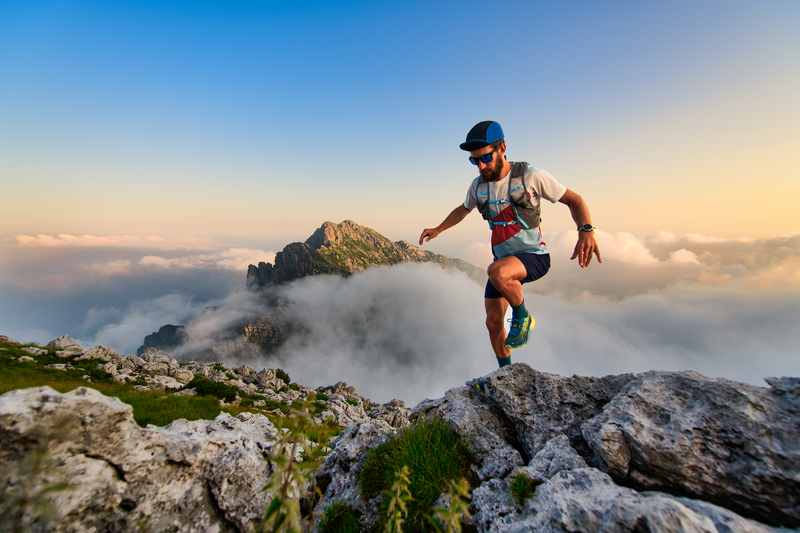
95% of researchers rate our articles as excellent or good
Learn more about the work of our research integrity team to safeguard the quality of each article we publish.
Find out more
ORIGINAL RESEARCH article
Front. Biomater. Sci. , 08 December 2022
Sec. Biomaterials Science for Regenerative Therapies
Volume 1 - 2022 | https://doi.org/10.3389/fbiom.2022.989708
This article is part of the Research Topic Comprehensive Biomaterial Strategies for Musculoskeletal Tissue Regeneration View all 9 articles
Osteoarthritis (OA) is a chronic degenerative joint disease, leading cause of disability in older adults and leads to pain, reduced mobility, and decreased quality of life. Mesenchymal stem cells (MSC) - based therapies are the precursor to all tissues within a joint and their potential in regeneration is complemented by a modulation of the local inflammatory response. The use of MSCS-based therapy for regenerative medicine, specifically OA, is challenged by the need to investigate the ideal MSC source, establish processing of harvesting and culture. Although bone marrow-derived mesenchymal stem cells (BM-MSCs) represent the gold standard in cell therapies for OA, synovial fluid-derived stem cells (SF-MSCs) can be a less invasive, promising alternative. Procedures to extract SFMSCs can be performed during arthrocentesis, arthroscopy or knee surgery with a minimally invasive act allowing personalized autologous therapies. SF-MSCs, isolated from human synovial fluid of patients suffering from advanced OA, retained stemness markers and inflammatory potential in 2D culture condition showing similar morphology and clonogenicity potential compared to BM-MSCs. To further boost their immunomodulatory properties, we coupled SF-MSCs with a biomimetic scaffold made of collagen and chondroitin sulfate (CL CS), previously reported as immune-tuning materials. The 3D culture further promoted immunosuppressive markers expression in SF-MSCs compared to 2D culture. Although ongoing clinical trials mainly used scaffold-free injection of MSCs, combination of mesenchymal cells and biomatrices could provide a useful tool to improve biological outcomes. A combination of SF-MSCs and 3D CL CS biomimetic scaffolds could represent a strong therapeutic effect as cell-based treatment for OA.
Osteoarthritis (OA) affects millions of people worldwide, more than 32.5 million adults in the United States, representing the most common form of chronic joint disease (Xia et al., 2014). It typically starts to develop over age 50 but can show up in younger individuals who mostly were affected by a prior joint injury, too (Hunter and Bierma-Zeinstra 2019). Many factors can contribute to the development, including an age-related pro-inflammatory state (termed “inflamm-aging”), picturing this disease as both a degenerative and inflammatory condition (Greene and Loeser 2015). Indeed, inflammatory mediators, and activation of synoviocytes, articular chondrocytes and other joint tissue cells into inflammatory phenotype are common in OA (Lieberthal et al., 2015), resulting in impairment of a proper healing. The standard of care for OA is mainly focused on reducing pain and improve function rather than a target therapy focused on tissue regeneration. However, cell therapies have been explored in the past 30 years in the attempt to introduce a regenerative therapy in the orthopedic practice (Jiang et al., 2011). The aim is to offer a long-term solution to restore mobility through promoting repair and regenerate cartilage, ease pain and symptoms and finally delay OA progression (Huang et al., 2018).
Among cell therapies available in the last few years, mesenchymal stem cells (MSCs) have been manifested as an ideal cell source for musculoskeletal tissue regeneration since their excellent properties. Sources of MSCs have been found in many tissues—including bone marrow (BM-MSCs), adipose tissue (adipose stem cells), synovium (SF-MSCs), and umbilical cord—are easily extracted, and do not imply ethical problems (Ren et al., 2012; Han et al., 2019). MSCs harbor self-renewal properties and directional differentiation into almost any end-stage lineage cells (Chen et al., 2008). Both in vitro and in vivo studies, showed their efficacy in treating various immune disorders, mainly due to their immunological properties (Aggarwal and Pittenger 2005; Uccelli et al., 2008; Gao et al., 2016). Among the sources cited, BM-MSCs represents the golden standard for regenerative treatments and, to date, BM-MSC have been one of the most investigated sources of MSCs in the treatments of OA. Promising outcomes were recorded in several studies, in which patients with knee OA were treated with ex-vivo expanded autologous bone marrow MSCs reported improvements in pain management, motility restoration, with no or mild transitory adverse events (Davatchi et al., 2011; Orozco et al., 2013; Davatchi et al., 2016; Soler et al., 2016; Al-Najar et al., 2017). Another source of MSCs with chondrogenic potential is SF-MSCs, often discarded like the infrapatellar fat pad during arthroscopy or open knee surgery (Ando et al., 2014; Jorgenson et al., 2018). SF- originate from the synovial membrane but also exist into a lubricating fluid within the joint cavity (Morito et al., 2008; Zhang et al., 2008). They represents a valuable cell source for regenerative medicine purposes since SF-MSCs are easily harvested in a minimally invasive manner through arthrocentesis (Koyama et al., 2011; Lee et al., 2012; Lee et al., 2012). Physiologically, MSCs are hypoimmunogenic, but they are responsive to inflammatory cytokines released in a pro-inflammatory environment (Bernardo and Fibbe 2013). For example, interferon-gamma (IFN-γ) together with TNF-α and IL-1β pro-inflammatory cytokines (Alsaleh et al., 2010) are cytokines mainly produced by natural killer cells, activated CD4+ Th1 cells and cytotoxic CD8+ cytotoxic T cells, which are strongly involved in the immune response of cartilage diseases (Schroder et al., 2004). Horse derived SF-MSCs showed immunosuppressive phenotype comparable with BM-MSC under IFN-γ stimulation (Zayed et al., 2021). In humans, however, this feature remains unclear for SF-MSCs (Garcia et al., 2016); indeed, while SF-MSCs growth, multipotency and CD profile characterization has been explored (Jones et al., 2008), their immunological and immunomodulatory properties is still under investigation and need to be defined.
Although many therapeutic strategies have been proposed to date, articular cartilage restoration still poses a treatment challenge, in part due to the inaccuracy of in vitro studies. To ameliorate in vitro studies relevance in studying both degeneration and inflammation mechanisms, three-dimensional (3D) culture systems were explored to investigate chondrogenesis and other processes related to OA progression in vitro, involving multiple cell types, including stem cells, stromal cells, macrophages, and other chondrogenic progenitors (Khurshid et al., 2018; Santos et al., 2018; Evans and Fiederlein 2020). To this aim, implementation of 3D culture systems with biomimetic acellular scaffold to accurately simulate cartilage physiological environment led to significant improvements in cartilage progenitor/stem cells immunomodulatory effects (Bauza et al., 2020). Combining cell-based therapy with 3D scaffolds chondrogenic and osteogenic potential those hybrid systems offer great potential as in vitro systems to model OA (Samvelyan et al., 2021).
In this research we mimicked native cartilage tissue utilizing a chondroitin sulfate (CS) porous collagen (CL) scaffold (previously developed by our group). Chondroitin sulfate is a sulfated glycosaminoglycan, building blocks of cartilage. It is also found in the extracellular matrix of many other connective tissues including bone, skin, ligaments and tendons (Hardingham 2008; Martel-Pelletier et al., 2008). Thanks to its anti-inflammatory and immunomodulatory activity (Monfort et al., 2008a), CS was explored as treatment of symptomatic OA, showing moderate efficacy rate (Monfort et al., 2008b). Indeed, CS functionalized scaffolds have been reported to direct macrophage immune modulation for tissue repair (Taraballi et al., 2016), recruiting and retaining immune cells after the implant, avoiding immunological rejection (Corradetti et al., 2017).
Our research exploited CS scaffold, an efficient 3D physiological chondrogenic niche mimicking model, to test SF-MSCs inflammatory modulation properties. SF-MSCs were evaluated as potential sources of MSCs in the treatments of OA while demonstrating the efficacy of advanced 3D culture techniques for the maintenance of primary cells’ intrinsic properties and phenotype in culture.
The Houston Methodist Research Institute IRB approved the collection of patient data and patient samples (IRB Pro00015718 and related protocols as noted below). All patients were consented prior to sample collection and sample processing. Their samples were anonymously marked and bio-banked with specific identification numbers.
Human synovial fluid, from 3 patients undergoing ACL reconstruction (Table 1), was obtained from the orthopedic biorepository at Houston Methodist Hospital Orthopedics and Sports Medicine Department (IRB CR00006624). The samples were individual and anonymously bio-banked with specific identification numbers. 4 ml of synovial fluid were washed three times with a solution of phosphate-buffered saline (PBS) 1:1 and then the whole extract was seeded in culture media (α-MEM) supplemented with 20% fetal bovine serum (FBS) and 1% penicillin (100 UI/ml)-streptomycin (100 mg/ml). Human bone marrow aspirate from two patients undergoing a total knee replacement surgery, was also obtained from the orthopedic biorepository at Houston Methodist Hospital Orthopedics and Sports Medicine Department (IRB CR00006624) and processed as previously reported (Bauza et al., 2020). Cells obtained after this process (p0) once at confluence were characterized for the expression of MSC-associated markers by Flow analysis (as describe bellow), expanded and cultured in α-MEM (Sigma-Aldrich, St. Louis, MO, United States) containing 20% (vol/vol) FBS supplemented with 1% penicillin (100 UI/ml)-streptomycin (100 mg/ml) (Gibco) and incubated at 37°C in low oxygen conditions Media was changed every 2 days until the cells were at 80% confluency, at which point they were passaged. Cells were used for experiments until P5 unless otherwise stated in specific experiments.
2D and 3D experiments were normally seeded simultaneously so direct comparisons could be made. For the 3D scaffold experiments, 2.5×105 cells were concentrated in 20 μL of medium and seeded on the center of each collagenous scaffold (CL and CL-CS) and kept in an incubator at 37°C, 5% CO2 for 30 min. Culture medium was then added to each well and scaffolds were kept in gentle agitation (Corradetti et al., 2016; Bauza et al., 2020; Paradiso F, et al., 2022). Medium was changed twice per week or according to the experiment design.
BM-MSCs and SF-MSCs were plated in concentrations of 500 cells, 200 cells, 100 cells, and 50 cells in P100 plates, cultured in αMEM, supplemented with FBS 20% and GlutaMAX 1%, and maintained for 2 weeks at 37°C in 5% CO2. The colonies were fixed to the plate via 10% neutral buffered formalin, stained with violet, and counted.
Both bone marrow and synovial fluid derived MSCs were collected and characterized for the expression of their associated surface cell markers. The percentage of stem cells was determined by Flow cytometer analysis not from the whole fluid but from the cells obtained after the BM and synovial fluid was processed. Briefly, cells were washed with Flow Cytometry Staining buffer and stained for 30 min at 4°C with negative cocktail markers (human leukocyte antigen–DR isotype HLA-DR, CD45, CD11b, CD19, and CD34) and positive anti-human cocktail markers (cluster differentiation CD90, CD73, CD105). Conjugated primary monoclonal antibodies and isotype controls were used as recommended by the manufacturer (BD Biosciences). Cells were analyzed on a FACS Fortessa.
The scaffolds were synthesized from type I bovine collagen (Viscofan) and fabricated with a freeze-dry technique. For CL scaffolds preparation, 5% acetic collagen slurry was prepared, adding 28 g of collagen to 50 ml of acetic acid 0.5 M. The same procedure was followed for CL CS but 2.8 g of chondroitin sulfate (Carbosynth, OC042731401) was added to the collagen slurry. The slurry was the washed 3 times with Milli-Q water (EMD Millipore) and sieved with a stainless-steel sieve and wet crosslinked adding 9 μL of 1,4-Butanediol diglycidyl ether (BDDGE) at RT for 24 h. Finally, the slurry was washed with Milli-Q water (EMD Millipore) and casted onto a 48-well plate and freeze dried using a ramping protocol from 25°C to −25°C and from −25°C to 25°C in 50 min under vacuum conditions (p = 0.20 mbar), to obtain the desired pore size and porosity.
CL and CL CS scaffolds of 0.5 cm thickness were soaked in PBS and loaded on UniVert Mechanical Test System. A load cell of 1 N was calibrated and used to perform a compression test with a stretch magnitude of 35%, stretch duration of 60 s, and relaxation time of 60 s. For each condition, 3 replicates were analyzed.
The morphology of the scaffold was characterized by FEI Nova NanoSEM 23. Scaffolds were coated with 7 nm of Pt/Pl (Nova NanoSEM 230) for scanning electron microscopy (SEM) examination. Scaffolds pores analysis was performed from SEM images, and 3 images from 3 areas were used for each scaffold at the same magnitude. For each image, porosity and circularity analysis was performed using ‘analyze particles’ measurement in ImageJ software (National Institutes of Health, Bethesda, MD, http://imagej.nih.gov/ij).
The samples were analyzed in attenuated total reflection (ATR)/Fourier transform infrared spectroscopy (FTIR) mode at 2 cm−1 resolution. 64points were recorded over the range of 500–4,000 cm−1 using a Nicolet 6700 spectrometer (ThermoFisher Scientific, Waltham, MA). The spectra were reported after background subtraction, baseline correction, and normalization on Amide I. Graph reported a range of 500–1800 cm−1 wavelength.
Viability staining was performed 48H after inflammation treatment using 2 μM Calcein-AM (Invitrogen, Cat #C3100MP) and 8 μM Ethidium Homodimer (Invitrogen, Cat #E1169) to stain live and dead cells respectively. Scaffolds were incubated with viability stains for 30 min at 37°C and transferred to glass cover slips prior to imaging. Z-stack images were acquired at ×10 magnification using A1 Nikon Confocal Imaging System (Nikon Corporation, Tokyo, Japan). Z-stacks are composed of ≥18 images at 15 μm intervals. Whole scaffold images were acquired at ×4 magnification using Keyence BZ×800 and final images produced via post-hoc stitching.
IDO secretion was quantified via enzyme-linked immunosorbent assay (ELISA) (Invitrogen, Cat #EH246RB) as per supplier’s instructions. Conditioned media was collected prior to inflammation, and following 48H inflammation with 40 ng/ml IFN-γ and TNF-α. Samples were diluted 1:10 prior to analysis. Standard curve was produced using 4-paramater fit generated via GraphPad Prism (Version 8.3.1).
Reverse transcription polymerase chain reaction (PCR) was performed on cells grown in 2D culture and on 3D scaffolds at different time points. Total RNA from 2D cultures and 3D culture were isolated using 1 ml of Trizol reagent (Life Technologies, ThermoFisher Scientific). Specifically, scaffolds with cells were washed in PBS and incubated with 1 ml TRIzol RT for 10 min under shaking conditions. After removal of the scaffold, standard procedure for RNA extraction was performed as followed: 200 µL of chloroform was added to all samples and inverted for 15 min, incubated on ice for 2 min, and centrifuged at 12,000× g for 15 min at 4°C. The aqueous phase was transferred to a 1.5 ml tube and 500 µL of isopropyl alcohol added, before incubating for 10 min at 4°C and centrifuging 12,000× g for 10 min at 4°C. After washing the pellet twice with 1 ml 70% ethanol, it was aspirated and allowed to dry before resuspending in 20 µL of water. For each sample, RNA concentration and purity were measured using a NanoDrop spectrophotometer (ND1000, NanoDrop, ThermoFisher Scientific). Total RNA (500 ng) was reverse transcribed into cDNA using the Bio-Rad iScript™ cDNA Synthesis Kit (Applied Biosystems, ThermoFisher Scientific). Quantitative PCR was performed using the TaqMan™ Fast Advanced Master Mix on a StepOnePlus Real-time PCR System (Applied Biosystems, Waltham, MA, United States).
The housekeeping marker included in the study was eukaryotic 18S rRNA (18S; Hs03003631_g). Immunosuppression associated markers were prostaglandin E synthase (PTGES; Hs01115610_m1), prostaglandin-endoperoxide synthase 2 (PTGS2; Hs00153133_m1), and indoleamine 2,3-dioxygenase 1 (IDO1; Hs00984148_m1).
Statistical analysis was performed using GraphPad for Windows (GraphPad Software). Three replicates for each experiment were performed, and the results are reported as mean ± SD, with p ≤ 0.05 used as a threshold for significance. Statistical analysis was performed using unpaired t-test with Welch’s correction and one-way ANOVA as specified in every experiment to compare differences between groups.
The immune phenotype of MSCs extracted from synovial fluid obtained from the 3 patients undergoing ACL reconstruction (Table 1) was characterized by evaluating the expression of stem cell markers. In particular, SF-MSCs have been selected as negative for hematopoietic markers, and positive for stem cell markers CD90, CD73 and CD105. Overall, we found and average of 22.12% of stem cells, precisely 22%, 27.84% and 16.53% from patient 1, patient 2 and patient 3, respectively (Figure 1A). As standard control, human BM-MSCs isolated from bone marrow collected from two patients undergoing a total knee replacement surgery (BM1 and BM2) were also selected for hematopoietic stem cell markers negativity, and positivity for stem cell markers (41% and 17% of MSCs from BM1 and BM2 respectively) (Table 1, Supplementary Figures S1A,B).
FIGURE 1. Synovial fluid MSCs isolation and stem cell characterization. (A) Extraction efficiency of SF-MSCs (stemness markers: hemopoietic markers-, CD90+CD73+CD105+). (B) Representative images of SF-MSCs in culture plate after their isolation. Scale bar = 10 μm. (C) Flow cytometry analysis of SF-MSCs: representative fluorescence intensity histogram displaying stem cell markers CD73+ (stemness markers: hematopoietic markers/CD45-, CD90+CD73+CD105+) at 24, 48, 72 h under 40 ng/ml TNFa/INFy treatment (untreated = control, basal level).
The clonogenicity potential of BM and SF-MSCs was also assessed. The colony-forming units consistently increased with the number of cells plated, as expected, with SF-MSCs showing higher colony formation than BM-MSCs for 100 and 500 cells seeding condition (Supplementary Figure S2).
SF-MSCs were expanded (Figure 1B) and culture for 48 h before being exposed to inflammatory mediators (40 ng/ml TNFα/INFy) to assess their stemness markers retention under inflammatory conditions. BM-MSCs and SF-MSCs did not show drastic loss of stemness markers (CD105, CD90 and CD73). Only after 72 h of treatment, a decrease of CD73 marker expression was observed in BM-MSCs (Supplementary Figure S1C) while only a slightly decrement in SF-MSCs (Figure 1C).
We used our previously developed chondroitin sulfate (CS) collagen based scaffold as tridimensional system to investigate SF-MSC properties, together with the control which is bare collagen (CL) (Volpi 2011). Before the cellular test, we characterized the overall scaffolds. To evaluate the CL and CL CS scaffolds’ properties we collected images using SEM after freeze-drying (Figure 2A). Scaffold structure was imaged, highlighting the interconnected pores with boundaries defined by sheet-like structures of fibrillar collagen, typical porous and fibrous substructure of collagen sponges.
FIGURE 2. Bare collagen (CL) and collagen and chondroitin sulfate (CL CS) scaffolds characterization. (A) SEM imaging were acquired for CL and CL CS scaffolds at different magnifications. Scale bar low magnification = 500μm; Scale bar high magnification = 5 μm. (B) FTIR spectra of CL and CL CS scaffolds plotting wavelength/absorbance. (C) Compression test analysis of CL and CL CS scaffolds. Data are mean +standard deviation (n = 3). t-test with Welch’s correction, *p < 0.05. (D) Live/dead staining of representative SF1 cells grown on CL and CL CS scaffolds. Scale bar: 200um. Z stack image (right) scale bar: 15um (blue stain collagen, green stain live cells, red staning dead cells).
FTIR was used to characterize scaffold composition with/without CS addition. FTIR spectral analysis for both CL and CL CS showed the amide I (1,700–1,600 cm−1), amide II (1600–1500 cm−1), and amide III (approximately 1,200–1,300 cm−1) peaks, which constitute the characteristic signature of collagen material (Figure 2B). In the CS CL, the peaks pattern changes between 1,000 and 1,085 cm2 may derived from the formation of bonding between afibrillar collagen and CS, specifically with the addition of a peak at approximately 1,058 cm2.
Scaffold mechanics were carried out using compressive tests. The results, summarized in Figure 2C, showed that higher force was required to compress CL CS (1.11 ± 0.22 N) compared to CL (0.22 ± 0.05 N).
Live/dead staining was performed on both SFMSCs and BMMSCs growing on CL and CL CS scaffolds with representative images shown in Figure 2D and Supplementary Figure S4A. Both cell types adhered to the 3D scaffolds (autofluorescence in blue channel) and showed positive staining for CalceinAM (live cell marker) demonstrating cellular adhesion to collagen fibers and viability on the 3D collagen sponges, both CL and CL CS.
As mentioned previously, CS addition on biomimetic scaffolds showed not only anti-inflammatory properties but also retained MSC immunosuppressive potential (Corradetti et al., 2016).
To assess the immune tuning capability of MSCs grown on 3D CL and CL CS compared to 2D culture, mRNA expression levels of key players in the suppression of chronic inflammation (ido1, ptges and ptgs2) were evaluated in SF-MSCs and BM-MSCs after 48 h of in vitro culture in 3D compared to 2D (Figure 3). Expression of ido1 was preferentially increased in patient SF1 and SF3 when cultured on CL CS (4,312-fold, p < 0.0001, and 7.9-fold, p < 0.0001, respectively) compared to 2D; while SF2 reported increase in ido1 expression on both CL (24-fold, p < 0.0001) and CL CS culture (16.2-fold, p < 0.0001; Figure 3A). Similar pattern was reported for ptges, showing a high increase of expression for SF1 growing on CL CS (1864-fold, p < 0.0001) and a comparable increase in both CL and CL CS condition for both SF2 (5.5-fold, p < 0.001, and 5.6-fold, p < 0.001, respectively) and SF3 (5.2-fold, p < 0.01, and 6.7-fold, p < 0.001, respectively; Figure 3B). Also, ptges2 expression levels showed a higher increase after culturing on CL CS compared to 2D for patient SF1 (1,525-fold, p < 0.0001) and a similar increase in both CL and CL CS for SF2 (7.6-fold, p < 0.001, and 12.9-fold, p < 0.001 respectively) and SF3 (82-fold, p < 0.01, and 84-fold, p < 0.001, respectively; Figure 3C). Instead, patient BM1 showed an increase in expression of only ptges2 when cultured on CL CS (38.6-fold, p < 0.0001) while BM2 reported an increase in the same gene expression preferentially on CL (132.7-fold, p < 0.0001) but lower on CL CS (45.1-fold, p < 0.0001) as well as lower ptges expression in both CL and CL CS (25.4-fold, p < 0.0001, and 28.9-fold, p < 0.0001 respectively; Supplementary Figure S4B).
FIGURE 3. Immunomodulatory analyses of Synovial fluid-derived mesenchymal stem cells (SF-MSCs): Relative expression of (A) ido1, (B) ptges and (C) ptgs2 after 48 h of culture on 2D and 3D CL (bare collagen) and 3D CL CS (collagen and chondroitin sulfate) scaffolds for SF1, SF2 and SF3. One-way ANOVA statistical test. Normalized on 2D. (**** = p < 0.0001; *** = p < 0.001; ** = p < 0.01).
To assess the immune tuning capability of MSCs under inflammatory stimulation, mRNA expression levels of ido1, ptges and ptgs2 was evaluated in both SF-MSCs and BM-MSCs grown on 3D CL and CL CS after 6-24-48-72 h of stimulation with TNFα and INFγ in vitro.
As previously published (Bauza et al., 2020), a concentration of 40 ng/ml TNFα plus INFγ is the optimal condition to evaluate immunosuppressive potential raised after inflammation in MSCs and it is associated with no significant cytotoxicity and moderate changes in MSC markers expression at 24, 48 and 72 h as demonstrated in Figure 1D.
When ido1 expression was analyzed on SF-MSCs, we observed significantly increased expression over time with a peak at 72 h for SF1 and SF2 (1,402.6-fold and 17,170.4-fold respectively, p < 0.0001) on CL scaffolds and a peak at 24 h and 72 h for SF1 and SF2 (22.7-fold and 62,380-fold respectively, p < 0.0001) on CL CS. Instead, SF3 showed a different pattern of expression with a peak at 48 h on CL (53,050.7-fold, p < 0.0001) and 24 h (4,161.5-fold, p < 0.0001) on CL CS with a decrement at 48 and 72 h. Culturing conditions for 2D showed a higher expression induction but different pattern of response with peaks of ido1 expression at 72 h and 48 h for SF1 and SF2, respectively (134,176.3-fold, p < 0.0001 and 142,755-fold, p < 0.0001), and a 24 h peak (6,914-fold, p < 0.0001) for SF3 (Figure 4A). Patient BM1 showed a peak of ido1 expression at 6 h followed by a decrease on both CL and CL CS (9,000-fold and 1,620-fold respectively, p < 0.0001) while BM2 reported a more constant increase in expression (72 h peak around 8,000-fold on CL and 2,900-fold on CL CS, p < 0.0001) while 2D response was following a similar pattern to the 3D for BM1 with a peak at 24 h (47,000-fold, p < 0.0001) while BM2 peak was recorded at 72 h (4,000-fold, p < 0.0001; Supplementary Figure S5A).
FIGURE 4. Immunomodulatory analyses of SF-MSCs under inflammatory conditions. (A) Relative expression of (A) ido1, (B) ptges and (C) ptgs2 after 24-48-72 h of treatment with 40 ng/ml TNFα and INFy for SF1, SF2, SF3. One-way ANOVA statistical test. Normalized on 0. (a = ****p < 0.0001; b = ***p < 0.001; c = **p < 0.01; d = *p < 0.05)
Ptges expression reported a higher peak of expression at 24 h (2.7-fold, p < 0.001) with a slow decrease until 72 h on CL for SF1 and no significant variations on CL CS, probably due to CS influence on ptges expression at time 0, reaching a plateau. Patient SF2 showed an increase of expression from 6 h with a peak at 72 h on CL (14.9-fold, p < 0.0001); instead, SF3 reported a peak at 48 h on CL (8.9-fold, p < 0.0001) and an increase in expression from 6 h to 24 h (24.5-fold, p < 0.0001) and then constant until 72 h for CL CS. Under 2D conditions, the ptges expression is delayed showing a peak at 72 h for SF1 and SF2 but at 24 h for SF3 (Figure 4B). Patient BM1 reported an increase in ptges marker expression mostly on 2D culture and a peak of expression only on CL (2.3-fold, p < 0.05) while BM2 showed peak of expression at 24 h on CL and CL CS (5.4-fold and 8.6-fold, respectively, p < 0.0001). 2D culturing conditions showed higher increase in both BM1 and BM2 with a peak at 24 h (4.2-fold, p < 0.0001) and 72 h (310-fold, p < 0.0001) respectively (Supplementary Figure S5B).
Finally, ptges2 expression reported a higher peak of expression at 6 h with a slow decrease until 72 h on both CL (6.1-fold, p < 0.0001) and CL CS (3.3-fold, p < 0.0001) culturing conditions for SF1 while expression was restored at 72 h for SF2. Patient SF3 reported a peak of expression after 6 h on CL (5.1-fold, p < 0.0001), and CL CS (8.5-fold, p < 0.0001), comparable to the other SF-MSCs (Figure 4C). Expression on 2D was delayed until 72 h for SF1 (319.3-fold, p < 0.0001) and SF2 (20.8-fold, p < 0.0001) while SF3 showed an increased expression from 6 h (28-fold, p < 0.0001). A similar pattern with a 6 h peak expression increase was recorded for BM1 (51-fold CL; 6-fold CL CS; 91-fold 2D, p < 0.0001) while BM2 cultured on 3D did not show any increase in expression but a delayed peak at 72 h on 2D (42-fold, p < 0.0001; Supplementary Figure S5C).
Additionally, to further investigate the immunomodulation of MSCs in 3D culture, IDO1 release in the supernatant was quantified after 48 h of TNFα and INFγ stimulation. Even if 2D cultured SFMSCs showed a higher release of IDO1 compared to BMMSCs; both SFMSCs and BMMSCs showed comparable IDO1 release in both CL and CL CS 3D (Supplementary Figure S6), supporting SFMSCs use as a valid alternative to gold standard BMMSCs and the pro-immunomodulatory effect related to 3D scaffold culturing conditions.
Osteoarthritis (OA), is by far the most common form of degenerative joint disease (Buckwalter and Martin 2006). Therapy is limited to activity modification and regular exercise, pain management, weight loss since no pharmaceutical or non-operative therapies have demonstrated unequivocal efficacy in preventing disease progression. In some cases, surgery can be suggested to strengthen, repair, or replace the affected joint (Hochberg et al., 2012). In focal articular cartilage lesions, surgical intervention can also be pursued by performing microfracture, osteochondral grafts, or chondrocyte implantation (Peterson et al., 2003; Bartlett et al., 2005; Henderson et al., 2005).
In the absence of effective strategies, to date, MSCs have been proposed as a promising biological therapeutic for degenerative conditions like OA (Sampson et al., 2015), thanks to their ability to differentiate into various cell lineages of musculoskeletal tissues (bone and cartilage) (Kolf et al., 2007) and their modulatory effect of the local inflammatory response (Glenn and Whartenby 2014). Since this cytotype was found in many different tissues, research main challenges are to define the optimal source for MSC derivation (Strioga et al., 2012). In addition to establish an optimize protocol for extraction and expansion of MSCs, testing 3D culturing conditions on scaffolds to potentiate regenerative and immunomodulatory features are critical challenges in the field. BM-MSCs were discovered first, and together with MSCs extracted from adipose tissue, were of primary interest for treating OA (Liu et al., 2007; Shafiee et al., 2011; Stockmann et al., 2012; Strioga et al., 2012). They are preferred over chondrocytes for multiple reasons: their clonal expansion ability is preserved for few several passages; culturing and expansion ex vivo is easier (Kolf et al., 2007), also because of their unique set of positive and negative surface markers expression which distinguished them from other cells, like hematopoietic and endothelial cells; MSCs can differentiate into all tissues within the joint, enabling restoration of lesions in the articular cartilage, ligaments, tendons and more complex osteochondral lesions (Glenn and Whartenby 2014). At the same time, MSCs have been proven to orchestrate the reparative response, acting on native cells and immune cells through paracrine signals (Glenn and Whartenby 2014). Following two different procedures, many ongoing clinical trials utilize autologous bone marrow- or adipose-derived MSCs for regeneration. First, autologous bone marrow concentrate or stromal vascular fraction can be harvested and transplanted same-day or expanded ex vivo expansion prior to transplantation (Michalek et al., 2015). Unfortunately, MSC dysfunctions have been documented in OA, osteoporosis and osteonecrosis (Hernigou and Beaujean 1997; Moerman et al., 2004; Lee et al., 2006) mostly impacting bone marrow-derived MSCs (Wyles et al., 2015). For this reason, different sources were explored in preclinical settings, resulting in growing interest in other locations such as synovium (Koga et al., 2007). To further potentiate the outcome of those therapies researchers evaluated the use of biocompatible MSC carriers (scaffolds) as vehicles for biological delivery (Bornes et al., 2014). To enhance efficient regeneration the scaffolds need to harbor specific properties: porous structure to allow cells migration but also support gas and nutrient permeability, being biocompatible and biodegradable, mechanical cue which resemble the joint while providing integrity to the tissue, inductivity and conductivity of osteochondral tissue (Nöth et al., 2008).
The two strategies to apply MSCs to therapy are either to potentiate their ability to promote regeneration or directly differentiate into lineage-specific cells and replace damaged tissue. In our research, we tested SF-MSCs’ potential as an alternative source of MSCs to bust regeneration processes through immunoregulation on 3D immunomodulating scaffolds. We demonstrated that after extraction, SF-MSCs’ retained anti-inflammatory, immune modulatory capacity in vitro. When compared directly to gold standard BM-MSC cell populations (Vega et al., 2015; Davatchi et al., 2016), SF-MSC cells showed similar morphological and proliferative capacity in 2D culture. Even if adult human MSC populations are commonly characterized by a unique set of positive and negative surface marker profile (CD34−, CD45−, CD73+, CD90+, and CD105+) which help distinguished them from hematopoietic and endothelial cells; different tissue source of extraction and correlating environment can determine specifically traits which serve to distinguish MSCs from one another (Kozlowska et al., 2019). Consequently, immunosuppressive potential, previously demonstrated for BM-MSCs (Gao et al., 2016), vary greatly depending on the specific tissue environment (Wang et al., 2014). Coupling those cells with a immunomodulatory 3D CL CS scaffold model to mimic the chondrogenic niche enhances our understanding of immune tuning capacity of the combination of MSCs population in an immunomodulatory environment in vitro (Ceredig 2013; Jacobs et al., 2013). Our data showed how SF-MSCs preferentially enhanced immunomodulatory capacity on CL CS scaffolds compared to BM-MSCs. This result correlates with literature reports, showing immunomodulatory and anti-inflammatory effects of CS (Akiyama et al., 2004; du Souich et al., 2009; Vallières and du Souich 2010). In line with previous reports demonstrating CS CL biomimetic scaffolds immunomodulatory and anti-inflammatory properties (Corradetti et al., 2017), culturing SF-MSCs on these platforms both induced an increase of master gene regulators in the suppression of chronic inflammation (ido1, ptges and ptgs2) at basal level compared to 2D and CL scaffolds, and sustained and promoted maintenance of anti-inflammatory gene expression under pro-inflammatory cytokines treatment such as IFN-γ and TNF-α. Ido1, ptges represent the key players in the suppression of inflammatory cascade during chronic inflammation (Donders R, et al., 2015; Moravej.et al., 2019). It was also previously demonstrated that ptges, controlled by ptgs2, triggers a PGE2-mediated TNFα down-regulation and IL10 up-regulation (Dennis and Norris 2015; Lee S, et al., 2015; Bauza G, et al., 2020). Moreover, ptgs2 is also responsible for the expression of several other specialized pro-resolving inflammatory mediators (such as PGI2) and it is commonly monitored in inflammatory research studies. Similarly, ido1, through INFγ response elements, inhibits innate and adaptive immunity and induces tolerance (Mellor and Munn, 2004; Puccetti and Grohmann 2007; Munn and Mellor, 2016).
On the other hand, direct comparison of 2D and 3D environment did not show further the expression of the immunomodulatory genes on 3D cultures in both SF-MSCs and BM-MSCs under inflammatory conditions compared to the untreated, suggesting that 3D culturing promoted an anti-inflammatory phenotype in MSCs at basal level which could serve as a powerful therapeutic tool for cell/scaffolds based regenerative therapy. Since this is the first attempt to describe SFMSCs immunomodulatory behavior on 3D pore scaffolds, at this moment we cannot claim which one can be better.
Further investigations and in vivo implantations will be fundamental to validate the beneficial role of combining SFMSCs with a CS based scaffold. Coupling the CS intrinsic stability, low immunogenicity and its important role in inflammation and immunity; with SFMSCs, easy and less invasive to harvest, could represent a new unexplored cell therapy for OA.
In conclusion, our work reported that 3D biomimetic scaffolds combined with SF-MSCs could become an interesting therapeutic strategy to be further explored, and a strategy to potentiate the immunomodulatory properties of the singular components. Indeed, the profoundly different expression pattern of immunosuppressive markers in the 2D versus the 3D environment further establish the need 3D tools to evaluate immunosuppressive potential before moving to clinical evaluation. Deeper preclinical investigation will be mandatory to establish SF-MSCs clinical and therapeutic strategy for OA, we believe that 3D biomimetic in vitro models are a powerful tool due to their immune tuning capacity and can help define acellular and cellular repair strategies to expand and improve current state of the art approaches.
The original contributions presented in the study are included in the article/Supplementary Materials, further inquiries can be directed to the corresponding author.
The studies involving human participants were reviewed and approved by Houston Methodist Hospital Orthopedics and Sports Medicine Department (IRB CR00006624). The patients/participants provided their written informed consent to participate in this study.
FP, PM, and FT conceived and designed the experiments; FP wrote the paper draft and interpreted the data; SL performed flow cytometry marker analysis under inflammatory conditions and PCR analysis assisted by MG; IR fabricated and characterized the scaffolds; MW performed Live/Dead staining and ELISA experiment; CV performed CFU experiment and cell extraction assisted by FT. PM performed the surgeries. PM and FT provided mentoring and contributed the funding support. All authors edited and approved the final version of the manuscript.
The project (19-048) was supported by a grant from the ON Foundation, Switzerland.
The authors would like to thank Jacob M. Kolman, MA, ISMPP CMPP™ (Academic Affairs, Houston Methodist Academic Institute) for scientific writing assistance in proofreading this manuscript.
The authors declare that the research was conducted in the absence of any commercial or financial relationships that could be construed as a potential conflict of interest.
All claims expressed in this article are solely those of the authors and do not necessarily represent those of their affiliated organizations, or those of the publisher, the editors and the reviewers. Any product that may be evaluated in this article, or claim that may be made by its manufacturer, is not guaranteed or endorsed by the publisher.
The Supplementary Material for this article can be found online at: https://www.frontiersin.org/articles/10.3389/fbiom.2022.989708/full#supplementary-material
ATR, attenuated total reflection; CL, bare collagen scaffold; CL CS, collagen and chondroitin sulfate; CS, chondroitin sulfate; FBS, fetal bovine serum; BM-MSC, bone marrow-derived mesenchymal stem cell; MSC, mesenchymal stem cell; OA, osteoarthritis; PBS, phosphate-buffered saline; PCR, polymerase chain reaction; SF-MSC, synovial fluid-derived mesenchymal stem cell.
Aggarwal, S., and Pittenger, M. F. (2005). Human mesenchymal stem cells modulate allogeneic immune cell responses. Blood 105 (4), 1815–1822. doi:10.1182/blood-2004-04-1559
Akiyama, H., Sakai, S., Linhardt, R. J., Goda, Y., Toida, T., and Maitani, T. (2004). Chondroitin sulphate structure affects its immunological activities on murine splenocytes sensitized with ovalbumin. Biochem. J. 382 (1), 269–278. doi:10.1042/bj20031851
Al-Najar, M., Khalil, H., Al-Ajlouni, J., Al-Antary, E., Hamdan, M., Rahmeh, R., et al. (2017). Intra-articular injection of expanded autologous bone marrow mesenchymal cells in moderate and severe knee osteoarthritis is safe: A phase I/II study. J. Orthop. Surg. Res. 12 (1), 190. doi:10.1186/s13018-017-0689-6
Alsaleh, G., Sparsa, L., Chatelus, E., Ehlinger, M., Gottenberg, J.-E., Wachsmann, D., et al. (2010). Innate immunity triggers IL-32 expression by fibroblast-like synoviocytes in rheumatoid arthritis. Arthritis Res. Ther. 12 (4), R135. doi:10.1186/ar3073
Ando, W., Kutcher, J. J., Krawetz, R., Sen, A., Nakamura, N., Frank, C. B., et al. (2014). Clonal analysis of synovial fluid stem cells to characterize and identify stable mesenchymal stromal cell/mesenchymal progenitor cell phenotypes in a porcine model: A cell source with enhanced commitment to the chondrogenic lineage. Cytotherapy 16 (6), 776–788. doi:10.1016/j.jcyt.2013.12.003
Bartlett, W., Skinner, J. A., Gooding, C. R., Carrington, R. W., Flanagan, A. M., Briggs, T. W., et al. (2005). Autologous chondrocyte implantation versus matrix-induced autologous chondrocyte implantation for osteochondral defects of the knee: A prospective, randomised study. J. Bone Jt. Surg. Br. volume 87 (5), 640–645. doi:10.1302/0301-620x.87b5.15905
Bauza, G., Pasto, A., McCulloch, P., Lintner, D., Brozovich, A., Niclot, F. B., et al. (2020). Improving the immunosuppressive potential of articular chondroprogenitors in a three-dimensional culture setting. Sci. Rep. 10 (1), 16610. doi:10.1038/s41598-020-73188-9
Bernardo, M. E., and Fibbe, W. E. (2013). Mesenchymal stromal cells: Sensors and switchers of inflammation. Cell Stem Cell 13 (4), 392–402. doi:10.1016/j.stem.2013.09.006
Bornes, T. D., Adesida, A. B., and Jomha, N. M. (2014). Mesenchymal stem cells in the treatment of traumatic articular cartilage defects: A comprehensive review. Arthritis Res. Ther. 16 (5), 432. doi:10.1186/s13075-014-0432-1
Buckwalter, J. A., and Martin, J. A. (2006). Osteoarthritis. Adv. Drug Deliv. Rev. 58 (2), 150–167. doi:10.1016/j.addr.2006.01.006
Ceredig, R. (2013). A look at the interface between mesenchymal stromal cells and the immune system. Immunol. Cell Biol. 91 (1), 3–4. doi:10.1038/icb.2012.68
Chen, Y., Shao, J. Z., Xiang, L. X., Dong, X. J., and Zhang, G. R. (2008). Mesenchymal stem cells: A promising candidate in regenerative medicine. Int. J. Biochem. Cell Biol. 40 (5), 815–820. doi:10.1016/j.biocel.2008.01.007
Corradetti, B., Taraballi, F., Corbo, C., Cabrera, F., Pandolfi, L., Minardi, S., et al. (2017). Immune tuning scaffold for the local induction of a pro-regenerative environment. Sci. Rep. 7 (1), 17030. doi:10.1038/s41598-017-16895-0
Corradetti, B., Taraballi, F., Minardi, S., Van Eps, J., Cabrera, F., Francis, L. W., et al. (2016). Chondroitin sulfate immobilized on a biomimetic scaffold modulates inflammation while driving chondrogenesis. Stem Cells Transl. Med. 5 (5), 670–682. doi:10.5966/sctm.2015-0233
Davatchi, F., Abdollahi, B. S., Mohyeddin, M., Shahram, F., and Nikbin, B. (2011). Mesenchymal stem cell therapy for knee osteoarthritis. Preliminary report of four patients. Int. J. Rheum. Dis. 14 (2), 211–215. doi:10.1111/j.1756-185x.2011.01599.x
Davatchi, F., Sadeghi Abdollahi, B., Mohyeddin, M., and Nikbin, B. (2016). Mesenchymal stem cell therapy for knee osteoarthritis: 5 years follow-up of three patients. Int. J. Rheum. Dis. 19 (3), 219–225. doi:10.1111/1756-185x.12670
Dennis, E. A., and Norris, P. C. (2015). Eicosanoid storm in infection and inflammation. Nat. Rev. Immunol. 15, 511–523. doi:10.1038/nri3859
Donders, R., Vanheusden, M., Bogie, J. F. J., Ravanidis, S., Thewissen, K., Stinissen, P., et al. (2015). Human Wharton's jelly-derived stem cells display immunomodulatory properties and transiently improve rat experimental autoimmune encephalomyelitis. Cell Transpl. 24, 2077–2098. doi:10.3727/096368914X685104
du Souich, P., García, A. G., Vergés, J., and Montell, E. (2009). Immunomodulatory and anti-inflammatory effects of chondroitin sulphate. J. Cell. Mol. Med. 13 (8), 1451–1463. doi:10.1111/j.1582-4934.2009.00826.x
Evans, J., and Fiederlein, A. (2020). Modeling osteoarthritis using three-dimensional culture. Am. J. Biomed. Res. 8, 72–74. doi:10.12691/ajbr-8-3-3
Gao, F., Chiu, S. M., Motan, D. A., Zhang, Z., Chen, L., Ji, H. L., et al. (2016). Mesenchymal stem cells and immunomodulation: Current status and future prospects. Cell Death Dis. 7 (1), e2062. doi:10.1038/cddis.2015.327
Garcia, J., Wright, K., Roberts, S., Kuiper, J. H., Mangham, C., Richardson, J., et al. (2016). Characterisation of synovial fluid and infrapatellar fat pad derived mesenchymal stromal cells: The influence of tissue source and inflammatory stimulus. Sci. Rep. 6 (1), 24295. doi:10.1038/srep24295
Glenn, J. D., and Whartenby, K. A. (2014). Mesenchymal stem cells: Emerging mechanisms of immunomodulation and therapy. World J. Stem Cells 6 (5), 526–539. doi:10.4252/wjsc.v6.i5.526
Greene, M. A., and Loeser, R. F. (2015). Aging-related inflammation in osteoarthritis. Osteoarthr. Cartil. 23 (11), 1966–1971. doi:10.1016/j.joca.2015.01.008
Han, Y., Li, X., Zhang, Y., Han, Y., Chang, F., and Ding, J. (2019). Mesenchymal stem cells for regenerative medicine. Cells 8 (8), 886. doi:10.3390/cells8080886
Hardingham, T. (2008). Extracellular matrix and pathogenic mechanisms in osteoarthritis. Curr. Rheumatol. Rep. 10 (1), 30–36. doi:10.1007/s11926-008-0006-9
Henderson, I., Francisco, R., Oakes, B., and Cameron, J. (2005). Autologous chondrocyte implantation for treatment of focal chondral defects of the knee--a clinical, arthroscopic, MRI and histologic evaluation at 2 years. Knee 12 (3), 209–216. doi:10.1016/j.knee.2004.07.002
Hernigou, P., and Beaujean, F. (1997). Abnormalities in the bone marrow of the iliac crest in patients who have osteonecrosis secondary to corticosteroid therapy or alcohol abuse. J. Bone Jt. Surg. 79 (7), 1047–1053. doi:10.2106/00004623-199707000-00011
Hochberg, M. C., Altman, R. D., April, K. T., Benkhalti, M., Guyatt, G., McGowan, J., et al. (2012). American College of Rheumatology 2012 recommendations for the use of nonpharmacologic and pharmacologic therapies in osteoarthritis of the hand, hip, and knee. Arthritis Care Res. Hob. 64 (4), 465–474. doi:10.1002/acr.21596
Huang, K., Li, Q., Li, Y., Yao, Z., Luo, D., Rao, P., et al. (2018). Cartilage tissue regeneration: The roles of cells, stimulating factors and scaffolds. Curr. Stem Cell Res. Ther. 13 (7), 547–567. doi:10.2174/1574888x12666170608080722
Hunter, D. J., and Bierma-Zeinstra, S. (2019). Osteoarthritis. Lancet 393 (10182), 1745–1759. doi:10.1016/s0140-6736(19)30417-9
Jacobs, S. A., Roobrouck, V. D., Verfaillie, C. M., and Van Gool, S. W. (2013). Immunological characteristics of human mesenchymal stem cells and multipotent adult progenitor cells. Immunol. Cell Biol. 91 (1), 32–39. doi:10.1038/icb.2012.64
Jiang, Y. Z., Zhang, S. F., Qi, Y. Y., Wang, L. L., and Ouyang, H. W. (2011). Cell transplantation for articular cartilage defects: Principles of past, present, and future practice. Cell Transpl. 20 (5), 593–607. doi:10.3727/096368910x532738
Jones, E. A., Crawford, A., English, A., Henshaw, K., Mundy, J., Corscadden, D., et al. (2008). Synovial fluid mesenchymal stem cells in health and early osteoarthritis: Detection and functional evaluation at the single-cell level. Arthritis Rheum. 58 (6), 1731–1740. doi:10.1002/art.23485
Jorgenson, K. D., Hart, D. A., Krawetz, R., and Sen, A. (2018). Production of adult human synovial fluid-derived mesenchymal stem cells in stirred-suspension culture. Stem cells Int. 2018, 1–16. doi:10.1155/2018/8431053
Khurshid, M., Mulet-Sierra, A., Adesida, A., and Sen, A. (2018). Osteoarthritic human chondrocytes proliferate in 3D co-culture with mesenchymal stem cells in suspension bioreactors. J. Tissue Eng. Regen. Med. 12 (3), e1418–e1432. doi:10.1002/term.2531
Koga, H., Muneta, T., Ju, Y. J., Nagase, T., Nimura, A., Mochizuki, T., et al. (2007). Synovial stem cells are regionally specified according to local microenvironments after implantation for cartilage regeneration. Stem Cells 25 (3), 689–696. doi:10.1634/stemcells.2006-0281
Kolf, C. M., Cho, E., and Tuan, R. S. (2007). Mesenchymal stromal cells. Biology of adult mesenchymal stem cells: Regulation of niche, self-renewal and differentiation. Arthritis Res. Ther. 9 (1), 204. doi:10.1186/ar2116
Koyama, N., Okubo, Y., Nakao, K., Osawa, K., Fujimura, K., and Bessho, K. (2011). Pluripotency of mesenchymal cells derived from synovial fluid in patients with temporomandibular joint disorder. Life Sci. 89 (19-20), 741–747. doi:10.1016/j.lfs.2011.09.005
Kozlowska, U., Krawczenko, A., Futoma, K., Jurek, T., Rorat, M., Patrzalek, D., et al. (2019). Similarities and differences between mesenchymal stem/progenitor cells derived from various human tissues. World J. Stem Cells 11 (6), 347–374. doi:10.4252/wjsc.v11.i6.347
Lee, D. H., Sonn, C. H., Han, S. B., Oh, Y., Lee, K. M., and Lee, S. H. (2012). Synovial fluid CD34− CD44+ CD90+ mesenchymal stem cell levels are associated with the severity of primary knee osteoarthritis. Osteoarthr. Cartil. 20 (2), 106–109. doi:10.1016/j.joca.2011.11.010
Lee, J. S., Lee, J. S., Roh, H. L., Kim, C. H., Jung, J. S., and Suh, K. T. (2006). Alterations in the differentiation ability of mesenchymal stem cells in patients with nontraumatic osteonecrosis of the femoral head: Comparative analysis according to the risk factor. J. Orthop. Res. 24 (4), 604–609. doi:10.1002/jor.20078
Lee, S., Kim, H. S., Roh, K. H., Lee, B. C., Shin, T. H., Yoo, J. M., et al. (2015). DNA methyltransferase inhibition accelerates the immunomodulation and migration of human mesenchymal stem cells. Sci. Rep. 5, 8020. doi:10.1038/srep08020
Lieberthal, J., Sambamurthy, N., and Scanzello, C. R. (2015). Inflammation in joint injury and post-traumatic osteoarthritis. Osteoarthr. Cartil. 23 (11), 1825–1834. doi:10.1016/j.joca.2015.08.015
Liu, T. M., Martina, M., Hutmacher, D. W., Hui, J. H., Lee, E. H., and Lim, B. (2007). Identification of common pathways mediating differentiation of bone marrow- and adipose tissue-derived human mesenchymal stem cells into three mesenchymal lineages. Stem Cells 25 (3), 750–760. doi:10.1634/stemcells.2006-0394
Martel-Pelletier, J., Boileau, C., Pelletier, J. P., and Roughley, P. J. (2008). Cartilage in normal and osteoarthritis conditions. Best. Pract. Res. Clin. Rheumatol. 22 (2), 351–384. doi:10.1016/j.berh.2008.02.001
Mellor, A. L., and Munn, D. H. (2004). Ido expression by dendritic cells: Tolerance and tryptophan catabolism. Nat. Rev. Immunol. 4, 762–774. doi:10.1038/nri1457
Michalek, J., Moster, R., Lukac, L., Proefrock, K., Petrasovic, M., Rybar, J., et al. (2015). Autologous adipose tissue-derived stromal vascular fraction cells application in patients with osteoarthritis. Cell Transpl. 20, 1–36. doi:10.3727/096368915X686760
Moerman, E. J., Teng, K., Lipschitz, D. A., and Lecka-Czernik, B. (2004). Aging activates adipogenic and suppresses osteogenic programs in mesenchymal marrow stroma/stem cells: The role of PPAR-γ2 transcription factor and TGF-β/BMP signaling pathways. Aging Cell 3 (6), 379–389. doi:10.1111/j.1474-9728.2004.00127.x
Monfort, J., Martel-Pelletier, J., and Pelletier, J. P. (2008a). Chondroitin sulphate for symptomatic osteoarthritis: Critical appraisal of meta-analyses. Curr. Med. Res. Opin. 24 (5), 1303–1308. doi:10.1185/030079908x297231
Monfort, J., Pelletier, J. P., Garcia-Giralt, N., and Martel-Pelletier, J. (2008b). Biochemical basis of the effect of chondroitin sulphate on osteoarthritis articular tissues. Ann. Rheum. Dis. 67 (6), 735–740. doi:10.1136/ard.2006.068882
Moravej, A., Kouhpayeh, A., Geramizadeh, B., Azarpira, N., Yaghobi, R., Mansoori, Y., et al. (2019). The effect of mesenchymal stem cells on the expression of ido and Qa2 molecules in dendritic cells. Adv. Pharm. Bull. 9, 56–63. doi:10.15171/apb.2019.007
Morito, T., Muneta, T., Hara, K., Ju, Y. J., Mochizuki, T., Makino, H., et al. (2008). Synovial fluid-derived mesenchymal stem cells increase after intra-articular ligament injury in humans. Rheumatol. Oxf. 47 (8), 1137–1143. doi:10.1093/rheumatology/ken114
Munn, D. H., and Mellor, A. L. (2016). Ido in the tumor microenvironment: Inflammation, counter-regulation, and tolerance. Trends Immunol. 37, 193–207. doi:10.1016/j.it.2016.01.002
Nöth, U., Steinert, A. F., and Tuan, R. S. (2008). Technology insight: Adult mesenchymal stem cells for osteoarthritis therapy. Nat. Clin. Pract. Rheumatol. 4 (7), 371–380. doi:10.1038/ncprheum0816
Orozco, L., Munar, A., Soler, R., Alberca, M., Soler, F., Huguet, M., et al. (2013). Treatment of knee osteoarthritis with autologous mesenchymal stem cells: A pilot study. Transplantation 95 (12), 1535–1541. doi:10.1097/tp.0b013e318291a2da
Paradiso, F., Quintela, M., Lenna, S., Serpelloni, S., James, D., Caserta, S., et al. (2022). Studying activated fibroblast phenotypes and fibrosis-linked mechanosensing using 3D biomimetic models. Macromol. Biosci. 22, 2100450. doi:10.1002/mabi.202100450
Peterson, L., Minas, T., Brittberg, M., and Lindahl, A. (2003). Treatment of osteochondritis dissecans of the knee with autologous chondrocyte transplantation: Results at two to ten years. J. Bone Jt. Surgery-American Volume 85-A (2), 17–24. doi:10.2106/00004623-200300002-00003
Puccetti, P., and Grohmann, U. J. (2007). Ido and regulatory T cells: A role for reverse signalling and non-canonical NF-κB activation. Nat. Rev. Immunol. 7, 817–823. doi:10.1038/nri2163
Ren, G., Chen, X., Dong, F., Li, W., Ren, X., Zhang, Y., et al. (2012). Concise review: Mesenchymal stem cells and translational medicine: Emerging issues. Stem cells Transl. Med. 1 (1), 51–58. doi:10.5966/sctm.2011-0019
Sampson, S., Botto-van Bemden, A., and Aufiero, D. (2015). Stem cell therapies for treatment of cartilage and bone disorders: Osteoarthritis, avascular necrosis, and non-union fractures. PM&R. 7 (4), S26–s32. doi:10.1016/j.pmrj.2015.01.023
Samvelyan, H. J., Hughes, D., Stevens, C., and Staines, K. A. (2021). Models of osteoarthritis: Relevance and new insights. Calcif. Tissue Int. 109 (3), 243–256. doi:10.1007/s00223-020-00670-x
Santos, V. H., Pfeifer, J. P. H., de Souza, J. B., Milani, B. H. G., de Oliveira, R. A., Assis, M. G., et al. (2018). Culture of mesenchymal stem cells derived from equine synovial membrane in alginate hydrogel microcapsules. BMC Vet. Res. 14 (1), 114. doi:10.1186/s12917-018-1425-0
Schroder, K., Hertzog, P. J., Ravasi, T., and Hume, D. A. (2004). Interferon-γ: An overview of signals, mechanisms and functions. J. Leukoc. Biol. 75 (2), 163–189. doi:10.1189/jlb.0603252
Shafiee, A., Seyedjafari, E., Soleimani, M., Ahmadbeigi, N., Dinarvand, P., and Ghaemi, N. (2011). A comparison between osteogenic differentiation of human unrestricted somatic stem cells and mesenchymal stem cells from bone marrow and adipose tissue. Biotechnol. Lett. 33 (6), 1257–1264. doi:10.1007/s10529-011-0541-8
Soler, R., Orozco, L., Munar, A., Huguet, M., Lopez, R., Vives, J., et al. (2016). Final results of a phase I–II trial using ex vivo expanded autologous Mesenchymal Stromal Cells for the treatment of osteoarthritis of the knee confirming safety and suggesting cartilage regeneration. Knee 23 (4), 647–654. doi:10.1016/j.knee.2015.08.013
Stockmann, P., Park, J., von Wilmowsky, C., Nkenke, E., Felszeghy, E., Dehner, J. F., et al. (2012). Guided bone regeneration in pig calvarial bone defects using autologous mesenchymal stem/progenitor cells - a comparison of different tissue sources. J. Cranio-Maxillofacial Surg. 40 (4), 310–320. doi:10.1016/j.jcms.2011.05.004
Strioga, M., Viswanathan, S., Darinskas, A., Slaby, O., and Michalek, J. (2012). Same or not the same? Comparison of adipose tissue-derived versus bone marrow-derived mesenchymal stem and stromal cells. Stem Cells Dev. 21 (14), 2724–2752. doi:10.1089/scd.2011.0722
Taraballi, F., Corradetti, B., Minardi, S., Powel, S., Cabrera, F., Van Eps, J. L., et al. (2016). Biomimetic collagenous scaffold to tune inflammation by targeting macrophages. J. Tissue Eng. 7, 204173141562466. doi:10.1177/2041731415624667
Uccelli, A., Moretta, L., and Pistoia, V. (2008). Mesenchymal stem cells in health and disease. Nat. Rev. Immunol. 8 (9), 726–736. doi:10.1038/nri2395
Vallières, M., and du Souich, P. (2010). Modulation of inflammation by chondroitin sulfate. Osteoarthr. Cartil. 18 (1), S1–S6. doi:10.1016/j.joca.2010.02.017
Vega, A., Martín-Ferrero, M. A., Del Canto, F., Alberca, M., García, V., Munar, A., et al. (2015). Treatment of knee osteoarthritis with allogeneic bone marrow mesenchymal stem cells: A randomized controlled trial. Transplantation 99 (8), 1681–1690. doi:10.1097/tp.0000000000000678
Volpi, N. (2011). Anti-inflammatory activity of chondroitin sulphate: New functions from an old natural macromolecule. Inflammopharmacology 19 (6), 299–306. doi:10.1007/s10787-011-0098-0
Wang, Y., Chen, X., Cao, W., and Shi, Y. (2014). Plasticity of mesenchymal stem cells in immunomodulation: Pathological and therapeutic implications. Nat. Immunol. 15 (11), 1009–1016. doi:10.1038/ni.3002
Wyles, C. C., Houdek, M. T., Crespo-Diaz, R. J., Norambuena, G. A., Stalboerger, P. G., Terzic, A., et al. (2015). Adipose-derived mesenchymal stem cells are phenotypically superior for regeneration in the setting of osteonecrosis of the femoral head. Clin. Orthop. Relat. Res. 473 (10), 3080–3090. doi:10.1007/s11999-015-4385-8
Xia, B., Di, C., Zhang, J., Hu, S., Jin, H., and Tong, P. (2014). Osteoarthritis pathogenesis: A review of molecular mechanisms. Calcif. Tissue Int. 95 (6), 495–505. doi:10.1007/s00223-014-9917-9
Zayed, M., Adair, S., and Dhar, M. (2021). Effects of normal synovial fluid and interferon gamma on chondrogenic capability and immunomodulatory potential respectively on equine mesenchymal stem cells. Int. J. Mol. Sci. 22 (12), 6391. doi:10.3390/ijms22126391
Keywords: MSCs, synovial, osteoarthritis, 3D model, biomaterials
Citation: Paradiso F, Lenna S, Isbell R, Garcia Garza MF, Williams M, Varner C, Mcculloch P and Taraballi F (2022) Immunosuppressive potential evaluation of synovial fluid mesenchymal stem cells grown on 3D scaffolds as an alternative source of MSCs for osteoarthritis cartilage studies. Front. Front. Biomater. Sci. 1:989708. doi: 10.3389/fbiom.2022.989708
Received: 08 July 2022; Accepted: 25 October 2022;
Published: 08 December 2022.
Edited by:
David B. Berry, University of California, San Diego, United StatesReviewed by:
Marley Dewey, University of Pittsburgh, United StatesCopyright © 2022 Paradiso, Lenna, Isbell, Garcia Garza, Williams, Varner, Mcculloch and Taraballi. This is an open-access article distributed under the terms of the Creative Commons Attribution License (CC BY). The use, distribution or reproduction in other forums is permitted, provided the original author(s) and the copyright owner(s) are credited and that the original publication in this journal is cited, in accordance with accepted academic practice. No use, distribution or reproduction is permitted which does not comply with these terms.
*Correspondence: Francesca Taraballi, ZnRhcmFiYWxsaTJAaG91c3Rvbm1ldGhvZGlzdC5vcmc=
Disclaimer: All claims expressed in this article are solely those of the authors and do not necessarily represent those of their affiliated organizations, or those of the publisher, the editors and the reviewers. Any product that may be evaluated in this article or claim that may be made by its manufacturer is not guaranteed or endorsed by the publisher.
Research integrity at Frontiers
Learn more about the work of our research integrity team to safeguard the quality of each article we publish.