- 1Department of Mechanical and Aerospace Engineering, Politecnico di Torino, Torino, Italy
- 2POLITO BioMedLab, Politecnico di Torino, Torino, Italy
- 33R, Interuniversity Center for the Promotion of the 3Rs Principles in Teaching and Research, Torino, Italy
- 4Department for Materials and Devices of the National Research Council, Institute for the Chemical and Physical Processes (CNR-IPCF UOS), Pisa, Italy
Environmental sustainability is a key challenge driven by the increased consumption of natural resources with limited availability. In this scenario agriculture has emerged as a privileged source of renewable resources, hence more efforts should be addressed to the study of plant-derived materials for medical applications. Zein is a biocompatible, biodegradable and amphiphilic prolamin protein extracted from the endosperm tissue of corn. For these reasons, its applications span from coatings for edible capsules, to the fabrication of bi- and tridimensional scaffolds for tissue engineering, and to develop drug delivery systems. This review aims at describing the properties and main applications of zein with a focus on the most recent and updated state of the art literature on zein based nanoparticles for the controlled delivery of various drugs. The main focus is to analyze the state of the art literature to understand how to implement sustainable methods for the preparation of zein NPs and to propose their exploitation as novel drug delivery systems for multiple applications, including oligonucleotide delivery. Main methods for zein NP preparation are described under an ecofriendly point of view, highlighting their environmental sustainability based on used solvents, waste products and energy consumption.
1 Introduction
The continuous growth of the world population (that according to predictions will reach around 8.5 billion in 2030 and 9.7 billion in 2050) has tremendously increased the demand for natural resources, which however show limited availability (Pimentel and Pimentel, 2006; FΑΟ, 2013; Gaigbe-Togbe et al., 2022). Based on that, new environmentally-sustainable materials and technologies should be urgently developed (Chakrapani et al., 2022; Tamasiga et al., 2022), following the “12 Principles of Green Chemistry” (Anastas and Eghbali, 2010; Duarte et al., 2014) and the “12 Principles of Green Engineering” (Brennecke, 2004) (Table 1). In this scenario, one main target is the shift from linear to circular economy, a new model of production and consumption based on the recycle and reuse of waste, aimed at the preservation of ecosystems and biodiversity. Based on the circular economy model, produced waste should be converted into renewable resources leading to the elimination of the concept of “waste” itself (Zimmerman et al., 2020).
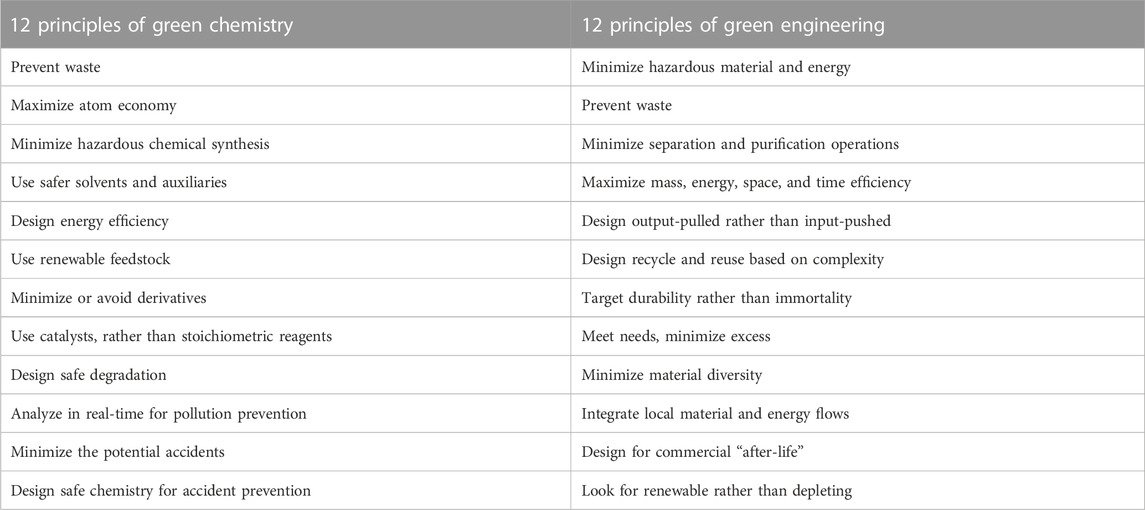
TABLE 1. The 12 principles of green chemistry (Anastas and Eghbali, 2010) and engineering (Brennecke, 2004).
Waste materials from agriculture (i.e., plant-derived materials) are particularly promising as green resources due to their high availability, renewability, low-cost, and potential exploitation in the food industry (for example, zein from corn crop waste as edible carriers for flavor compounds, to deliver food colorants or to give luster to the coating materials in confectionery products) (Kasaai, 2018), as well as for biomedical and pharmaceutical applications (for example, pectin from juice, apple, and cider waste industries or starch from cereal and potato waste for bioprinting applications) (Jovic et al., 2019; Chakrapani et al., 2022). Plant-derived materials include: proteins (such as wheat gluten, soy and zein), polysaccharides (such as pectin, cellulose and starch), lignin and plant extracts rich in polyphenols (such as Aloe Vera) (Iravani and Varma, 2019). Among them, plant-derived proteins show promising properties for applications in drug delivery and tissue engineering (Akharume et al., 2021; Tortorella et al., 2021). Cereals by-products provide a valuable source of prolamins, which are the main storage proteins present in most cereals (Song et al., 2021). Among them, herein we focus on zein, which is the major storage protein present in the cytoplasm of endosperm cells of maize (Zea mays), constituting almost 50% of corn proteins (Corradini et al., 2014). Other cereals, such as wheat, barley and sorghum are sources for other plant-derived proteins, respectively gliadin, hordein and karifin, with similar properties to zein. However, compared to other plant-derived proteins, zein is advantageous due to the high availability of materials for its extraction (from agricultural waste) and high extraction yield (Anderson and Lamsal, 2011). Indeed, corn is the main global staple cereal in terms of annual production, exceeding 1 billion metric tons and expected to rise with an estimated annual increase of 37 million tons (Erenstein et al., 2022). Feedstock for zein extraction can be obtained from a primary process (including agricultural crops) or from a secondary process (which includes agro-industrial waste resulting from the processing of maize as a by-product by wet-milling or dry-milling) (Tapia-Hernández et al., 2019). Prolamin extraction is generally carried out by washing with aqueous ethanol; however, sustainable and innovative protocols for zein extraction have recently been developed to minimize environmental impact of prolamin processing, reducing energy consumption and solvent volumes (Chen et al., 2022a). Zein is also characterized by other favorable properties, such as biocompatibility, biodegradability and amphiphilicity, which is responsible for its interaction with both hydrophobic and hydrophilic materials and/or drugs. Zein has also been certified as Generally Recognized As Safe (GRAS) in the 1985 and approved as safe biomaterial for medical use by the Food and Drug Administration (FDA) (Weissmueller et al., 2016; Labib, 2018). Previous literature has reported the use of zein for the production of edible capsules (Bouman et al., 2016), films (Wang et al., 2005; Zhang et al., 2015; Hasanzadeh et al., 2021), hydrogels (Gao et al., 2007), scaffolds for tissue engineering (Torres-Giner et al., 2009; Ali et al., 2014; Khatri et al., 2020) and drug-loaded NPs (Abdelsalam et al., 2021). Due to its promising properties, zein has been the subject of previous review papers, focused on zein extraction and general applications (Shukla and Cheryan, 2001; Anderson and Lamsal, 2011), as well as zein biomedical applications (Corradini et al., 2014; Paliwal and Palakurthi, 2014; Pérez-Guzmán and Castro-Muñoz, 2020; Tortorella et al., 2021) including the design of zein-based NPs (Zhang et al., 2016; Abdelsalam et al., 2021; Yuan et al., 2022a; Yuan et al., 2022b). Zein NPs are advantageous over lipid and synthetic polymer NPs due to their superior biocompatibility, while compared to NP based on animal-derived proteins, they are not affected by the risk for zoonotic disease transmission (Reddy and Yang, 2011; Podaralla and Perumal, 2012). Furthermore zein is amphiphilic, as composed by hydrophobic and neutral amino acids, such as leucine, proline and alanine, and by hydrophilic glutamine residues (Shukla and Cheryan, 2001). Zein amphiphilicity affects its solubility and drug loading ability: 1) zein is insoluble in water and soluble in water-alcohol, ketone and highly concentrated salt and alkaline solutions; 2) zein NPs can encapsulate both hydrophobic and hydrophilic drugs. However, in most studies zein NPs have been used for the release of food and nutrient components (Kasaai, 2018), and lipophilic drugs, such as curcumin (Shukla and Cheryan, 2001), gallic acid (Wiggers et al., 2022), gitoxin (Muthuselvi and Dhathathreyan, 2006) and fluorouracil (Lai and Guo, 2011). Only a few recent reports have proposed the encapsulation of hydrophilic drugs (e.g., doxorubicin and chloroquine) (Dong et al., 2016; Chen X. et al., 2022) or plasmid DNA (El Sharkawi et al., 2017) into zein NPs. Such recent studies exploit the possibility to vary zein charge depending on the pH to promote its electrostatic interactions with hydrophilic molecules with opposite charge, enhancing drug encapsulation efficiency. Furthermore, surface coatings have been applied on zein NPs to prepare core-shell nanocarriers with specific properties, including enhanced stability to centrifugation and freeze-drying (Tortorella et al., 2021). As zein has the ability to protect labile drugs from degradation and is more resistant to degradation than other proteins, it has been widely applied for oral drug delivery (Luo et al., 2010; Lee et al., 2013). However other routes of administration, such as nasal, transdermal delivery, intramuscular, intravenous and subcutaneous delivery have also been exploited (Hurtado-López and Murdan, 2010; Lai and Guo, 2011; Li H. et al., 2019; Yu et al., 2022).
This review provides an initial overview on the chemistry and structure of zein molecules as preliminary to a focus on the use of zein as a green material in the field of nanomedicine, exploiting sustainable preparation processes. Hence, novelty of this review derives from focusing on zein as a byproduct material from agricultural waste, with proper physicochemical properties for the preparation of added-value pharmaceutical products under environmentally friendly production processes. To this aim, methods to prepare zein NPs are initially described and their sustainability is discussed, in terms of solvent types and ratio, consumed energy and produced waste. The possibility to exploit zein NPs for the loading and release of hydrophilic drugs, such as oligonucleotides, is also discussed, taking advantage of the superior biocompatibility of zein over state of the art materials for nanocarrier production. Potential issues associated with the in vivo administration of zein NPs, such as immune response, are also critically discussed (Zhang et al., 2016).
Extensive state of the art research has been carried out, covering a wide range of publications with a focus between 2017 and 2022 to highlight the most innovative preparation processes and applications of zein in the drug delivery field. However, older literature was also considered for the analysis of zein physicochemical characteristics. The main databases employed for state of the art research were Science Direct, PubMed and Scopus with a focus on keywords such as “zein nanoparticles” and “green drug delivery systems”. The innovative contribution of this review paper is to inspire the future application of zein derived from agricultural waste for the preparation of green NPs by sustainable methods, and to encourage their use in advanced therapies, such as the release of RNA molecules.
2 Chemical and molecular structure of zein
2.1 Composition of plant-derived zein
Zein has a peculiar aminoacidic composition, with a prevalent proportion of nonpolar amino acids respect to polar ones. Hydrophobic and neutral amino acid residues include a high percentage of leucine (∼20%), proline (∼10%) and alanine (∼10%), while hydrophilic residues have a content of approximately 21%–26% glutamic acid (Abdelsalam et al., 2021). Notably, the following amino acids are present at very low amounts: lysine (∼0%), tryptophan (∼0.16%), arginine (∼5%) and histidine (∼1%) (Geraghty et al., 1981; Shukla and Cheryan, 2001). Overall, zein is an amphiphilic protein with an isoelectric point of 6.2–6.8, close to the physiological pH. Therefore, at physiological pH zein aggregation occurs due to enhanced hydrophilic interactions and decreased electrostatic repulsions. On the other hand, at low and high pH zein is charged: at acidic pH it acquires an overall positive charge while at basic pH it becomes negatively charged. Therefore, zein NPs are often exploited for oral delivery of compounds to prevent their degradation in the harsh gastrointestinal tract (GIT) conditions because at acidic pH they remain stable against aggregation. Zein NPs are often studied in simulated GIT fluids (at acidic pH, with pepsin) to evaluate NPs stability (Abdelsalam et al., 2021). The functionality of zein is given by its numerous functional groups, among them amines, amides, hydroxyls, carboxylates and phenols (Corradini et al., 2014). Amines from glutamine residues are more exposed on the peptide chains thanks to the tertiary structure of zein, described in the Par. 2.2. Crosslinking of zein could be obtained using chemical crosslinking agents, such as EDC/N-hydroxysuccinimide (NHS), formaldehyde, glutaraldehyde, succinic acid, citric acid and eugenol (Corradini et al., 2014; Tortorella et al., 2021). The self-crosslinking of zein molecules mediated by EDC/NHS was reported for film fabrication, linking amines with carboxyl groups (Kim et al., 2004). Another study describes zein films prepared by using succinic anhydride, succinic acid, citric acid, or eugenol, separately or together, as natural crosslinkers to increase their tensile strength (Khalil et al., 2015). Zein electrospun membranes were crosslinked through non-toxic citric acid, thus forming amido linkages between the carboxyl groups of citric acid and the amino groups of zein. Results showed higher mechanical properties, longer water stability and higher cell adhesion compared to uncrosslinked electrospun nanofibers (Jiang et al., 2010). Zein based crosslinked hydrogels were also prepared with increased absorption capacity, using N,N′-methylene bisacrylamide as the crosslinker (Ni et al., 2018), or with increased mechanical properties combining chemical (through glutaraldehyde, linking amine and aldehyde groups) and physical crosslinking (through hydrophobic interactions and hydrogen bonding upon a post-treatment with water) (Wang et al., 2019).
Composition confers zein unique solubility behavior: zein is insoluble in pure water, while it is soluble in water-alcohol, water-acetone, highly alkaline and urea solutions, and in the presence of anionic detergents (Lawton, 2002; Deo et al., 2003; Paliwal and Palakurthi, 2014). Due to the abundance of nonpolar amino acids and the insolubility in water, zein has been initially investigated for the preparation of tough greaseproof coatings of films resistant to acid and microbial attack, adhesives for the introduction of flavors and medical tablets (Shukla and Cheryan, 2001; Liu et al., 2005; Zhong and Jin, 2009). Only recently, zein has been proposed for the preparation of NPs for controlled and sustained drug release (Zhang et al., 2016; Tran et al., 2019; Abdelsalam et al., 2021).
Zein can be classified into four main groups with different peptide composition, molecular weight, solubility and charge: a-zein, ß-zein, γ-zein and δ-zein. Zein contains a mixture of them and has an average molecular weight of 10–30 kDa. The main component is a-zein, representing around 80% of the total prolamin content present in corn. This protein fraction has an average molecular weight of 19–22 kDa and is the most abundant in commercial zein, among the four groups (Cabra et al., 2006; Cabra et al., 2007). The second most abundant fraction is ß-zein, which represents 10%–15% of zein and has an average molecular weight of 17–18 kDa. Respect to the aminoacidic composition of a-zein, ß-zein has less proline, arginine, methionine and histidine. γ-zein shows the highest molecular weight (27–28 kDa), but it constitutes only 5%–10% of zein. Finally, δ-zein is the minor fraction in zein (only 3%) and has the lowest molecular weight (9–10 kDa) (Esen, 1986; Wilson, 1991; Parris and Dickey, 2001). Among major food and pharmaceutical companies, Flo Chemical Corp (Ashburnham, MA), which is specialized in distribution of zein for various applications, and Showa Sangyo (Tokyo, Japan), that sales zein together with other food derivates, hold the record in zein commercialization (Shukla and Cheryan, 2001; Anderson and Lamsal, 2011).
2.2 From composition to structure of zein protein molecules
Literature has reported various studies aimed at elucidating the tertiary structure of a-zein, being the mostly available zein fraction (Argos et al., 1982; Garratt et al., 1993; Matsushima et al., 1997; Forato et al., 2004; Momany et al., 2006). Molecular models of a-zein tertiary structure include cylinder model, ribbon-like model, hairpin model and super-helical structural model (Figure 1). Such models approximate a-zein as composed by two main fractions with 19 kDa and 22 kDa molecular weight (referred to as Z19 and Z22, respectively), based on data on zein composition obtained from sodium dodecyl sulfate-polyacrylamide gel electrophoresis analysis (SDS-PAGE) (Lee et al., 1976).
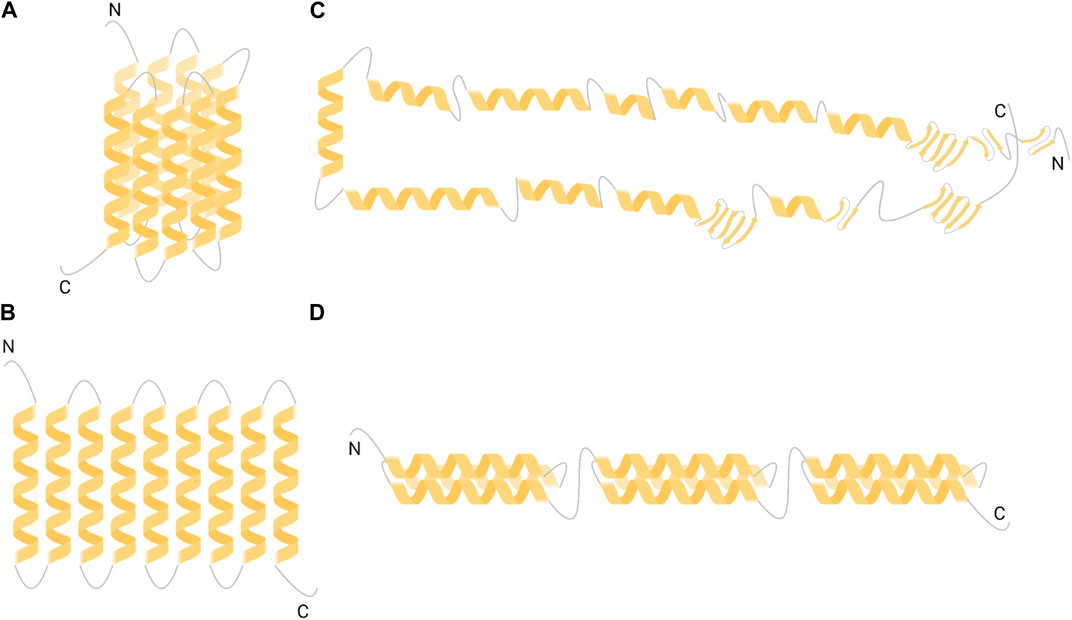
FIGURE 1. Models of a-zein tertiary structure (A) cylinder-like model (Argos et al., 1982) (B) ribbon-like model (Matsushima et al., 1997) (C) hairpin-like model (Forato et al., 2004) and (D) triple-helix model (Momany et al., 2006). Created with BioRender.com.
The cylinder model is one of the firstly developed structural models of a-zein (Figure 1A) and was derived from circular dichroism (CD) data (Argos et al., 1982). CD spectrum of a mixture of Z19 and Z22 fractions in 70% methanol aqueous solution was measured in the 190–240 nm wavelength range. Data suggested that a-zein tertiary structure prevalently consists of a-helixes (50%–60%), with turns or random coils in the remaining chain portions. Notably, a-helix content was in agreement with data from previous optical rotary dispersion measurements, performed on zein solutions in 50%–80% ethanol-water (Danzer et al., 1975). According to the cylinder model, 9 homologous repeating a-helix units are topologically arranged adjacent and antiparallel, forming a cylindrical structure with turns linking the a-helices (Figure 1A). The cylinder has an oval cross-section with the helix and cylinder axes aligned. As a-helices have mainly a hydrophobic nature, with only a few polar amino acids, the cylinder-like structure shows hydrophobic behavior except for the top and bottom surfaces, containing turns rich in hydrophilic glutamine residues. This cylindrical structure with polar glutamine-rich turns is stabilized by side chain interactions, specifically Van der Waals interactions and hydrogen bonds.
The ribbon-like model (Figure 1B) was developed based on data from small-angle X-ray scattering (SAXS) analysis, performed on a-zeins solutions in 70% v/v ethanol-water. Data suggested the presence of 9 and 10 repeating a-helix units in Z19 and Z22 respectively, joined by glutamine-rich loops (Tatham et al., 1993). The ribbon-model described by Matsushima et al. is based on the linear stacking of 9–10 adjacent antiparallel a-helices, perpendicular respect to the helical axis, and does not contain ß-sheet, forming an elongated prism-like structure with 13 × 1.2 × 3 nm3 size (Matsushima et al., 1997) (Figure 1B). Therefore, this two-dimensional ribbon-like structure is organized with hydrophilic ends and hydrophobic sides, enhancing the stability of a-zein. This slender prism model with hydrophilic extremities and hydrophobic surfaces was also employed to understand the orientations of zein molecules on hydrophilic and hydrophobic surfaces, as well as zein self-assembly from single molecules into NPs (Wang et al., 2008; Wang and Padua, 2012).
The hairpin-like model was developed, based on nuclear magnetic resonance (NMR), SAXS and Fourier transform infrared spectroscopy (FTIR) analyses, performed on Z19 fraction only (Forato et al., 2004). Particularly, FTIR analysis showed that Z19 structure contains a high content of a-helices (46%) and a limited amount of ß-sheet (22%). In the hairpin-like model, helices are connected to each other to form rings, sheets or loops, with dimensions coherent with the previous model proposed by Matsushima and coworkers (Figure 1C). This structure has the ability to refold on itself or to extend based on the employed solvent. The hairpin-like model confirmed the presence of 20 amino acids for each a-helix, as stated in previous models (Argos et al., 1982; Matsushima et al., 1997). The hairpin-like model is useful to explain the process of zein fiber formation.
More recently, a super-helical structural (or triple-helix) model of Z19 tertiary structure was developed, based on molecular mechanics and dynamic simulations, in order to overcome incoherencies between previous models and experimental data (Momany et al., 2006). In this model, each super-helix contains three antiparallel a-helices, linked together by glutamine-rich turns (Figure 1D). Probability algorithms suggested that a-zein mainly forms coiled coils rather than pure a-helices. Coiled coils show a slight curvature along the helical axis, conferring the helices a twist spatial organization. Based on the amino acid composition of a single a-helix (from 14 to 19 residues), the a-helix percentage was estimated to be 62%, which is consistent with FTIR data.
3 Zein micro and nanoparticles: towards green methods for their production
3.1 Solvent selection for zein solubilization
Solvent selection is important for zein extraction, purification and for the manufacturing of zein substrates, including products for regenerative medicine, such as scaffolds, hydrogels or particles. Generally, zein can be solubilized into three types of solvents: primary, secondary and tertiary solvents. Primary solvents are single solvents able to dissolve zein such as glycerol and acetic acid (Evans and Manley, 1941). They generally have hydroxyl, amine, amide and carboxyl functional groups. Indeed, solvents with the above characteristics display balanced polar and nonpolar features and are thus able to dissolve amphiphilic zein. Typically, primary solvents produce more stable zein solutions than binary solvents containing additional water (Evans and Manley, 1941; Anderson and Lamsal, 2011). On the other hand, studies on zein solubility showed its improvement in binary solvent mixtures by introducing water (Dill, 1927; Swallen, 1941). Different binary solvent combinations were studied and results showed that water-anhydrous alcohol, water-ketone, aromatic hydrocarbon-anhydrous alcohol, and alkaline agent-water represent effective secondary solvents for zein. For each secondary solvent, solubility varies depending on the composition of the binary mixture and on other parameters, such as temperature. An in-depth understanding of zein solubility in secondary solvent mixtures has required throughout investigations (Manley and Evans, 1943). In detail, experiments were carried out by adding minor amounts of aliphatic alcohols, ketones, or glycols to water, aromatic hydrocarbons, chlorinated hydrocarbons, nitroparaffins, aldehydes, or cyclic ethers. Results showed that zein solutions in aqueous binary mixtures show time and heat dependent behavior, with possible undesirable formation of gels or foams respect to non-aqueous systems with increasing zein and water concentration. In addition to widely used alcohol-water solvents, mixtures of acetone, acetonyl-acetone, dioxane, and dioxolane with water are also good solvents for zein. Particularly, zein solutions in acetone-water solvents have shown lower viscosities respect to solutions in other binary solvents with the same concentration. Indeed, zein solutions with 20% w/v concentration in 70% v/v acetone-water and in 70% v/v isopropyl alcohol-water showed viscosity of 20 cP and 83 cP at 30°C, respectively. Solution viscosity is an important parameter for the selection of the most appropriate solvent mixture for zein solubilization. Solution viscosity value is also relevant to assess zein absorption rate from solutions, to obtain zein coatings on substrates, such as films and NPs.
Finally, a thorough investigation of ternary solvents for zein was also conducted using both aqueous and non-aqueous mixtures (Evans and Manley, 1944). Such studies established that zein solutions in aqueous ternary solvents containing water, acetone and an aldehyde showed similar behavior to zein solutions in aqueous alcohol and aqueous aldehyde binary mixtures, but had higher resistance to gelling when at least 5% v/v aldehyde was added to the mixture because aldehydes can be employed as gelation inhibitors in aqueous zein solutions.
Despite the many studies on zein solution behavior as a function of different solvents, the effects of zein solution concentration, solvent ratio and temperature on zein conformation are still poorly known. More recent studies have focused on the understanding of the properties of zein products obtained from solutions in commonly used ethanol-water and acetic acid-water solvents (Fu and Weller, 1999; Shi et al., 2009; Zhong and Ikeda, 2012). Such research studies have found that zein films cast from ethanol-water or acetic acid-water solutions on silicon wafers have different surface morphologies and hydrophilicity, as assessed by tapping mode atomic force microscopy (TP-AFM), water contact angle measurements and X-ray photoelectron spectroscopy (XPS). In detail, zein films prepared from acetic acid-water solutions showed smoother surface and higher hydrophobicity respect to those prepared from ethanol-water solution. This behavior was explained based on the influence of acidic or basic environment on the properties of zein molecules (Zhang et al., 2011). Indeed, in acidic solution (i.e., in acetic acid-water solutions), zein molecules tend to preferentially expose their hydrophobic groups in contact with air because the silicon wafer surface contains mainly silanol groups that confer a negative charge. On the contrary, zein films prepared from ethanol-water solutions at neutral pH are more hydrophilic because they expose a large number of polar amino acid residues lining up perpendicularly to the surface, also causing higher surface roughness. Acidic or basic treatments also have a great influence on the properties of zein-coated particles, as zein coatings have stronger binding affinity and higher mass adsorption on surfaces exposing carboxyl groups rather than alkyl groups (Wang et al., 2006). The assembly and structure of zein in solutions is a complex function of solvent polarity and zein charge.
Later, another study investigated changes in zein conformation as a function of solvent chemistry using CD analysis. The secondary and tertiary structure of zein protein could be varied by the type of solvent and the temperature (Selling et al., 2007). The secondary structure was weakly affected by ethanol to water ratio variation, while the tertiary structure slightly changed in the presence of acetic acid-water solutions. Reversible changes of the primary and tertiary structures were observed by heating zein solution in 80% v/v ethanol-water up to 70°C followed by cooling. With the aim to understand protein−solvent interaction and whether zein behaves as a polyelectrolyte or differently, SAXS, rheology and CD analyses were carried out on zein solutions in acetic acid-water (Li et al., 2011). Zein solutions in acetic acid behaved similarly to polyelectrolyte solutions in good solvents, as proved by molecular measures of protein folding, such as the concentration scaling behavior which was near the theoretical predictions for polyelectrolytes. Indeed, the folding or unfolding behavior of zein may change the surface of interaction with the solvents, leading to different dissolution. Afterwards, the same research group progressed their study on zein solution properties also in ethanol-water solvents (Li et al., 2012). Protonation of zein was identified as a key parameter for its solubilization. Hence, acetic acid-water was found to improve zein solubility respect to ethanol-water, because of the more favorable zein protonation in the presence of acetic acid. They also found out that zein molecule size increased by decreasing the content of ethanol in aqueous mixtures (from 80% to 70% v/v); the largest zein size was found in solutions containing acetic acid-water solvents suggesting that zein was more unfolded or swollen in the presence of acetic acid. As predicted by the polyelectrolyte behavior, with increasing zein concentration upon 43 mg/mL, zein size slightly decreased in solutions containing acetic acid-water: this behavior was due to overlapping and mutually penetrating behavior, because of crowding and repulsive long-range electrostatic interactions among zein chains. Another study on zein conformational changes in solution was carried out more recently (Erickson et al., 2020). CD spectra of zein solutions showed that, by increasing water in ethanol-water solvents, random coils and ß-sheets began to appear instead of a-helix structures. This result was confirmed by molecular dynamics simulation performed on zein solutions in 45% and 75% v/v ethanol-water for the validation of two a-zein molecular models: the hairpin-like (Figure 1C) and the triple-helix models (Figure 1D). The hairpin-like model best represented zein conformation with increased ß-sheets in 45% v/v ethanol-water. SEM analysis showed spherical zein aggregates (0.1–10 μm) in zein solution with 45% v/v ethanol-water and this could be explained by the increase in ß-sheets occurring at higher water content.
A recent investigation on zein solubility in ethanol-water solvents evidenced the influence of amino acid composition of zein fractions (α-, ß-, γ-, and δ-zein) on the physicochemical properties and exposed functionalities of zein based products prepared from those solutions (Gao et al., 2021). In detail, the three zein fractions allowed the successful production of NPs from ethanol-water solvents, with different characteristics in terms of wettability, hydrodynamic size and surface hydrophobicity. ß-zein based NPs showed poor surface lipophilicity, as measured through an optical contact angle meter, using medium-chain triglyceride as the oil phase. a-zein NPs showed the highest surface hydrophobicity, leading to high oil-water interfacial adsorption speed. γ-zein based NPs showed low surface lipophilicity and the highest hydrodynamic size (383 ± 26 nm) compared to a-zein (207 ± 12 nm) and ß-zein NPs (155 ± 9 nm). Furthermore, respect to a-zein and ß-zein, γ-zein contains more cysteine residues forming a crosslinked structure by disulfide bonds.
Recently attention is focused on the use of the most environmentally friendly and sustainable solvents for zein. As water represents the greenest solvent, aqueous binary solvents represent the best choice for zein solubilization under environmentally-friendly conditions. Table 2 reports a list of aqueous binary mixtures used for zein solubilization (Lawton, 2002) together with their level of greenness according to the Pfizer guide (Alfonsi et al., 2008; Byrne et al., 2016). Other solvent selection guides have also been developed to classify solvents based on environmental impact, safety, waste management, effects on human health or life cycle, in order to state which solvents can be considered ‘greener’ and which ones should be replaced because of significant issues (GSK, 2010; ACS-GCI, 2011; Henderson et al., 2011; Prat et al., 2013). A comparison among the different guides shows that they are in agreement, with only slight discrepancies in few cases. Interestingly, all solvents used in aqueous binary mixtures for zein solubilization are classified as “usable”, except for dioxane.
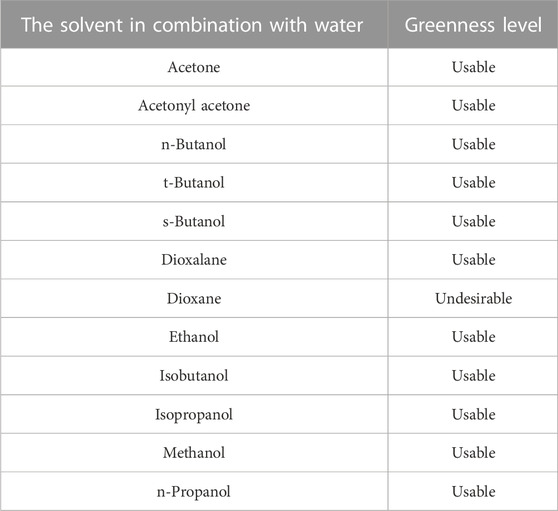
TABLE 2. List of aqueous binary solvents (Lawton, 2002) and their greenness level according to Pfizer guide (Byrne et al., 2016; Joshi and Adhikari, 2019).
Considering the wide range of possible solvents available for zein solubilization, a thorough understanding of zein molecule assembly and their phase behavior in these solvents is still lacking.
3.2 Methods for the preparation of zein nanoparticles
Various methods have been employed for the preparation of zein NPs for drug delivery. The selection of the method depends on solvent types and ratio, which are crucial to obtain specific characteristics for the nanocarriers.
Zein NPs have been generally prepared from binary solvent systems containing water, using a method that exploits the solubility of zein in various solvents and that has been firstly called phase separation method, but investigations in literature report also other names such anti-solvent precipitation (Cheng et al., 2019), liquid-liquid dispersion (Zou et al., 2012) and coacervation (Madan, 1978; Arshady, 1990; Swarbrick, 2007) methods (Yan et al., 2022). This technique induces NP formation by the dispersion of a primary phase, where zein is soluble, into a non-solvent for zein (typically water) which is miscible with the solvent. It is characterized by basic experimental settings and steps, is low-cost and usually shows high drug encapsulation efficiency, so represents the most widespread method to prepare zein NPs (Pascoli et al., 2018). Liquid-liquid dispersion has been first reported for the preparation of micro-rods (Alargova et al., 2004; Alargova et al., 2006): rods were obtained by mixing a polymer solution into an organic solvent, which was unable to dissolve the polymer while was miscible with the solvent. The same principle was then applied for the production of zein NPs (Zhong and Jin, 2009). According to this method, zein dissolved in an aqueous binary mixture (organic phase) was added to an anti-solvent (aqueous phase) under stirring. Due to the good miscibility of the used organic solvent with water, the inter-diffusion mechanism of solvents reduces the solubility of zein, leading to its precipitation into particles. Process parameters such as solvent ratio, method of injection (drop-wise or continuous addiction), and stirring speed and method (magnetic stirring, vortex, sonicator, etc.) highly affect the results, such as NP size and morphology, leading to low experimental reproducibility and scalability for large volumes. Zhong and Jin conducted an interesting research on the effects of different process parameters (shear stress, aqueous ethanol ratio and zein concentration) on zein NPs (Zhong and Jin, 2009). Effects of shear stress, by applying different stirring speeds (5,000, 10,000 and 15,000 rpm) were studied, while keeping constant ethanol to water ratio and zein concentration. DLS and SEM showed that the use of lower speeds allowed NP dispersion into droplets with spherical shape and higher sizes (smaller than 200 nm). The influence of ethanol concentration increase from 55% to 90% on NP features was evaluated at the fixed stirring speed of 5,000 rpm. DLS and SEM analyses were then performed, showing that higher ethanol concentrations led to lower NP diameters. This result was due to NP formation kinetics: higher initial ethanol concentration in the solvent was associated with a slower decrease of ethanol concentration in the dispersed droplets till the solubility limit could be reached. During this time, zein droplets could be sheared and broken-up to achieve smaller sizes before precipitation and solidification. Finally, the impact of zein concentration from 6.5 to 300 mg/mL was evaluated. DLS analysis showed that lower zein concentrations allowed the preparation of smaller NPs; on the other hand, at higher concentration than 100 mg/mL large agglomerates formed. This behavior depends on the fact that by decreasing zein concentration, zein solution viscosity also decreased and zein droplets could be more easily deformed, allowing the formation of smaller NPs.
Recently, innovative dialysis methods have been studied as alternatives to traditional approaches. Rodríguez-Félix et al. developed an antisolvent-dialysis method in order to form zein micro- and nanoparticles through an easy, one-step and reproducible process (Rodríguez-Félix et al., 2020). Despite being innovative, this method is also highly eco-friendly in terms of low energy consumption and waste production, due to the simultaneous combination of zein purification from hydrophobic xanthophylls, the yellow pigments of zein. This leads to the production of white zein particles without the need of a second processing step, thus decreasing the amount of solvent needed for both operations. This allows a reduction in the amount of solvents required for carrying out the operations separately. Figure 2 shows a schematic representation of the antisolvent-dialysis method. Basically, zein solution is put into a dialysis membrane inside a dialyzer, filled with distilled water at 1:10 ratio under magnetic stirring for 12 h at room temperature. This method induces zein self-assembly by the concentration gradient and polarity change of the solvents: the diffusion kinetics of the organic solvent through the dialysis membrane slow down gradually up to the nucleation of zein, with the formation of micro- and nanoparticles. This method allowed the production of a mixed population of microparticles (0.74 ± 0.2 μm) and NPs (243.2 ± 94.5 nm). The authors proposed to apply these particles as natural and edible dyes for coatings in substitution to inorganic dyes or as polymeric matrices protecting molecules of interest in food industry.
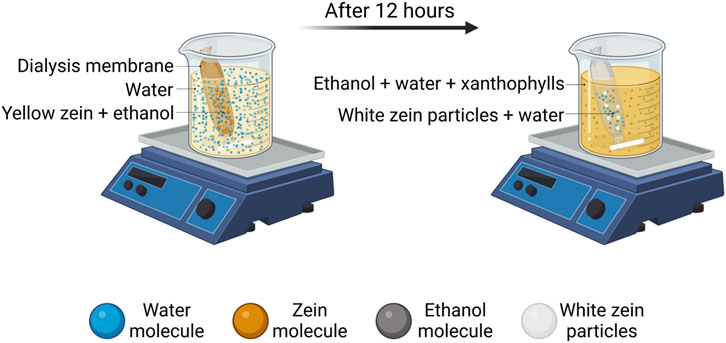
FIGURE 2. Antisolvent-dialysis method for the production of white zein micro- and nanoparticles (Rodríguez-Félix et al., 2020). Created with BioRender.com.
Chen et al. performed a multi-frequency ultrasound-assisted dialysis to produce curcumin-loaded zein-sodium caseinate NPs (Chen et al., 2022b). They dissolved 1% w/v zein and sodium caseinate in 1,2-propanediol aqueous solution at 80% v/v by stirring and centrifugation to remove larger particles. Then zein-sodium caseinate solution was put into a dialysis membrane inside a dialyzer filled with deionized water in a 1:2 ratio, and the entire system was placed into an ultrasonic bath reactor (20–28–40 Hz, 300 W, 25°C, 10 min). After this process, dialysis was completed at room temperature to obtain the effective formation of stable zein NPs. Results showed that the optimal frequency of 20/40 kHz allowed the production of uniform and regular spherical NPs with 225 ± 4 nm size, low polydispersity index and negative zeta potential. The sonication process together with the use of sodium caseinate improved storage and thermal stability of NPs, and allowed high encapsulation efficiency and antioxidant activity of curcumin. They finally demonstrated controlled curcumin release under simulated gastrointestinal digestion conditions, confirming the success of the multi-frequency ultrasound-assisted dialysis technique and the possibility to extend its application to other hydrophobic compounds.
Few studies focused on the production of zein NPs through unconventional scalable electrospraying and supercritical antisolvent precipitation techniques.
Electrospraying is a single-step method that is based on the use of an electric field to induce the separation of the zein solution (extruded through a metal nozzle) into charged droplets. The principle is the same as for the electrospinning technique, but instead of obtaining nanofibers, it is possible to produce monodispersed NPs with different characteristics by modulating the electric voltage, solution concentration and flow rate, and nozzle-collector distance. Gomez-Estaca et al. obtained curcumin-loaded zein NPs with sizes ranging from 175 to 900 nm increasing polymer concentration (Gomez-Estaca et al., 2012). They found unaltered morphology of NPs after curcumin encapsulation and the process also allowed high encapsulation efficiency (85%–90%). An implementation of this method is coaxial electrospraying, which allows to produce hybrid NPs (Kang et al., 2021). Kang et al. prepared drug loaded polymer-lipid hybrid NPs loaded with tamoxifen citrate (a hydrophobic anticancer drug) by electrospraying. The drug-zein solution in aqueous ethanol at 70% v/v was exploited as the core working fluid while a solution of lipid in pure ethanol was exploited as the shell fluid. Zein core–lipid shell nanostructures with a round shape were obtained. The major disadvantages of this technique are the high cost and energy consumption which limit its application in the pharmaceutical, biotechnology, and medical industries as competitive drug delivery systems.
Supercritical antisolvent precipitation techniques (SAS) consists of a feed, continuously sprayed into supercritical CO2 that acts as an anti-solvent for the majority of polymers (Franco and Marco, 2020). Rosa et al. studied the feasibility of SAS for the co-precipitation of a vitamin complex containing riboflavin, δ-tocopherol and ß-carotene in zein microcapsules (Rosa et al., 2020). They obtained spherical microcapsules with a size varying in the 8–18 μm range depending on which vitamin was encapsulated. Despite the scalability of the process, SAS is incompatible with several pharmaceuticals and requires a specific and expensive setting. Therefore phase separation conventional method remains the most applied for the preparation of zein NPs.
3.3 Zein nanoparticles for drug delivery
Protein-based NPs usually show biocompatibility, biodegradability and minimization of hypersensitivity reactions (Mishra et al., 2010). Furthermore, protein-based NPs are generally prepared avoiding the use of toxic chemicals or organic solvents (Lohcharoenkal et al., 2014). Zein protein NPs have been mostly exploited for the encapsulation of hydrophobic compounds (e.g., essential oils): their large amount of nonpolar amino acid residues favor zein interaction with hydrophobic drugs to achieve high drug loading efficiency. However, in a few cases, hydrophilic drugs, including oligonucleotides, have also been encapsulated into zein NPs. The majority of research studies prepared zein NPs using zein solutions in binary solvents containing water which is the greenest solvent.
The following paragraphs describe the preparation of zein NPs loaded with hydrophobic and hydrophilic drugs using zein solutions in different solvent mixtures. The dependence of NP size and physicochemical properties on used binary aqueous solvents and ratio between them is also highlighted. Table 3 collects main features of exemplary zein NPs discussed in this paper.
3.3.1 Hydrophobic drug delivery by zein nanoparticles
Over the years, zein has been mainly studied as a nanocarrier for the release of hydrophobic compounds and NPs have been generally prepared from solutions in ethanol-water solvents (Tran et al., 2019) (Table 3). One advantage of zein NPs over other carriers is their superior stability in the gastric and intestinal tract upon oral administration thanks to its isoelectric point that allows to maintain stability against aggregation of zein NPs at acidic pH. Furthermore, zein shows resistance to degradation at acidic pH and in the presence of proteolytic enzymes. These characteristics allow the use of zein NPs as carriers for drugs that need to be protected during oral delivery against the harsh gastrointestinal conditions (Lee et al., 2013; Cheng et al., 2019).
Therefore, zein NPs are often exploited for oral delivery of compounds to prevent their degradation in the harsh gastrointestinal tract (GIT) conditions because at acidic pH they remain stable against aggregation.
Cheng et al. prepared zein NPs encapsulating lutein, a carotenoid compound (a xanthophyll) naturally present in corn and also in leafy green vegetables, and known to have beneficial effects on reducing the risk of heart disease, stroke and cataract formation (Cheng et al., 2019). However, bio-accessibility of lutein naturally present in zein and other plant derivates is low (14%–55%) (Serrano et al., 2005; Reboul et al., 2006). Zein NPs represent valuable carriers for lutein delivery, protecting this compound from heat, oxygen and light. In this work, lutein-loaded zein NPs with an average size of 75 nm were prepared by anti-solvent precipitation, dissolving zein (25 mg/mL) in 80% v/v aqueous ethanol solution and lutein (0.5 mg/mL) in ethanol. NPs were formed by drop-wise dispersion in water.
Zou et al. produced zein NPs encapsulating cranberry procyanidins (CPs) through a liquid-liquid process (Zou et al., 2012). Procyanidins have antioxidant properties, thus reducing the risk of cardiovascular diseases and cancers. Among them, cranberry procyanidins show beneficial effects against urinary infections (Foo et al., 2000; Santos-Buelga and Scalbert, 2000; Prior and Gu, 2005). Procyanidins have a high binding affinity to proteins rich in prolines and they are usually employed to remove excessive tannins from wines. Since zein has a proline content of around 10%, innovative CPs-loaded zein NPs were prepared in order to improve the oral bioavailability of CPs. Different concentrations of CPs and zein (1 mg/mL) were dissolved in 80% v/v ethanol-water, then water was added, causing NP formation. By increasing CPs to zein weight ratio (from 1:8 to 1:2 wt/wt), the hydrodynamic diameter was found to increase from 392 nm to 447 nm, while zeta-potential decreased from 20.9 mV to 14.2 mV. The encapsulation efficiency increased by decreasing CPs to zein weight ratio, from 10% to 86%. CPs-loaded NPs showed superior in vitro biocompatibility compared to CPs alone, as assessed through in vitro cell tests with human promyelocytic leukemia HL-60 cells.
Zein NPs encapsulating 5-fluorouracil (5-FU) were also developed for intravenous delivery to target liver tissue (Lai and Guo, 2011). Zein was selected to protect 5-FU from enzymatic degradation and to shield its potential toxicity. NPs were prepared by the phase separation method. 5-FU and zein (12.5 mg/mL) were dissolved in 70% v/v ethanol-water, then added to distilled water. The hydrodynamic diameter was 114.9 ± 59.4 nm, zeta potential was −45 ± 0.3 mV and encapsulation efficiency was 60.7% ± 1.74%. Initial burst release was due to weakly bonded drug and attributed to zein NP core-shell structure. Indeed, during the synthesis process zein first forms a core, and then slowly adsorbs free zein to form a shell under a layer-by-layer assembly architecture. Therefore, burst release of drug may be probably due to the release of the drug portion present in the shell or at the core-shell interface. In vitro release was complete within 24 h.
Xu et al. prepared hollow zein NPs for various drug delivery applications (Figure 3A) (Xu et al., 2011). The anti-diabetic drug metformin was used as a drug model. Hollow NPs were produced by phase separation method dissolving zein (91 mg/mL) and sodium carbonate crystal (7 mg/mL) in 70% v/v ethanol-water. Then, this solution was precipitated into water using different volumes. Since sodium carbonate is highly soluble in water, the sacrificial cores immediately dissolved after zein precipitation on its surface, forming hollow NPs. After, an aqueous solution of metformin was added to the NP dispersion for 5 h at 50°C. Control solid zein NPs were prepared by precipitating zein solution in ethanol-water (12 mg/mL) into water. The size of both hollow and solid NPs presented a decreasing trend (from 102.3 nm to 62.9 nm for hollow NPs and from 160 nm to 135 nm for solid NPs) by increasing water to zein solution volume ratio. Hollow NPs showed lower sizes than solid NPs probably due to the presence of sodium carbonate nanocrystals acting as heterogeneous nucleation sites, and limiting the increase of diameters. Hollow NPs showed slower release kinetics and released a higher overall drug amount respect to solid NPs. In vitro biocompatibility and cell internalization was assessed using NIH 3T3 mouse fibroblast cells.
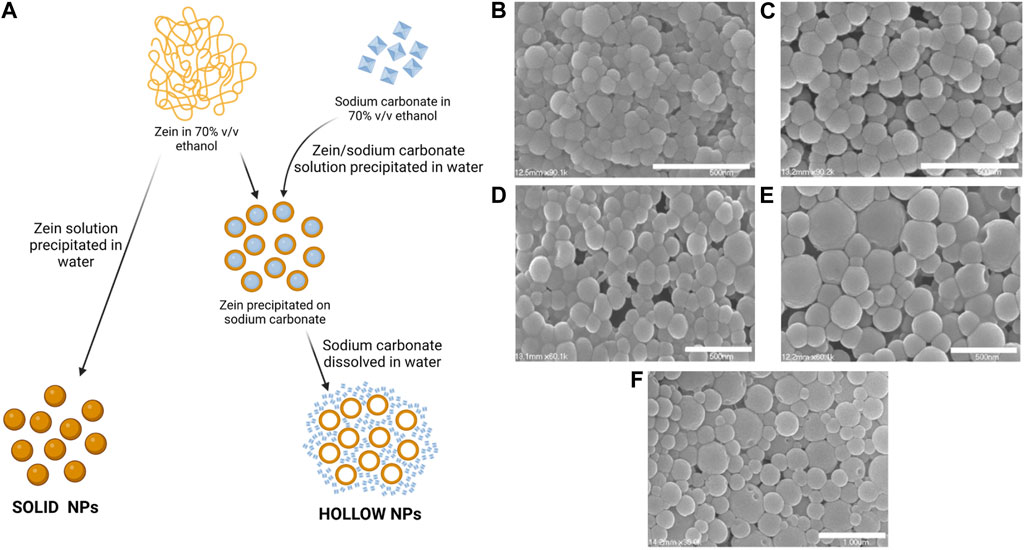
FIGURE 3. (A) Scheme of solid and hollow NP preparation (Xu et al., 2011). Created with BioRender.com. SEM of zein NPs loaded with pDNA formed at different zein to pDNA ratios ((B) 20:1 (C) 40:1 (D) 80:1 (E) 160:1 (F) 250:1). Adapted under Creative Commons CC BY license from (Regier et al., 2012).
Yu et al. described maytansine (DM1)-loaded zein NPs prepared by phase separation method using dimethyl sulfoxide (DMSO) solvent (Yu et al., 2020). DM1 has high and broad antitumor activity. DM1 and zein (60 mg/mL) were dissolved separately in a DMSO solution, then mixed together and dropped into distilled water while stirring. NPs showed a hydrodynamic diameter of 112 nm, a polydispersity index of 0.21, a zeta potential of 37 mV and high encapsulation efficiency (83%). In vitro release kinetics was characterized by a burst release within the first 8 h of about 20%, followed by a release of 40% after 24 h (Li et al., 2017; Sabra et al., 2018). DM1-loaded zein NPs were stable in Fetal Bovine Serum (FBS) in vitro. High cellular uptake of NPs was demonstrated with perinuclear disposition and strong inhibitory effect on A549 cells. Successful lung tumor treatment and nontoxic effects were also demonstrated by in vivo mouse tests.
3.3.2 Essential oils delivery
Zein NPs have been widely investigated for the loading and release of essential oils. Essential oils are hydrophobic and volatile compounds derived from plants, with a broad spectrum of biological activities, including antifungal, insecticidal, antimicrobial, and antioxidant properties. Examples include oregano, thyme, lavender and lemon oils.
To the best of our knowledge, the first work on essential oils encapsulation into zein NPs was published by Parris et al. (Parris et al., 2008). Three essential oils (oregano, red thyme, and cassia) were loaded into zein NPs by phase separation method. Zein (66.7 mg/mL) and essential oils were dissolved in an aqueous ethanol solution (85% v/v), then dispersed in water. Zein NPs encapsulated 20% oregano and thyme, and 13% cassia oil.
Later, another study focused on the encapsulation of two essential oils (thymol and carvacrol) into zein NPs, using liquid-liquid dispersion method (Wu et al., 2012). Essential oils and zein (50 mg/mL) were dissolved in 70% v/v aqueous ethanol solution and then subjected to shear stresses in water through homogenization. NPs showed an average hydrodynamic size below 330 nm and a polydispersity index below 0.3. Encapsulation efficiency was more than 60%, with higher thymol loading compared to carvacrol although with no significant differences. The authors hypothesized that encapsulation efficiency, measured after lyophilization, was slightly lower than the real value, due to the volatility of essential oils. Although carvacrol has a higher solubility in water, no difference in the release behavior of the two tested essential oils was detected.
In another study, zein NPs were found to encapsulate more efficiently non-volatile fish oil (above 90%) than other volatile essential oils (Zhong et al., 2009). Fish oil was loaded into zein NPs via liquid-liquid dispersion (Shaw et al., 2007). In this case, 90% v/v isopropanol was used to dissolve zein (40–67 mg/mL) and fish oil. Even if isopropanol is not a GRAS solvent, it was used because fish oil has a reduced solubility in ethanol solutions. The isopropanol percentage was selected taking into account zein insolubility at higher isopropanol concentrations. NP sizes ranged between 350 and 450 nm, and did not show a round morphology differently from zein NPs prepared in ethanol-water solutions. Lyophilized NPs were able to protect loaded fish oil from oxidation.
3.3.3 Hydrophilic drug delivery by zein micro- and nanoparticles
Zein NPs encapsulating water-soluble compounds have been less investigated for oral drug delivery. Lau et al. extended the use of a coacervation method, previously developed for the preparation of zein microparticles loaded with prednisolone, a hydrophobic anti-inflammatory drug, to the encapsulation of hydrophilic agents (Lau et al., 2012). By this method, they prepared zein microparticles loaded with two drugs for the treatment of inflammatory bowel disease: a hydrophobic drug with similar chemical structure to prednisolone, called hydrocortisone, and a structurally different water-soluble drug, called mesalazine (Lau et al., 2013). Zein microparticles were prepared by dissolving zein into 70% v/v aqueous ethanol solution. They also optimized the method for solution stirring, usually not well described in published protocols, with the aim to enhance microparticle reproducibility and drug encapsulation efficiency. In details, vortex mixer allowed higher drug encapsulation efficiency compared to magnetic stirring. Mesalazine-loaded zein microparticles showed higher loading efficiency but lower reproducibility compared to hydrocortisone-loaded zein microparticles.
Another study explored the encapsulation of two antioxidant proteins, catalase and superoxide dismutase (SOD), as scavengers for reactive oxygen species (ROS) generated by macrophages in rheumatoid arthritis (Lee et al., 2013). These two proteins were loaded into zein NPs for oral delivery to protect them from harsh environment in the gastrointestinal tract. Glisodin®, the first effective oral delivery system for SOD release is based on gliadin, a prolamin from wheat, which may induce Celiac disease. Therefore, authors prepared NPs based on an alternative prolamin, zein, for the delivery of the same proteins. NPs were prepared by phase separation method by dissolving zein (55 mg/mL) in 80% v/v ethanol-water, then adding the therapeutic proteins. NPs showed an average hydrodynamic diameter of 255 nm at pH 7.0 and the encapsulation efficiency was 31% for SOD and 45% for catalase. Zein conjugated with folic acid by an amide linkage to lysine residues allowed targeted drug release to activated macrophages, demonstrated by in vitro cell tests.
Dong and coworkers prepared zein NPs loaded with doxorubicin (DOX), a water-soluble anti-cancer drug, in order to provide effective drug dose with no cytotoxic effects (Dong et al., 2016). Zein (10 mg/mL) and DOX were dissolved in 80% v/v ethanol-water and NPs were produced by phase separation method. The average hydrodynamic diameter of DOX-loaded zein NPs ranged from 200 to 240 nm, the polydispersity index was around 0.15–0.20, zeta potential was −50 mV and the encapsulation efficiency was about 90%. In vitro release tests displayed a pH-responsive trend. Three pH conditions were tested (5.0, 6.5 and 7.4) and the cumulative release of zein NPs reached 69%, 83% and 60% after 100 h, respectively, thus achieving higher values at more acidic pH. The anti-proliferative effect of DOX-loaded NPs on HeLa cells was studied. NPs entered the cytoplasm of HeLa cells via micropinocytosis and DOX was released in a controlled and sustained manner from zein NPs.
More recently, zein NPs were selected for chloroquine loading, an anti-malarian drug employed to decrease inflammation after spinal cord injury (Chen X. et al., 2022). Zein (6.5 mg/mL) was dissolved in 80% v/v ethanol-water, then water and chloroquine were added separately. Chloroquine-loaded zein NPs showed a hydrodynamic diameter ranging from 172 to 186 nm, a low polydispersity index, negative zeta potentials between −30 and −40 mV and a high encapsulation efficiency (61%–77%). An in vitro controlled delivery of 4 days was monitored. In vitro tests using microglial BV2 cells showed a reduction of pro-inflammatory factors after zein NP treatment. Microglia polarization towards the M2 phenotype was enhanced by zein NPs. Indeed, zein contributed to the attenuation of inflammation thanks to the beneficial effects of its degradation products. In vivo local administration on rats caused improved functional recovery, inhibited neuronal apoptosis and suppressed neuroinflammation.
3.3.4 Delivery of oligonucleotides by zein nanoparticles
Non-viral gene therapy has received widespread attention for its ability to introduce foreign exogenous DNAs or RNAs into cells affecting cell behavior (e.g., replacing genes or regulating their expression) (Shim et al., 2018). Zein NPs protect oligonucleotide cargo from degradation and allow their controlled and sustained release for efficient transfection. However, the use of zein NPs for oligonucleotide encapsulation has been poorly investigated to date.
To the best of our knowledge, Regier et al. were the first ones to develop zein NPs encapsulating plasmid DNA (pDNA) by the coacervation technique for kidney targeting (Regier et al., 2012). Zein is an amphiphilic protein with a high content of polar and protonable glutamine residues and an isoelectric point of 6.2–6.8, allowing the encapsulation of negatively charged pDNA via electrostatic interaction with positively charged zein chains, upon pH regulation. Zein (10 mg/mL) was dissolved in 70% v/v ethanol-water at pH 3, then pure ethanol at pH 3 was added, followed by addition of pDNA aqueous solution. Depending on zein to pDNA ratio, prepared NPs showed sizes ranging from 158 to 397 nm (Figures 3B–F) and zeta potential values ranging from −20 mV to −50 mV. Degradation tests in the presence of DNase I confirmed integrity of loaded pDNA. Zein NPs showed sustained but incomplete in vitro pDNA release in PBS within 7 days, with only 18% of loaded pDNA released, after an initial burst release within the first 12 h. In vitro cell tests showed biocompatibility, and efficient NP internalization by Caco-2 (Human colon carcinoma cells) and HEK 293 T (Human embryonic epithelial kidney cells) cells. Indeed, the N-terminal region of γ-zein, rich in proline, probably served as a peptide carrier interacting with the phosphate diester anionic polar heads of the cell membrane, favoring the endocytosis of zein NPs, with pDNA transfer into the cell cytoplasm (Fernández-Carneado et al., 2004). Interestingly, cell internalization could be analyzed by confocal microscopy exploiting zein autofluorescence (with excitation wavelengths at 365–395 nm and 465–485 nm).
A few years later, zein NPs were employed again in gene therapy, for the encapsulation of pDNAs encoding Phosphatase and Tensin homolog (PTEN) and Tumor Necrosis Factor - Related Apoptosis-Inducing Ligand (TRAIL) for the treatment of hepatocellular carcinoma (El Sharkawi et al., 2017). Zein NPs were prepared by the coacervation method with slight modifications compared to the previous protocol. Zein (20 mg/mL) was dissolved in highly concentrated ethanol solution (90% v/v) at pH 3 and then pDNA solution at pH 4 was added. The average NP hydrodynamic diameter and zeta potential ranged from 132 to 238 nm and from −37 to −24 mV, respectively. Encapsulation efficiency varied from 12% to 42%. In vitro tests showed decreased cell viability and proliferation rate of liver tumor cells (HepG2) treated with NPs. In vivo experiments in rat tumor model confirmed a significant decrease of liver metastasis by increased mRNA expression of p53 (a marker of apoptosis) and decreased mRNA levels of VEGF and MMP-2 (markers for angiogenesis and metastasis, respectively), measured from liver homogenates.
Overall, only a few studies have been published on the use of zein NPs delivering hydrophilic therapeutic compounds. Hence, further research is needed to optimize the preparation of such NPs with the aim to obtain higher encapsulation efficiency.
3.3.5 Greener preparation methods for zein nanoparticles
The herein performed literature survey on zein NPs has shown that phase separation method using ethanol-water (Table 3) is the most frequently used approach. As underlined at Par. 3.2, this is due to the simple experimental settings and low-cost of the technique. However, ethanol-water binary solvents are suboptimal considering their environmental impact.
Greener phase preparation methods should be developed, for example, by exploiting acetone-water solvents. Acetone has been defined as the least toxic industrial solvent (Joshi and Adhikari, 2019). However, only a few examples of zein nanocarriers prepared from acetone-water solutions have been published for applications in the botany field (Table 3) with the aim to address the challenge of controlled delivery of pesticides and fertilizers to plants. Indeed, in the field more than 90% of conventional agrochemicals are unable to reach the desired target using traditional administration methods (Pérez-Guzmán and Castro-Muñoz, 2020). In botany, zein NPs delivering pesticides and fertilizers have been proposed by exploiting their adhesion to the root system or the foliage (Prasad et al., 2017). Prasad et al. prepared positively charged zein NPs for pesticide delivery and evaluated their uptake and translocation to sugar cane plants through plant roots (Prasad et al., 2017). Zein NPs were prepared by dissolving zein (40 mg/mL) in 80% v/v acetone-water solution, followed by its dropping into an aqueous phase (containing a positively charged stabilizing surfactant for the specific purpose of this work). Spherical NPs with a hydrodynamic size of 135 nm and a polydispersity index of 0.20 were obtained (Table 3). Later, the same research group studied the biodistribution of zein NPs, from the root to the leaves of soybean plants, with the aim to widen the application of zein NPs for pesticide delivery to different crops (Ristroph et al., 2017). Zein NPs were prepared with slight variations respect to the previously developed protocol (32 mg/mL of zein dissolved in 80% v/v acetone-water) (Table 3). Further research by another group investigated the effects of positively-charged zein NPs, prepared by the same method described above, on three models of larval noctuid pests, as a potential alternative strategy for pest control (Bonser et al., 2020) (Table 3). More recently, zein NPs were prepared by the same protocol and then surface modified with a negatively charged coating for the encapsulation and controlled delivery of a pesticide (Hanna et al., 2022) (Table 3).
Despite zein NPs prepared through greener conditions have been applied in botany only, their use could be extended to other therapeutic applications in regenerative medicine.
3.3.6 Zein-based core-shell nanoparticles
Although zein has been frequently used alone for the preparation of drug-loaded micro- and nanoparticles, a great number of studies have also focused on the preparation of zein-based core-shell NPs in combination with another biomaterial (Demir et al., 2017). Two applications have been investigated: 1) the use of zein as coating on NPs based on another material, exploiting zein favorable stability at acid pH and ability for sustained release; 2) the use of zein as core for NPs coated with a different material, in order to overcome colloidal instability of zein NPs (Yuan et al., 2022b).
As a relevant example of zein-coated NPs, Luo et al. prepared chitosan/tripolyphosphate NPs loaded with selenite and coated with zein (Luo et al., 2010). Zein coated NPs maintained nanoscale dimensions (200–400 nm), showed improved encapsulation efficiency (with an increase from 60% to 95% after zein coating) and release profile (with reduced burst release within the first 4 h in PBS). A few years later, chitosan/tripolyphosphate NPs were employed again for oral gene delivery and coated with zein (Farris et al., 2017). As shown in Figure 4A, DNA-loaded chitosan/tripolyphosphate NPs were encapsulated in zein microparticles. Zein coating caused an increase in DNA encapsulation efficiency and a slower in vitro release kinetics in simulated gastric fluid compared to DNA-loaded chitosan/tripolyphosphate NPs. In vivo tests in mouse model confirmed successful cell transfection. More recently, the introduction of synthetic PLGA NPs coated with zein was proposed for the release of docosahexaenoic acid (Liu et al., 2021).
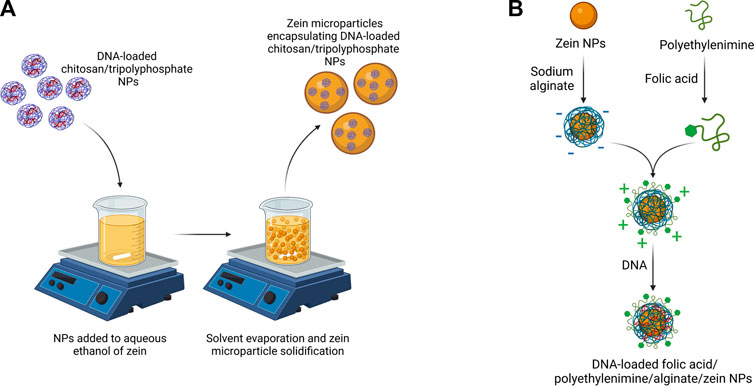
FIGURE 4. (A) Scheme of DNA-loaded chitosan/tripolyphosphate NPs coated with zein (Farris et al., 2017) (B) Scheme of the preparation of DNA-loaded zein NPs coated with alginate, poly (ethylenimine) and folic acid (Chen Y. et al., 2022). Created with BioRender.com.
A recent review paper has discussed the effect of composition, preparation and storage methods of zein-based NPs with zein core on their stability (Yuan et al., 2022a). Different materials have been proposed as coatings for zein NPs, such as polysaccharides, proteins and surfactants (Li and Yu, 2020). Such NPs have been prepared by simple methods, such as anti-solvent precipitation, layer-by-layer coating or by exploiting zein properties, such as surface charge tunability with pH. In some cases, polysaccharide-coated zein NPs were not stable, due to steric hindrance between polysaccharide coatings and functional moieties on zein NPs. This issue was not detected with alginate coating which has been deeply investigated in literature as stabilizer for NPs. For example, alginate has been used both alone for doxorubicin delivery (Ye et al., 2022a), or in combination with other charged polymers to generate stable complexes for DNA and folic acid (Figure 4B) (Chen Y. et al., 2022) or curcumin delivery (Yao et al., 2018; Liu et al., 2019). Propylene glycol alginate, an alginate derivative, was combined with zein to form a noncovalent binary complex for the release of quercetagetin (Sun et al., 2016), ß-carotene (Wei et al., 2018) and resveratrol (Wei et al., 2019). Other examples include soybean shell/zein NPs for quercetin release, exploiting soybean coating stability to pH (in the two to eight range), ionic strength and temperature (Li H. et al., 2019). Same NPs were applied for lutein release (Li et al., 2020). Zein-carrageenan core-shell NPs were successfully loaded with curcumin and piperine through a combination of electrostatic attraction, hydrogen bonding, and hydrophobic interactions (Chen et al., 2020). Pectin coating was also exploited to avoid agglomeration of zein NPs, loaded with tannic acid (Liang et al., 2021). In another application, ethanol-soluble pectin from sugar beet was exploited as a coating stabilizing zein NPs and favoring the delivery of curcumin (Zhang et al., 2022). Literature also reports coatings with pectin together with sodium caseinate (Chang et al., 2017a; Chang et al., 2017b). Hyaluronic acid was also applied as coating of zein NPs for quercetagetin (Chen et al., 2019b) and tetrahydrocurcumin (Zhu et al., 2022) delivery. Chitosan coating was applied on zein based NPs for the release of a-tocopherol (Luo et al., 2011), retinol (Park et al., 2015), quercetin (Ma et al., 2018), curcumin (Baspinar et al., 2018; Li et al., 2018) and folic and caffeic acid (Wusigale et al., 2021). Alternatively, carboxymethyl chitosan coating was used for zein NPs encapsulating vitamin D3 (Luo et al., 2012) and natamycin (Lin et al., 2020). Blend coatings applied on zein NPs include: i) chitosan/alginate for resveratrol delivery (Khan et al., 2019); ii) chitosan/dextran sulfate for the release of crocin carotenoid for Alzheimer’s disease treatment (Song et al., 2022); iii) chitosan/hyaluronic acid for the co-delivery of curcumin and piperine (Chen et al., 2019a); iv) chitosan hydrochloride/sodium caseinate for thymol delivery (Zhang et al., 2014). Indeed, sodium caseinate is the mostly studied zein electrosteric stabilizer: it is an amphiphilic stabilizer which is adsorbed on the surface of zein NPs to provide an electrostatic and steric repulsion against aggregation (Patel et al., 2010; Luo et al., 2013; Ahmed et al., 2015; Pan and Zhong, 2016; Li et al., 2017; Zhang and Han, 2018; Chen et al., 2022a).
Concerning the use of surfactants as coatings for zein NPs, an interesting study compared zein based NPs and PLGA NPs for their ability to release rutin, with sodium deoxycholate monohydrate or poloxamer 188 as stabilizers (Gagliardi et al., 2021). The aim of the investigation was to compare physico-chemical characteristics of NPs prepared with the synthetic (PLGA) and the natural (zein) polymers, the capacity of PLGA or zein NPs to encapsulate and release rutin and NP antioxidant effect. Zein NPs were prepared by dissolving zein in 65% ethanol-water and adding sodium deoxycholate monohydrate or poloxamer 188. Results showed the enhanced ability of zein NPs to encapsulate rutin and release it in a controlled and sustained way, also thanks to the surfactant-based coating. Moreover, zein-based NPs showed increased in vitro antioxidant activity thanks to the synergistic effect of rutin and zein degradation products, compared to PLGA NPs. Other studies made use of other surfactants, such as lecithin (Dai et al., 2016) and poloxamer 407 (Zhang et al., 2021) for zein NP coating, while Lucio et al. prepared zein NPs in the presence of lysine as stabilizer (Lucio et al., 2017).
Table 4 collects the main hybrid core-shell zein NPs discussed in this paragraph.
3.3.7 In vivo immune response of zein nanoparticles
Beside safety, in vivo immunogenicity is a key parameter for in vivo application of particles, and it depends on their physicochemical properties and administration route. Zein is biocompatible and biodegradable. However, being a plant-derived protein, potential immune response in vivo may represent an issue. To date, studies on in vivo immune response induced by zein are still limited and have shown controversial results (Zhang et al., 2016). For example, zein microspheres were found to be immunogenic when administered intramuscularly and orally. Intramuscular administration of blank zein microspheres enhanced antibody reaction with immunoglobulin G (IgG), while oral administration increased immunoglobulin A (IgA) expression in the intestine area, keeping low IgG levels (Hurtado-López and Murdan, 2010). Some years later, Li and coauthors confirmed that zein NPs administered via intramuscular, subcutaneous and interpleural routes provoked enhancement of IgG antibodies in mice (Li F. et al., 2019). On the other side, non-immunogenic zein NPs (with hydrodynamic diameters ranging from 100 to 400 nm) were also patented (Perumal et al., 2009). The same authors showed that zein NPs with sizes above 400 nm, administrated via subcutaneous injection, caused an in vivo immune response in mice.
Very recently, another study for the repair of sciatic nerve defects in rat model made use of zein microparticles confirming that size is a discriminating parameter for immunogenicity. Authors of this study highlighted other influencing factors on immunogenicity, such as the type of encapsulated drug (in their case, dexamethasone, an anti-inflammatory drug with immunosuppressive effects) and the porosity of the implanted structure, as it may inhibit neutrophil recruitment and enhance macrophage polarization towards the M2 phenotype. They studied the immunogenicity of zein compared to other materials, such as alginate, poly (lactic-co-glycolic acid) (PLGA) and polystyrene in rat model. They found out that zein first triggered an inflammatory reaction, similarly to non-degradable polystyrene; then, zein degradation occurred and the level of antigenicity was similar to that induced by alginate and PLGA, demonstrating zein safety (Yu et al., 2022).
Zein may be combined with polyethylene glycol (PEG) to inhibit immunogenicity. PEG is hydrophilic, highly water-soluble, anti-fouling and non-toxic. Moreover, it may improve zein colloidal stability in physiological environment. Two different methods have been investigated to produce zein-PEG NPs: PEG coating after NP formation and PEG chemical conjugation to zein protein before NP formation. PEG coatings can be easily obtained on zein NPs leading to improved serum stability, hemocompatibility, and good physical stability (El-Lakany et al., 2018; Reboredo et al., 2021; Ye et al., 2022b; Reboredo et al., 2022; Wiggers et al., 2022). However, PEG surface content is generally low and not homogeneous, hence it does not completely solve the issue of zein NP aggregation. On the other hand, zein chemical modification with PEG through carbodiimide reaction is laborious and requires longer time (zein functionalization needs days for reaction and dialysis). However, obtained NPs are advantageous for their low immunogenicity and improved serum stability (Podaralla et al., 2012; Soe et al., 2019; Meewan et al., 2022). A recent investigation has proposed the preparation of zein NPs by mixing PEG-modified zein (at least 20%) with unmodified zein through a microfluidics device, thus obtaining stable NPs in physiological medium (van Ballegooie et al., 2022).
According to previous reports, a definite conclusion on zein in vivo immunogenicity cannot be driven. More investigations are needed to understand and classify the influence of various factors, such as NP shape, size, surface properties and concentration, drug type and concentration, and administration routes on zein antigenicity.
4 Critical discussion
This review work was focused on the analysis of the physicochemical properties of zein, as a green material for use in nanomedicine. Due to the continuous growth of the world population, the demand for natural resources and the amount of produced waste materials will increase (Tamasiga et al., 2022). Hence, the implementation of circular economy strategies has become an urgent need. According to the circular economy model, corn waste may be reused as a low-cost raw material for zein extraction, a plant-based protein with a wide range of potential applications in packaging, botany, pharmaceutical and biomedical fields. Zein is low-cost, biocompatible, biodegradable and its amphiphilicity ensures interactions with materials or drugs with different wettability behavior. One interesting zein application explored in this review is its use for the formulation of efficient drug-loaded NPs able to protect therapeutics from early degradation and to convey them to target cells.
To ensure full sustainability, methods for zein NP preparation should also be environmentally friendly. Zein NPs have been mainly produced through phase separation methods, especially using ethanol-water solutions, showing different sizes and drug encapsulation efficiencies depending on solvent types and ratio, zein concentration, zein to drug ratio, mixing type/speed and pH conditions (Figure 3B; Table 3). Typically, the average hydrodynamic sizes of zein NPs were in the 100–400 nm range and drug encapsulation efficiency ranged from about 10% to 85% for both hydrophobic and hydrophilic compounds (Table 3). Despite its acceptable greenness level (Table 2), ethanol replacement with acetone, one of the safest industrial solvents (Joshi and Adhikari, 2019) would enhance sustainability of NP preparation methods. However, to date zein NPs prepared by phase separation using zein solutions in acetone-water have been used for botany applications only (Table 3). The adoption of such methods to the preparation of zein NPs for other applications is thus encouraged.
Sustainable preparation methods for zein NPs would also minimize energy consumption and waste generation (in terms of residual solvents and material). Phase separation methods are advantageous compared to other approaches with high cost and energy consumption, such as electrospraying and supercritical antisolvent precipitation. NP preparation by phase separation, avoiding a subsequent centrifugation step, also minimizes the production of waste material. Furthermore, antisolvent-dialysis combines in one process zein purification from hydrophobic xanthophylls and NP production, decreasing the amount of waste solvent needed for the two consecutive steps.
Although different methods and process conditions have been explored for zein NP preparation (Table 3), one key issue is represented by scale-up production. To this aim, the best zein NP preparation methods are electrospraying, supercritical antisolvent precipitation and microfluidic-based approaches (van Ballegooie et al., 2022). However, among them, only microfluidic methods are environmentally sustainable and low-cost.
Overall, zein NPs are advantageous because they may derive from agricultural waste, as added-value products with efficient drug loading, reproducibility and stability under optimized preparation conditions. Most zein NPs have been applied for the release of lipophilic compounds, such as curcumin, 5-fluorouracil, essential oils, with only a few examples for the release of hydrophilic drugs, such as doxorubicin and chloroquine (Table 3; Table 4). Methods for efficient encapsulation of both hydrophobic and hydrophilic drugs might increase the interest for zein use in nanomedicine. Particularly, gene therapies have recently attracted interest by the scientific community and could represent a promising application for zein NPs. Indeed, to date only a few studies have reported on zein NPs loaded with plasmid DNAs for gene therapy (Table 3), prepared by pH-triggered complexation. Additionally, such NPs have shown a moderate encapsulation efficiency of 12%–65% (Table 3), deserving improvement. Furthermore, by optimizing preparation methods, zein NPs should be proposed for the encapsulation and release of other oligonucleotide molecules, such as messenger RNAs (mRNAs), microRNAs (miRNAs) and small interference RNAs (siRNAs).
One main application of zein NPs is in oral drug delivery thanks to their resistance to acidic pH, protecting drugs from early degradation. Interestingly, degradation products of zein NPs may also exert beneficial anti-inflammatory effects for their high content of xanthophyll pigments (8%–9%) in yellow zein, such as lutein, zeaxanthin and ß-cryptoxanthin. Synergy between zein intrinsic anti-inflammatory effect together with the therapeutic action of encapsulated drugs represents an added-value property.
One of the main reported drawbacks of zein NPs is their poor colloidal stability at physiological conditions. Hybrid core-shell zein NPs have been proposed to address the issue, by the employment polysaccharides, proteins or surfactants as coatings for zein NPs (Table 4). Among them, polysaccharides have been the mostly studied polymers as zein NP coatings, due to their biocompatibility, wide availability and superior stability. Further advantages of core-shell zein-based NPs include higher encapsulation ability and more controlled release kinetics respect to zein NPs. However, in an attempt to propose green zein NP preparation, the addition of other components should not compromise product sustainability. In this regard, zein NPs coated with pectin derived from agricultural waste represent a sustainable solution.
Another key issue of zein NPs is represented by their potential in vivo immunogenicity which still remains controversial and would need additional in vivo safety studies.
Currently, zein is used as an excipient in food, pharmaceutical industries and vaccine delivery (Tortorella et al., 2021), while drug-loaded zein NPs are still under development and have not reached the approval for clinical use, yet. However, an injectable zein solution, called Ethibloc®, has been clinically approved in Canada. Ethibloc® is percutaneously injected for the treatment of epistaxis, aneurysmal bone cysts, and lymphangiomas (van Ballegooie et al., 2022). Only minor short-term complications related to fever symptoms have been observed, with no long-term side effects. Although Ethibloc® is not a nanomedicine product, its approval has demonstrated zein safety for use in humans. Furthermore, one clinical trial is in progress (GLUCOCAPS, Clinical Trial NCT05560412) on the use of zein NPs for glycemic control (Clinica Universidad de Navarra, 2022). According to this trial, patients with prediabetes will consume daily three capsules with nano-encapsulated zein (1 g of zein nano-encapsulated per day), compared to non-encapsulated zein. This study is expected to end in September 2023. Hence, in addition to its higher biocompatibility than synthetic polymers and lipids, and higher safety than animal-derived proteins, zein has an intrinsic therapeutic effect, which might be exploited in synergy with encapsulated drugs. In this regard, more studies are encouraged, particularly on zein use as carrier for oligonucleotide release for the development of new advanced therapies.
Zein NPs have also been investigated for uses in botany for the delivery of pesticides to plants, as an environmental friendly and safer alternative to NPs based on inorganic materials, such as commonly used gold, silver, copper, and iron oxide, which are affected by overproduction of reactive oxygen species and the release of potentially toxic ions. Finally, based on its biocompatibility and low-cost, the use of zein NPs in veterinary medicine might represent an interesting and not yet explored application field.
5 Conclusion
Zein has been proposed as a biocompatible and biodegradable plant-based protein for drug delivery applications. Extensive literature on the topic showed that zein NPs are advantageous because of the availability and low cost of the raw material. Physicochemical properties of zein such as aminoacidic composition and solvent miscibility have been demonstrated to be critical parameters for the preparation of green zein NPs. Among the available production methods, we underlined superiority of phase separation methods using acetone-water solutions for a sustainable preparation of drug-loaded zein NPs. Antisolvent-dialysis method was also found to be an interesting option for zein NP preparation, as able to combine zein purification and NP production in one step, thus decreasing the volumes of used solvent. On the other hand, zein NP production scalability is an important issue for lab to market translation. In this regards, novel microfluidic based approaches, based on phase separation methods, represent an optimal and sustainable choice.
Zein NPs have been mainly employed to deliver lipophilic drugs, such as curcumin and essential oils: such drugs are efficiently encapsulated by zein NPs because they interact with the hydrophobic aminoacidic residues of zein. However, a few recent investigations have also highlighted the possibility to release hydrophilic compounds from zein NPs, among which oligonucleotides. In this regard, examples reported so far have only focused on the encapsulation of plasmid DNAs into zein NPs, while the loading of other oligonucleotides such as mRNAs, siRNAs and miRNAs has yet to be investigated and could pave the way to the application of zein NPs for advanced RNA-based therapies.
To conclude, this review provides an innovative understanding of zein NP state of the art and provides useful information for the development of a new generation of sustainable, biodegradable, safe and innovative drug delivery vectors based on zein.
Author contributions
FT: conceptualization, formal analysis, writing—original draft; VC: supervision (project supervision); conceptualization, writing—review and editing, project administration, resources, funding acquisition. All authors listed have made a substantial, direct, and intellectual contribution to the work and approved it for publication. All authors contributed to the article and approved the submitted version.
Funding
The work was supported by Research and Innovation NOP 2014-2020 for Doctoral Research programmes with specific reference to Action IV.5 “PhD programmes on sustainability-based topics”. Support from the European Research Council (ERC) under European Union’s Horizon 2020 research and innovation (BIORECAR; grant no: 772168) is also acknowledged. FT acknowledges support from the joint “Doctorate of Bioengineering and Medical-Surgical Sciences” of University of Turin and Politecnico di Torino.
Conflict of interest
The authors declare that the research was conducted in the absence of any commercial or financial relationships that could be construed as a potential conflict of interest.
The author VC declared that they were an editorial board member of Frontiers, at the time of submission. This had no impact on the peer review process and the final decision.
Publisher’s note
All claims expressed in this article are solely those of the authors and do not necessarily represent those of their affiliated organizations, or those of the publisher, the editors and the reviewers. Any product that may be evaluated in this article, or claim that may be made by its manufacturer, is not guaranteed or endorsed by the publisher.
References
Abdelsalam, A. M., Somaida, A., Ayoub, A. M., Alsharif, F. M., Preis, E., Wojcik, M., et al. (2021). Surface-tailored zein nanoparticles: Strategies and applications. Pharmaceutics 13 (9), 1354. doi:10.3390/pharmaceutics13091354
ACS-GCI (2011). The American chemical society green chemistry Institute pharmaceutical roundtable. Solvent selection guide: Version 2.0.
Ahmed, O. A. A., Hosny, K. M., Al-Sawahli, M. M., and Fahmy, U. (2015). Optimization of caseinate-coated simvastatin-zein nanoparticles: Improved bioavailability and modified release characteristics. Drug Des. Dev. Ther. 9, 655–662. doi:10.2147/DDDT.S76194
Akharume, F. U., Aluko, R. E., and Adedeji, A. A. (2021). Modification of plant proteins for improved functionality: A review. Compr. Rev. Food Sci. Food Saf. 20 (1), 198–224. doi:10.1111/1541-4337.12688
Alargova, R. G., Bhatt, K. H., Paunov, V. N., and Velev, O. D. (2004). Scalable synthesis of a new class of polymer microrods by a liquid-liquid dispersion technique. Adv. Mater. 16 (18), 1653–1657. doi:10.1002/adma.200400112
Alargova, R. G., Paunov, V. N., and Velev, O. D. (2006). Formation of polymer microrods in shear flow by emulsification - solvent attrition mechanism. Langmuir 22 (2), 765–774. doi:10.1021/la051825v
Alfonsi, K., Colberg, J., Dunn, P. J., Fevig, T., Jennings, S., Johnson, T. A., et al. (2008). Green chemistry tools to influence a medicinal chemistry and research chemistry based organisation. Green Chem. 10 (1), 31–36. doi:10.1039/b711717e
Ali, S., Khatri, Z., Oh, K. W., Kim, I. S., and Kim, S. H. (2014). Zein/cellulose acetate hybrid nanofibers: Electrospinning and characterization. Macromol. Res. 22 (9), 971–977. doi:10.1007/s13233-014-2136-4
Anastas, P., and Eghbali, N. (2010). Green chemistry: Principles and practice. Chem. Soc. Rev. 39 (1), 301–312. doi:10.1039/b918763b
Anderson, T. J., and Lamsal, B. P. (2011). Review: Zein extraction from corn, corn products, and coproducts and modifications for various applications: A review. Cereal Chem. J. 88 (2), 159–173. doi:10.1094/CCHEM-06-10-0091
Argos, P., Pedersen, K., Marks, M. D., and Larkins, B. A. (1982). A structural model for maize zein proteins. J. Biol. Chem. 257 (17), 9984–9990. doi:10.1016/s0021-9258(18)33974-7
Arshady, R. (1990). Microspheres and microcapsules, a survey of manufacturing techniques Part II: Coacervation. Polym. Eng. Sci. 30 (75), 905–914. doi:10.1002/pen.760301505
Baspinar, Y., Üstündas, M., Bayraktar, O., and Sezgin, C. (2018). Curcumin and piperine loaded zein-chitosan nanoparticles: Development and in-vitro characterisation. Saudi Pharm. J. 26 (3), 323–334. doi:10.1016/j.jsps.2018.01.010
Bonser, C. A. R., Chen, X., Astete, C. E., Sabliov, C. M., and Davis, J. A. (2020). Elucidating efficacy of ingested positively charged zein nanoparticles against noctuidae. J. Econ. Entomology 113 (6), 2739–2744. doi:10.1093/jee/toaa199
Bouman, J., Belton, P., Venema, P., Van Der Linden, E., De Vries, R., and Qi, S. (2016). Controlled release from zein matrices: Interplay of drug hydrophobicity and pH. Pharm. Res. 33 (3), 673–685. doi:10.1007/s11095-015-1818-8
Byrne, F. P., Jin, S., Paggiola, G., Petchey, T. H. M., Clark, J. H., Farmer, T. J., et al. (2016). Tools and techniques for solvent selection: Green solvent selection guides. Sustain. Chem. Process. 4 (1), 7–24. doi:10.1186/s40508-016-0051-z
Cabra, V., Arreguin, R., Vazquez-Duhalt, R., and Farres, A. (2007). Effect of alkaline deamidation on the structure, surface hydrophobicity, and emulsifying properties of the Z19 α-zein. J. Agric. Food Chem. 55 (2), 439–445. doi:10.1021/jf061002r
Cabra, V., Arreguin, R., Vazquez-Duhalt, R., and Farres, A. (2006). Effect of temperature and pH on the secondary structure and processes of oligomerization of 19 kDa alpha-zein. Biochimica Biophysica Acta - Proteins Proteomics 1764 (6), 1110–1118. doi:10.1016/j.bbapap.2006.04.002
Chakrapani, G., Zare, M., and Ramakrishna, S. (2022). Biomaterials from the value-added food wastes. Bioresour. Technol. Rep. 19, 101181. doi:10.1016/j.biteb.2022.101181
Chang, C., Wang, T., Hu, Q., and Luo, Y. (2017b). Zein/caseinate/pectin complex nanoparticles: Formation and characterization. Int. J. Biol. Macromol. 104, 117–124. doi:10.1016/j.ijbiomac.2017.05.178
Chang, C., Wang, T., Hu, Q., Zhou, M., Xue, J., and Luo, Y. (2017a). Pectin coating improves physicochemical properties of caseinate/zein nanoparticles as oral delivery vehicles for curcumin. Food Hydrocoll. 70, 143–151. doi:10.1016/j.foodhyd.2017.03.033
Chen, H., Li, Y., Liu, A., Wu, L., Yan, W., Tong, Y., et al. (2022a). Circular extraction: Innovative use of a switchable composite extractant for prolamin extraction from grain byproducts. ACS Food Sci. Technol. 2, 630–637. doi:10.1021/acsfoodscitech.1c00413
Chen, H., Xu, B., Zhou, C., Yagoub, A. E. G. A., Cai, Z., and Yu, X. (2022b). Multi-frequency ultrasound-assisted dialysis modulates the self-assembly of alcohol-free zein-sodium caseinate to encapsulate curcumin and fabricate composite nanoparticles. Food Hydrocoll. 122, 107110. doi:10.1016/j.foodhyd.2021.107110
Chen, S., Han, Y., Wang, Y., Yang, X., Sun, C., Mao, L., et al. (2019b). Zein-hyaluronic acid binary complex as a delivery vehicle of quercetagetin: Fabrication, structural characterization, physicochemical stability and in vitro release property. Food Chem. 276 (17), 322–332. doi:10.1016/j.foodchem.2018.10.034
Chen, S., Li, Q., McClements, D. J., Han, Y., Dai, L., Mao, L., et al. (2020). Co-delivery of curcumin and piperine in zein-carrageenan core-shell nanoparticles: Formation, structure, stability and in vitro gastrointestinal digestion. Food Hydrocoll. 99, 105334. doi:10.1016/j.foodhyd.2019.105334
Chen, S., McClements, D. J., Jian, L., Han, Y., Dai, L., Mao, L., et al. (2019a). Core-shell biopolymer nanoparticles for Co-delivery of curcumin and piperine: Sequential electrostatic deposition of hyaluronic acid and chitosan shells on the zein core. ACS Appl. Mater. Interfaces 11 (41), 38103–38115. doi:10.1021/acsami.9b11782
Chen, X., Wang, B., Mao, Y., Al Mamun, A., Wu, M., Qu, S., et al. (2022c). Zein nanoparticles loaded with chloroquine improve functional recovery and attenuate neuroinflammation after spinal cord injury. Chem. Eng. J. 450 (1), 137882. doi:10.1016/j.cej.2022.137882
Chen, Y., Liu, C., Yang, Z., Sun, Y., Chen, X., and Liu, L. (2022d). Fabrication of zein-based hydrophilic nanoparticles for efficient gene delivery by layer-by-layer assembly. Int. J. Biol. Macromol. 217, 381–397. doi:10.1016/j.ijbiomac.2022.07.042
Cheng, C. J., Ferruzzi, M., and Jones, O. G. (2019). Fate of lutein-containing zein nanoparticles following simulated gastric and intestinal digestion. Food Hydrocoll. 87, 229–236. doi:10.1016/j.foodhyd.2018.08.013
Clinica Universidad de Navarra (2022). Zein nanoparticles for glycemic control (GLUCOCAPS), ClinicalTrials.gov. Available at: https://clinicaltrials.gov/ct2/show/study/NCT05560412#wrapper.
Corradini, E., Curti, P. S., Meniqueti, A. B., Martins, A. F., Rubira, A. F., and Muniz, E. C. (2014). Recent advances in food-packing, pharmaceutical and biomedical applications of zein and zein-based materials. Int. J. Mol. Sci. 15 (12), 22438–22470. doi:10.3390/ijms151222438
Dai, L., Sun, C., Wang, D., and Gao, Y. (2016). The interaction between zein and lecithin in ethanol-Water solution and characterization of zein-Lecithin composite colloidal nanoparticles. PLoS ONE 11 (11), e0167172. doi:10.1371/journal.pone.0167172
Danzer, L. A., Ades, H., and Rees, E. D. (1975). The helical content of zein, a water insoluble protein, in non-aqueous solvents. non-aqueous solvents 386 (5205), 26–31. doi:10.1016/0005-2795(75)90242-1
Demir, M., Ramos-Rivera, L., Silva, R., Nazhat, S. N., and Boccaccini, A. R. (2017). Zein-based composites in biomedical applications. J. Biomed. Mater. Res. Part A 105 (6), 1656–1665. doi:10.1002/jbm.a.36040
Deo, N., Jockusch, S., Turro, N. J., and Somasundaran, P. (2003). Surfactant interactions with zein protein. Langmuir 19 (12), 5083–5088. doi:10.1021/la020854s
Dill, D. B. (1927). The behavior of the prolamins in mixed solvents. II. J. Biol. Chem. 72 (1), 239–247. doi:10.1016/s0021-9258(18)84375-7
Dong, F., Dong, X., Zhou, L., Xiao, H., Ho, P. Y., Wong, M. S., et al. (2016). Doxorubicin-loaded biodegradable self-assembly zein nanoparticle and its anti-cancer effect: Preparation, in vitro evaluation, and cellular uptake. Colloids Surfaces B Biointerfaces. 140, 324–331. doi:10.1016/j.colsurfb.2015.12.048
Duarte, K., Justino, C. I. L., Gomes, A. M., Rocha-Santos, T., and Duarte, A. C. (2014). Green analytical methodologies for preparation of extracts and analysis of bioactive compounds. Compr. Anal. Chem. 65, 59–78. Elsevier B.V. doi:10.1016/B978-0-444-63359-0.00004-5
El Sharkawi, F. Z., Ewais, S. M., Fahmy, R. H., and Rashed, L. A. (2017). PTEN and TRAIL genes loaded zein nanoparticles as potential therapy for hepatocellular carcinoma. J. Drug Target. 25 (6), 513–522. doi:10.1080/1061186X.2017.1289536
El-Lakany, S. A., Elgindy, N. A., Helmy, M. W., Abu-Serie, M. M., and Elzoghby, A. O. (2018). Lactoferrin-decorated vs PEGylated zein nanospheres for combined aromatase inhibitor and herbal therapy of breast cancer. Expert Opin. Drug Deliv. 15 (9), 835–850. doi:10.1080/17425247.2018.1505858
Erenstein, O., Jaleta, M., Sonder, K., Mottaleb, K., and Prasanna, B. M. (2022). Global maize production, consumption and trade: Trends and R&D implications. Food Secur. 14 (5), 1295–1319. doi:10.1007/s12571-022-01288-7
Erickson, D. P., Ozturk, O. K., Selling, G., Chen, F., Campanella, O. H., and Hamaker, B. R. (2020). Corn zein undergoes conformational changes to higher β-sheet content during its self-assembly in an increasingly hydrophilic solvent. Int. J. Biol. Macromol. 157, 232–239. doi:10.1016/j.ijbiomac.2020.04.169
Esen, A. (1986). Separation of alcohol-soluble proteins (zeins) from maize into three fractions by differential solubility. Plant Physiol. 80, 623–627. doi:10.1104/pp.80.3.623
Evans, C. D., and Manley, R. H. (1941). Solvents for zein. Primary solvents. Industrial Eng. Chem. 33 (11), 1416–1417. doi:10.1021/ie50383a019
Evans, C. D., and Manley, R. H. (1944). Ternary solvents for zein. Industrial Eng. Chem. 36 (5), 408–410. doi:10.1021/ie50413a010
Farris, E., Brown, D. M., Ramer-tait, A. E., and Pannier, A. K. (2017). Chitosan-zein nano-in-microparticles capable of mediating in vivo transgene expression following oral delivery. J. Control. Release 249, 150–161. doi:10.1016/j.jconrel.2017.01.035
Fernández-Carneado, J., Kogan, M. J., Castel, S., and Giralt, E. (2004). Potential peptide carriers: Amphipathic proline-rich peptides derived from the N-terminal domain of γ-zein. Angew. Chem. - Int. Ed. 43 (14), 1811–1814. doi:10.1002/anie.200352540
Foo, L. Y., Lu, Y., Howell, A. B., and Vorsa, N. (2000). The structure of cranberry proanthocyanidins which inhibit adherence of uropathogenic P-fimbriated Escherichia coli in vitro. Phytochemistry 54 (2), 173–181. doi:10.1016/S0031-9422(99)00573-7
Forato, L. A., Doriguetto, A. C., Fischer, H., Mascarenhas, Y. P., Craievich, A. F., and Colnago, L. A. (2004). Conformation of the Z19 prolamin by FTIR, NMR, and SAXS. J. Agric. Food Chem. 52, 2382–2385. doi:10.1021/jf035020+
Franco, P., and Marco, I. D. (2020). Supercritical antisolvent process for pharmaceutical applications: A review. Processes 8 (8), 938. doi:10.3390/PR8080938
Fu, D., and Weller, C. L. (1999). Rheology of zein solutions in aqueous ethanol. J. Agric. Food Chem. 47 (5), 2103–2108. doi:10.1021/jf9811121
Gagliardi, A., Paolino, D., Costa, N., Fresta, M., and Cosco, D. (2021). Zein-vs PLGA-based nanoparticles containing rutin: A comparative investigation. Mater. Sci. Eng. 118, 111538. doi:10.1016/j.msec.2020.111538
Gaigbe-Togbe, V., Bassarsky, L., Gu, D., Spoorenberg, T., and Zeifman, L. (2022). World population prospects 2022: Summary of results. New York: United Nations Department of Economic and Social Affairs. World Population Prospects 2022: Summary of Results.
Gao, Z., Chen, G., Lu, W., Wu, Y., Hu, B., Xuet, L., et al. (2021). Interfacial and emulsion-stabilizing properties of zein nanoparticles: Differences among zein fractions (α-β-and γ-zein). Food Funct. 12 (3), 1361–1370. doi:10.1039/d0fo02536d
Gao, Z., Ding, P., Zhang, L., Shi, J., Yuan, S., Wei, J., et al. (2007). Study of a Pingyangmycin delivery system: Zein/Zein-SAIB in situ gels. Int. J. Pharm. 328 (1), 57–64. doi:10.1016/j.ijpharm.2006.07.048
Garratt, R., Oliva, G., Caracelli, I., Leite, A., and Arruda, P. (1993). Studies of the zein-like α-prolamins based on an analysis of amino acid sequences: Implications for their evolution and three-dimensional structure. Protein Struct. Funct. Genet. 15 (1), 88–99. doi:10.1002/prot.340150111
Geraghty, D., Peifer, M. A., Rubenstein, I., and Messing, J. (1981). The primary structure of a plant storage protein: Zein. Nucleic Acids Res. 9 (19), 5163–5174. doi:10.1093/nar/9.19.5163
Gomez-Estaca, J., Balaguer, M. P., Gavara, R., and Hernandez-Munoz, P. (2012). Formation of zein nanoparticles by electrohydrodynamic atomization: Effect of the main processing variables and suitability for encapsulating the food coloring and active ingredient curcumin. Food Hydrocoll. 28 (1), 82–91. doi:10.1016/j.foodhyd.2011.11.013
Hanna, E. A., Mendez Lopez, O. E., Salinas, F., Astete, C. E., Tamez, C., Wang, Y., et al. (2022). Zein nanoparticles for enhanced translocation of pesticide in soybean (Glycine max). ACS Agric. Sci. Technol. 2 (5), 1013–1022. doi:10.1021/acsagscitech.2c00160
Hasanzadeh, M., Farhadi, K., and Tajik, H. (2021). Zein film as a novel natural biopolymer membrane in electrochemical detections. J. Solid State Electrochem. 25 (4), 1327–1337. doi:10.1007/s10008-021-04910-4
Henderson, R. K., Jiménez-González, C., Constable, D. J. C., Alston, S. R., Inglis, G. G. A., Fisher, G., et al. (2011). Expanding GSK’s solvent selection guide – embedding sustainability into solvent selection starting at medicinal chemistry. Green Chem. 13 (4), 854–862. doi:10.1039/c0gc00918k
Hurtado-López, P., and Murdan, S. (2010). An investigation into the adjuvanticity and immunogenicity of zein microspheres being researched as drug and vaccine carriers. J. Pharm. Pharmacol. 58 (6), 769–774. doi:10.1211/jpp.58.6.0007
Iravani, S., and Varma, R. S. (2019). Plants and plant-based polymers as scaffolds for tissue engineering. Green Chem. 21 (18), 4839–4867. doi:10.1039/c9gc02391g
Jiang, Q., Reddy, N., and Yang, Y. (2010). Cytocompatible cross-linking of electrospun zein fibers for the development of water-stable tissue engineering scaffolds. Acta Biomater. 6 (10), 4042–4051. doi:10.1016/j.actbio.2010.04.024
Joshi, D. R., and Adhikari, N. (2019). An overview on common organic solvents and their toxicity. J. Pharm. Res. Int. 28 (3), 1–18. doi:10.9734/jpri/2019/v28i330203
Jovic, T. H., Kungwengwe, G., Mills, A. C., and Whitaker, I. S. (2019). Plant-derived biomaterials: A review of 3D bioprinting and biomedical applications. Front. Mech. Eng. 5 (19), 1–18. doi:10.3389/fmech.2019.00019
Kang, S., He, Y., Yu, D. G., Li, W., and Wang, K. (2021). Drug–zein@lipid hybrid nanoparticles: Electrospraying preparation and drug extended release application. Colloids Surfaces B Biointerfaces 201, 111629. doi:10.1016/j.colsurfb.2021.111629
Kasaai, M. R. (2018). Zein and zein -based nano-materials for food and nutrition applications: A review. Trends Food Sci. Technol. 79, 184–197. doi:10.1016/j.tifs.2018.07.015
Khalil, A. A., Deraz, S. F., Elrahman, S. A., and El-fawal, G. (2015). Enhancement of mechanical properties, microstructure, and antimicrobial activities of zein films cross-linked using succinic anhydride, eugenol, and citric acid. Prep. Biochem. Biotechnol. 45, 551–567. doi:10.1080/10826068.2014.940967
Khan, M. A., Yue, C., Fang, Z., Hu, S., Cheng, H., Bakry, A. M., et al. (2019). Alginate/chitosan-coated zein nanoparticles for the delivery of resveratrol. J. Food Eng. 258, 45–53. doi:10.1016/j.jfoodeng.2019.04.010
Khatri, M., Khatri, Z., El-Ghazali, S., Hussain, N., Qureshi, U. A., Kobayashi, S., et al. (2020). Zein nanofibers via deep eutectic solvent electrospinning: Tunable morphology with super hydrophilic properties. Sci. Rep. 10 (1), 15307–15311. doi:10.1038/s41598-020-72337-4
Kim, S., Sessa, D. J., and Lawton, J. W. (2004). Characterization of zein modified with a mild cross-linking agent. Industrial Crops Prod. 20 (3), 291–300. doi:10.1016/j.indcrop.2003.10.013
Labib, G. (2018). Overview on zein protein: A promising pharmaceutical excipient in drug delivery systems and tissue engineering. Expert Opin. Drug Deliv. 15 (1), 65–75. doi:10.1080/17425247.2017.1349752
Lai, L. F., and Guo, H. X. (2011). Preparation of new 5-fluorouracil-loaded zein nanoparticles for liver targeting. Int. J. Pharm. 404 (1–2), 317–323. doi:10.1016/j.ijpharm.2010.11.025
Lau, E. T. L., Giddings, S. J., Mohammed, S. G., Dubois, P., Johnson, S. K., Stanley, R. A., et al. (2013). Encapsulation of hydrocortisone and mesalazine in zein microparticles. Pharmaceutics 5 (2), 277–293. doi:10.3390/pharmaceutics5020277
Lau, E. T. L., Johnson, S. K., Mikkelsen, D., Halley, P. J., and Steadman, K. J. (2012). Preparation and in vitro release of zein microparticles loaded with prednisolone for oral delivery. J. Microencapsul. 29 (7), 706–712. doi:10.3109/02652048.2012.686527
Lawton, J. W. (2002). Zein: A history of processing and use. Cereal Chem. 79 (1), 1–18. doi:10.1094/CCHEM.2002.79.1.1
Lee, K. H., Jones, R. A., Dalby, A., and Tsai, C. Y. (1976). Genetic regulation of storage protein content in maize endosperm. Biochem. Genet. 14 (6100), 641–650. doi:10.1007/BF00485842
Lee, S., Alwahab, N. S. A., and Moazzam, Z. M. (2013). Zein-based oral drug delivery system targeting activated macrophages. Int. J. Pharm. 454 (1), 388–393. doi:10.1016/j.ijpharm.2013.07.026
Li, F., Chen, Y., Liu, S., Pan, X., Liu, Y., Zhao, H., et al. (2019a). The effect of size, dose, and administration route on zein nanoparticle immunogenicity in BALB/c mice. Int. J. Nanomedicine 14, 9917–9928. doi:10.2147/IJN.S226466
Li, F., Chen, Y., Liu, S., Qi, J., Wang, W., Wang, C., et al. (2017). Size-controlled fabrication of zein nano/microparticles by modified anti-solvent precipitation with/without sodium caseinate. Int. J. Nanomedicine 12, 8197–8209. doi:10.2147/IJN.S143733
Li, H., Wang, D., Liu, C., Zhu, J., Fan, M., Sun, X., et al. (2019b). Fabrication of stable zein nanoparticles coated with soluble soybean polysaccharide for encapsulation of quercetin. Food Hydrocoll. 87, 342–351. doi:10.1016/j.foodhyd.2018.08.002
Li, H., Yuan, Y., Zhu, J., Wang, T., Wang, D., and Xu, Y. (2020). Zein/soluble soybean polysaccharide composite nanoparticles for encapsulation and oral delivery of lutein. Food Hydrocoll. 103, 105715. doi:10.1016/j.foodhyd.2020.105715
Li, M. F., Chen, L., Xu, M. Z., Zhang, J. L., Wang, Q., Zeng, Q. Z., et al. (2018). The formation of zein-chitosan complex coacervated particles: Relationship to encapsulation and controlled release properties. Int. J. Biol. Macromol. 116, 1232–1239. doi:10.1016/j.ijbiomac.2018.05.107
Li, M., and Yu, M. (2020). Development of a nanoparticle delivery system based on zein/polysaccharide complexes. J. Food Sci. 85 (12), 4108–4117. doi:10.1111/1750-3841.15535
Li, Y., Li, J., Xia, Q., Zhang, B., Wang, Q., and Huang, Q. (2012). Understanding the dissolution of α-zein in aqueous ethanol and acetic acid solutions. J. Phys. Chem. B 116 (39), 12057–12064. doi:10.1021/jp305709y
Li, Y., Xia, Q., Shi, K., and Huang, Q. (2011). Scaling behaviors of α-zein in acetic acid solutions. J. Phys. Chem. B 115 (32), 9695–9702. doi:10.1021/jp203476m
Liang, X., Cao, K., Li, W., Li, X., McClements, D. J., and Hu, K. (2021). Tannic acid-fortified zein-pectin nanoparticles: Stability, properties, antioxidant activity, and in vitro digestion. Food Res. Int. 145, 110425. doi:10.1016/j.foodres.2021.110425
Lin, M., Fang, S., Zhao, X., Liang, X., and Wu, D. (2020). Natamycin-loaded zein nanoparticles stabilized by carboxymethyl chitosan: Evaluation of colloidal/chemical performance and application in postharvest treatments. Food Hydrocoll. 106, 105871. doi:10.1016/j.foodhyd.2020.105871
Liu, E., Su, Z., Yang, C., Ji, Y., Liu, B., and Meng, X. (2021). Fabrication, characterization and properties of DHA-loaded nanoparticles based on zein and PLGA. Food Chem. 360, 129957. doi:10.1016/j.foodchem.2021.129957
Liu, Q., Jing, Y., Han, C., Zhang, H., and Tian, Y. (2019). Encapsulation of curcumin in zein/caseinate/sodium alginate nanoparticles with improved physicochemical and controlled release properties. Food Hydrocoll. 93, 432–442. doi:10.1016/j.foodhyd.2019.02.003
Liu, X., Sun, Q., Wang, H., Zhang, L., and Wang, J. (2005). Microspheres of corn protein, zein, for an ivermectin drug delivery system. Biomaterials 26 (1), 109–115. doi:10.1016/j.biomaterials.2004.02.013
Lohcharoenkal, W., Wang, L., Chen, Y. C., and Rojanasakul, Y. (2014). Protein nanoparticles as drug delivery carriers for cancer therapy. BioMed Res. Int. 2014 (1), 1–12. doi:10.1155/2014/180549
Lucio, D., Martínez-Ohárriz, M. C., Jaras, G., Aranaz, P., González-Navarro, C. J., Radulescu, A., et al. (2017). Optimization and evaluation of zein nanoparticles to improve the oral delivery of glibenclamide. in vivo study using C. elegans. Eur. J. Pharm. Biopharm. 121, 104–112. doi:10.1016/j.ejpb.2017.09.018
Luo, Y., Teng, Z., and Wang, Q. (2012). Development of zein nanoparticles coated with carboxymethyl chitosan for encapsulation and controlled release of vitamin D3. J. Agric. Food Chem. 60 (3), 836–843. doi:10.1021/jf204194z
Luo, Y., Teng, Z., Wang, T. T. Y., and Wang, Q. (2013). Cellular uptake and transport of zein nanoparticles: Effects of sodium caseinate. J. Agric. Food Chem. 61 (31), 7621–7629. doi:10.1021/jf402198r
Luo, Y., Zhang, B., Cheng, W., and Wang, Q. (2010). Preparation, characterization and evaluation of selenite-loaded chitosan/TPP nanoparticles with or without zein coating. Carbohydr. Polym. 82 (3), 942–951. doi:10.1016/j.carbpol.2010.06.029
Luo, Y., Zhang, B., Whent, M., Yu, L. L., and Wang, Q. (2011). Preparation and characterization of zein/chitosan complex for encapsulation of α-tocopherol, and its in vitro controlled release study. Colloids Surfaces B Biointerfaces 85 (2), 145–152. doi:10.1016/j.colsurfb.2011.02.020
Ma, J. J., Yu, Y. G., Yin, S. W., Tang, C. H., and Yang, X. Q. (2018). Cellular uptake and intracellular antioxidant activity of zein/chitosan nanoparticles incorporated with quercetin. J. Agric. Food Chem. 66 (48), 12783–12793. doi:10.1021/acs.jafc.8b04571
Madan, P. L. (1978). Microencapsulation I. Phase separation or coacervation. Drug Dev. Industrial Pharm. 4 (1), 95–116. doi:10.3109/03639047809055641
Manley, R. H., and Evans, C. D. (1943). Binary solvents for zein. Industrial Eng. Chem. 35 (6), 661–665. doi:10.1021/ie50402a008
Matsushima, N., Danno, G., Takezawa, H., and Izumi, Y. (1997). Three-dimensional structure of maize α-zein proteins studied by small-angle X-ray scattering. Biochimica Biophysica Acta (BBA) - Protein Struct. Mol. Enzym. 1339 (1), 14–22. doi:10.1016/S0167-4838(96)00212-9
Meewan, J., Somani, S., Almowalad, J., Laskar, P., Mackenzie, G., Khadke, S., et al. (2022). Preparation of zein-based nanoparticles: Nanoprecipitation versus microfluidic-assisted manufacture, effects of PEGylation on nanoparticle characteristics and cellular uptake by melanoma cells. Int. J. Nanomedicine 17, 2809–2822. doi:10.2147/IJN.S366138
Mishra, B., Patel, B. B., and Tiwari, S. (2010). Colloidal nanocarriers: A review on formulation technology, types and applications toward targeted drug delivery. Nanomedicine Nanotechnol. Biol. Med. 6 (1), 9–24. doi:10.1016/j.nano.2009.04.008
Momany, F. A., Sessa, D. J., Lawton, J. W., Selling, G. W., Hamaker, S. A. H., and Willett, J. L. (2006). Structural characterization of α-zein. J. Agric. Food Chem. 54 (2), 543–547. doi:10.1021/jf058135h
Muthuselvi, L., and Dhathathreyan, A. (2006). Simple coacervates of zein to encapsulate Gitoxin. Colloids Surfaces B Biointerfaces. 51 (1), 39–43. doi:10.1016/j.colsurfb.2006.05.012
Ni, N., Zhang, D., and Dumont, M. J. (2018). Synthesis and characterization of zein-based superabsorbent hydrogels and their potential as heavy metal ion chelators. Polym. Bull. 75, 31–45. doi:10.1007/s00289-017-2017-z
Paliwal, R., and Palakurthi, S. (2014). Zein in controlled drug delivery and tissue engineering. J. Control. Release 189, 108–122. doi:10.1016/j.jconrel.2014.06.036
Pan, K., and Zhong, Q. (2016). Low energy, organic solvent-free co-assembly of zein and caseinate to prepare stable dispersions. Food Hydrocoll. 52, 600–606. doi:10.1016/j.foodhyd.2015.08.014
Park, C. E., Park, D. J., and Kim, B. K. (2015). Effects of a chitosan coating on properties of retinol-encapsulated zein nanoparticles. Food Sci. Biotechnol. 24 (5), 1725–1733. doi:10.1007/s10068-015-0224-7
Parris, N., Cooke, P. H., Moreau, R. A., and Hicks, K. B. (2008). Encapsulation of essential oils in zein nanospherical particles. ACS Symp. Ser. 992, 175–192. doi:10.1021/bk-2008-0992.ch010
Parris, N., and Dickey, L. C. (2001). Extraction and solubility characteristics of zein proteins from dry-milled corn. J. Agric. Food Chem. 49 (8), 3757–3760. doi:10.1021/jf0011790
Pascoli, M., de Lima, R., and Fraceto, L. F. (2018). Zein nanoparticles and strategies to improve colloidal stability: A mini-review. Front. Chem. 6 (6), 6. doi:10.3389/fchem.2018.00006
Patel, A. R., Bouwens, E. C. M., and Velikov, K. P. (2010). Sodium caseinate stabilized zein colloidal particles. J. Agric. Food Chem. 58 (23), 12497–12503. doi:10.1021/jf102959b
Pérez-Guzmán, C. J., and Castro-Muñoz, R. (2020). A review of zein as a potential biopolymer for tissue engineering and nanotechnological applications. Processes 8 (11), 1376. doi:10.3390/pr8111376
Perumal, O., Podaralla, S., and Kaushik, R. (2009). Method of forming non-immunogenic hydrophobic protein nanoparticles, and uses therefor. International Publication Number: WO2009137112A1, PCT/US2009/002935.
Pimentel, D., and Pimentel, M. (2006). Global environmental resources versus world population growth. Ecol. Econ. 59 (2), 195–198. doi:10.1016/j.ecolecon.2005.11.034
Podaralla, S., Averineni, R., Alqahtani, M., and Perumal, O. (2012). Synthesis of novel biodegradable methoxy poly(ethylene glycol)-zein micelles for effective delivery of curcumin. Mol. Pharm. 9 (9), 2778–2786. doi:10.1021/mp2006455
Podaralla, S., and Perumal, O. (2012). Influence of formulation factors on the preparation of zein nanoparticles. AAPS PharmSciTech 13 (3), 919–927. doi:10.1208/s12249-012-9816-1
Prasad, A., Astete, C. E., Bodoki, A. E., Windham, M., Bodoki, E., and Sabliov, C. M. (2017). Zein nanoparticles uptake and translocation in hydroponically grown sugar cane plants. J. Agric. Food Chem. 66 (26), 6544–6551. doi:10.1021/acs.jafc.7b02487
Prat, D., Pardigon, O., Flemming, H., Letestu, S., Ducandas, V., Isnard, P., et al. (2013). Sanofi ’s solvent selection guide: A step toward more sustainable processes. Org. Process Res. Dev. 17, 1517–1525. doi:10.1021/op4002565
Prior, R. L., and Gu, L. (2005). Occurrence and biological significance of proanthocyanidins in the American diet. Phytochemistry 66 (18), 2264–2280. doi:10.1016/j.phytochem.2005.03.025
Reboredo, C., González-Navarro, C. J., Martínez-López, A. L., and Irache, J. M. (2022). Oral administration of zein-based nanoparticles reduces glycemia and improves glucose tolerance in rats. Int. J. Pharm. 628, 122255. doi:10.1016/j.ijpharm.2022.122255
Reboredo, C., González-Navarro, C. J., Martínez-Oharriz, C., Martínez-López, A. L., and Irache, J. M. (2021). Preparation and evaluation of PEG-coated zein nanoparticles for oral drug delivery purposes. Int. J. Pharm. 597, 120287. doi:10.1016/j.ijpharm.2021.120287
Reboul, E., Richelle, M., Perrot, E., Desmoulins-Malezet, C., Pirisi, V., and Borel, P. (2006). Bioaccessibility of carotenoids and vitamin E from their main dietary sources. J. Agric. Food Chem. 54 (23), 8749–8755. doi:10.1021/jf061818s
Reddy, N., and Yang, Y. (2011). Potential of plant proteins for medical applications. Trends Biotechnol. 29 (10), 490–498. doi:10.1016/j.tibtech.2011.05.003
Regier, M. C., Taylor, J. D., Borcyk, T., Yang, Y., and Pannier, A. K. (2012). Fabrication and characterization of DNA-loaded zein nanospheres. J. Nanobiotechnology 10, 44–13. doi:10.1186/1477-3155-10-44
Ristroph, K. D., Astete, C. E., Bodoki, E., and Sabliov, C. M. (2017). Zein nanoparticles uptake by hydroponically grown soybean plants. Environ. Sci. Technol. 51 (24), 14065–14071. doi:10.1021/acs.est.7b03923
Rodríguez-Félix, F., Del-Toro-Sánchez, C. L., and Tapia-Hernández, J. A. (2020). A new design for obtaining of white zein micro- and nanoparticles powder: Antisolvent-dialysis method. Food Sci. Biotechnol. 29 (5), 619–629. doi:10.1007/s10068-019-00702-9
Rosa, M. T. M. G., Alvarez, V. H., Albarelli, J. Q., Santos, D. T., Meireles, M. A. A., and Saldaña, M. D. A. (2020). Supercritical anti-solvent process as an alternative technology for vitamin complex encapsulation using zein as wall material: Technical-economic evaluation. J. Supercrit. Fluids 159, 104499. doi:10.1016/j.supflu.2019.03.011
Sabra, S. A., Elzoghby, A. O., Sheweita, S. A., Haroun, M., Helmy, M. W., Eldemellawy, M. A., et al. (2018). Self-assembled amphiphilic zein-lactoferrin micelles for tumor targeted co-delivery of rapamycin and wogonin to breast cancer. Eur. J. Pharm. Biopharm. 128, 156–169. doi:10.1016/j.ejpb.2018.04.023
Santos-Buelga, C., and Scalbert, A. (2000). Proanthocyanidins and tannin-like compounds - nature, occurrence, dietary intake and effects on nutrition and health. J. Sci. Food Agric. 80 (7), 1094–1117. doi:10.1002/(SICI)1097-0010(20000515)80:7<1094::AID-JSFA569>3.0.CO;2-1
Selling, G. W., Hamaker, S. A. H., and Sessa, D. J. (2007). Effect of solvent and temperature on secondary and tertiary structure of zein by circular dichroism. Cereal Chem. 84 (3), 265–270. doi:10.1094/CCHEM-84-3-0265
Serrano, J., Goñi, I., and Saura-Calixto, F. (2005). Determination of β-carotene and lutein available from green leafy vegetables by an in vitro digestion and colonic fermentation method. J. Agric. Food Chem. 53 (8), 2936–2940. doi:10.1021/jf0480142
Shaw, L. A., McClements, D. J., and Decker, E. A. (2007). Spray-dried multilayered emulsions as a delivery method for ω-3 fatty acids into food systems. J. Agric. Food Chem. 55 (8), 3112–3119. doi:10.1021/jf063068s
Shi, K., Kokini, J. L., and Huang, Q. (2009). Engineering zein films with controlled surface morphology and hydrophilicity. J. Agric. Food Chem. 57 (6), 2186–2192. doi:10.1021/jf803559v
Shim, G., Kim, D., Le, Q., Park, G. T., Kwon, T., and Oh, Y. (2018). Nonviral delivery systems for cancer gene therapy: Strategies and challenges. Curr. Gene Ther. 18, 3–20. doi:10.2174/1566523218666180119121949
Shukla, R., and Cheryan, M. (2001). Zein: The industrial protein from corn. Industrial Crops Prod. 13 (3), 171–192. doi:10.1016/S0926-6690(00)00064-9
Soe, Z. C., Ou, W., Gautam, M., Poudel, K., Kim, B. K., Pham, L. M., et al. (2019). Development of folate-functionalized PEGylated zein nanoparticles for ligand-directed delivery of paclitaxel. Pharmaceutics 11 (11), 562. doi:10.3390/pharmaceutics11110562
Song, G., Liu, J., Wang, Q., Wang, D., Chu, B., Li, L., et al. (2022). Layer-by-layer self-assembly of hollow dextran sulfate/chitosan-coated zein nanoparticles loaded with crocin: Fabrication, structural characterization and potential biological fate. Food Hydrocoll. 125, 107420. doi:10.1016/j.foodhyd.2021.107420
Song, J., Liu, J., Wang, Q., Wang, D., Chu, B., Li, L., et al. (2021). Prolamin-based complexes: Structure design and food-related applications. Compr. Rev. Food Sci. Food Saf. 20, 1120–1149. doi:10.1111/1541-4337.12713
Sun, C., Dai, L., and Gao, Y. (2016). Binary complex based on zein and propylene glycol alginate for delivery of quercetagetin. Biomacromolecules 17 (12), 3973–3985. doi:10.1021/acs.biomac.6b01362
Swallen, L. C. (1941). Zein. A new industrial protein. Industrial Eng. Chem. 33 (3), 394–398. doi:10.1021/ie50375a026
Swarbrick, J. (2007). “Tablet manufacture by direct compression,” in Encyclopedia of pharmaceutical Technology (Boca Raton: CRC Press), 11. doi:10.1201/b19309-20
Tamasiga, P., Miri, T., Onyeaka, H., and Hart, A. (2022). Food waste and circular economy: Challenges and opportunities. Sustainability 14 (16), 9896. doi:10.3390/su14169896
Tapia-Hernández, J. A., Del-Toro-Sánchez, C. L., Cinco-Moroyoqui, F. J., Juárez-Onofre, J. E., Ruiz-Cruz, S., Carvajal-Millan, E., et al. (2019). Prolamins from cereal by-products: Classification, extraction, characterization and its applications in micro- and nanofabrication. Trends Food Sci. Technol. 90, 111–132. doi:10.1016/j.tifs.2019.06.005
Tatham, A. S., Field, J. M., Morris, V. J., I'Anson, K. J., Cardle, L., Dufton, M. J., et al. (1993). Solution conformational analysis of the alpha-zein proteins of maize. J. Biol. Chem. 268 (35), 26253–26259. doi:10.1016/S0021-9258(19)74308-7
Torres-Giner, S., Ocio, M. J., and Lagaron, J. M. (2009). Novel antimicrobial ultrathin structures of zein/chitosan blends obtained by electrospinning. Carbohydr. Polym. 77 (2), 261–266. doi:10.1016/j.carbpol.2008.12.035
Tortorella, S., Maturi, M., Vetri Buratti, V., Vozzolo, G., Locatelli, E., Sambri, L., et al. (2021). Zein as a versatile biopolymer: Different shapes for different biomedical applications. RSC Adv. 11 (62), 39004–39026. doi:10.1039/d1ra07424e
Tran, P. H. L., Duan, W., Lee, B., and Tran, T. T. D. (2019). The use of zein in the controlled release of poorly water-soluble drugs. Int. J. Pharm. 566, 557–564. doi:10.1016/j.ijpharm.2019.06.018
van Ballegooie, C., Wretham, N., Ren, T., Popescu, I. M., Yapp, D. T., and Bally, M. B. (2022). PEG conjugated zein nanoparticles for in vivo use. Pharmaceutics 14 (9), 1831. doi:10.3390/pharmaceutics14091831
Wang, H. J., Lin, Z. X., Liu, X. M., Sheng, S. Y., and Wang, J. Y. (2005). Heparin-loaded zein microsphere film and hemocompatibility. J. Control. Release 105 (1–2), 120–131. doi:10.1016/j.jconrel.2005.03.014
Wang, J. J., Wang, Y., Wang, Q., Yang, J., Hu, S. Q., and Chenet, L. (2019). Mechanically strong and highly tough prolamin protein hydrogels designed from double-cross-linked assembled networks. ACS Appl. Polym. Mater. 1, 1272–1279. doi:10.1021/acsapm.9b00066
Wang, Q., Wang, Q., Wang, X., and Padua, G. W. (2006). Zein dynamic adsorption to carboxylic and alkyl coated surfaces. J. Agric. Food Chem. 54 (2), 517–522. doi:10.1021/jf051545l
Wang, Q., Xian, W., Li, S., Liu, C., and Padua, G. W. (2008). Topography and biocompatibility of patterned hydrophobic/hydrophilic zein layers. Acta Biomater. 4 (4), 844–851. doi:10.1016/j.actbio.2008.01.017
Wang, Y., and Padua, G. W. (2012). Nanoscale characterization of zein self-assembly. Langmuir 28 (5), 2429–2435. doi:10.1021/la204204j
Wei, Y., Sun, C., Dai, L., Zhan, X., and Gao, Y. (2018). Structure, physicochemical stability and in vitro simulated gastrointestinal digestion properties of β-carotene loaded zein-propylene glycol alginate composite nanoparticles fabricated by emulsification-evaporation method. Food Hydrocoll. 81, 149–158. doi:10.1016/j.foodhyd.2018.02.042
Wei, Y., Yu, Z., Lin, K., Sun, C., Dai, L., Yang, S., et al. (2019). Fabrication and characterization of resveratrol loaded zein-propylene glycol alginate-rhamnolipid composite nanoparticles: Physicochemical stability, formation mechanism and in vitro digestion. Food Hydrocoll. 95 (17), 336–348. doi:10.1016/j.foodhyd.2019.04.048
Weissmueller, N. T., Lu, H. D., Hurley, A., and Prud'Homme, R. K. (2016). Nanocarriers from GRAS zein proteins to encapsulate hydrophobic actives. Biomacromolecules 17 (11), 3828–3837. doi:10.1021/acs.biomac.6b01440
Wiggers, H. A., Fin, M. T., Khalil, N. M., and Mainardes, R. M. (2022). Polyethylene glycol-stabilized zein nanoparticles containing gallic acid. Food Technol. Biotechnol. 60 (2), 145–154. doi:10.17113/ftb.60.02.22.6981
Wilson, C. M. (1991). Multiple zeins from maize endosperms characterized by reversed-phase high performance liquid chromatography. Plant Physiol. 95 (3), 777–786. doi:10.1104/pp.95.3.777
Wu, Y., Luo, Y., and Wang, Q. (2012). Antioxidant and antimicrobial properties of essential oils encapsulated in zein nanoparticles prepared by liquid-liquid dispersion method. LWT - Food Sci. Technol. 48 (2), 283–290. doi:10.1016/j.lwt.2012.03.027
Wusigale, W. T., Hu, Q., Xue, J., Khan, M. A., Liang, L., Luo, Y., et al. (2021). Partition and stability of folic acid and caffeic acid in hollow zein particles coated with chitosan. Int. J. Biol. Macromol. 183, 2282–2292. doi:10.1016/j.ijbiomac.2021.05.216
Xu, H., Jiang, Q., Reddy, N., and Yang, Y. (2011). Hollow nanoparticles from zein for potential medical applications. J. Mater. Chem. 21 (45), 18227–18235. doi:10.1039/c1jm11163a
Yan, X., Li, M., Xu, X., Liu, X., and Liu, F. (2022). Zein-based nano-delivery systems for encapsulation and protection of hydrophobic bioactives: A review. Front. Nutr. 9, 999373. doi:10.3389/fnut.2022.999373
Yao, K., Chen, W., Song, F., McClements, D. J., and Hu, K. (2018). Tailoring zein nanoparticle functionality using biopolymer coatings: Impact on curcumin bioaccessibility and antioxidant capacity under simulated gastrointestinal conditions. Food Hydrocoll. 79, 262–272. doi:10.1016/j.foodhyd.2017.12.029
Ye, W., Zhang, G., Liu, X., Ren, Q., Huang, F., and Yan, Y. (2022a). Fabrication of polysaccharide-stabilized zein nanoparticles by flash nanoprecipitation for doxorubicin sustained release. J. Drug Deliv. Sci. Technol. 70, 103183. doi:10.1016/j.jddst.2022.103183
Ye, W., Zhu, F., Cai, Y., Wang, L., Zhang, G., Zhao, G., et al. (2022b). Improved paclitaxel delivery with PEG-b-PLA/zein nanoparticles prepared via flash nanoprecipitation. Int. J. Biol. Macromol. 221, 486–495. doi:10.1016/j.ijbiomac.2022.09.021
Yu, J., Lin, Y., Wang, G., Song, J., Hayat, U., Liu, C., et al. (2022). Zein-induced immune response and modulation by size, pore structure and drug-loading: Application for sciatic nerve regeneration. Acta Biomater. 140, 289–301. doi:10.1016/j.actbio.2021.11.035
Yu, X., Wu, H., Hu, H., Dong, Z., Dang, Y., Qi, Q., et al. (2020). Zein nanoparticles as nontoxic delivery system for maytansine in the treatment of non-small cell lung cancer. Drug Deliv. 27 (1), 100–109. doi:10.1080/10717544.2019.1704942
Yuan, Y., Ma, M., Wang, D., and Xu, Y. (2022a). A review of factors affecting the stability of zein-based nanoparticles loaded with bioactive compounds: From construction to application. Crit. Rev. Food Sci. Nutr. 7, 1–17. doi:10.1080/10408398.2022.2047881
Yuan, Y., Ma, M., Xu, Y., and Wang, D. (2022b). Surface coating of zein nanoparticles to improve the application of bioactive compounds: A review. Trends Food Sci. Technol. 120, 1–15. doi:10.1016/j.tifs.2021.12.025
Zhang, B., Luo, Y., and Wang, Q. (2011). Effect of acid and base treatments on structural, rheological, and antioxidant properties of α-zein. Food Chem. 124 (1), 210–220. doi:10.1016/j.foodchem.2010.06.019
Zhang, S., and Han, Y. (2018). Preparation, characterisation and antioxidant activities of rutin-loaded zein-sodium caseinate nanoparticles. PLoS ONE 13 (3), e0194951. doi:10.1371/journal.pone.0194951
Zhang, T., Yu, S., Tang, X., Ai, C., Chen, H., Lin, J., et al. (2022). Ethanol-soluble polysaccharide from sugar beet pulp for stabilizing zein nanoparticles and improving encapsulation of curcumin. Food Hydrocoll. 124 (PA), 107208. doi:10.1016/j.foodhyd.2021.107208
Zhang, Y., Cui, L., Che, X., Zhang, H., Shi, N., Li, C., et al. (2015). Zein-based films and their usage for controlled delivery: Origin, classes and current landscape. J. Control. Release 206 (2699), 206–219. doi:10.1016/j.jconrel.2015.03.030
Zhang, Y., Cui, L., Li, F., Shi, N., Li, C., Yu, X., et al. (2016). Design, fabrication and biomedical applications of zein-based nano/micro-carrier systems. Int. J. Pharm. 513 (1–2), 191–210. doi:10.1016/j.ijpharm.2016.09.023
Zhang, Y., Li, Y., Lin, C., Zhang, J., Gao, H., and Chen, J. (2021). Dioscin-loaded zein nanoparticles alleviate lipopolysaccharide-induced acute kidney injury via the microRNA-let 7i signalling pathways. IET Nanobiotechnology 15 (5), 465–472. doi:10.1049/nbt2.12051
Zhang, Y., Niu, Y., Luo, Y., Ge, M., Yang, T., Yu, L., et al. (2014). Fabrication, characterization and antimicrobial activities of thymolloaded zein nanoparticles stabilized by sodium caseinate-chitosan hydrochloride double layers. Food Chem. 142, 269–275. doi:10.1016/j.foodchem.2013.07.058
Zhong, Q., and Ikeda, S. (2012). Viscoelastic properties of concentrated aqueous ethanol suspensions of α-zein. Food Hydrocoll. 28 (1), 46–52. doi:10.1016/j.foodhyd.2011.11.014
Zhong, Q., and Jin, M. (2009). Zein nanoparticles produced by liquid-liquid dispersion. Food Hydrocoll. 23 (8), 2380–2387. doi:10.1016/j.foodhyd.2009.06.015
Zhong, Q., Tian, H., and Zivanovic, S. (2009). Encapsulation of fish oil in solid particles by liquid-liquid dispersion. J. Food Process. Preserv. 33, 255–270. doi:10.1111/j.1745-4549.2009.00390.x
Zhu, J. J., Huang, X. N., Yang, T., Tang, C. H., Yin, S. W., Jia, X. J., et al. (2022). An eco-friendly zein nanoparticle as robust cosmetic ingredient ameliorates skin photoaging. Industrial Crops Prod. 177, 114521. doi:10.1016/j.indcrop.2022.114521
Zimmerman, J. B., Anastas, P. T., Erythropel, H. C., and Leitner, W. (2020). Designing for a green chemistry future. Science 367 (6476), 397–400. doi:10.1126/science.aay3060
Keywords: drug delivery, green technologies, nanoparticles, zein, sustainable materials
Citation: Tivano F and Chiono V (2023) Zein as a renewable material for the preparation of green nanoparticles for drug delivery. Front. Front. Biomater. Sci. 2:1156403. doi: 10.3389/fbiom.2023.1156403
Received: 01 February 2023; Accepted: 28 April 2023;
Published: 12 May 2023.
Edited by:
Amir K. Miri, New Jersey Institute of Technology, United StatesReviewed by:
Muhammad Usman Ghori, University of Huddersfield, United KingdomMehtap Sahiner, Çanakkale Onsekiz Mart University, Türkiye
Berivan Cecen, Rowan University, United States
Copyright © 2023 Tivano and Chiono. This is an open-access article distributed under the terms of the Creative Commons Attribution License (CC BY). The use, distribution or reproduction in other forums is permitted, provided the original author(s) and the copyright owner(s) are credited and that the original publication in this journal is cited, in accordance with accepted academic practice. No use, distribution or reproduction is permitted which does not comply with these terms.
*Correspondence: Valeria Chiono, dmFsZXJpYS5jaGlvbm9AcG9saXRvLml0