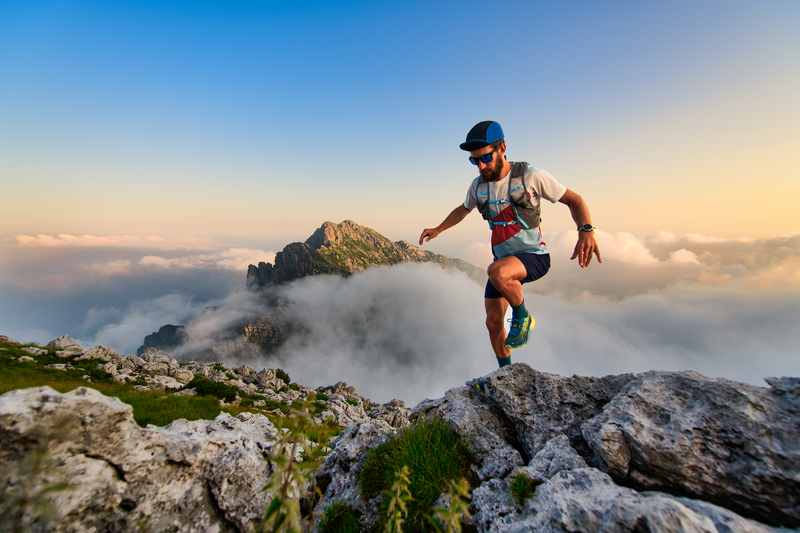
95% of researchers rate our articles as excellent or good
Learn more about the work of our research integrity team to safeguard the quality of each article we publish.
Find out more
MINI REVIEW article
Front. Biomater. Sci. , 19 July 2022
Sec. Biomaterials Science for Regenerative Therapies
Volume 1 - 2022 | https://doi.org/10.3389/fbiom.2022.953837
Tissue engineering, which involves the use of therapeutic biologicals supported by implantable materials, represents a promising tool to repair damaged tissues or organs. Among the most proper supporting materials and scaffolds, natural extracellular matrix (ECM) constitutes a dynamic platform of structural and functional fibers and biomolecules that confers a suitable microenvironment for cell attachment, proliferation and differentiation via activation of host signaling cues. In this context, ECM derived from human pericardium emerges as a supportive porous biomaterial to regenerate post-infarcted myocardium. In specific, pericardial ECM highlights as a potential clinical option for administering those active components grown and purified from large-scale cell cultures, such as mesenchymal stromal cells and derived extracellular vesicles, and to locally generate a vascularized bioactive niche promoting modulation of post-ischemic inflammation and cardiac repair.
Myocardial ischemia, which is caused by coronary artery occlusion, leads to severe blood supply reduction and necrosis of cardiac tissue. Current treatments mainly focus on restoration of blood flow, increasing patients’ life quality and expectancy, but do not address the complete recovery of the scarred tissue. Alternatively, cardiac tissue engineering (TE) has emerged as an advanced therapeutic option with engineering innovations that hold promise for clinical use (Castells-Sala et al., 2013). To that end, efforts have been made to develop scaffolding materials that mimic the native three-dimensional (3-D) cardiac tissue architecture and promote a viable microenvironment for regenerative cell attachment, proliferation, and differentiation (Badylak et al., 2011; Castells-Sala and Semino, 2012). In general, the ideal TE material should be biodegradable and biocompatible, allowing tissue revascularization and triggering low inflammatory response within the tissues to be substituted or repaired (Loh and Choong 2013; Grigorian Shamagian et al., 2019). Moreover, cardiac grafts are needed to empower electrical and mechanical coupling with remaining host tissue increasing cardiac function output following myocardial infarction (MI) (Vunjak-Novakovic et al., 2010).
Inner body membranes, which comprise thin sheets or layers of cells covering the surface of internal organs, the outside of the body and lines various body cavities, are good candidates to be used as versatile tools in TE applications. In particular, these membranes are classified into two main categories which are epithelial membranes (amniotic membrane, mesentery, omentum, pericardium, peritoneum, and pleura) and connective tissue membranes (fascia, periosteum, and synovial membrane). In comparison with synthetic materials, these biological membranes have better biocompatibility, less cytotoxicity and induce lower inflammatory reactions (Inci et al., 2020). They also have unquestionable advantages such as their natural hemodynamic resistance, absence of anticoagulant therapy and reduction of the risk of thromboembolic complications and anticoagulant-related hemorrhage (Grebenik et al., 2020). In this context, pericardium is a conical double-walled sac of fibrous tissue that surrounds the heart and the roots of the great blood vessels. The pericardial membrane is composed of an epithelium and connective tissue rich in collagen, glycoproteins and glycosaminoglycans, which mechanically stabilizes and protects the heart, and maintains cardiac geometry and pressure-volume relationships of the cardiac chambers (Rodriguez and Tan 2017). Pericardium can be used as a good scaffolding material providing a 3-D structure and promoting a viable microenvironmentardial in cardiac tissue engineering through different approaches (Figure 1). As previously described in bovine pericardium, human pericardium exhibits anisotropy of its surface ECM niches, with the serous layer (i.e., parietal pericardium) containing mostly basement membrane components (e.g., laminin, LAM) and the fibrous layer (i.e., mediastinal surface) being composed primarily of type I collagen (Col I) (Xing et al., 2021) (Figure 1).
FIGURE 1. Schematic representation of decellularized pericardium approaches in cardiac tissue engineering. The flowchart resumes from the pericardium retrieval, following decellularization to obtain the scaffold, and its combination with therapeutic biologicals for surgical implantation covering the myocardial scar. Representative confocal microscopy images of basement membrane laminin (LAM) and collagen I (Col I) stainings in native and decellularized pericardium are also presented. Nuclear counterstaining with DAPI revealed that decellularized ECM are completely acellular. LAM expression analysis demonstrates retention of native tissue LAM content and organization only on the serous side of the decellularized pericardial ECM. Scale bars = 50 µm. The images included in this figure are original or unpublished and thus it can be reproduced with no permission. Some of the illustrations have been obtained from smart.servier.com.
Clinically, pericardial tissue from both human and xenogeneic origins has widely been used in the context of both cardiac and non-cardiac surgeries. In brief, pericardium grafts have been applied to correct intracardiac and diaphragm defects (Ricci et al., 2014; Yaliniz et al., 2014; Ascaso et al., 2021), as well as ischemic ventricular septal defect after acute MI (Mihalj et al., 2022). On the other hand, non-cardiac pericardium-based applications include replacement of brain dura mater (Sun et al., 2018), bleb repair, conjunctival reconstruction, cover of severe corneal wound or tendon elongation in the eye (Niegowski et al., 2020; Ashena et al., 2021; Hedergott et al., 2021; Chen et al., 2022), odontology (Solakoğlu et al., 2022) and eardrum reconstruction (de Dorldodot et al., 2013; Sainsbury et al., 2022). Furthermore it has been employed to generate a variety of bioprostheses such as vascular grafts, patches for abdominal or vaginal wall reconstruction and heart valves (Rémi et al., 2010).
Beyond these current clinical options, decellularization has been revealed as a procedure to obtain less immunoreactive and highly biocompatible pericardial ECM for TE purposes. Here, we focus on the use of human decellularized pericardial ECM to generate supportive porous scaffold for administering bioactive components grown and purified from large-scale cell cultures such as mesenchymal stromal cells (MSC) and derived extracellular vesicles (EV). The specific requirements governing the processing of pericardium as starting material and the regulatory oversight for their potential clinical application post MI are also highlighted.
Decellularization of native tissues consists in the elimination of the original cellular material offering an available source of biocompatible ECM allografts. Of note, its efficacy is mainly based on DNA and cell remnants removal, overlooking the preservation of structural, mechanical and biological properties of the native ECM. The resulting 3-D porous scaffolds are potentially capable of reducing the probability of exacerbated immunological response and Graft-Versus-Host Disease, and graft rejection once implanted. Commonly, cellular components removal can be achieved by the combination of physical, chemical and biological methods (Gilbert et al., 2006; Badylak et al., 2011; Crapo et al., 2011). In this sense, the optimal decellularization protocol does not only depend on the tissue itself (cellularity, density, lipid contents, and thickness), but also on the final application of the resulting ECM-based graft. To date, different approaches have been developed by using pericardium from bovine, porcine and human origins (reviewed in Inci et al., 2020; Grebenik et al., 2020). Interestingly, human decellularized pericardium has demonstrated a reduced reactive response and immunological rejection both in vitro and in vivo (Prat-Vidal and Bayes-Genis 2020). Moreover, it has been described the neoformation of blood vessels and nerves in cell-free pericardial scaffolds applied over infarcted tissue as well as significant improvement in cardiac function parameters and decrease in infarct size in pigs (Gálvez-Montón et al., 2015).
In addition, one of the main challenges in cardiac TE is to find an optimal biomaterial to serve as a scaffold for the delivery of bioactive components. Specifically, pore integrity and size, stiffness and elasticity are crucial to yield a scaffolding biomaterial with the optimal characteristics before being filled with the active ingredient (Loh and Choong 2013). The main goal is to facilitate the retention and local delivery with the aim of increasing the action of therapeutic biologicals, such as cells or EV. In this area of study, different pre-clinical studies based on the combination of pericardial ECM scaffolds and cells have been developed for the treatment of MI. Decellularized scaffolds, which are completely composed of ECM, constitute the natural environment of cells in the body, thus supporting basic cell functions including migration, proliferation, and differentiation (Inci et al., 2020). Importantly, the specific composition, 3-D structure and microenvironment of scaffold affect the survival and growth of the in vitro reseeded cells, together with a successful integration of the biomaterial into the host tissue (Grayson et al., 2009; Sarig and Machluf, 2011). In this line, it has been reported that the preserved mechanical and structural properties of porous pericardial ECM following decellularization favors cell infiltration, retention and survival (Perea-Gil et al., 2018), thus making it an optimal scaffold for the manufacturing of reparative constructs (Prat-Vidal and Bayes-Genis 2020; Prat-Vidal et al., 2020). For instance, 3-D macroporous human pericardial scaffolds with well-defined architecture and interconnected pores enabled human Sca-1+ cardiac progenitor cells to migrate, survive, proliferate and differentiate at higher rates compared with collagen scaffolds (Rajabi-Zeleti et al., 2014). Moreover, histological examination of subcutaneous transplanted scaffolds after 1 month revealed low immunological response, enhanced angiogenesis and cardiac muscle repair in rats treated with pericardial scaffolds instead of grafts based in collagen (Rajabi-Zeleti et al., 2014). Allogeneic porcine induced pluripotent stem cells have also been delivered in decellularized pericardial ECM into pigs with experimentally induced MI, although this bioengineered construct was not effective in restoring cardiac function (Gálvez-Montón et al., 2017). It is also important to point out that the use of MSC in combination with pericardium, probably exerts a paracrine action of scaffold-retained cells while synthesizing new endogenous ECM which, in turn, increases the mobilization of resident precursor cells and ultimately the promotion of cardiac repair. MSC are self-renewing, ex vivo culture-expandable stem cell populations, with multipotent differentiation capacity and marked immune modulation potential, thus, considered immune privileged (Thompson et al., 2020). Indeed, in the pre-clinical setting, decellularized pericardial ECM has shown to be an optimal supportive scaffold for the delivery of cardiac adipose tissue-derived MSC to regenerate infarcted myocardium (Prat-Vidal et al., 2014; Gálvez-Montón et al., 2015; Gálvez-Montón et al., 2017; Perea-Gil et al., 2018). Cell-embedded pericardial ECM scaffolds limited the sequelae associated to MI, particularly reducing infarct size and improving cardiac function after implantation in post-infarcted pigs (Gálvez-Montón et al., 2017; Perea-Gil et al., 2018).
On the other hand, EV are the subject of growing research because they modulate efficient intercellular communication by transferring a variety of bioactive molecules such as proteins, lipids and non-coding nucleic acids (Yáñez-Mó et al., 2015). Thus, EV induce similar effects as the parental cells. For that, the use of EV for cardiac repair following acute MI has already begun to be explored pre-clinically. For example, the benefits of a newly-developed cardiac tissue engineering bioimplant combining EV derived from MSC with pericardial ECM has already been confirmed in infarcted pigs (Monguio-Tortajada et al., 2021). In particular, this therapeutic strategy was designed to use human decellularized pericardium refilled with EV obtained from porcine cardiac adipose tissue-derived MSC in order to guarantee local and sustained EV release over the infarcted area. As a result, the bioimplant was efficiently integrated into the post-infarcted myocardium after implantation, promoting new vessel formation and decreasing tissue inflammation. EV were also detected into the infarcted myocardial area reducing tissue fibrosis and macrophage infiltration (Monguio-Tortajada et al., 2021). Indeed, the use of ECM comprising EV instead of the parental cells could have advantages, including the fact that EV are neither viable nor replicative components and its manipulation do not require as many precautions as with living cells. Also, due to EV size ranging from 50 to 200 nm in diameter, EV could reach more target sites with no thromboembolic complications once administered in vivo (Moll, 2022). Lastly, EV could be more powerful effectors over time because they constitute lipid bilayer-protected packages of multiple bioactive molecules.
Human decellularized pericardial ECM can also be processed into an injectable hydrogel and administered alone or in conjunction with active components to self-assemble in situ for cardiac repair. To date, although manufacturing standards are not well established for injectable types of ECM, ECM-derived hydrogels have been also studied due to their capability of being delivered in a minimally invasive manner in vivo (Hernández et al., 2020). For example, pericardium hydrogel injected in the left ventricular-free wall of a rat model, has been demonstrated to self-assemble shortly after injection. Moreover, it also provides a template for cell infiltration and neovascularization into damaged myocardium (Seif-Naraghi et al., 2010). Furthermore, the use of porcine decellularized pericardial hydrogel has not been associated with adverse inflammatory processes (Seif-Naraghi et al., 2012). In accordance, Seif-Naraghi et al. demonstrated that the sulfated glycosaminoglycan content of decellularized pericardial ECM hydrogel provides prolonged retention and delivery rates of basic fibroblast growth factor (bFGF). Also, in rodents, intramyocardial injection of bFGF embedded into a pericardial matrix enhanced neovascularization compared to an alternative delivery approach using collagen (Seif-Naraghi et al., 2012).
TE approaches still raise many questions regarding safety, quality, and efficacy according to the requirements of the European Tissue and Cells Directives (2004/23/EC and 2006/17/EC). At all stages of the human tissue manufacturing process, a quality management system must be applied, from identification of potential donors through processing and storage to the final product for application to the patient. According to Good Tissue Practices (GTP), all the processes must be documented and validated to demonstrate that the quality and efficacy of the final product has not been compromised and avoid the potential risks such as microbial contamination or tissue damage (EUROGTPII Guide, 2018; Guide to the quality and safety of tissues and cells for human application, 2022). In particular, pericardium is obtained from cadaveric donors for transplantation purposes after the revision of social and medical history, physical examination, complete serological and microbiological testing, histopathological analysis, as well as any other information pertaining to risk factors for relevant communicable diseases (Figure 2). From the retrieval, the pericardium should be preserved in sterile containers until additional processing in a clean room environment. Processing steps include all operations involved in the preparation, manipulation, microbiological decontamination, preservation and packaging. The operations included in the preparation can comprise different activities such as thawing, washing, cutting, and soaking in decontamination solutions, sterilization, decellularization, lyophilization, and preservation before further product release. Importantly, all reagents used should be sterile, of the appropriate grade suitable for use in humans, conform to the national regulations and, when possible, should be approved for human use and CE (Conformité Européenne) marked. During processing and pre-packaging stages, effective and meaningful macroscopical and microbiological controls must be performed (Figure 2) (Guide to the quality and safety of tissues and cells for human application).
FIGURE 2. Stages of decellularized pericardial ECM biomanufacturing from cadaveric retrieval to the ultimate packaging prior clinical use. The images included in this figure are original or unpublished and thus it can be reproduced with no permission. Some of the illustrations have been obtained from smart.servier.com.
Recently, the use of human decellularized pericardial ECM, as a supportive platform to deliver therapeutic cells to the infarcted myocardium, has become a reality in clinical practice. In brief, we developed a novel double allogeneic pericardium-based investigational medicinal product (IMP), termed PeriCord, which combines MSC and human pericardium, to treat patients with a chronic myocardial scar (Prat-Vidal et al., 2020). The pericardial ECM is decellularized, lyophilized, sterilized and banked following GTP guidelines. The pericardial ECM is colonized with viable human allogeneic clinical-grade Wharton’s jelly-derived MSC expanded and banked according to good manufacturing practices (GMP). The release criteria of the human decellularized pericardium included, among others, negative serological and microbiological testing of the tissue donors, no evidence of microbial growth, residual moisture <10%, and gamma irradiation (25–35 kGy). On the other hand, implanted clinical-grade Wharton’s jelly-derived MSC meet all safety and quality standards regarding the number of cumulative population doublings, genomic stability, and sterility release criteria of the prior PeriCord manufacturing (Oliver-Vila et al., 2016). Remarkably, this IMP constitutes the first scalability and GTP/GMP-compliant biofabrication process that has reached clinical application in the context of MI, and is now being evaluated in the phase I PERISCOPE clinical trial (NCT03798353). Treated patients receive the PeriCord bioimplant, in the routinely surgical intervention, to cover the infarcted myocardial tissue. Preliminary safety data have already been reported after surgical implantation over a myocardial area unsuitable for revascularization from a 63-year-old male patient (Prat-vidal et al., 2020). Full safety confirmation and potential benefit are expected from the ongoing PERISCOPE clinical trial.
Several pre-clinical studies have shown that implantation of natural ECM is a promising cardiac TE approach for post-MI treatment. In specific, the use of pericardial ECM scaffolds or its injectable version permits to increase the retention and beneficial effects of therapeutic biologics (e.g., MSC and derived EV) on the increase in myocardial revascularization and reduction of adverse inflammatory response. However, in order to guarantee its clinical translation, the biomanufacturing of these novel TE products must be faced from the tissue and cell banks according to the requirements of the European Tissue and Cells Directives. Moreover, the whole procedure must follow GTP and GMP.
CC-S, CP-V, and SR review data, wrote the manuscript and expressed final approval of the manuscript for publication. PL-C, OF, AB-G, LR-G, and AV critically revised the manuscript and expressed final approval of the manuscript for publication.
This work was supported by the grants from the Spanish Ministry of Economy and Competitiveness–MICINN (PID 2019-110137RB-I00), Instituto de Salud Carlos III (ICI20/00135), Red RICORS-Terapias Avanzadas (PI21/01703), CIBER Cardiovascular (CB16/11/00403) projects as a part of the Plan Nacional de I + D + I, and it was co-funded by ISCIII-Subdirección General de Evaluación y el Fondo Europeo de Desarrollo Regional (FEDER) and AGAUR (2017-SGR-483).
The authors declare that the research was conducted in the absence of any commercial or financial relationships that could be construed as a potential conflict of interest.
All claims expressed in this article are solely those of the authors and do not necessarily represent those of their affiliated organizations, or those of the publisher, the editors and the reviewers. Any product that may be evaluated in this article, or claim that may be made by its manufacturer, is not guaranteed or endorsed by the publisher.
3-D, three-dimensional; Col I, type I collagen; ECM, extracellular matrix; EV, extracellular vesicles; bFGF, basic fibroblast growth factor; GMP, good manufacturing practices; GTP, good tissue practices; IMP, investigational medicinal product; LAM, laminin; MI, myocardial infarction; MSC, mesenchymal stromal cells; TE, tissue engineering.
Ascaso, M., Pruna-Guillen, R., Sandoval Martínez, E., and Quintana, E. (2021). Postinfarction posterior ventricular septal defect repair: Infarct exclusion technique. Multimed. Man. Cardiothorac. Surg. 29, 2021. doi:10.1510/mmcts.2021.078
Ashena, Z., Holmes, C., and Nanavaty, M. A. (2021). Pericardium patch graft for severe corneal wound burn. J. Curr. Ophthalmol. 33 (3), 342. doi:10.4103/joco.joco_195_20
Badylak, S. F., Taylor, D., and Uygun, K. (2011). Whole-organ tissue engineering: Decellularization and recellularization of three-dimensional matrix scaffolds. Annu. Rev. Biomed. Eng. 13, 27–53. doi:10.1146/annurev-bioeng-071910-124743
Castells-Sala, C., and Semino, C. E. (2012). Biomaterials for stem cell culture and seeding for the generation and delivery of cardiac myocytes. Curr. Opin. Organ Transpl. 17 (6), 681–687. doi:10.1097/mot.0b013e32835a34a6
Castells-Sala, C., Alemany-Ribes, M., Fernandez-Muiños, T., Recha-Sancho, L., and Lopez-Chicon, P. (2013). Current applications of tissue engineering in biomedicine. J. Biochip Tissue chip S2, 004. doi:10.4172/2153-0777.S2-004
Chen, F., Deng, J., Luo, L., Zhu, Y., Dong, Y., Yang, Y., et al. (2022). Crosslinked decellularized porcine pericardium as a substrate for conjunctival reconstruction. Stem Cells Int. 15, 7571146. doi:10.1155/2022/7571146
Crapo, P. M., Gilbert, T. W., and Badylak, S. F. (2011). An overview of tissue and whole organ decellularization processes. Biomaterials 32 (12), 3233–3243. doi:10.1016/j.biomaterials.2011.01.057
de Dorlodot, C., De Bie, G., Deggouj, N., Decat, M., and Gérard, J. M. (2015). Are bovine pericardium underlay xenograft and butterfly inlay autograft efficient for transcanal tympanoplasty? Eur. Arch. Otorhinolaryngol. 272 (2), 327–331. doi:10.1007/s00405-013-2855-8
EUROGTPII Guide (2018). Good tissue practices. Available at: www.eurogtps.com. see For guidance http://eurogtps.com/Portals/0/pdf/Euro%20GTP%20Final%20Delivery. pdf (accessed December 26, 2018).
Gálvez-Montón, C., Fernandez-Figueras, M. T., Martí, M., Soler-Botija, C., Roura, S., Perea-Gil, I., et al. (2015). Neoinnervation and neovascularization of acellular pericardial-derived scaffolds in myocardial infarcts. Stem Cell Res. Ther. 6 (1), 108. doi:10.1186/s13287-015-0101-6
Gálvez-Montón, C., Soler-Botija, C., Iborra-Egea, O., Díaz-Güemes, I., Martí, M., Iglesias-García, O., et al. (2017). Preclinical safety evaluation of allogeneic induced pluripotent stem cell-based therapy in a swine model of myocardial infarction. Tissue Eng. Part C. Methods 23 (11), 736–744. doi:10.1089/ten.tec.2017.0156
Gilbert, T. W., Sellaro, T. L., and Badylak, S. F. (2006). Decellularization of tissues and organs. Biomaterials 27 (19), 3675–3683. doi:10.1016/j.biomaterials.2006.02.014
Grayson, W. L., Martens, T. P., Eng, G. M., Radisic, M., and Vunjak-Novakovic, G. (2009). Biomimetic approach to tissue engineering. Semin. Cell Dev. Biol. 20 (6), 665–673. doi:10.1016/j.semcdb.2008.12.008
Grebenik, E. A., Gafarova, E. R., Istranov, L. P., Istranova, E. V., Ma, X., Xu, J., et al. (2020). Mammalian pericardium-based bioprosthetic materials in xenotransplantation and tissue engineering. Biotechnol. J. 15 (8), e1900334. doi:10.1002/biot.201900334
Grigorian Shamagian, L., Madonna, R., Taylor, D., Climent, A. M., Prosper, F., Bras-Rosario, L., et al. (2019). Perspectives on directions and priorities for future preclinical studies in regenerative medicine. Circ. Res. 124, 938–951. doi:10.1161/circresaha.118.313795
Guide to the quality and safety of tissues and cells for human application (2022). Blood supply contingency and emergency plan (B-SCEP) project. Available at: https://freepub.edqm.eu/publications.
Hedergott, A., Pink-Theofylaktopoulos, U., Neugebauer, A., and Fricke, J. (2021). Tendon elongation with bovine pericardium in strabismus surgery-indications beyond Graves' orbitopathy. Graefes Arch. Clin. Exp. Ophthalmol. 259 (1), 145–155. doi:10.1007/s00417-020-04939-7
Hernandez, M. J., Yakutis, G. E., Zelus, E. I., Hill, R. C., Dzieciatkowska, M., Hansen, K. C., et al. (2020). Manufacturing considerations for producing and assessing decellularized extracellular matrix hydrogels. Methods 171, 20–27. doi:10.1016/j.ymeth.2019.09.015
Inci, I., Norouz Dizaji, A., Ozel, C., Morali, U., Dogan Guzel, F., Avci, H., et al. (2020). Decellularized inner body membranes for tissue engineering: A review. J. Biomaterials Sci. Polym. Ed. 31 (10), 1287–1368. doi:10.1080/09205063.2020.1751523
Loh, Q. L., and Choong, C. (2013). Three-dimensional scaffolds for tissue engineering applications: Role of porosity and pore size. Tissue Eng. Part B Rev. 19, 485–502. doi:10.1089/ten.teb.2012.0437
Mihalj, M., Pasic, M., and Carrel, T. (2022). The 'beating-heart butterfly technique' for repair of basal post-infarction ventricle septum defect. Interact. Cardiovasc Thorac. Surg. 3, ivac026. doi:10.1093/icvts/ivac026
Moll, G., Ankrum, J. A., Olson, S. D., and Nolta, J. A. (2022). Improved MSC minimal criteria to maximize patient safety: A call to embrace tissue factor and hemocompatibility assessment of MSC products. Stem Cells Transl. Med. 11 (1), 2–13. doi:10.1093/stcltm/szab005
Monguió-Tortajada, M., Prat-Vidal, C., Moron-Font, M., Clos-Sansalvador, M., Calle, A., Gastelurrutia, P., et al. (2021). Local administration of porcine immunomodulatory, chemotactic and angiogenic extracellular vesicles using engineered cardiac scaffolds for myocardial infarction. Bioact. Mater 6 (10), 3314–3327. doi:10.1016/j.bioactmat.2021.02.026
Niegowski, L. J., Bravetti, G. E., Gillmann, K., Mansouri, K., and Mermoud, A. (2020). Pericardium patch graft (Tutoplast) for bleb repair and bleb remodelling after nonpenetrating filtering surgery: 6-Month outcomes. J. Glaucoma 29 (5), 347–350. doi:10.1097/ijg.0000000000001474
Oliver-Vila, I., Coca, M. I., Grau-Vorster, M., Pujals-Fonts, N., Caminal, M., Casamayor-Genescà, A., et al. (2016). Evaluation of a cell-banking strategy for the production of clinical grade mesenchymal stromal cells from Wharton's jelly. Cytotherapy 18 (1), 25–35. doi:10.1016/j.jcyt.2015.10.001
Perea-Gil, I., Gálvez-Montón, C., Prat-Vidal, C., Jorba, I., Segú-Vergés, C., Roura, S., et al. (2018). Head-to-head comparison of two engineered cardiac grafts for myocardial repair: From scaffold characterization to pre-clinical testing. Sci. Rep. 8 (1), 6708. doi:10.1038/s41598-018-25115-2
Prat-Vidal, C., and Bayes-Genis, A. (2020). Decellularized pericardial extracellular matrix: The preferred porous scaffold for regenerative medicine. Xenotransplantation 27 (1), e12580. doi:10.1111/xen.12580
Prat-Vidal, C., Gálvez-Montón, C., Puig-Sanvicens, V., Sanchez, B., Díaz-Güemes, I., Bogónez-Franco, P., et al. (2014). Online monitoring of myocardial bioprosthesis for cardiac repair. Int. J. Cardiol. 174 (3), 654–661. doi:10.1016/j.ijcard.2014.04.181
Prat-Vidal, C., Rodríguez-Gómez, L., Aylagas, M., Nieto-Nicolau, N., Gastelurrutia, P., Agustí, E., et al. (2020). First-in-human PeriCord cardiac bioimplant: Scalability and GMP manufacturing of an allogeneic engineered tissue graft. EBioMedicine 54, 10272. doi:10.1016/j.ebiom.2020.102729
Rajabi-Zeleti, S., Jalili-Firoozinezhad, S., Azarnia, M., Khayyatan, F., Vahdat, S., Nikeghbalian, S., et al. (2014). The behavior of cardiac progenitor cells on macroporous pericardium-derived scaffolds. Biomaterials 35 (1), 970–982. doi:10.1016/j.biomaterials.2013.10.045
Rémi, E., Khelil, N., Di centa, I., Roques, C., Ba, M., and Madhahed-Hamidi, F. (2011). “Pericardial Processing: Challenges, Outcomes and Future Prospects,” in Biomaterials Science and Engineering. Editor R. Pignatello (London, United Kingdom: IntechOpen), Available at: https://www.intechopen.com/chapters/19731. (Accessed July 11, 2022).
Ricci, K. B., Higgins, R., Daniels, V. C., and Kilic, A. (2014). Bovine pericardial reconstruction of the diaphragm after a heart transplant. Exp. Clin. Transpl. 12, 277–278. doi:10.6002/ect.2013.0068
Rodriguez, E. R., and Tan, C. D. (2017). Structure and anatomy of the human pericardium. Prog. Cardiovasc. Dis. 59 (4), 327–340. doi:10.1016/j.pcad.2016.12.010
Sainsbury, E., do Amaral, R., and Blayney, A. W. (2022). Tissue engineering and regenerative medicine strategies for the repair of tympanic membrane perforations. Biomaterials Biosyst, 6, 100046. doi:10.1016/j.bbiosy.2022.100046
Sarig, U., and Machluf, M. (2011). Engineering cell platforms for myocardial regeneration. Expert Opin. Biol. Ther. 11 (8), 1055–1077. doi:10.1517/14712598.2011.578574
Seif-Naraghi, S. B., Salvatore, M. A., Schup-Magoffin, P. J., Hu, D. P., and Christman, K. L. (2010). Design and characterization of an injectable pericardial matrix gel: A potentially autologous scaffold for cardiac tissue engineering. Tissue Eng. Part A 16 (6), 2017–2027. doi:10.1089/ten.tea.2009.0768
Seif-Naraghi, S. B., Horn, D., Schup-Magoffin, P. J., and Christman, K. L. (2012). Injectable extracellular matrix derived hydrogel provides a platform for enhanced retention and delivery of a heparin-binding growth factor. Acta Biomater. 8 (10), 3695–3703. doi:10.1016/j.actbio.2012.06.030
Solakoğlu, Ö., Ofluoğlu, D., Schwarzenbach, H., Heydecke, G., Reißmann, D., Ergun, S., et al. (2022). A 3-year prospective randomized clinical trial of alveolar bone crest response and clinical parameters through 1, 2, and 3 years of clinical function of implants placed 4 months after alveolar ridge preservation using two different allogeneic bone-grafting materials. Int. J. Implant Dent. 8 (1), 5. doi:10.1186/s40729-022-00402-w
Sun, H., Wang, H., Diao, Y., Tu, Y., Li, X., Zhao, W., et al. (2018). Large retrospective study of artificial dura substitute in patients with traumatic brain injury undergo decompressive craniectomy. Brain Behav. 8 (5), e00907. doi:10.1002/brb3.907
Thompson, M., Mei, S. H. J., Wolfe, D., Champagne, J., Fergusson, D., Stewart, D. J., et al. (2020). Cell therapy with intravascular administration of mesenchymal stromal cells continues to appear safe: An updated systematic review and meta-analysis. EClinicalMedicine 19, 100249. doi:10.1016/j.eclinm.2019.100249
Vunjak-Novakovic, G., Tandon, N., Godier, A., Maidhof, R., Marsano, A., Martens, T. P., et al. (2010). Challenges in cardiac tissue engineering. Tissue Eng. Part B Rev. 16, 169–187. doi:10.1089/ten.teb.2009.0352
Xing, Q., Parvizi, M., Lopera Higuita, M., and Griffiths, L. G. (2021). Basement membrane proteins modulate cell migration on bovine pericardium extracellular matrix scaffold. Sci. Rep. 11 (1), 4607. doi:10.1038/s41598-021-84161-5
Yaliniz, H., Salih, O. K., Atalay, A., Keklik, V., Gocen, U., Topcuoglu, M. S., et al. (2014). Short- and mid-term results of xenograft-bovine pericardial patch in the repair of intracardiac defects: Final results of a single-centre study. Cardiol. Young 24, 510–514. doi:10.1017/s1047951113000711
Keywords: pericardium, decellularization, extracellular matrix, mesenchymal stromal cells, extracellular vesicles, cardiac tissue engineering, cardiac repair
Citation: Castells-Sala C, Prat-Vidal C, Roura S, López-Chicón P, Fariñas Ó, Bayes-Genis A, Rodríguez-Gómez L and Vilarrodona A (2022) Human pericardial extracellular matrix: An implantation platform for cardiac tissue engineering. Front. Front. Biomater. Sci. 1:953837. doi: 10.3389/fbiom.2022.953837
Received: 26 May 2022; Accepted: 30 June 2022;
Published: 19 July 2022.
Edited by:
Elisabeth Engel, Institute for Bioengineering of Catalonia (IBEC), SpainReviewed by:
Ana Mora Boza, Georgia Institute of Technology, United StatesCopyright © 2022 Castells-Sala, Prat-Vidal, Roura, López-Chicón, Fariñas, Bayes-Genis, Rodríguez-Gómez and Vilarrodona. This is an open-access article distributed under the terms of the Creative Commons Attribution License (CC BY). The use, distribution or reproduction in other forums is permitted, provided the original author(s) and the copyright owner(s) are credited and that the original publication in this journal is cited, in accordance with accepted academic practice. No use, distribution or reproduction is permitted which does not comply with these terms.
*Correspondence: Anna Vilarrodona, YXZpbGFycm9kb25hQGJzdC5jYXQ=
†These authors have contributed equally to this work and share first authorship
Disclaimer: All claims expressed in this article are solely those of the authors and do not necessarily represent those of their affiliated organizations, or those of the publisher, the editors and the reviewers. Any product that may be evaluated in this article or claim that may be made by its manufacturer is not guaranteed or endorsed by the publisher.
Research integrity at Frontiers
Learn more about the work of our research integrity team to safeguard the quality of each article we publish.