivo carotid artery samples. Biomed. Opt. Express 5 (3), 895–906. doi:10.1364/BOE.5.000895
Rosario, D. J., Reilly, G. C., Ali Salah, E., Glover, M., Bullock, A. J., and MacNeil, S. (2008). Decellularization and sterilization of porcine urinary bladder matrix for tissue engineering in the lower urinary tract. Regen. Med. 3 (2), 145–156. doi:10.2217/17460751.3.2.145
Sander, E. A., Lynch, K. A., and Boyce, S. T. (2014). Development of the mechanical properties of engineered skin substitutes after grafting to full-thickness wounds. J. Biomech. Eng. 136 (5), 051008. doi:10.1115/1.4026290
Sharma, P., Cartmell, S., and El Haj, A. J. (2005). “Bone tissue engineering,” in Applications of cell immobilisation biotechnology. Editors V. Nedović, and R. Willaert (Dordrecht: Springer Netherlands), 153–166.
Shire, N. J., Yin, M., Chen, J., Railkar, R. A., Fox-Bosetti, S., Johnson, S. M., et al. (2011). Test-retest repeatability of MR elastography for noninvasive liver fibrosis assessment in hepatitis C. J. Magn. Reson Imaging 34 (4), 947–955. doi:10.1002/jmri.22716
Singh, M., Han, Z., Nair, A., Schill, A., Twa, M. D., and Larin, K. V. (2017). Applanation optical coherence elastography: noncontact measurement of intraocular pressure, corneal biomechanical properties, and corneal geometry with a single instrument. J. Biomed. Opt. 22, 1. doi:10.1117/1.jbo.22.2.020502
Tejo-Otero, A., Fenollosa-Artés, F., Achaerandio, I., Rey-Vinolas, S., Buj-Corral, I., Mateos-Timoneda, M. Á., et al. (2022). Soft-tissue-mimicking using hydrogels for the development of phantoms. Gels 8 (1), 40. doi:10.3390/gels8010040
Thitaikumar, A., Mobbs, L. M., Kraemer-Chant, C. M., Garra, B. S., and Ophir, J. (2008). Breast tumor classification using axial shear strain elastography: a feasibility study. Phys. Med. Biol. 53 (17), 4809–4823. doi:10.1088/0031-9155/53/17/022
Thittai, A. K., Yamal, J. M., Mobbs, L. M., Kraemer-Chant, C. M., Chekuri, S., Garra, B. S., et al. (2011). Axial-shear strain elastography for breast lesion classification: further results from in vivo data. Ultrasound Med. Biol. 37 (2), 189–197. doi:10.1016/j.ultrasmedbio.2010.11.001
Trommelmans, L., Selling, J., and Dierickx, K. (2008). Ethical reflections on clinical trials with human tissue engineered products. J. Med. Ethics 34 (9), e1. doi:10.1136/jme.2007.022913
Twa, M. D., Lan, G., Aglyamov, S., and Larin, K. (2019). Clinical application of optical coherence elastography for corneal biomechanics. Investigative Ophthalmol. Vis. Sci. 60 (9), 6828.
Van Kelle, M. A. J., Oomen, P. J. A., Bulsink, J. A., Janssen-van den Broek, M., Lopata, R. G. P., Rutten, M. C. M., et al. (2017). A bioreactor to identify the driving mechanical stimuli of tissue growth and remodeling. Tissue Eng. Part C Methods 23 (6), 377–387. doi:10.1089/ten.TEC.2017.0141
Van Reeth, E., Tham, I. W. K., Tan, C. H., and Poh, C. L. (2012). Super-resolution in magnetic resonance imaging: a review. Concepts Magnetic Reson. Part A 40A (6), 306–325. doi:10.1002/cmr.a.21249
Vijayavenkataraman, S., Yan, W.-C., Lu, W. F., Wang, C.-H., and Fuh, J. Y. H. (2018). 3D bioprinting of tissues and organs for regenerative medicine. Adv. Drug Deliv. Rev. 132, 296–332. doi:10.1016/j.addr.2018.07.004
Wang, S., and Larin, K. V. (2015). Optical coherence elastography for tissue characterization: a review. J. Biophot. 8 (4), 279–302. doi:10.1002/jbio.201400108
Wang, S., Larin, K. V., Li, J., Vantipalli, S., Manapuram, R. K., Aglyamov, S., et al. (2013). A focused air-pulse system for optical-coherence-tomography-based measurements of tissue elasticity. Laser Phys. Lett. 10 (7), 075605. doi:10.1088/1612-2011/10/7/075605
Webb, W. R., Dale, T. P., Lomas, A. J., Zeng, G., Wimpenny, I., El Haj, A. J., et al. (2013). The application of poly(3-hydroxybutyrate-co-3-hydroxyhexanoate) scaffolds for tendon repair in the rat model. Biomaterials 34 (28), 6683–6694. doi:10.1016/j.biomaterials.2013.05.041
Xu, X., Farach-Carson, M. C., and Jia, X. (2014). Three-dimensional in vitro tumor models for cancer research and drug evaluation. Biotechnol. Adv. 32 (7), 1256–1268. doi:10.1016/j.biotechadv.2014.07.009
Yanagisawa, O., Niitsu, M., Kurihara, T., and Fukubayashi, T. (2011). Evaluation of human muscle hardness after dynamic exercise with ultrasound real-time tissue elastography: a feasibility study. Clin. Radiol. 66 (9), 815–819. doi:10.1016/j.crad.2011.03.012
Yang, Y., Bagnaninchi, P. O., Ahearne, M., Wang, R. K., and Liu, K.-K. (2007). A novel optical coherence tomography-based micro-indentation technique for mechanical characterization of hydrogels. J. R. Soc. Interface 4 (17), 1169–1173. doi:10.1098/rsif.2007.1044
Yang, Y., Dubois, A., Qin, X.-pei, Li, J., El Haj, A., and Wang, R. K. (2006). Investigation of optical coherence tomography as an imaging modality in tissue engineering. Phys. Med. Biol. 51 (7), 1649–1659. doi:10.1088/0031-9155/51/7/001
Yang, Y., Wang, R., Guyot, E., El Haj, A., and Dubois, A. (2005). Application of optical coherence tomography for tissue engineering. Vol. , 5690, SPIE BiOS: SPIE. doi:10.1117/12.592691
Yin, Z., Schmid, T. M., Yasar, T. K., Liu, Y., Royston, T. J., and Magin, R. L. (2014). Mechanical characterization of tissue-engineered cartilage using microscopic magnetic resonance elastography. Tissue Eng. Part C Methods 20 (8), 611–619. doi:10.1089/ten.TEC.2013.0408
Yu, J., Takanari, K., Hong, Y., Lee, K. W., Amoroso, N. J., Wang, Y., et al. (2013). Non-invasive characterization of polyurethane-based tissue constructs in a rat abdominal repair model using high frequency ultrasound elasticity imaging. Biomaterials 34 (11), 2701–2709. doi:10.1016/j.biomaterials.2013.01.036
Zhang, Y. N., Fowler, K. J., Ozturk, A., Potu, C. K., Louie, A. L., Montes, V., et al. (2020). Liver fibrosis imaging: a clinical review of ultrasound and magnetic resonance elastography. J. Magn. Reson Imaging 51 (1), 25–42. doi:10.1002/jmri.26716
Zhou, K., Le, N., Huang, Z., and Li, C. (2018). High-intensity-focused ultrasound and phase-sensitive optical coherence tomography for high resolution surface acoustic wave elastography. J. Biophot. 11 (2). doi:10.1002/jbio.201700051
Keywords: mechanical testing, elastography, stiffness, tissue engineering, magnetic resonance imaging, ultrasound imaging, optical coherence tomography
Citation: Luo L, Okur KE, Bagnaninchi PO and El Haj AJ (2024) Current challenges in imaging the mechanical properties of tissue engineered grafts. Front. Biomater. Sci. 3:1323763. doi: 10.3389/fbiom.2024.1323763
Received: 18 October 2023; Accepted: 12 April 2024;
Published: 10 May 2024.
Edited by:
Kathryn Stok, The University of Melbourne, AustraliaReviewed by:
Karanvir Saini, University of Pennsylvania, United StatesValeria Graceffa, Institute of Technology, Ireland
Copyright © 2024 Luo, Okur, Bagnaninchi and El Haj. This is an open-access article distributed under the terms of the Creative Commons Attribution License (CC BY). The use, distribution or reproduction in other forums is permitted, provided the original author(s) and the copyright owner(s) are credited and that the original publication in this journal is cited, in accordance with accepted academic practice. No use, distribution or reproduction is permitted which does not comply with these terms.
*Correspondence: Pierre O. Bagnaninchi, cGllcnJlLmJhZ25hbmluY2hpQGVkLmFjLnVr; Alicia J. El Haj, YS5lbGhhakBiaGFtLmFjLnVr
†These authors have contributed equally to this work and share first authorship