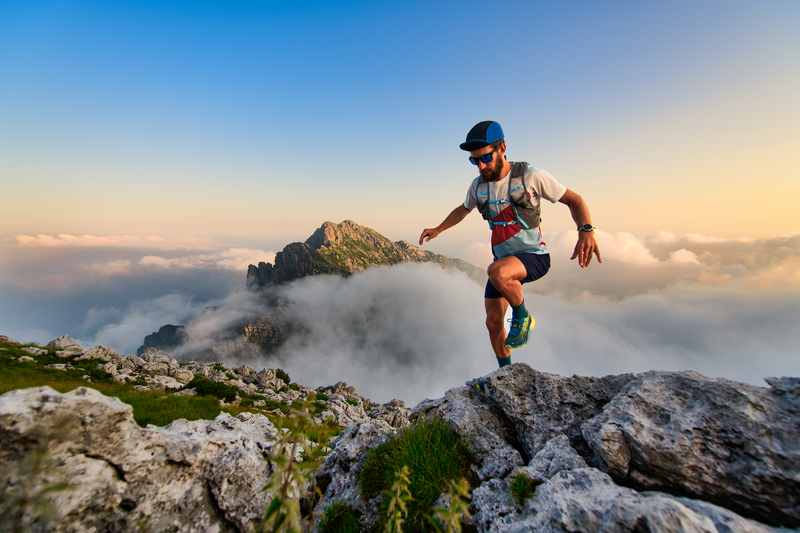
95% of researchers rate our articles as excellent or good
Learn more about the work of our research integrity team to safeguard the quality of each article we publish.
Find out more
REVIEW article
Front. Biomater. Sci. , 07 December 2023
Sec. Delivery Systems and Controlled Release
Volume 2 - 2023 | https://doi.org/10.3389/fbiom.2023.1305379
This article is part of the Research Topic Women in Biomaterials Science 2023 View all 12 articles
Sepsis is a common and life-threatening disorder with an alarmingly high mortality rate. Unfortunately, this rate has not decreased significantly over the last decade and the number of septic cases is increasing each year. Despite sepsis affecting millions of people annually, there is still not an established standard of care. The development of a therapy that targets the thromboinflammation characteristic of sepsis is imperative. Until recently, research has focused on uncovering individual pathways to target. As more of the pathophysiology of sepsis has become understood and more biomarkers uncovered, the interplay between endothelial cells, platelets, and leukocytes has emerged as a critical event. Therefore, a multi-targeted approach is clearly required for designing an effective treatment for sepsis. The versatility of biomaterials offers a promising solution in that they can be designed to target and affect multiple pathways and systems and safely inhibit excessive inflammation while maintaining hemostasis. Already, studies have demonstrated the ability of biomaterials to target different processes and stages in sepsis-induced inflammation and coagulopathy. Moreover, some biomaterials offer inherent anti-inflammatory and hemostatic qualities. This review aims to discuss the most recent advancements in biomaterial development designed to address inflammation, coagulopathy, and thromboinflammation.
Sepsis is as life-threatening host response to infection, characterized by organ dysfunction (Semeraro et al., 2010; Angus and Poll, 2012; Singer et al., 2016), and this condition is estimated to cause almost 50 million cases globally each year (WHO, 2023). The causes for sepsis include any infectious organisms: bacteria, fungi, viruses, and parasites can all lead to sepsis (Rudd et al., 2020). Sepsis is most commonly caused by bacterial pneumonia, but intra-abdominal and urinary tract infections are also frequent underlying causes (Angus and Poll, 2012). These infections lead to uncontrolled inflammation, resulting in tissue damage and coagulopathy, organ dysfunction, and even death. People who are immunocompromised, such as babies, geriatric populations, and those on immunosuppressive treatments such as chemotherapy, are most at risk (Angus and Poll, 2012; Rudd et al., 2020; Weiss et al., 2020). The respiratory and cardiovascular systems are most severely affected. Clinical signs include difficulty breathing and low blood pressure. Current treatment strategies depend on the individual and their clinical presentation, so a standard of care has not yet been established. Treatment often involves rescuing the cardiovascular and pulmonary systems through mechanical ventilation, vasopressor administration, and obtaining cultures to guide antimicrobial therapy (Angus and Poll, 2012; Weiss et al., 2020). Despite intensive treatment, sepsis remains a significant health concern, accounting for nearly 20% of all global deaths (WHO, 2023), with a high mortality rate of approximately 33% (Bauer et al., 2020). In the United States alone, hospitals spend over $24 billion each year on septic patient care (Rudd et al., 2020). Over the last decade, mortality rates have not lowered significantly (40% in 2009% vs. 34% in 2019) (Bauer et al., 2020) and the frequency of septic cases has risen every year by 13% on average (Tanne et al., 2002; Gaieski et al., 2013). These trends most likely result from increased life expectancies and the rise of antimicrobial resistance, both of which may contribute to sepsis becoming more difficult to treat (Cánovas-Cervera et al., 2023). Moreover, the relatively stagnant mortality rates in sepsis reflect the lack of significant advancements in therapeutics. Biomaterials and the design of multi-targeted therapeutics offer promising solutions to treating this complex disorder.
Biomaterials are most frequently considered for use as drug delivery vehicles with the aims of improving drug stability, prolonging blood circulation, and controlling spatiotemporal drug release to enhance local effects while minimizing systemic side effects. Accomplishing these goals ultimately improves patient outcomes and reduces drug-related toxicities and coagulopathies. Biomaterials offer inherent benefits based on their physical properties. For instance, silver is a natural antimicrobial, and when used as nanoparticles, it can amplify the antibacterial effects of antibiotics (Sun and Gupta, 2020; Vishwanath et al., 2022). This synergy allows for the use of lower antibiotic doses while achieving effective antibacterial responses. Biomaterials can be manipulated extensively to address several different diseases of different bodily systems as well. Material composition, size, shape, charge, molecular weight, and flexibility can all be optimized for specific purposes. With inflammation, nano-sized biomaterials can take advantage of the enhanced permeability and passively accumulate in inflamed tissues. For active targeting, ligands can be conjugated to biomaterials for selective binding. As for drug release, biomaterials can be designed to respond to changes in pH, temperature, shear forces, enzymes, radiation, and even ultrasonography and magnetic forces. For example, superparamagnetic iron oxide nanoparticles (SPIONs) have frequently been studied for drug delivery since they can be directed to specific areas for targeted drug delivery (Friedrich et al., 2021). Given these possibilities, the development of a biomaterial capable of effectively treating severe sepsis and associated thromboinflammation is imminent.
This review delves into investigations of state-of-the-art biomaterials and the challenges in the development of biomaterials for sepsis-induced thromboinflammation, as well as inflammation and hemostasis individually. Anti-inflammatory biomaterials have historically been engineered from either synthetic materials or cells. Excitingly, recent innovative biomaterials incorporate both synthetic and cellular components. Biomaterials addressing coagulopathy have also expanded to reflect that coagulation is a dynamic process involving both clot formation and lysis. This review will examine biomaterials for modulating the different stages of coagulation, including furthering hemostasis, preventing thrombosis, and enhancing fibrinolysis. Lastly, investigations into multifunctional therapeutic biomaterials for sepsis-induced thromboinflammation will be discussed.
The characteristic uncontrolled inflammatory response is initiated by the detection of the invading microbes through their pathogen-associated molecular patterns (PAMPs), such as lipopolysaccharide (LPS). Additionally, damage inflicted by the invading microbes to the host leads to the release of damage-associated molecular patterns (DAMPs), including high mobility group box 1 (HMGB1). These patterns bind to pattern recognition receptors (PRRs), which are displayed by both circulating innate immune cells and endothelial cells. These PRRs include Toll-like receptors (TLRs), C-type lectin receptors (CLRs), and NOD-like receptors (NLRs) (Lasola et al., 2020; Friedrich et al., 2021; Yu et al., 2022). This detection activates inflammatory signaling pathways, such as nuclear factor-kappa B (NF-κB), c-Jun N-terminal kinase (JNK), and p38 mitogen-activated protein kinase (MAPK). Activation of these pathways induces neutrophils, monocytes, and macrophages to phagocytize the pathogens and synthesize pro-inflammatory cytokines and chemokines, such as tumor necrosis factor-alpha (TNFα), interleukin-1 (IL-1), and interleukin-6 (IL-6) (Vuong et al., 2017; Huang et al., 2019). These inflammatory signals recruit more immune cells, induce vasodilation, and increase vascular permeability to promote immune cell infiltration (Semeraro et al., 2010; Jackson et al., 2019). Microbes and cytokines stimulate neutrophils to release neutrophil extracellular traps (NETs), which capture and kill microbes (DeBont et al., 2019). However, in sepsis, the excessive accumulation of immune cells is harmful to the body. The high leukocyte numbers release a “cytokine storm,” resulting in tissue damage and paradoxically reduced pathogen clearance (Huang et al., 2019). This is largely due to activated natural killer (NK) cells, which amplify inflammation by secreting interferon-gamma (IFN-γ) and further tissue damage by releasing cytotoxic granzymes and perforins (Guo et al., 2018). NK cells also induce immune cell apoptosis with their ligands, ultimately reducing pathogen uptake and clearance (Michel et al., 2012; Thorén et al., 2012). The activated endothelium also increases its expression of adhesion molecules, including von Willebrand factor (vWF), P-selectins, vascular cell adhesion molecule-1 (VCAM-1), and platelet-endothelial cell adhesion molecule (PECAM)-1 (Greineder et al., 2013; Banka et al., 2023). These molecules enhance the binding of neutrophils, monocytes, and platelets, promoting coagulation and providing targets for site-specific nanoparticles. Biomaterials have also been developed to block inflammation from neutralizing PAMPs and removing reactive oxygen species to altering leukocyte recruitment and phagocytic capabilities and interactions (Lasola et al., 2020) (Figure 1).
FIGURE 1. Sepsis Pathophysiology. Innate immune cells recognize pathogens such as bacteria, viruses, and parasitic DNA with pattern recognition receptors. Pro-inflammatory signaling cascades including NF-κB, JNK, and MAPK are activated. Cells secrete cytokines and reactive oxygen species, activating immune cells to phagocytize, neutrophils to release neutrophil extracellular traps, and platelets and endothelial cells to switch to a pro-coagulant phenotype. Cellular interactions amplify inflammation, cause endothelial cell damage, and induce thrombosis. Created with BioRender.
Traditionally, biomaterials have been synthetic, including polymers, ceramics, and metals. Examples of commonly used synthetic biomaterials for targeted drug delivery include lipid nanoparticles, liposomes, dendrimers, and poly(ethylene glycol) (PEG) conjugates (Greineder et al., 2013). Conjugating PEG to compounds or nanoparticles, also known as “PEGylation,” is a common technique to improve the “stealth” of nanoparticles and increase circulation time (Sun et al., 2008; El Mohtadi et al., 2019; Yan et al., 2019; Huang et al., 2021; Nunoi et al., 2022). PEGylation creates a hydrophilic layer, sterically repelling proteins and thereby limiting the adsorption of opsonins that signal to immune cells to uptake and destroy (Kelley et al., 2016; Sobczynski et al., 2017). Moreover, the hydrophilic layer produced by PEGylation can increase drug solubility (Greineder et al., 2013). Of course, PEGylation has a drawback: studies have indicated that PEGylated products can lead to acquired immunogenicity, limiting its use for drugs that require repeat dosing (Kelley et al., 2016). Moreover, the acquired immunogenicity results in accelerated clearance of the particles from the bloodstream, limiting the “stealth” of the materials (El Mohtadi et al., 2019). Therefore, other polymers with stealth capabilities are also being investigated, including poly(propylene sulfoxide) which also has antioxidant properties (El Mohtadi et al., 2019), poly(2-oxazoline) (POx), and zwitterionic polymers (Fam et al., 2020). As of yet, none of these other polymers have been found to induce acquired immunogenicity nor result in accelerated blood clearance.
Several synthetic biomaterials are currently being investigated for attenuating inflammation in sepsis models (Table 1). The following studies emphasize the inherent anti-inflammatory abilities of empty biomaterials in ameliorating sepsis-induced pro-inflammatory cytokine release based on their physicochemical properties. Empty polymer nanoparticles, synthesized from poly(lactic-co-glycolic acid) (PLGA) and poly(lactic acid) (PLA), reduced TLR activation and subsequent pro-inflammatory cytokine release in an LPS-induced sepsis mouse model (Casey et al., 2019). Unloaded high-density lipoprotein nanoparticles targeted to VCAM-1 have been shown to inhibit leukocyte adhesion to activated endothelium, reducing proinflammatory cytokine release in an LPS-induced acute lung inflammation mouse model (Yu et al., 2022). Cargo-free liposomes developed from fish roe lipids similarly imparted anti-oxidant and anti-inflammatory effects in LPS-stimulated macrophages (Guedes et al., 2021). Similar in concept, eggshell membrane particles are under investigation for their anti-inflammatory properties, attributed to the proteins and carbohydrates they contain. In addition to reducing NF-κB activity and associated pro-inflammatory cytokines, these particles also increased anti-inflammatory cytokine interleukin-10 (IL-10) (Vuong et al., 2017). Other anti-inflammatory and antioxidant polymers that have demonstrated protection from LPS include N-acetylcysteine gels (Kim et al., 2021), graphene oxide-polypropylene nanoparticles (Fonticoli et al., 2023), and polyphenol gels (Luque et al., 2023), the latter two of which also have antibacterial benefits. In a cecal-puncture and ligation sepsis mouse model, dendrimers scavenged pro-inflammatory cytokines, resulting in a significantly improved survival rate of 100% (Shi et al., 2020). Bioactive glasses have also been examined for their anti-inflammatory and antibacterial potential (Nedelec et al., 2008). Mesoporous bioactive glass nanoparticles (MBGNPs) carrying cerium, an antioxidant, successfully demonstrated anti-inflammatory and antibacterial activity against both gram-positive and gram-negative bacteria in in vitro assays (Kurtuldu et al., 2021). Similarly, MBGNPs loaded with gallium exhibited antibacterial and hemostatic benefits in vitro (Kurtuldu et al., 2022). In general, synthetic biomaterials are the easiest drug delivery vehicles to produce and have high manufacturing consistency, but cell-derived biomaterials also offer their own advantages (Witwer and Wolfram, 2021).
TABLE 1. Biomaterials for Altering Inflammation. The composition, preparation methods, sizes, charges, and investigated anti-inflammatory properties of synthetic biomaterials, cell-derived biomaterials, and combined synthetic and cell-derived biomaterials. PLGA: poly(lactic-co-glycolic acid); PLA: polylatic acid; NAC: N-acetylcysteine; TMOS: tetramethoxysilane; CTAB: cetyltrimethylammonium bromide; PVA: poly(vinyl alcohol); PEG: polyethylene glycol; DAMP: damage-associated molecular pattern; PAMP: pathogen-associated molecular pattern; i.p.: intraperitoneal; i.t.: intratracheal; i.n.: intranasal; p.o.: per os; MHV: mouse hepatitis virus.
Cell-derived biomaterials, including extracellular vesicles and cell-derived products, are being investigated as drug delivery vehicles for their inherent biocompatibility (Table 1). Exosomes are an exciting group of extracellular vesicles known as “natural nanoparticles.” Exosomes are particularly promising due to their cell membrane proteins and natural cargo of nucleic acids, proteins, and lipids, meaning exosomes display parent cell-specific targeting and functions (Popowski et al., 2020). These particles’ biocompatible membranes are also able to avoid immune recognition and reduce toxic effects (Lutz et al., 2021). Furthermore, exosome surfaces can be engineered to display ligands for improved targeting and controlled release and their cargo can be altered to carry pharmaceutical drugs (Lutz et al., 2019). In an LPS-induced sepsis mouse model, extracellular vesicles carrying a glucocorticoid reduced renal inflammation and fibrosis, and no side effects common with glucocorticoids were observed (Tang et al., 2019; Lasola et al., 2020). A significant challenge with extracellular vesicles are scaling up production to translating research findings to clinical doses. A potential solution being explored is nitrogen cavitation, which has shown promise by increasing extracellular vesicle production by 16-fold (Gao et al., 2017; Hahm et al., 2021; Ng et al., 2022). Additionally, extracellular vesicles generated using this method retained their ability to efficiently load drugs and deliver them effectively to reduce LPS-induced acute lung inflammation (Gao et al., 2017). Another cell-derived product is heparin, which is especially useful as a biomaterial for sepsis due to its antiviral activity and hemostatic properties (Kemp and Linhardt, 2010). Already, heparin has been found to reduce in-hospital mortality in septic patients by reducing inflammation and rescuing platelet counts over 28 days (Zou et al., 2022; Zhang Z et al., 2023).
Cell-based biomaterials are frequently examined in opposition to synthetic materials, for each type has its own advantages and disadvantages. After all, synthetic materials have more flexibility in terms of composition and designing the sizes and shapes for different applications. In contrast, modification of cell-derived materials are limited to certain sizes and shapes, such as 30–200 nm spherical exosomes (Gurung et al., 2021) or 7 μm discoid red blood cells (Muzykantov, 2013). Still, even with all the design options for synthetic materials, none have been shown to match the four-month lifespan of red blood cells in circulation (Chen et al., 2023). In terms of applications, synthetic materials have been found to provide better intracellular delivery (Muro et al., 2008), and in the context of sepsis, anti-inflammatory drug delivery to damaged tissues is critical. At the same time, red blood cells remain attractive for intravascular drug delivery in sepsis to deliver antithrombotic agents and cytokine inhibitors (Kolesnikova et al., 2013). Due to the unique advantages maintained by each type of biomaterial, research groups are investigating strategies to combine synthetic and cell-derived materials to produce more efficacious drug delivery systems.
One of the products of combined synthetic and cell-derived materials is the development of biomimetic nanoparticles (Table 1). These nanoparticles use membranes from platelets and other blood cells to encapsulate drug-carrying nanoparticles. Karami et al. investigated the ability of PLGA nanoparticles covered in monocyte membrane, carrying the anti-inflammasome drug Gliclazide, to reduce pro-inflammatory cytokine release in monocytes treated with LPS. They also found that in an atherosclerotic rabbit study, these “nanoghosts” shifted macrophages from a pro-inflammatory to anti-inflammatory phenotype and inhibited plaque development (Karami et al., 2023). Macrophage biomimetic nanoparticles have been studied as a therapeutic for COVID-19, which can progress into sepsis. These nanoparticles exhibited both anti-inflammatory and antiviral effects, ultimately resulting in improved survival in a mouse coronavirus model (Tan et al., 2021). However, further research in more relevant models is needed. While research into biomimetic materials specifically for sepsis-based inflammation is limited, many groups have demonstrated the potential of biomimetic nanoparticles for attenuating other inflammatory diseases, including osteoarthritis (Yan et al., 2020), myocardial infarction (Bao et al., 2022; Chen et al., 2022), and neuroinflammation (Zhang M et al., 2023), by suppressing pro-inflammatory cytokine secretion and enhancing the expression of anti-inflammatory cytokines.
Despite the wide range of biomaterials investigated recently for controlling inflammation in sepsis, several obstacles persist in pre-clinical studies. Firstly, the models used for sepsis are too narrowly focused (Choi et al., 2023). Many studies rely on LPS-induced sepsis models and are limited to in vitro studies using monocytes and macrophages (Jin et al., 2019; Kong et al., 2019; Lee et al., 2020; Hemmingsen et al., 2021). Due to the extensive literature on the role of LPS in sepsis, it is obviously understandable to pursue investigations in an established model. However, sepsis can result from various infectious organisms, including viruses, fungi, and parasites and it would be remiss not to broaden studies to include these. With the advent of SARS-CoV-2, several studies have investigated COVID-19-related sepsis, but more sepsis models from different causes remain to studied. Moreover, LPS is specific to gram-negative bacteria and does not account for the pathogenicity and different inflammatory pathways activated by gram-positive bacteria, such as methicillin-resistant Staphylococcus aureus (MRSA) (Dickson and Lehmann, 2019). Even within bacterial species, different strains carry unique lipid A endotoxins, leading to varying interactions with cells and levels of toxicity (Dickson and Lehmann, 2019; Harm et al., 2019). Additionally, pre-clinical models often expose animals to their first infectious agents, while human sepsis patients must also combat opportunistic pathogens and underlying comorbidities. Another challenge is the need to shift research toward developing multitargeted biomaterials. Current strategies often focus on single-target approaches to minimalize side effects. However, sepsis is a complex disorder that affects multiple body systems and processes, necessitating a multitargeted solution.
One of the processes severely affected by sepsis is hemostasis. Under normal conditions, endothelial cells maintain an anticoagulant state and secrete platelet antagonists to prevent adhesion (Sloos et al., 2022). During sepsis or any inflammatory event, thrombosis is stimulated (Jackson et al., 2019). As previously noted, inflammation also activates the endothelial cells to increase their expression of adhesion proteins, thereby increasing the binding of neutrophils, monocytes, and platelets. Furthermore, the NETs released by neutrophils ensnare platelets as well as bacteria, promoting coagulation and clot formation. When injured, endothelial cells shift to a pro-coagulant state and secrete tissue factor, thrombin, and plasminogen activation inhibitor-1 (PAI-1) (Dudnick et al., 1991). The vasculature initially constricts, increasing platelet interactions with endothelial collagen (Cánovas-Cervera et al., 2023). The platelets activate and aggregate in a process known as primary hemostasis. Following, secondary hemostasis involves interactions between coagulation factors to stabilize the clot with fibrin formation (Raghunathan et al., 2022). In normal circumstances, once the damaged endothelium is repaired, the clot becomes subjected to fibrinolysis with tissue-plasminogen activator (tPA) converting inactive plasminogen to plasmin, which degrades and removes the clot. During sepsis, clot formation may be aberrant, resulting in clots that are either too weak to fulfill hemostasis or too strong to undergo lysis (Dudnick et al., 1991). Therefore, there is a need for biomaterials that can safely act on the endothelium to promote hemostasis and stimulate clot lysis as appropriate.
Currently, there are several pharmacologic treatments that are used to stabilize hemostasis, including desmopressin, aprotinin, and tranexamic acid. However, there is a need for drugs that directly promote hemostasis (Dudnick et al., 1991; Sloos et al., 2022). Another pro-hemostatic option is whole blood or platelet transfusions. Unfortunately, this option is not always available due to donor shortages, high costs, and the risk of immunogenic reactions (Dudnick et al., 1991; Gao et al., 2020). Moreover, when available, platelets face a short storage life of only five to 7 days (Dudnick et al., 1991; Fernandez-Moure et al., 2018; Raghunathan et al., 2022; Gao et al., 2023). Lyophilization of blood products has been explored as a method to extend storage life and improve accessibility by enabling storage at higher temperatures. Lyo-protectants and cryo-protectants preserve platelet morphology and function. In several animal models, lyophilized platelets have been found to reduce bleeding time and blood loss (Cap and Perkins, 2011). Unfortunately, lyophilized platelets have been found to only last roughly 36 h in circulation before being cleared by macrophages, so while they may be useful in acute trauma, lyophilized platelets have limited use as a prophylactic (Raghunathan et al., 2022). Therefore, a branch of research has focused on developing biosynthetic blood cells, particularly platelet mimetics (Kelley et al., 2016). Some groups use platelet membranes to coat their nanoparticle surfaces, but as with extracellular vesicles, they face scale-up challenges. In this case, the lack of platelet availability from which to derive membranes was the primary obstacle (Raghunathan et al., 2022). To overcome the challenge of platelet membrane availability, some research groups coat nanoparticles with hemostatic proteins found on platelets, including fibrin knob B (Nandi et al., 2021), fibrin antibodies (Brown et al., 2014), phosphatidylserine (Sekhon et al., 2022), vWF-binding peptides, and collagen-binding peptides (Gao et al., 2020; Girish et al., 2022; Gao et al., 2023) (Table 2). These platelet-mimicking particles promote clotting, reduce blood loss, and decrease bleeding time in various models, including liver laceration, tail-clip injury, hemophilia, and human plasma from patients with coagulopathy.
TABLE 2. Biomaterials for Coagulopathies. The composition, preparation methods, sizes, charges, and investigated hemostatic, anticoagulant, and fibrinolytic properties of biomaterials. pNIPAm: poly(N-isopropylacrylamide); AAC: acrylic acid; ULC: ultra-low crosslinked; DSPC: distearoylphosphatidylcholine; DSPE: 1,2-distearoyl-sn-glycero-3-phosphoethanolamine; VBP: vWF binding peptide; CBP: collagen binding peptide; PAH: poly(allylamine hydrochloride); BSA: bovine serum albumin; FMP: fibrinogen-mimetic peptide; TDI: 2,4-toluene diisocyanate; TCP: thrombin-cleavable peptide; i.v.: intravenous.
During systemic vascular injuries such as sepsis, blood clots can form and become lodged in the microvasculature, blocking blood flow, and causing ischemia. In clinical cases of thrombosis, anticoagulants are commonly administered. However, available anticoagulants have narrow therapeutic ranges and can interfere with hemostasis, posing a bleeding risk. As a result, the administration of anticoagulants, including heparin, antithrombin-III (ATIII), hirudin, activated protein C, thrombomodulin, and tissue factor pathway inhibitor, comes with a risk of bleeding, which can be equally life-threatening. Heparin is an attractive anticoagulant due to its binding with antithrombin-III, rending clots weak and easily lysed (Baier et al., 2015). Furthermore, heparin inhibits the complement system, thereby imparting “stealth” properties to nanoparticles hidden within and increasing blood circulation time (Argyo et al., 2012). Although studies have shown that heparin nanoparticles and heparin-coated nanoparticles increase clotting times, heparin carries a bleeding risk and also induces thrombocytopenia (Peter et al., 2000; Xu et al., 2020). Hirudin, a thrombin inhibitor independent of ATIII, is a potential heparin replacement. Hirudin can achieve anticoagulant activity without inducing thrombocytopenia and the magnitude of hirudin’s effects is directly proportional to its dosage. In pulmonary embolism and arterial thrombosis mouse models, hirudin-loaded nanogels demonstrated clot-dependent release, inhibiting clot formation. Importantly, bleeding times were not significantly prolonged in tail-clip injuries (Xu et al., 2020). In these studies, the nanogels controlled hirudin release using thrombin-cleavable peptides.
Another approach to target active clot formation sites is to decorate delivery vehicles with antibodies or antibody-fragments specific to proteins expressed exclusively during endothelial injury. This method, nor its use in sepsis research, is new (Colman et al., 1988), but as understanding of sepsis and coagulopathy pathophysiology improves, novel targets and targeting ligands have recently developed. Polymeric micelles with a single-chain fragment variable antibody (scFv) that binds to GPIIb/IIIa, a glycoprotein receptor found only on activated platelets, accumulated at platelet-rich thrombi. When loaded with thrombomodulin, they effectively inhibited thrombus formation (W. Kim et al., 2015). Another strategy involved blocking platelet glycoproteins with an scFv to prevent platelet interactions with thrombin and inhibit thrombus formation. Additional loading of an antithrombotic, an α1-antitrypsin variant, enhanced clot inhibition (Sanrattana et al., 2022). This particular set of studies is notable due to its multi-targeted strategy in both blocking platelet binding with scFv as well as inhibiting thrombin with the α1-antitrypsin variant. Following this multitargeted approach, a research group targeted clot formation from the trapping of activated platelets in NETs. This group developed nanoparticles with antibody fragments to selectively target and inhibit activated neutrophils from forming NETs. The nanoparticles were also loaded with hydroxychloroquine to inhibit platelet aggregation and adhesion, thereby inhibiting clot formation through two separate mechanisms (Cruz et al., 2023). Antibody fragments offer advantages such as avoiding cellular activation, minimizing immunogenicity, and binding with high specificity. However, it’s important to note that while scFv-bound antithrombotic drugs localize at clots, their accessibility diminishes, potentially limiting their effectiveness and the ability to use lower dosages to reduce bleeding risk (Greineder et al., 2013).
Anticoagulants prevent thrombus formation, while fibrinolytics dissolve existing clots. The most common fibrinolytics are plasminogen activators, mainly tPA and urokinase. Unfortunately, as with anticoagulants, administration of plasminogen activators is associated with increased bleeding risk (Wang et al., 2020; Helms et al., 2023). Moreover, plasminogen activators are notorious for extremely short circulation times making their administration impractical in most cases. Delivery in a biomaterial vehicle can resolve both of these issues. Already, fusing tPA to PEG increases circulation time up to five times as long as naked tPA (M. Sun and Gupta, 2020) but doesn’t affect tPA-induced hemorrhage (Greineder et al., 2013). When conjugated to an anti-fibrin antibody, tPA potency and selectivity improved, meaning further investigation is necessary to determine if a subclinical dose of tPA-bound anti-fibrin antibody could effectively dissolve clots and avoid bleeding (Runge et al., 1987). Thus far, delivery of subclinical tPA doses has yet to be examined, and this may be due to the discovery of nattokinase. Nattokinase, a plant-derived fibrinolytic with anti-inflammatory and antioxidant activities, directly degrades fibrin in clots without causing bleeding and inhibits platelet aggregation (Ye et al., 2018; Wu et al., 2020). Therefore, nattokinase overcomes the limitations of tPA without the need for additional modifications. Nattokinase is still a relatively novel fibrinolytic, so it is definitely a compound to keep an eye on as more studies examine its potential and establish its limits. As for urokinase, its clinical usage has thus far been limited to diagnosing sepsis and predicting prognosis based on blood level elevations of its receptor, soluble urokinase plasminogen activator receptor, correlating to disease severity (Sharma et al., 2020; Efat et al., 2021; Ma et al., 2023). Urokinase is not widely used as a thrombolytic due to its markedly reduced specificity for fibrin and increased bleeding risk in comparison to tPA (Adivitiya and Khasa, 2017; Kadir and Bayraktutan, 2020).
Special considerations are necessary for biomaterials administered intravenously to ensure compatibility with blood. The complement system plays a role in immune surveillance and recognizes foreign materials and pathogens (Figure 2). It can be activated through three different pathways: the classical, the lectin, and the alternative pathways. Each of these pathways result in the production of anaphylatoxins C3a and C5a (Maisha et al., 2020; Maisha et al., 2021). Once generated, these anaphylatoxins can trigger allergic reactions, including histamine release and vasodilation. When trying to improve clotting, vasodilation is counter-effective by increasing blood flow and minimizing platelet interactions with the endothelium. In severe sepsis, anaphylatoxins are already generated (Landsem et al., 2022) and administering therapeutic biomaterials may actually worsen a patient’s condition. It is crucial to conduct in vitro testing for cell lysis of blood cells, platelet activation, plasma clotting, and C3a levels. Additionally, in vivo testing in an animal model with complement activation similar to that in humans is essential for anticipating adverse effects and advancing biomaterials (Harm et al., 2019; Maisha et al., 2020).
FIGURE 2. The complement system. The immune system can be notified of pathogens or foreign substances in the blood by activation of the complement system through the classical, lectin, or alternative pathways. A cascade of signals ultimately results in the formation of C3a and C5a, which induce inflammation, and C5b, which signals to immune cells to lyse the pathogen. Created with Biorender.
As sepsis worsens, it can progress to thromboinflammation, which involves the inappropriate and unregulated formation of blood clots due to an extreme immune response (Banka et al., 2023). In more detail, inflammation activates the complement system and induces thrombosis (Vagionas et al., 2022). For example, activated neutrophils form neutrophil extracellular traps (NETs), which both activate platelets and entrap platelets, amplifying clot formation. Activated neutrophils also enhance platelet release from the bone marrow, contributing to thrombi formation (Petzold et al., 2022). In turn, activated platelets release pro-inflammatory signals, further increasing inflammation and injuring tissues in a pathologic cycle (Jackson et al., 2019; Senchenkova et al., 2019; Perrella et al., 2021). Thromboinflammation is a commonality in cardiovascular diseases, including atherosclerosis, COVID-19, and disseminated intravascular coagulation (DIC). In DIC, coagulation factors, platelets, and fibrinogen are consumed leading to the inappropriate formation of microthrombi and subsequent activation of fibrinolysis. Excessive platelet activation has been hypothesized to lead to platelet dysfunction and impaired response (Sloos et al., 2022). Clinically, this can present with prothrombotic and/or hemorrhagic phenotypes, resulting in contradictory treatment strategies. The concurrent ischemia, hemorrhage, and pro-inflammatory cytokines potentiate inflammation and injury, leading to multiple organ dysfunction syndrome (MODS). As expected, MODS is associated with a significantly worse prognosis (Helms et al., 2023). Therefore, there is an urgent need for the development of a single therapeutic that can simultaneously address inflammation, thrombosis, and hemorrhage.
To reduce thromboinflammation, studies have shown that cargo-free polystyrene microparticles can disrupt leukocyte adhesion to the endothelium in LPS models, emphasizing the importance in biomaterial physical characteristics (Banka et al., 2023) (Figure 3). Specifically, carriers with rod-like shapes and diameters between 2 and 5 microns demonstrate enhanced margination along the endothelium compared to spherical nanoparticles. This increased interaction with endothelial cells improves adhesion and targeting efficiency (Kelley et al., 2016; Van Der Meel et al., 2014; Y. Gao et al., 2020). At this moment, most studies focus on altering platelet signaling pathways involved in thromboinflammation by administering agonists or inhibitors. For example, increased expression of platelet chemokine receptor ACKR3/CXCR7 and administration of ACKR3/CXCR7 agonists reduced neutrophil activation, platelet activation, and thrombosis (Cebo et al., 2022). Another mechanism to reduce platelet-lymphocyte interactions is to eliminate platelet glycoprotein GPIb binding abilities to neutrophils. This was found to inhibit downstream Sema7a signaling and subsequent thrombus formation (Gauer et al., 2022). Alternatively, inhibition of CLEC-2 signaling by knocking out platelet expression of CLEC-2 or administering antibodies was found to inhibit thrombosis, reduce the release of pro-inflammatory mediators, and limit the severity of sepsis-related symptoms in mice (Meng et al., 2021).
FIGURE 3. Common strategies for targeting thromboinflammation. Currently, efforts to inhibit thromboinflammation focus on (A) disrupting signaling pathways to reduce neutrophil and platelet activation, thrombus formation, and inflammatory cytokine release; (B) inhibiting cell-cell interactions, such as leukocyte-endothelial and leukocyte-platelet interactions; and (C) developing biomaterials with physical properties that enhance endothelial margination. Created with Biorender.
Thrombosis can also lead to acute ischemic stroke, which currently only has one FDA approved therapy: tPA. As previously discussed, tPA presents a significant bleeding risk (Beyer et al., 2022). To address this, our research group has designed fibrin-targeting nanogels that site-specifically deliver anti-clotting drugs while also enhancing fibrin-crosslinking and clot formation. When loaded with tPA, we found that our nanogels accomplished a dual function of targeting and dissolving existing clots while promoting clotting at sites of hemorrhage, eliminating the bleeding risk posed by tPA (Mihalko et al., 2021; Mihalko et al., 2022). Other investigations using fibrin-specific systems have been similar in loading anti-fibrin antibody nanoparticles with fibrinolytics (Marsh et al., 2011), while more others target thrombi by decorating liposomes with anti-fibrin protein binders (Petroková et al., 2019) and conjugating anti-fibrin antibodies directly to fibrinolytics (Peter et al., 2000; El-Sherbiny et al., 2014). Recognizing the complexity of thromboinflammation, other strategies involve targeting different cell types. Some research groups have explored multitargeting by using nanoparticles with multiple ligands to interact with multiple cell types and receptors. This approach enhances the specificity of nanoparticle interactions, drug release sites, and reduces systemic side effects (Eniola and Hammer, 2005; Rafat et al., 2012; Anselmo et al., 2014) (Table 3).
TABLE 3. Biomaterials for Thromboinflammation. The composition, preparation methods, sizes, and investigated anti-thromboinflammatory properties of biomaterials. PEMA: poly(ethylene-co-maleic acid); PVA: polyvinyl alcohol.
Sepsis continues to be associated with high mortality, despite decades of research. Until recently, treatments for sepsis focused solely on reducing inflammation. Now, researchers understand that the pathophysiology of sepsis is driven by a close interplay between inflammation and hemostasis, termed “thromboinflammation” – a term first introduced only 25 years ago. As more information about thromboinflammation emerges, biomaterials continue to be the most promising solution for addressing thromboinflammation and treating sepsis. However, challenges remain in developing a single biomaterial that can effectively control inflammation, endothelial damage, and coagulopathy. Further examination and identification of the advantages of synthetic biomaterials and cell-derived biomaterials is necessary. Incorporating elements from each type of biomaterial appears effective in developing biomaterials that have improved blood circulation times and endothelial margination. Moreover, biomaterials have thus far been largely designed to target specific cell interactions, either reducing pro-inflammatory cytokines or preventing inappropriate thrombus formation separately. Recognizing the complexity of sepsis pathology and developing a multifunctional biomaterial is required to produce an effective therapy for this deadly disease.
HL: Conceptualization, Visualization, Writing–original draft, Writing–review and editing. AB: Funding acquisition, Project administration, Resources, Supervision, Writing–review and editing.
The author(s) declare financial support was received for the research, authorship, and/or publication of this article. This study is supported by NIH NHLBI R01HL146701, NIH NHLBI 1R01HL162809-01A1, and Grifols GATRA program.
The authors declare that the research was conducted in the absence of any commercial or financial relationships that could be construed as a potential conflict of interest.
All claims expressed in this article are solely those of the authors and do not necessarily represent those of their affiliated organizations, or those of the publisher, the editors and the reviewers. Any product that may be evaluated in this article, or claim that may be made by its manufacturer, is not guaranteed or endorsed by the publisher.
Adivitiya, , and Khasa, Y. P. (2017). The evolution of recombinant thrombolytics: current status and future directions. Bioengineered 8 (4), 331–358. doi:10.1080/21655979.2016.1229718
Angus, D. C., and Poll, T. (2012). Severe sepsis and septic shock. N. Engl. J. Med. 369, 840–851. doi:10.1056/nejmra1208623
Anselmo, A. C., Modery-Pawlowski, C. L., Menegatti, S., Kumar, S., Vogus, D. R., Tian, L. L., et al. (2014). Platelet-like nanoparticles: mimicking shape, flexibility, and surface biology of platelets to target vascular injuries. ACS Nano 8 (11), 11243–11253. doi:10.1021/nn503732m
Argyo, C., Cauda, V., Engelke, H., Rädler, J., Bein, G., and Bein, T. (2012). Heparin-coated colloidal mesoporous silica nanoparticles efficiently bind to antithrombin as an anticoagulant drug-delivery system. Chem. - A Eur. J. 18 (2), 428–432. doi:10.1002/chem.201102926
Baier, G., Winzen, S., Messerschmidt, C., Frank, D., Fichter, M., Gehring, S., et al. (2015). Heparin-based nanocapsules as potential drug delivery systems. Macromol. Biosci. 15 (6), 765–776. doi:10.1002/mabi.201500035
Banka, A. L., Guevara, M. V., Brannon, E. R., Nguyen, N. Q., Song, S., Cady, G., et al. (2023). Cargo-free particles divert neutrophil-platelet aggregates to reduce thromboinflammation. Nat. Commun. 14 (1), 2462. doi:10.1038/s41467-023-37990-z
Bao, L., Dou, G., Tian, R., Lv, Y., Ding, F., Liu, S., et al. (2022). Engineered neutrophil apoptotic bodies ameliorate myocardial infarction by promoting macrophage efferocytosis and inflammation resolution. Bioact. Mater. 9, 183–197. doi:10.1016/j.bioactmat.2021.08.008
Bauer, M., Gerlach, H., Vogelmann, T., Preissing, F., Stiefel, J., and Adam, D. (2020). Mortality in sepsis and septic shock in europe, north America and Australia between 2009 and 2019-results from a systematic review and meta-analysis. Crit. Care 24 (1), 239–9. doi:10.1186/s13054-020-02950-2
Beyer, M., France, J., Lavik, E., Knight, R., and Lewandowski, C., (2022). Unaffected ex vivo clotting cascade by experimental hemostatic nanoparticles when introduced in the presence of recombinant tissue plasminogen activator. Brain Circ. 8 (4), 228. doi:10.4103/bc.bc_45_22
Brown, A., Stabenfeldt, S. E., Ahn, B., Hannan, R. T., Dhada, K. S., Herman, E. S., et al. (2014). Ultrasoft microgels displaying emergent platelet-like behaviours. Nat. Mater. 13 (12), 1108–1114. doi:10.1038/nmat4066
Cánovas-Cervera, I., Nacher-Sendra, E., Osca-Verdegal, R., Dolz-Andrés, E., Beltrán-García, J., Rodríguez-Gimillo, M., et al. (2023). The intricate role of non-coding RNAs in sepsis-associated disseminated intravascular coagulation. Int. J. Mol. Sci. 24 (3), 2582. doi:10.3390/ijms24032582
Cap, A. P., and Perkins, J. G. (2011). Lyophilized platelets: challenges and opportunities. J. Trauma - Inj. Infect. Crit. Care 70 (5 Suppl. L), 59–60. doi:10.1097/TA.0b013e31821a606d
Casey, L. M., Kakade, S., Decker, J. T., Rose, J. A., Deans, K., Shea, L. D., et al. (2019). Cargo-less nanoparticles program innate immune cell responses to toll-like receptor activation. Biomaterials 218 (June), 119333. doi:10.1016/j.biomaterials.2019.119333
Cebo, M., Dittrich, K., Fu, X., Manke, M. C., Emschermann, F., Rheinlaender, J., et al. (2022). Platelet ACKR3/CXCR7 favors antiplatelet lipids over an atherothrombotic lipidome and regulates thromboinflammation. Blood 139 (11), 1722–1742. doi:10.1182/blood.2021013097
Chen, J., Song, Y., Wang, Q., Li, Q., Tan, H., Gao, J., et al. (2022). Targeted neutrophil-mimetic liposomes promote cardiac repair by adsorbing proinflammatory cytokines and regulating the immune microenvironment. J. Nanobiotechnology 20 (1), 218–317. doi:10.1186/s12951-022-01433-6
Chen, M., Leng, Y., Li, X., Zhao, L., and Qu, Y., (2023). Red blood cells: a potential delivery system. J. Nanobiotechnology 21 (1), 288–319. doi:10.1186/s12951-023-02060-5
Choi, S.-H., Kim, S. Y., Kim, K. M., Mony, T. J., Bae, H. J., Kim, M. S., et al. (2023). Fermented sprouts of codonopsis lanceolata suppress LPS-induced inflammatory responses by inhibiting NF-κb signaling pathway in RAW 264.7 macrophages and CD1 mice. Pharmaceutics 15 (7), 1793. doi:10.3390/pharmaceutics15071793
Colman, R. W., Flores, D. N., De La Cadena, R. A., Scott, C. F., Cousens, L., Barr, P. J., et al. (1988). Recombinant alpha 1-antitrypsin Pittsburgh attenuates experimental gram-negative septicemia. Am. J. Pathology 130 (2), 418–426.
Cruz, M. A., Bohinc, D., Andraska, E. A., Alvikas, J., Raghunathan, S., Masters, N. A., et al. (2023). Nanomedicine platform for targeting activated neutrophils and neutrophil-platelet complexes using an α1-antitrypsin-derived peptide motif. Nat. Nanotechnol. 17 (9), 1004–1014. doi:10.1038/s41565-022-01161-w
DeBont, C. M., Boelens, W. C., and Pruijin, G. J. M. (2019). NETosis, complement, and coagulation: a triangular relationship. Cell. Mol. Immunol. 16, 19–27. doi:10.1038/s41423-018-0024-0
Dickson, K., and Lehmann, C. (2019). Inflammatory response to different toxins in experimental sepsis models. Int. J. Mol. Sci. 20 (18), 4341. doi:10.3390/ijms20184341
Dudnick, R., Martin, P., and Friedman, L. S. (1991). Management of bleeding ulcers. Med. Clin. N. Am. 75 (4), 947–965. doi:10.1016/S0025-7125(16)30423-0
Efat, A., Shoeib, S. A., Arafa, A. F., Dawod, A. A., Abd ElHafez, M. A., Abd ElMohsen, E. A., et al. (2021). Thrombo-inflammatory biomarkers to predict sepsis outcome. Int. J. Immunopathol. Pharmacol. 35, 205873842110485. doi:10.1177/20587384211048561
El Mohtadi, F., d'Arcy, R., Yang, X., Turhan, Z. Y., Alshamsan, A., and Tirelli, N. (2019). Main chain polysulfoxides as active ‘stealth’ polymers with additional antioxidant and anti-inflammatory behaviour. Int. J. Mol. Sci. 20 (18), 1–14. doi:10.3390/ijms20184583
El-Sherbiny, I., Elkholi, I. E., and Yacoub, M. H. (2014). Tissue plasminogen activator-based clot busting: controlled delivery approaches. Glob. Cardiol. Sci. Pract. 2014 (3), 46. doi:10.5339/gcsp.2014.46
Eniola, A. O., and Hammer, D. A. (2005). In vitro characterization of leukocyte mimetic for targeting therapeutics to the endothelium using two receptors. Biomaterials 26 (34), 7136–7144. doi:10.1016/j.biomaterials.2005.05.005
Fam, S. Y., Chee, C. F., Yong, C. Y., Ho, K. L., Mariatulqabtiah, A. R., and Tan, W. S. (2020). Stealth coating of nanoparticles in drug-delivery systems. Nanomaterials 10 (4), 787–818. doi:10.3390/nano10040787
Fernandez-Moure, J., Maisha, N., Lavik, E. B., and Cannon, J. W. (2018). The chemistry of lyophilized blood products. Bioconjugate Chem. 29 (7), 2150–2160. doi:10.1021/acs.bioconjchem.8b00271
Fonticoli, L., Diomede, F., Nanci, A., Fontana, A., Della Rocca, Y., Guadarrama Bello, D., et al. (2023). Enriched graphene oxide-polypropylene suture threads buttons modulate the inflammatory pathway induced by Escherichia coli lipopolysaccharide. Int. J. Mol. Sci. 24 (7), 6622. doi:10.3390/ijms24076622
Friedrich, B., Auger, J. P., Dutz, S., Cicha, I., Schreiber, E., Band, J., et al. (2021). Hydroxyapatite-coated SPIONs and their influence on cytokine release. Int. J. Mol. Sci. 22 (8), 4143. doi:10.3390/ijms22084143
Gaieski, D. F., Edwards, J. M., Kallan, M. J., and Carr, B. G. (2013). Benchmarking the incidence and mortality of severe sepsis in the United States. Crit. Care Med. 41 (5), 1167–1174. doi:10.1097/CCM.0b013e31827c09f8
Gao, J., Wang, S., and Wang, Z. (2017). High yield, scalable and remotely drug-loaded neutrophil-derived extracellular vesicles (EVs) for anti-inflammation therapy. Biomaterials 135, 62–73. doi:10.1016/j.biomaterials.2017.05.003
Gao, Y., Ikeda-Imafuku, M., Zhao, Z., Joshi, M., and Mitragotri, S. (2023). A polymer-based systemic hemostat for managing uncontrolled bleeding. Bioeng. Transl. Med. 8 (3), 1–11. doi:10.1002/btm2.10516
Gao, Y., Sarode, A., Kokoroskos, N., Ukidve, A., Zhao, Z., Guo, S., et al. (2020). A polymer-based systemic hemostatic agent. Sci. Adv. 6 (31), 1–12. doi:10.1126/sciadv.aba0588
Gauer, J. S., Ajjan, R. A., and Ariëns, R. A. S. (2022). Platelet–neutrophil interaction and thromboinflammation in diabetes: considerations for novel therapeutic approaches. J. Am. Heart Assoc. 11 (20), 1–14. doi:10.1161/JAHA.122.027071
Girish, A., Jolly, K., Alsaadi, N., de la Fuente, M., Recchione, A., An, R., et al. (2022). Platelet-inspired intravenous nanomedicine for injury-targeted direct delivery of thrombin to augment hemostasis in Coagulopathies. ACS Nano 16 (10), 16292–16313. doi:10.1021/acsnano.2c05306
Greineder, C. F., Howard, M. D., Carnemolla, R., Cines, D. B., and Muzykantov, V. R. (2013). Advanced drug delivery systems for antithrombotic agents. Blood 122 (9), 1565–1575. doi:10.1182/blood-2013-03-453498
Guedes, M., Vieira, S. F., Reis, R. L., Ferreira, H., and Neves, N. M. (2021). Fishroesomes as carriers with antioxidant and anti-inflammatory bioactivities. Biomed. Pharmacother. 140 (March), 111680. doi:10.1016/j.biopha.2021.111680
Guo, Y., Patil, N. K., Luan, L., Bohannon, J. K., and Sherwood, E. R. (2018). The biology of natural killer cells during sepsis. Immunology 153 (2), 190–202. doi:10.1111/imm.12854
Gurung, S., Perocheau, D., Touramanidou, L., and Baruteau, J. (2021). The exosome journey: from biogenesis to uptake and intracellular signalling. Cell Commun. Signal. 19 (1), 47–19. doi:10.1186/s12964-021-00730-1
Hahm, J., Kim, J., and Park, J. (2021). Strategies to enhance extracellular vesicle production. Tissue Eng. Regen. Med. 18 (4), 513–524. doi:10.1007/s13770-021-00364-x
Harm, S., Lohner, K., Fichtinger, U., Schildböck, C., Zottl, J., and Hartmann, J. (2019). Blood compatibility—an important but often forgotten aspect of the characterization of antimicrobial peptides for clinical application. Int. J. Mol. Sci. 20 (21), 5426. doi:10.3390/ijms20215426
Helms, J., Iba, T., Connors, J. M., Gando, S., Levi, M., Meziani, F., et al. (2023). How to manage Coagulopathies in critically ill patients. Intensive Care Med. 49 (3), 273–290. doi:10.1007/s00134-023-06980-6
Hemmingsen, L., Giordani, B., Pettersen, A. K., Vitali, B., Basnet, P., and Škalko-Basnet, N. (2021). Liposomes-in-Chitosan hydrogel boosts potential of chlorhexidine in biofilm eradication in vitro. Carbohydr. Polym. 262, 117939. doi:10.1016/j.carbpol.2021.117939
Huang, M., Cai, S., and Su, J. (2019). The pathogenesis of sepsis and potential therapeutic targets. Int. J. Mol. Sci. 20 (21), 5376. doi:10.3390/ijms20215376
Huang, Y., Gu, B., Salles-Crawley, I. I., Taylor, K. A., Yu, L., Ren, J., et al. (2021). Fibrinogen-mimicking, multiarm nanovesicles for human thrombus-specific delivery of tissue plasminogen activator and targeted thrombolytic therapy. Sci. Adv. 7 (23), eabf9033. doi:10.1126/sciadv.abf9033
Jackson, S. P., Darbousset, R., and Schoenwaelder, S. M. (2019). Thromboinflammation: challenges of therapeutically targeting coagulation and other host defense mechanisms. Blood 133 (9), 906–918. doi:10.1182/blood-2018-11-882993
Jin, L., Chen, C., Li, Y., Yuan, F., Gong, R., Wu, J., et al. (2019). A biodegradable Mg-based alloy inhibited the inflammatory response of THP-1 cell-derived macrophages through the TRPM7–PI3K–AKT1 signaling Axis. Front. Immunol. 10 (December), 1–15. doi:10.3389/fimmu.2019.02798
Kadir, R. R. A., and Bayraktutan, U. (2020). Urokinase plasminogen activator: a potential thrombolytic agent for ischaemic stroke. Cell. Mol. Neurobiol. 40 (3), 347–355. doi:10.1007/s10571-019-00737-w
Karami, Z., Mehrzad, J., Akrami, M., and Hosseinkhani, S. (2023). Anti-inflammation-based treatment of atherosclerosis using gliclazide-loaded biomimetic nanoghosts. Sci. Rep. 13 (1), 13880–13912. doi:10.1038/s41598-023-41136-y
Kelley, W. J., Safari, H., Lopez-Cazares, G., and Eniola-Adefeso, O. (2016). Vascular-targeted nanocarriers: design considerations and strategies for successful treatment of atherosclerosis and other vascular diseases. Wiley Interdiscip. Rev. Nanomedicine Nanobiotechnology 8 (6), 909–926. doi:10.1002/wnan.1414
Kemp, M. M., and Linhardt, R. J. (2010). Heparin-based nanoparticles. WIRES Nanomed Nanobiotechnol 2, 77–87. doi:10.1002/wnan.68
Kim, S., Rho, S. J., Song, S. H., and Kim, C. H., (2021). Biocompatible N-Acetyl-Nanoconstruct alleviates lipopolysaccharide-induced acute lung injury in vivo. Sci. Rep. 11 (1), 22662–22712. doi:10.1038/s41598-021-01624-5
Kim, W., Haller, C., Dai, E., Wang, X., Hagemeyer, C. E., Liu, D. R., et al. (2015). Targeted antithrombotic protein micelles. Angew. Chem. - Int. Ed. 54 (5), 1461–1465. doi:10.1002/anie.201408529
Kolesnikova, T., Skirtach, A. G., and Möhwald, H. (2013). Red blood cells and polyelectrolyte multilayer capsules: natural carriers versus polymer-based drug delivery vehicles. Expert Opin. Drug Deliv. 10 (1), 47–58. doi:10.1517/17425247.2013.730516
Kong, F., Lee, B. H., and Kun, W. (2019). 5-Hydroxymethylfurfural mitigates lipopolysaccharide-stimulated inflammation via suppression of MAPK, NF-κb and MTOR activation in RAW 264.7 cells. Molecules 24 (2), 275. doi:10.3390/molecules24020275
Kurtuldu, F., Kaňková, H., Beltrán, A. M., Liverani, L., Galusek, D., and Boccaccini, A. R. (2021). Anti-inflammatory and antibacterial activities of cerium-containing mesoporous bioactive glass nanoparticles for drug-free biomedical applications. Mater. Today Bio 12, 100150. doi:10.1016/j.mtbio.2021.100150
Kurtuldu, F., Mutlu, N., Boccaccini, A. R., and Galusek, D. (2022). Gallium containing bioactive materials: a review of anticancer, antibacterial, and osteogenic properties. Bioact. Mater. 17 (October 2021), 125–146. doi:10.1016/j.bioactmat.2021.12.034
Landsem, A., Emblem, Å., Lau, C., Christiansen, D., Gerogianni, A., Karlsen, B. O., et al. (2022). Complement C3b contributes to Escherichia coli-induced platelet aggregation in human whole blood. Front. Immunol. 13 (December), 1–16. doi:10.3389/fimmu.2022.1020712
Lasola, J., Kamdem, H., McDaniel, M. W., and Pearson, R. M. (2020). Biomaterial-driven immunomodulation: cell biology-based strategies to mitigate severe inflammation and sepsis. Front. Immunol. 11, 1726. doi:10.3389/fimmu.2020.01726
Lee, M. R., Kim, J., Park, J., Choi, J., Song, B., Choi, Y., et al. (2020). Protective role of fermented mulberry leave extract in LPS-induced inflammation and autophagy of RAW264.7 macrophage cells. Mol. Med. Rep. 22 (6), 4685–4695. doi:10.3892/mmr.2020.11563
Luque, G. C., Moya, M., Picchio, M. L., Bagnarello, V., Valerio, I., Bolaños, J., et al. (2023). Polyphenol iongel patches with antimicrobial, antioxidant and anti-inflammatory properties. Polymers 15 (5), 1076. doi:10.3390/polym15051076
Lutz, H., Hu, S., Dinh, P. U., and Cheng, K. (2019). Cells and cell derivatives as drug carriers for targeted delivery. Med. Drug Discov. 3, 100014. doi:10.1016/j.medidd.2020.100014
Lutz, H., Popowski, K. D., Dinh, P. U. C., and Cheng, K. (2021). Advanced nanobiomedical approaches to combat coronavirus disease of 2019. Adv. NanoBiomed Res. 1 (3), 2000063. doi:10.1002/anbr.202000063
Ma, J., Chen, X., Wang, X., Liang, J., Guo, L., Su, Y., et al. (2023). The accuracy of soluble urokinase-type plasminogen activator receptor for the diagnosis of neonatal sepsis: a meta-analysis. Front. Med. 10 (April), 1–9. doi:10.3389/fmed.2023.1169114
Maisha, N., Coombs, T., and Lavik, E. (2020). Development of a sensitive assay to screen nanoparticles in vitro for complement activation. ACS Biomaterials Sci. Eng. 6 (9), 4903–4915. doi:10.1021/acsbiomaterials.0c00722
Maisha, N., Naik, N., Okesola, M., Coombs, T., Zilberberg, R., Pandala, N., et al. (2021). Engineering PEGylated polyester nanoparticles to reduce complement-mediated infusion reaction. Bioconjugate Chem. 32 (10), 2154–2166. doi:10.1021/acs.bioconjchem.1c00339
Marsh, J. N., Hu, G., Scott, M. J., Zhang, H., Goette, M. J., Gaffney, P. J., et al. (2011). A fibrin-specific thrombolytic nanomedicine approach to acute ischemic stroke. Nanomedicine 6 (4), 605–615. doi:10.2217/nnm.11.21
Meng, D., Luo, M., and Liu, B. (2021). The role of CLEC-2 and its ligands in thromboinflammation. Front. Immunol. 12 (June), 688643. doi:10.3389/fimmu.2021.688643
Michel, T., Hentges, F., and Zimmer, J. (2012). Consequences of the crosstalk between monocytes/macrophages and natural killer cells. Front. Immunol. 3 (JAN), 403–406. doi:10.3389/fimmu.2012.00403
Mihalko, E. P., Nellenbach, K., Krishnakumar, M., Moiseiwitsch, N., Sollinger, J., Cooley, B. C., et al. (2022). Fibrin-specific poly(N-isopropylacrylamide) nanogels for targeted delivery of tissue-type plasminogen activator to treat thrombotic complications are well tolerated in vivo. Bioeng. Transl. Med. 7 (2), 1–16. doi:10.1002/btm2.10277
Mihalko, E. P., Sandry, M., Mininni, N., Nellenbach, K., Deal, H., Daniele, M., et al. (2021). Fibrin-modulating nanogels for treatment of disseminated intravascular coagulation. Blood Adv. 5 (3), 613–627. doi:10.1182/bloodadvances.2020003046
Muro, S., Garnacho, C., Champion, J. A., Leferovich, J., Gajewski, C., Schuchman, E. H., et al. (2008). Control of endothelial targeting and intracellular delivery of therapeutic enzymes by modulating the size and shape of ICAM-1-targeted carriers. Mol. Ther. 16 (8), 1450–1458. doi:10.1038/mt.2008.127
Muzykantov, V. R. (2013). Drug delivery carriers on the fringes: natural red blood cells versus synthetic multilayered capsules. Expert Opin. Drug Deliv. 10 (1), 1–4. doi:10.1517/17425247.2013.750292
Nandi, S., Mihalko, E., Nellenbach, K., Castaneda, M., Schneible, J., Harp, M., et al. (2021). Synthetic platelet microgels containing fibrin knob B mimetic motifs enhance clotting responses. Adv. Ther. 4 (5), 2100010. doi:10.1002/adtp.202100010
Nedelec, J. M., Courtheoux, L., Jallot, E., Kinowski, C., Lao, J., Laquerriere, P., et al. (2008). Materials doping through sol-gel chemistry: a little something can make a big difference. J. Sol-Gel Sci. Technol. 46 (3), 259–271. doi:10.1007/s10971-007-1665-0
Ng, C. Y., Kee, L. T., Al-Masawa, M. E., Lee, Q. H., Subramaniam, T., Kok, D., et al. (2022). Scalable production of extracellular vesicles and its therapeutic values: a review. Int. J. Mol. Sci. 23 (14), 7986. doi:10.3390/ijms23147986
Nunoi, H., Xie, P., Nakamura, H., Aratani, Y., Fang, J., Nishimura, T., et al. (2022). Treatment with polyethylene glycol–conjugated fungal d-amino acid oxidase reduces lung inflammation in a mouse model of chronic granulomatous disease. Inflammation 45 (4), 1668–1679. doi:10.1007/s10753-022-01650-z
Perrella, G., Nagy, M., Watson, S. P., and Heemskerk, J. W. (2021). Platelet GPVI (glycoprotein VI) and thrombotic complications in the venous system. Arteriosclerosis, Thrombosis, Vasc. Biol. 41 (11), 2681–2692. doi:10.1161/ATVBAHA.121.316108
Peter, K., Graeber, J., Kipriyanov, S., Zewe-Welschof, M., Runge, M. S., Kubler, W., et al. (2000). Construction and functional evaluation of a single-chain antibody fusion protein with fibrin targeting and thrombin inhibition after activation by factor xa. Circulation 101 (10), 1158–1164. doi:10.1161/01.CIR.101.10.1158
Petroková, H., Mašek, J., Kuchař, M., Vítečková Wünschová, A., Štikarová, J., Bartheldyová, E., et al. (2019). Targeting human thrombus by liposomes modified with anti-fibrin protein binders. Pharmaceutics 11 (12), 642. doi:10.3390/pharmaceutics11120642
Petzold, T., Zhang, Z., Ballesteros, I., Saleh, I., Polzin, A., Thienel, M., et al. (2022). Neutrophil ‘plucking’ on megakaryocytes drives platelet production and boosts cardiovascular disease. Immunity 55 (12), 2285–2299.e7. doi:10.1016/j.immuni.2022.10.001
Popowski, K., Lutz, H., Hu, S., George, A., Dinh, P.-U., and Cheng, K. (2020). Exosome therapeutics for lung regenerative medicine. J. Extracell. Vesicles 9 (1), 1785161. doi:10.1080/20013078.2020.1785161
Rafat, M., Rotenstein, L. S., You, J. O., and Auguste, D. T. (2012). Dual functionalized PVA hydrogels that adhere endothelial cells synergistically. Biomaterials 33 (15), 3880–3886. doi:10.1016/j.biomaterials.2012.02.017
Raghunathan, S., Rayes, J., and Sen Gupta, A. (2022). Platelet-inspired nanomedicine in hemostasis thrombosis and thromboinflammation. J. Thrombosis Haemostasis 20 (7), 1535–1549. doi:10.1111/jth.15734
Rudd, K. E., Johnson, S. C., Agesa, K. M., Shackelford, K. A., Tsoi, D., Kievlan, D. R., et al. (2020). Global, regional, and national sepsis incidence and mortality, 1990–2017: analysis for the global burden of disease study. Lancet 395 (10219), 200–211. doi:10.1016/S0140-6736(19)32989-7
Runge, M. S., Bode, C., Matsueda, G. R., and Haber, E. (1987). Antibody-enhanced thrombolysis: targeting of tissue plasminogen activator in vivo. Proc. Natl. Acad. Sci. U. S. A. 84 (21), 7659–7662. doi:10.1073/pnas.84.21.7659
Sanrattana, W., Smits, S., Barendrecht, A. D., van Kleef, N. D., El Otmani, H., Zivkovic, M., et al. (2022). Targeted serpin (TaSER): a dual-action antithrombotic agent that targets platelets for serpin delivery. J. Thrombosis Haemostasis 20 (2), 353–365. doi:10.1111/jth.15554
Sekhon, U., Swingle, K., Girish, A., Luc, N., de la Fuente, M., Alvikas, J., et al. (2022). Platelet-mimicking procoagulant nanoparticles augment hemostasis in animal models of bleeding. Sci. Transl. Med. 14 (629), 1–12. doi:10.1126/scitranslmed.abb8975
Semeraro, N., Ammollo, C. T., Semeraro, F., and Colucci, M. (2010). Sepsis-associated disseminated intravascular coagulation and thromboembolic disease. Mediterr. J. Hematol. Infect. Dis. 2 (3), e2010024. doi:10.4084/MJHID.2010.024
Senchenkova, E. Y., Ansari, J., Becker, F., Vital, S. A., Al-Yafeai, Z., Sparkenbaugh, E. M., et al. (2019). Novel role for the AnxA1-fpr2/ALX signaling Axis as a key regulator of platelet function to promote resolution of inflammation. Circulation 140 (4), 319–335. doi:10.1161/CIRCULATIONAHA.118.039345
Sharma, A., Ray, S., Mamidipalli, R., Jain, R., Ghalaut, M. S., and Choudhury, S. (2020). A comparative study of the diagnostic and prognostic utility of soluble urokinase-type plasminogen activator receptor and procalcitonin in patients with sepsis and systemic inflammation response syndrome. Indian J. Crit. Care Med. 24 (4), 245–251. doi:10.5005/jp-journals-10071-23385
Shi, C., Wang, X., Wang, L., Meng, Q., Guo, D., Chen, L., et al. (2020). A nanotrap improves survival in severe sepsis by attenuating hyperinflammation. Nat. Commun. 11 (1), 3384–3413. doi:10.1038/s41467-020-17153-0
Singer, M., Deutschman, C. S., Seymour, C. W., Shankar-Hari, M., Annane, D., Bauer, M., et al. (2016). The third international consensus definitions for sepsis and septic shock (Sepsis-3). JAMA 315 (8), 801–810. doi:10.1001/jama.2016.0287
Sloos, P. H., Vulliamy, P., van 't Veer, C., Gupta, A. S., Neal, M. D., Brohi, K., et al. (2022). Platelet dysfunction after trauma: from mechanisms to targeted treatment. Transfusion 62 (S1), S281–S300. doi:10.1111/trf.16971
Sobczynski, D. J., Fish, M. B., Fromen, C. A., and Carasco-Teja, M. (2017). Drug carrier interaction with blood: a critical aspect for highefficient vascular-targeted drug delivery systems. Physiology Behav. 176 (5), 139–148. doi:10.4155/TDE.15.38
Sun, M., and Gupta, A. S. (2020). Vascular nanomedicine: current status, opportunities, and challenges. Seminars Thrombosis Hemostasis 46 (5), 524–544. doi:10.1055/s-0039-1692395
Sun, X., Liu, Z., Welsher, K., Robinson, J. T., Goodwin, A., Zaric, S., et al. (2008). Nano-graphene oxide for cellular imaging and drug delivery. Nano Res. 1 (3), 203–212. doi:10.1007/s12274-008-8021-8
Tan, Q., He, L., Meng, X., Wang, W., Pan, H., Yin, W., et al. (2021). Macrophage biomimetic nanocarriers for anti-inflammation and targeted antiviral treatment in COVID-19. J. Nanobiotechnology 19 (1), 173–216. doi:10.1186/s12951-021-00926-0
Tang, T. T., Lv, L. L., Wang, B., Cao, J. Y., Feng, Y., Li, Z. L., et al. (2019). Employing macrophage-derived microvesicle for kidney-targeted delivery of dexamethasone: an efficient therapeutic strategy against renal inflammation and fibrosis. Theranostics 9 (16), 4740–4755. doi:10.7150/thno.33520
Tanne, D., Kasner, S. E., Demchuk, A. M., Koren-Morag, N., Hanson, S., Grond, M., et al. (2002). Markers of increased risk of intracerebral hemorrhage after intravenous recombinant tissue plasminogen activator therapy for acute ischemic stroke in clinical practice: the multicenter rt-PA acute stroke survey. Circulation 105 (14), 1679–1685. doi:10.1161/01.CIR.0000012747.53592.6A
Thorén, F. B., Riise, R. E., Ousbäck, J., Della Chiesa, M., Alsterholm, M., Marcenaro, E., et al. (2012). Human NK cells induce neutrophil apoptosis via an NKp46-and fas-dependent mechanism. J. Immunol. 188 (4), 1668–1674. doi:10.4049/jimmunol.1102002
Vagionas, D., Papadakis, D. D., Politou, M., Koutsoukou, A., and Vasileiadis, I. (2022). Thromboinflammation in sepsis and heparin: a review of literature and pathophysiology. Vivo 36 (6), 2542–2557. doi:10.21873/invivo.12991
Van Der Meel, R., Fens, M. H., Vader, P., van Solinge, W. W., Eniola-Adefeso, O., and Schiffelers, R. M. (2014). Extracellular vesicles as drug delivery systems: lessons from the liposome field. J. Control. Release 195, 72–85. doi:10.1016/j.jconrel.2014.07.049
Vishwanath, N., Whitaker, C., Allu, S., Clippert, D., Jouffroy, E., Hong, J., et al. (2022). Silver as an antibiotic-independent antimicrobial: review of current formulations and clinical relevance. Surg. Infect. 23 (9), 769–780. doi:10.1089/sur.2022.229
Vuong, T. T., Rønning, S. B., Suso, H. P., Schmidt, R., Prydz, K., Lundström, M., et al. (2017). The extracellular matrix of eggshell displays anti-inflammatory activities through NF-κB in LPS-triggered human immune cells. J. Inflamm. Res. 10, 83–96. doi:10.2147/JIR.S130974
Wang, J., Hajizadeh, N., Moore, E. E., McIntyre, R. C., Moore, P. K., Veress, L. A., et al. (2020). Tissue plasminogen activator (TPA) treatment for COVID-19 associated acute respiratory distress syndrome (ARDS): a case series. J. Thrombosis Haemostasis 18 (7), 1752–1755. doi:10.1111/jth.14828
Weiss, S. L., Peters, M. J., Alhazzani, W., Agus, M. S. D., Flori, H. R., Inwald, D. P., et al. (2020). Surviving sepsis campaign international guidelines for the management of septic shock and sepsis-associated organ dysfunction in children. Intensive Care Med. 46 (s1), 10–67. doi:10.1007/s00134-019-05878-6
WHO (2023). Sepsis. World Health Organization. 2023. Available at: https://www.who.int/news-room/fact-sheets/detail/sepsis.
Witwer, K. W., and Wolfram, J. (2021). Extracellular vesicles versus synthetic nanoparticles for drug delivery. Nat. Rev. Mater. 6 (2), 103–106. doi:10.1038/s41578-020-00277-6
Wu, H., Wang, Y., Zhang, Y., Xu, F., Chen, J., Duan, L., et al. (2020). Breaking the vicious loop between inflammation, oxidative stress and coagulation, a novel anti-thrombus insight of nattokinase by inhibiting LPS-induced inflammation and oxidative stress. Redox Biol. 32 (March), 101500. doi:10.1016/j.redox.2020.101500
Xu, X., Huang, X., Zhang, Y., Shen, S., Feng, Z., Dong, H., et al. (2020). Self-regulated hirudin delivery for anticoagulant therapy. Sci. Adv. 6 (41), 1–10. doi:10.1126/sciadv.abc0382
Yan, F., Zhong, Z., Wang, Y., Feng, Y., Mei, Z., Li, H., et al. (2020). Exosome-based biomimetic nanoparticles targeted to inflamed joints for enhanced treatment of rheumatoid arthritis. J. Nanobiotechnology 18 (1), 115–15. doi:10.1186/s12951-020-00675-6
Yan, X., Fang, W. W., Xue, J., Sun, T. C., Dong, L., Zha, Z., et al. (2019). Thermoresponsive in situ forming hydrogel with sol-gel irreversibility for effective methicillin-resistant Staphylococcus aureus infected wound healing. ACS Nano 13 (9), 10074–10084. doi:10.1021/acsnano.9b02845
Ye, W., Wang, N., Hu, K., Zhang, L., Liu, A., Pan, C., et al. (2018). Bio-inspired microcapsule for targeted antithrombotic drug delivery. RSC Adv. 8 (48), 27253–27259. doi:10.1039/c8ra04273j
Yu, M., Hong, K., Adili, R., Mei, L., Liu, L., He, H., et al. (2022). Development of activated endothelial targeted high-density lipoprotein nanoparticles. Front. Pharmacol. 13 (August), 1–14. doi:10.3389/fphar.2022.902269
Zhang, M., Chen, H., Zhang, W., Liu, Y., Ding, L., Gong, J., et al. (2023). Biomimetic remodeling of microglial riboflavin metabolism ameliorates cognitive impairment by modulating neuroinflammation. Adv. Sci. 10 (12), 1–21. doi:10.1002/advs.202300180
Zhang, Z., Yan, T., Ren, D., Zhou, J., Liu, L., Li, J., et al. (2023). Low-molecular-weight heparin therapy reduces 28-day mortality in patients with sepsis-3 by improving inflammation and coagulopathy. Front. Med. 10, 1157775. doi:10.3389/fmed.2023.1157775
Zou, Z., Huang, J. j., Luan, Y. y., Yang, Z. j., Zhou, Z. p., Zhang, J. j., et al. (2022). Early prophylactic anticoagulation with heparin alleviates mortality in critically ill patients with sepsis: a retrospective analysis from the MIMIC-IV database. Burns Trauma 10, tkac029. doi:10.1093/burnst/tkac029
Keywords: biomaterials, sepsis, inflammation, hemostasis, thromboinflammation, targeted delivery
Citation: Lutz H and Brown AC (2023) Biomaterials for treating sepsis-induced thromboinflammation. Front. Front. Biomater. Sci. 2:1305379. doi: 10.3389/fbiom.2023.1305379
Received: 01 October 2023; Accepted: 21 November 2023;
Published: 07 December 2023.
Edited by:
Silviya Petrova Zustiak, Saint Louis University, United StatesReviewed by:
Elizabeth Cosgriff-Hernandez, The University of Texas at Austin, United StatesCopyright © 2023 Lutz and Brown. This is an open-access article distributed under the terms of the Creative Commons Attribution License (CC BY). The use, distribution or reproduction in other forums is permitted, provided the original author(s) and the copyright owner(s) are credited and that the original publication in this journal is cited, in accordance with accepted academic practice. No use, distribution or reproduction is permitted which does not comply with these terms.
*Correspondence: Ashley C. Brown, YWVjYXJzbzJAbmNzdS5lZHU=
Disclaimer: All claims expressed in this article are solely those of the authors and do not necessarily represent those of their affiliated organizations, or those of the publisher, the editors and the reviewers. Any product that may be evaluated in this article or claim that may be made by its manufacturer is not guaranteed or endorsed by the publisher.
Research integrity at Frontiers
Learn more about the work of our research integrity team to safeguard the quality of each article we publish.