- 1Department of Pharmacology, Monash University, Clayton, VIC, Australia
- 2Biomedicine Discovery Institute, Monash University, Clayton, VIC, Australia
Numerous promising drug leads are regularly abandoned due to having poor pharmacokinetic profiles. Biomaterials are often used as drug delivery systems to improve the pharmacokinetics of these otherwise promising drug candidates. Hydrogels are a subset of biomaterials that offer porous matrices, permeable to endogenous nutrients in aqueous in vivo environments. Environmentally sensitive hydrogels have become of interest to further tailor these materials to only allow therapeutic release in response to specific environmental cues instead of simple encapsulation and subsequent diffusion. Enzyme-responsive materials allow for the exploitation of endogenous tissue enzyme expression levels and/or altered expression levels during pathological states. The simplest and most common method for stimulus-dependant release is through the destruction of the matrix to release encapsulated therapeutics that would otherwise be trapped indefinitely. A second approach is to covalently attach therapeutics to the hydrogel scaffold and include enzymatically sensitive cross linkages throughout the scaffold backbone. The third, and least common approach, is to use labile linkers between the therapeutic and the scaffold which affords controlled, precise release of the therapeutic with a known molecular structure. These linkers can also be tailored to specific enzymes that are elevated in certain disease states. This review will; 1) briefly describe matrix degradation; 2) present the cleavage of covalently attached therapeutics and; 3) highlight the few examples of targeted cleavage of therapeutics from specific matrix locations and the potential use of these systems in biomedicine.
Introduction
Hydrogels are permeable three-dimensional matrices of fibres that exhibit physical characteristics of soft tissues. Extensive research has been invested in engineering these biomaterials to serve various clinical applications such as diagnostics (Schiffer et al., 2015), providing physical support (Griffin et al., 2015), tissue regeneration (Dang et al., 2017), cell delivery (McFetridge et al., 2018) and drug delivery (Thambi et al., 2017). Currently there are over 30 injectable hydrogel products approved by the European Medicines Agency and the US Food and Drug Administration and a plethora of models being investigated in clinical trials (Mandal et al., 2020).
Many drugs are abandoned due to poor pharmacokinetics (PK), particularly biomolecules. Tailored biomaterials like hydrogels provide a solution which could become important in streamlining pharmacological research and translation into clinical use. Poor PK of lead compounds could be overcome through the use of such biomaterials and could present new avenues in drug treatment. Overall, hydrogels can drastically improve the effectiveness of therapies while also reducing dosage, dose frequency and adverse effects associated with high systemic exposure of delivered therapeutics. Hydrogels provide protection from non-specific cellular uptake, enzymatic degradation, and rapid clearance of therapeutics (Li and Mooney, 2016).
Stimulus-dependant release has been explored to further tailor biomaterials to only commence releasing the therapeutic payload in response to specific environmental cues instead of simple encapsulation and subsequent diffusion. These stimuli include pH, light, redox conditions or enzymatic action or various combinations of these for further refinement (Vemula et al., 2011; Chandrawati, 2016; Wang et al., 2019). The ability to selectively release encapsulated drugs in the presence of chosen environmental cues can be extremely advantageous as it not only provides control over drug dosage but avoids burst release and subsequent high plasma concentrations.
The use of enzymes for pro-drug activation and drug metabolism has been established over the last few decades (Fukami and Yokoi, 2012) and it comes as no surprise that they are now becoming incorporated into responsive DDS. Enzyme-responsive materials are highly advantageous as endogenous enzyme expression can be used in controlling the release rate of various therapeutics for a wide range of applications. This variety is also evident in the array of functional groups that have been incorporated into hydrogel scaffolds that change in structure or are cleaved via exposure to enzymatic activity (Hu et al., 2012). Some systems have been designed to use phasic enzyme expression levels in certain disease states to release a payload only when needed during a pathological state (Joshi et al., 2018). This review will describe the use of enzymatically sensitive components incorporated into DDSs to release encapsulated therapeutics through matrix degradation, as well as conjugated therapeutics through targeted cleavage utilising endogenous enzymes (Figure 1).
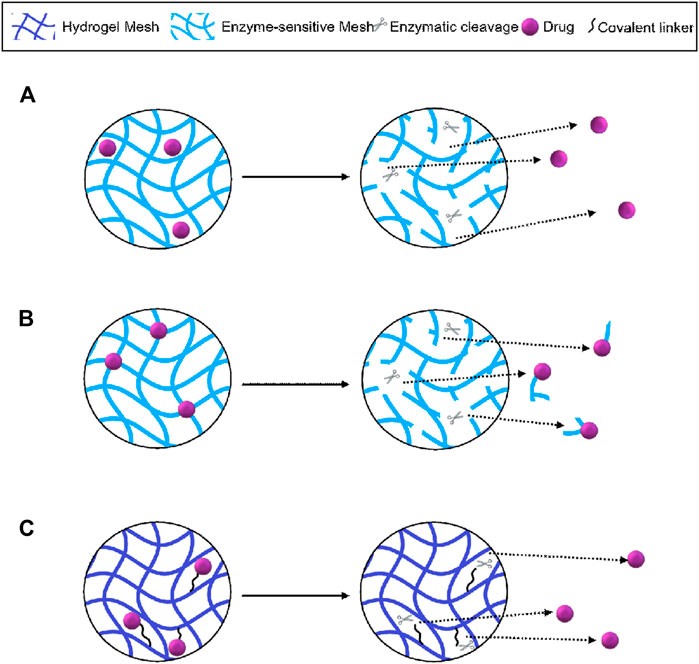
FIGURE 1. Schematic representation of hydrogel-mediated controlled release using; (A) matrix degradation and diffusion of encapsulated drugs; (B) non-specific enzymatic cleavage and release of conjugated therapeutics and (C) targeted cleavage of drugs conjugated via enzyme-sensitive linkers. Figure adapted from Li and Mooney (2016).
Matrix-Degradation Mediated Release of Encapsulated Molecules
The rational design of enzyme-responsive biomaterials can allow exploitation of differences in tissue expression levels or altered physiological environments during pathological states. Encapsulated therapeutic compounds can be released in the presence of specific enzymes by generating hydrogels entirely from suitable substrates or by incorporating degradable sections within integral fibres or crosslinkers. In this section the variety of such designs and their applications in diverse applications are briefly discussed and presented in Table 1, where relevant studies are categorized by the cleaving enzyme [matrix metalloproteinase (Shepard et al., 2010; Amer and Bryant, 2016; Foster et al., 2017; Wang H. H. et al., 2018; Guo et al., 2019; Chakroun et al., 2020); digestive enzymes (Knipe et al., 2016; Wooster et al., 2019); carboxylesterase (Zhang et al., 2015; Joshi et al., 2018; Liu et al., 2022); protein tyrosine kinase (Wu et al., 2018) and endoglycosidase (Zhang et al., 2015; Lyu et al., 2018)]. Note that DNA-based hydrogels have also been designed for drug delivery in response to DNases and nucleases (Lyu et al., 2018) and the breadth of protease-responsive materials have been extensively reviewed elsewhere (Gacanin et al., 2020). Studies that include the encapsulation of cells have been excluded as they are beyond the scope of this review.
A key consideration in the design of individual tailored DDSs is the type and expression levels of the enzymes that are characteristic within certain tissues. Likewise, the environmental conditions, found within tissues, like pH or any oxidative species present, at can also be utilised for tailored delivery. For example, the gastro-intestinal tract has divergent enzyme expression levels and acidity, that is, dependant on the location and whether it is in the fed or unfed state. Different chemistries can be used to selectively deliver therapeutics to the lower intestine after surviving the route through the highly acidic stomach environment. For example, Knipe et al. (2016), designed a polymeric material that adopted a condensed structure at low pH levels to protect encapsulated trypsin-degradable nanogels. The polymer backbone was then able to swell at more neutral pH levels to expose the nanogels to trypsin-mediated degradation, releasing sequestered siRNA for an anti-inflammatory effect in a mouse model of inflammatory bowel disease. Another approach is to control the porosity such that within gastric conditions the main mechanism of hydrogel degradation is surface erosion in contrast to intestinal conditions where enzymatic digestion prevails. Wooster et al. (2019), partitioned their DDS by encapsulating lipid-gelatin cores within a highly dense protein and polysaccharide hydrogel shell. Through an in vitro simulation of gastric and intestinal conditions they demonstrated that increasing the protein content of the hydrogel enhanced matrix density which prolonged the delayed burst release profile (Wooster et al., 2019). A third approach is to avoid the gastric environment entirely and administer the DDS via an enema. Zhang et al. (2015), generated an ascorbyl palmitate-based hydrogel that contained labile ester bonds in the backbone and encapsulated the anti-inflammatory corticosteroid, dexamethasone. Administration of this DDS in mice with colitis significantly reduced inflammation and exhibited lower peak drug concentrations. They also demonstrated that negative surface charge of the hydrogel targets inflamed tissue due to increased expression of positively charged proteins on inflamed intestinal mucosa in human patient tissue samples (Zhang et al., 2015). A limitation of the latter approach is that while enemas provide a targeted treatment, oral administration is generally favored due to patient compliance and convenience. Esterases can be advantageous in cleaving biomaterials as they are expressed in most tissues and can be upregulated in disease (Zhang et al., 2015). Another application that exploits lipase activity and phasic expression levels in disease states was published by Joshi et al. (2018). They designed a small molecule amphiphile (triglycerol monostearate) based hydrogel to deliver encapsulated corticosteroids. The hydrogel backbone contained ester bonds and would only degrade and release the therapeutic when exposed to an enzyme cocktail or synovial fluid from arthritic patients in vitro. Furthermore, the DDS reduced the paw thickness and clinical score of an arthritic mouse model (Joshi et al., 2018). Another enzyme, that is, known to have a pathology-dependant expression levels are protein tyrosine kinases (PTK), which are key signalling molecules that phosphorylate tyrosine residues of target peptides using ATP. PTK is known to be activated on T cells after organ transplant and has been used as a selective stimulus to release an immunosuppressive drug in liver-transplant recipient rats by Wu et al. (2018). This is yet another example of exploiting endogenous shifts in enzyme expression levels to generate truly targeted materials.
In addition, there have been multiple studies including additional components such as requiring more than one enzyme to degrade hydrogel matrices or particles encapsulated within that contain the therapeutic payload (Hu et al., 2012). For example, Tulhadar et al. (2020), generated a composite system comprising of two individual therapeutics within separate Poly (lactic-co-glycolic acid) (PLGA) microparticles that were encapsulated within a hyaluronic acid (HA) and methylcellulose hydrogel. The purpose of this multi-faceted DDS was to target the inflammatory phase as well as the recovery phase of ischemic stroke. The authors demonstrated burst release of an anti-inflammatory factor and slow release of an angiogenic factor over 32 days (Tuladhar et al., 2020). Liu et al. (2022), added greater complexity to this approach by generating different nanocarriers for each drug that were then encapsulated within a hyaluronic acid (HA) hydrogel. The HA scaffold would be degraded by hyaluronidase and the nanocarriers by esterases. Using a photothrombotic model of stroke in rats, they observed significant anti-inflammatory action, increases in angiogenic markers and improvement in behavioural measures. Other similar composite DDSs have been reviewed elsewhere (Kass and Nguyen, 2021).
Cleavage and Release of Covalently Tethered Therapeutics
Multiple studies have used covalent tethering to further control the release or exposure of the payloads to the physiological environment. Although the synthesis of such conjugated materials can be more challenging than encapsulation and subsequent diffusion, it provides the means to select appropriate conditions required for drug release. For example, Edelbrock et al. (2017), delivered a neurotrophic factor through conjugating its non-functional lysine to a carboxylic acid functionalized hexaethylene glycol which was linked to protease-sensitive self-assembling (SA) peptide amphiphile monomers. Murine primary cortical neurons demonstrated increased phosphorylation of the target TrkB receptor by their DDS and free neurotrophic factor as compared to non-functional negative controls. The authors also compared the efficacy of their DDS and simple encapsulation through examining cortical neurons 1 week after being seeded on various hydrogel compositions. The encapsulation and conjugation techniques presented similar phenotypically mature levels and significantly increased action potential firing to a similar extent. Although, the conjugation approach demonstrated significantly greater infiltration of neurites from the top of the hydrogel surface than any other group, likely due to burst release of the encapsulated group for which there is no published kinetic data.
A similar strategy involved gelating HA that was modified to conjugate bisphosphonate to the HA backbone with a hydrazone linkage (Varghese et al., 2009). The ubiquitously expressed HA-specific enzyme, hyaluronidase, was hypothesized to fragment the hydrogel scaffold for selective endocytosis through CD44 receptors that are over-expressed on cancer cells. The covalently bound bisphosphonate would then cause cell-specific toxicity and apoptosis. The specificity of cellular uptake was investigated by using two cell lines that had either high (HCT-116 cells) or low (HEK-293T cells) expression levels of CD44. Firstly, they demonstrated that fragmentation of the matrix fibres was necessary for endocytosis as addition of hyaluronidase was required for cellular uptake after 3 days of incubation with HA. They then demonstrated bisphosphonate concentration-dependant cytotoxicity of the two cell lines, the extent of which correlated with their divergent CD44 expression levels. The effect of various modifications to HA-based materials on CD44-binding has been further explored in recent years (Kwon et al., 2019).
Although the rationale of using HA scaffold fragments to target endocytosis through cell-specific machinery is justified, Varghese et al. (2009), do note the variability in potency of free drug and the resulting product post-cleavage (Fan et al., 2019). The IC50 of bisphosphonate in the two cell lines, measured via cytostatic MTT assay, were reduced by six- and ten-fold due to the non-specific cleavage along HA fibres that results in drug-scaffold conjugates that are structurally diverse. This draws attention to the unpredictability of scaffold-drug physicochemical properties that prevents accurate quantification of drug release kinetics which forces researchers to rely on indirect functional outcomes to infer drug release. This is an unfortunate shortcoming as one of the main benefits of responsive DDS is the ability to slow release rates which would otherwise be driven by osmotic pressure. Varghese et al. (2009), are the only authors to comment on this directly and provide experimental data for effects on potency. Importantly, this structural variety could also hinder the efficacy of such approaches by steric or electrochemical interference at the drug-receptor interface and cause off-target effects. Therefore, DDSs that adopt a more targeted cleavage mechanism by introducing enzyme-sensitive linkers to conjugate therapeutics to the hydrogel scaffold are of potentially greater clinical relevance and are discussed further.
Targeted Cleavage of Synthesized Covalent Linkers
The benefits of hydrogel-mediated drug delivery have been established, though generation of highly versatile systems with finely tuned control over release rate via enzymatic cleavage for specific applications remains a challenge. This has resulted in few published examples, even though the approach lends well to tailored therapy as the expression of enzymes in pathophysiological conditions can control the release. As discussed previously, it is advantageous to ensure predictability of physicochemical properties of the resulting cleaved product which can be achieved through targeted cleavage sites designed between the cargo and scaffold. This is where the composition of the released therapeutic is minimally altered which reduces the potential for interference with drug-receptor interfacing and non-selective activity. Rather than covalently attaching drugs to degradable hydrogel matrices, an approach that results in uniform products post-cleavage is the design of degradable linkers that are shorter lived than the matrix itself. This ensures predictable size, structure, and bioactivity of released therapeutics.
As discussed previously, MMPs are upregulated in response to myocardial infarction (MI), especially MMP-2. Fan et al. (2019), designed a DDS to deliver basic fibroblast growth factor (bFGF) to support angiogenesis and prevent negative cardiac remodelling post-MI. They used a unique DDS that involves a covalent group attached to the cargo that interacts non-covalently with an additional component of the hydrogel matrix. The bFGF was modified to include a tissue inhibitor of metalloproteinases (TIMP) peptide with a glutathione S-transferase (GST) moiety appended to the TIMP. The hydrogel scaffold was composed of collagen with glutathione (GSH) covalently bound. Subsequent mixing of the modified growth factor and hydrogel monomers resulted in a disulphide bond between the GST and GSH components (Figure 2A). The inclusion of the TIMP peptide portion of this linker inhibited MMP-2, which was hypothesised to inhibit the remodelling effects of MMPs post-MI. Although there was a slight burst release within 24 h of bFGF in all hydrogel conditions tested, the inclusion of the TIMP peptide segment and MMP-2 caused a significantly higher release at all timepoints tested over an 8-day period. The efficacy of this DDS was assessed in rats subjected to simultaneous MI and intra-myocardial hydrogel injection after 30 days. Cardiac function as measured by echocardiography revealed significant functional improvement. Histochemical analysis also demonstrated inhibition of negative cardiac remodelling processes. The designed DDS was also compared to simple encapsulation in collagen-based hydrogel by measuring bFGF levels in blood from the rat study as the GST-TIMP-bFGF showed slow, gradual release whereas simple encapsulation resulted in a burst release that diminished over the 5-day period measured. Fan et al. (2019), designed a system that to our knowledge is the first to include a cleavable linker on the therapeutic which is then joined to the matrix through further modification of a non-covalent interaction. Subsequent studies discussed herein have a more direct approach of covalent drug-matrix conjugation via various linkers.
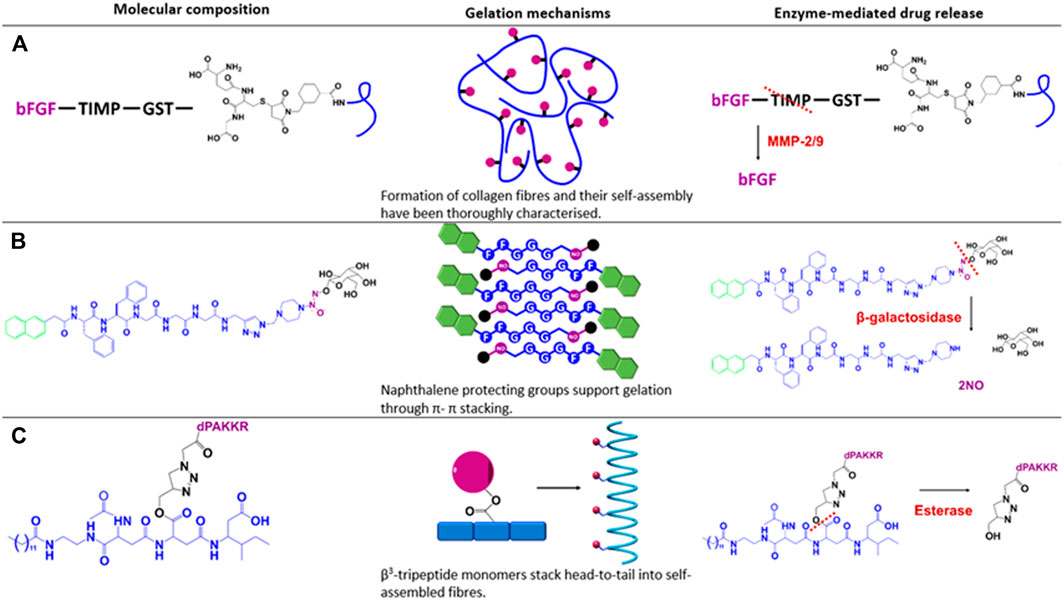
FIGURE 2. Depiction of the composition, assembly mechanism and targeted cleavage of the discussed drug delivery systems. Blue indicates the hydrogel backbone, purple indicates delivered therapeutics, green indicates protecting groups, red lines indicate the cleavage site for the corresponding enzymes in red text. Figure adapted from (A) Purcell et al. (2014), Fan et al. (2019); (B) Gao et al. (2013), Gacanin et al. (2020); and (C) Kwon et al. (2019), Kulkarni et al. (2021).
Another example of cleavage-induced inhibition of deleterious enzymes was designed by Maitz et al. (2013), who conjugated carboxylic acid moieties of heparin to starPEG via a thrombin-sensitive peptide sequence crosslink for the application of post-operative clot prevention. They demonstrated the suitability of the peptide sequence as a thrombin substrate as replacing it with a scrambled sequence showed substantially lower heparin release after a 3 h incubation with whole blood. The release rate of their DDS exhibited a linear release over an 8 h time course that was tuneable by altering the level of peptide crosslinking with increased crosslinking leading to slower release rates. They also demonstrated reduced thrombin activity in static conditions and in the presence of a pro-coagulant stimulus. Lastly, incubation of freshly drawn whole blood with thrombin-responsive hydrogels prevented coagulation over the 1 h observed while complete coagulation was noted in non-responsive hydrogels which was consistent with an additional resupply of blood and incubation for 1 h.
Gao et al. (2013), designed a hydrogel based on the SA peptide, Nap-FFGGG, that was covalently linked to an azide-containing, galactose-protected nitric oxide (NO) donor for topical wound healing. In contrast to most studies discussed thus far, the release of NO was primarily dependant on an exogenous addition of the enzyme β-galactosidase, which removes the galactose through hydrolysis and releases the protected NO (Figure 2B). An 8-hour time course demonstrated NO release to be linear and β-galactosidase-concentration dependant, with zero NO detected from hydrogels in the absence of β-galactosidase. In mice with excision of dorsal skin demonstrated the most significant healing and neo-vascularization with the NO gel and β-galactosidase exposure over 7 days as compared to other controls.
Another DDS that involves exogenous enzymes was based on poly (vinyl alcohol) (PVA) with a topically applied multilayered microneedle comprising a core and shell for appending onto a topical patch (Wang J. et al., 2018). This segregated material is designed for the generation of H2O2 by glucose oxidase (GOx)-induced oxidation of blood glucose to degrade the chemical linker attaching insulin to the matrix in the core as well as cross linkages that are included to help gelate the PVA matrix. Both the insulin linker and H2O2-labile cross linkers are oxidized and hydrolysed by the action of GOx. The H2O2 is reduced by the covalently bound H2O2-scavenging enzyme, catalase, to water for reduced cytotoxicity to neighbouring tissues. To ensure immobilization of both enzymes, they were encased in an acrylate nanogel that was subsequently linked to the PVA matrix in their respective sections. Therefore, increased glucose levels will release insulin to return levels to homeostatic conditions. The glucose-responsiveness of their system was ultimately exemplified in an in vitro assay that exposed the hydrogel to repeating cycles of normoglycemic and hyperglycaemic glucose concentrations, eliciting pulsatile insulin release. The efficacy of this DDS was also shown in vivo as normoglycemic blood glucose levels were maintained for 40 h in drug-induced diabetic mice. Additionally, application of the patches to healthy mice caused a significantly lower hypoglycaemic response as compared to subcutaneous insulin injection.
Our group has also investigated targeted cleavage via esterase-sensitive linkers incorporated into a β3-tripeptide backbone. We have shown that β3-tripeptides will self-assemble into injectable hydrogels despite the conjugation of larger structures (Kulkarni et al., 2016; Motamed et al., 2016; Kulkarni et al., 2018). Given this observation, we proceeded to conjugate several ligands onto the peptide scaffold. We demonstrated targeted release of conjugated lactose over 28 days, which only occurred in the presence of serum esterases. Esterase-mediated release of conjugated BDNF mimetics was observed via LC-MS and responsible for enhanced neuronal survival of primary murine dorsal root ganglion neurons (Figure 2C). Normal neuronal function was also supported after 7 days as assessed via calcium imaging (Kulkarni et al., 2021). Although there was a portion of linker attached to the cleaved product, our results indicate that the bioactivity of the molecule was not affected. However, radioligand binding is required to determine whether the potency of the product is altered compared to the original molecule.
While all previously discussed DDS achieve controlled release through the activity of specific enzymes, several studies have designed materials that also require an additional environmental condition to achieve drug release. For example, Badeau et al. (2018), has designed a PEG based hydrogel to deliver small molecules following multiple environmental triggers. An azido group anchors dual linkers that are sensitive to MMP-8, light or chemical reduction to the PEG scaffold. Doxorubicin was covalently attached to the PEG scaffold via these input-dependant linkers. This group has since modified their systems using model small molecules such as fluorescein (Badeau et al., 2018) and enhanced green fluorescent proteins (GFP) (Ruskowitz et al., 2019). Through incubating hydrogels with different combinations of dual linkers in different conditions they were able to demonstrate the selective degradation of linkers in specific conditions and the requirement for dual inputs in the case of dual linkers. However, no release kinetics were reported. The creation of these DDS is highly technical and has contributed much to the field of materials science, though the applicability in the clinic over enzyme-only responsive materials has not been well described. All discussed examples have been summarised in Table 2.
Future Developments in Enzymatically Sensitive DDS
The goal of designing enzymatically sensitive DDS is to provide a high degree of control over drug dosage over the medium-long term from a minimally invasive treatment. Achieving this would reduce treatment frequency and associated costs while increasing patient compliance and therapeutic efficacy. This is particularly important as the demand for long-term drug release continues to grow as average lifespan and subsequent burden of chronic disease rises. Thus far, there are only ten clinically available drug-delivering injectable hydrogels (Mandal et al., 2020). Seven of these deliver a burst release of an analgesic to sooth injection-related pain and all ten deliver via osmotic pressure. The stark contrast between the overarching goal of the field and the current lack of clinical translation can, in part, be explained by the relative youth of the drug delivery field that began in the 1950s and has only begun exploring enzyme-mediated release from hydrogels in the 2000s. In addition, the vast variety in hydrogel composition, delivered therapeutic, disease setting and design approach as presented in this review could be contributing to a slow, but steady, progression breadthwise. It can also be explained by the intensive research devoted to generating formulations in nanomedicine that have certainly provided medical breakthroughs and contributed to the current market but were not as successful as predicted in terms of long-term delivery (Park et al., 2022). Finally, Bae and Park (2020), have expressed a pessimistic view that advances in drug delivery technologies are stalled by the current funding systems that favour modified existing techniques and discourage new radical ideas.
An important aspect to consider in future clinical translation is the high variability of enzyme expression between patients and during disease states. These characteristics must be thoroughly understood for the relevant medical application before such treatments can be administered to patients. However, with the rising trend of personalised medicine and continual progress in processing patient biomarkers, this may well be accomplished in the coming decade. There are already many examples of increased enzyme levels being used as biomarkers for the diagnosis of disease (including various cancers, neurodegenerative diseases, cardiac and liver diseases) (Jansen et al., 2015; Lazo et al., 2015; Parnetti et al., 2019; Kaul et al., 2021). It is quite possible that there will be simple biomarker tests available in the near future that could solve this particular obstacle.
Biomedical understanding of disease pathophysiologies has improved over the years as a function of medical research. Wholistic understanding of a diseases progression or phasic changes in enzyme expression profiles allows for the design of systems that are uniquely suited to those environments. As time passes, there will be further breakthroughs, increases in efficiency and lowered cost in generating these materials. Considering the complexity of generating true targeted cleavage-driven DDS, they will likely remain the lesser researched category of DDS. Polymer-based materials, small molecules and simple encapsulation will continue to dominate the market due to low cost, low effort and high yield. However, some drugs may might be reformulated to be more suited to covalent conjugation to suitable scaffolds, increasing the chance of success. In addition, enzyme-responsive DDSs are a relatively new field and the relevance in dynamic diseases where enzyme expression is changed will be of great interest with the rise of personalised medicine (Gawade et al., 2019).
As this promising field continues to grow and generate highly precise drug delivery control, it is important to be mindful of translatability to the clinical setting and ensure that they are logistically feasible. This will include not over-complicating these systems unnecessarily, relying primarily on endogenous enzymatic cleavage and considering convertibility of a DDS to other applications. Given the need for these materials to deliver drugs over the course of many weeks to months, the ability of the materials to evade any foreign body response or to mitigate any phagocytic attack is likely to be the most critical and difficult obstacle. Controlling clearance of the material is crucial as any removal would likely include the delivered therapeutic, thus impacting on the total dose. Ultimately, a material, that is, invisible to the body whilst still responding to enzymatic processes would indeed present a paradigm-shifting advance in the field. Researchers within the field understand that pharmacokinetics underpins this field, and by that measure, these materials should be utilised for therapeutics with typically poor pharmacokinetic profiles. The emergence of materials that are able to deliver these molecules over sustained periods will assist in patient compliance and provide new or alternate therapies for poorly treated diseases, much like the effect that monthly intravenous infusion of antibodies has had in the clinic. Therefore, pharmacokinetic quantification is paramount for any proper progression into a clinical setting.
Conclusion
Enzyme-mediated drug delivery from biomaterials presents a valuable area that could become a tool in pharmacological research and development to combat the issues of poor PK, particularly with biomolecules including peptides and proteins. Furthermore, we have presented evidence that these DDS can also be designed to respond to divergent enzyme-expressions in disease states, and up or downregulating them as required. As discussed, the most specific and promising approach is the incorporation of cleavable covalent linkers between the hydrogel scaffold and delivered therapeutic to generate bespoke materials capable of highly controlled release of predictable molecules.
Author Contributions
MD and RM contributed equally to the idea generation, manuscript writing, proofing and preparation.
Conflict of Interest
The authors declare that the research was conducted in the absence of any commercial or financial relationships that could be construed as a potential conflict of interest.
Publisher’s Note
All claims expressed in this article are solely those of the authors and do not necessarily represent those of their affiliated organizations, or those of the publisher, the editors and the reviewers. Any product that may be evaluated in this article, or claim that may be made by its manufacturer, is not guaranteed or endorsed by the publisher.
References
Amer, L. D., and Bryant, S. J. (2016). The In Vitro and In Vivo Response to MMP-Sensitive Poly(Ethylene Glycol) Hydrogels. Ann. Biomed. Eng. 44 (6), 1959–1969. doi:10.1007/s10439-016-1608-4
Badeau, B. A., Comerford, M. P., Arakawa, C. K., Shadish, J. A., and DeForest, C. A. (2018). Engineered Modular Biomaterial Logic Gates for Environmentally Triggered Therapeutic Delivery. Nat. Chem. 10 (3), 251–258. doi:10.1038/nchem.2917
Bae, Y. H., and Park, K. (2020). Advanced Drug Delivery 2020 and beyond: Perspectives on the Future. Adv. Drug Deliv. Rev. 158, 4–16. doi:10.1016/j.addr.2020.06.018
Chakroun, R. W., Sneider, A., Anderson, C. F., Wang, F., Wu, P. H., Wirtz, D., et al. (2020). Supramolecular Design of Unsymmetric Reverse Bolaamphiphiles for Cell‐Sensitive Hydrogel Degradation and Drug Release. Angew. Chem. Int. Ed. 59 (11), 4434–4442. doi:10.1002/anie.201913087
Chandrawati, R. (2016). Enzyme-responsive Polymer Hydrogels for Therapeutic Delivery. Exp. Biol. Med. (Maywood) 241 (9), 972–979. doi:10.1177/1535370216647186
Dang, Q., Liu, K., Zhang, Z., Liu, C., Liu, X., Xin, Y., et al. (2017). Fabrication and Evaluation of Thermosensitive Chitosan/collagen/α, β-glycerophosphate Hydrogels for Tissue Regeneration. Carbohydr. Polym. 167 (1), 145–157. doi:10.1016/j.carbpol.2017.03.053
Edelbrock, A. N., Àlvarez, Z., Simkin, D., Fyrner, T., Chin, S. M., Sato, K., et al. (2018). Supramolecular Nanostructure Activates TrkB Receptor Signaling of Neuronal Cells by Mimicking Brain-Derived Neurotrophic Factor. Nano Lett. 18 (10), 6237–6247. doi:10.1021/acs.nanolett.8b02317
Fan, C., Shi, J., Zhuang, Y., Zhang, L., Huang, L., Yang, W., et al. (2019). Myocardial‐Infarction‐Responsive Smart Hydrogels Targeting Matrix Metalloproteinase for On‐Demand Growth Factor Delivery. Adv. Mat. 31 (40), 1902900–1902912. doi:10.1002/adma.201902900
Foster, G. A., Headen, D. M., González-García, C., Salmerón-Sánchez, M., Shirwan, H., and García, A. J. (2017). Protease-Degradable Microgels for Protein Delivery for Vascularization. Biomaterials 113, 170–175. doi:10.1016/j.biomaterials.2016.10.044
Fukami, T., and Yokoi, T. (2012). The Emerging Role of Human Esterases. Jpn. Soc. Study Xenobiotics 27 (5), 466–477. doi:10.2133/dmpk.dmpk-12-rv-042
Gacanin, J., Synatschke, C. V., and Weil, T. (2020). Biomedical Applications of DNA-Based Hydrogels. Adv. Funct. Mater. 30 (4), 25. doi:10.1002/adfm.201906253
Gao, J., Zheng, W., Zhang, J., Guan, D., Yang, Z., Kong, D., et al. (2013). Enzyme-controllable Delivery of Nitric Oxide from a Molecular Hydrogel. Chem. Commun. (Camb) 49 (80), 9173–9175. doi:10.1039/c3cc45666h
Gawade, P. M., Shadish, J. A., Badeau, B. A., and DeForest, C. A. (2019). Logic‐Based Delivery of Site‐Specifically Modified Proteins from Environmentally Responsive Hydrogel Biomaterials. Adv. Mat. 31 (33), 1902462–1902468. doi:10.1002/adma.201902462
Griffin, D. R., Weaver, W. M., Scumpia, P. O., Di Carlo, D., and Segura, T. (2015). Accelerated Wound Healing by Injectable Microporous Gel Scaffolds Assembled from Annealed Building Blocks. Nat. Mater 14, 737–744. doi:10.1038/nmat4294
Guo, J., Sun, H., Lei, W., Tang, Y., Hong, S., Yang, H., et al. (2019). MMP-8-Responsive Polyethylene Glycol Hydrogel for Intraoral Drug Delivery. J. Dent. Res. 98 (5), 564–571. doi:10.1177/0022034519831931
Hu, J., Zhang, G., and Liu, S. (2012). Enzyme-responsive Polymeric Assemblies, Nanoparticles and Hydrogels. Chem. Soc. Rev. 41 (18), 5933–5949. doi:10.1039/c2cs35103j
Jansen, E., Beekhof, P., Viezeliene, D., Muzakova, V., and Skalicky, J. (2015). Long-term Stability of Cancer Biomarkers in Human Serum: Biomarkers of Oxidative Stress and Redox Status, Homocysteine, CRP and the Enzymes ALT and GGT. Biomarkers Med. 9 (5), 425–432. doi:10.2217/bmm.15.14
Joshi, N., Yan, J., Levy, S., Bhagchandani, S., Slaughter, K. V., Sherman, N. E., et al. (2018). Towards an Arthritis Flare-Responsive Drug Delivery System. Nat. Commun. 9 (1275), 1275–1311. doi:10.1038/s41467-018-03691-1
Kass, L. E., and Nguyen, J. (2021). Nanocarrier-hydrogel Composite Delivery Systems for Precision Drug Release. Nanomedicine Nanobiotechnol. 14 (2), 1–23. doi:10.1002/wnan.1756
Kaul, A., Short, W. D., Wang, X., and Keswani, S. G. (2021). Hyaluronidases in Human Diseases. Int. J. Mol. Sci. 22 (6), 3204–3215. doi:10.3390/ijms22063204
Knipe, J. M., Strong, L. E., and Peppas, N. A. (2016). Enzyme- and pH-Responsive Microencapsulated Nanogels for Oral Delivery of siRNA to Induce TNF-α Knockdown in the Intestine. Biomacromolecules 17 (3), 788–797. doi:10.1021/acs.biomac.5b01518
Koss, K. M., Tsui, C., and Unsworth, L. D. (2019). Enzymatic Activity in Fractal Networks of Self-Assembling Peptides. Biomacromolecules 20 (1), 422–434. doi:10.1021/acs.biomac.8b01496
Kulkarni, K., Motamed, S., Habila, N., Perlmutter, P., Forsythe, J. S., Aguilar, M. I., et al. (2016). Orthogonal Strategy for the Synthesis of Dual-Functionalised β(3)-peptide Based Hydrogels. Chem. Commun. (Camb) 52 (24), 5844–5847. doi:10.1039/c6cc00624h
Kulkarni, K., Hung, J., Fulcher, A. J., Chan, A. H. P., Hong, A., Forsythe, J. S., et al. (2018). β3-Tripeptides Coassemble into Fluorescent Hydrogels for Serial Monitoring In Vivo. ACS Biomater. Sci. Eng. 4 (11), 3843–3847. doi:10.1021/acsbiomaterials.8b01065
Kulkarni, K., Minehan, R. L., Gamot, T., Coleman, H. A., Bowles, S., Lin, Q., et al. (2021). Esterase-Mediated Sustained Release of Peptide-Based Therapeutics from a Self-Assembled Injectable Hydrogel. ACS Appl. Mater. Interfaces 13 (49), 58279–58290. doi:10.1021/acsami.1c14150
Kwon, M. Y., Wang, C., Galarraga, J. H., Puré, E., Han, L., and Burdick, J. A. (2019). Influence of Hyaluronic Acid Modification on CD44 Binding towards the Design of Hydrogel Biomaterials. Biomaterials 222, 119451–119459. doi:10.1016/j.biomaterials.2019.119451
Lazo, M., Rubin, J., Clark, J. M., Coresh, J., Schneider, A. L. C., Ndumele, C., et al. (2015). The Association of Liver Enzymes with Biomarkers of Subclinical Myocardial Damage and Structural Heart Disease. J. Hepatol. 62, 841–847. doi:10.1016/j.jhep.2014.11.024
Li, J., and Mooney, D. J. (2016). Designing Hydrogels for Controlled Drug Delivery. Nat. Rev. Mater 1 (12), 1–17. doi:10.1038/natrevmats.2016.71
Liu, Y., Zhang, F., Long, L., Li, J., Liu, Z., Hu, C., et al. (2022). Dual-function Hydrogels with Sequential Release of GSK3β Inhibitor and VEGF Inhibit Inflammation and Promote Angiogenesis after Stroke. Chem. Eng. J. 433 (3), 133671. doi:10.1016/j.cej.2021.133671
Lyu, D., Chen, S., and Guo, W. (2018). Liposome Crosslinked Polyacrylamide/DNA Hydrogel: A Smart Controlled-Release System for Small Molecular Payloads. Small 14 (15), e1704039. doi:10.1002/smll.201704039
Maitz, M. F., Freudenberg, U., Tsurkan, M. V., Fischer, M., Beyrich, T., and Werner, C. (2013). Bio-responsive Polymer Hydrogels Homeostatically Regulate Blood Coagulation. Nat. Commun. 4 (2168), 2168–2177. doi:10.1038/ncomms3168
Mandal, A., Clegg, J. R., Anselmo, A. C., and Mitragotri, S. (2020). Hydrogels in the Clinic. Bioeng. Transl. Med. 5 (2), e10158–12. doi:10.1002/btm2.10158
McFetridge, M. L., Del Borgo, M. P., Aguilar, M.-I., and Ricardo, S. D. (2018). The Use of Hydrogels for Cell-Based Treatment of Chronic Kidney Disease. Clin. Sci. 132 (17), 1977–1994. doi:10.1042/cs20180434
Motamed, S., Del Borgo, M. P., Kulkarni, K., Habila, N., Zhou, K., Perlmutter, P., et al. (2016). A Self-Assembling β-peptide Hydrogel for Neural Tissue Engineering. Soft Matter 12 (8), 2243–2246. doi:10.1039/c5sm02902c
Park, H., Otte, A., and Park, K. (2022). Evolution of Drug Delivery Systems: From 1950 to 2020 and beyond. J. Control. Release 342, 53–65. doi:10.1016/j.jconrel.2021.12.030
Parnetti, L., Gaetani, L., Eusebi, P., Paciotti, S., Hansson, O., El-Agnaf, O., et al. (2019). CSF and Blood Biomarkers for Parkinson's Disease. Lancet Neurol. 18, 573–586. doi:10.1016/s1474-4422(19)30024-9
Purcell, B. P., Kim, I. L., Chuo, V., Guenin, T., Dorsey, S. M., and Burdick, J. A. (2014). Incorporation of Sulfated Hyaluronic Acid Macromers into Degradable Hydrogel Scaffolds for Sustained Molecule Delivery. Biomater. Sci. 2 (5), 693–702. doi:10.1039/c3bm60227c
Ruskowitz, E. R., Comerford, M. P., Badeau, B. A., and DeForest, C. A. (2019). Logical Stimuli-Triggered Delivery of Small Molecules from Hydrogel Biomaterials. Biomater. Sci. 7 (2), 542–546. doi:10.1039/c8bm01304g
Schiffer, D., Tegl, G., Heinzle, A., Sigl, E., Metcalf, D., Bowler, P., et al. (2015). Enzyme-responsive Polymers for Microbial Infection Detection. Expert Rev. Mol. Diagnostics 15 (9), 1125–1131. doi:10.1586/14737159.2015.1061935
Shepard, J. A., Huang, A., Shikanov, A., and Shea, L. D. (2010). Balancing Cell Migration with Matrix Degradation Enhances Gene Delivery to Cells Cultured Three-Dimensionally within Hydrogels. J. Control. Release 146 (1), 128–135. doi:10.1016/j.jconrel.2010.04.032
Thambi, T., Li, Y., and Lee, D. S. (2017). Injectable Hydrogels for Sustained Release of Therapeutic Agents. J. Control. Release 267, 57–66. doi:10.1016/j.jconrel.2017.08.006
Tuladhar, A., Obermeyer, J. M., Payne, S. L., Siu, R. C. W., Zand, S., Morshead, C. M., et al. (2020). Injectable Hydrogel Enables Local and Sustained Co-delivery to the Brain: Two Clinically Approved Biomolecules, Cyclosporine and Erythropoietin, Accelerate Functional Recovery in Rat Model of Stroke. Biomaterials 235, 119794. doi:10.1016/j.biomaterials.2020.119794
Varghese, O. P., Sun, W., Hilborn, J., and Ossipov, D. A. (2009). In Situ Cross-Linkable High Molecular Weight Hyaluronan-Bisphosphonate Conjugate for Localized Delivery and Cell-specific Targeting: A Hydrogel Linked Prodrug Approach. J. Am. Chem. Soc. 131 (25), 8781–8783. doi:10.1021/ja902857b
Vemula, P. K., Boilard, E., Syed, A., Campbell, N. R., Muluneh, M., Weitz, D. A., et al. (2011). On-demand Drug Delivery from Self-Assembled Nanofibrous Gels: A New Approach for Treatment of Proteolytic Disease. J. Biomed. Mat. Res. 97A (2), 103–110. doi:10.1002/jbm.a.33020
Wang, H. H., Fu, Z. G., Li, W., Li, Y. X., Zhao, L. S., Wen, L., et al. (2018). The Synthesis and Application of Nano Doxorubicin- Indocyanine Green Matrix Metalloproteinase-Responsive Hydrogel in Chemophototherapy for Head and Neck Squamous Cell Carcinoma. Int. J. Nanomedicine 14, 623–638. doi:10.2147/IJN.S191069
Wang, J., Ye, Y., Yu, J., Kahkoska, A. R., Zhang, X., Wang, C., et al. (2018). Core-Shell Microneedle Gel for Self-Regulated Insulin Delivery. ACS Nano 12 (3), 2466–2473. doi:10.1021/acsnano.7b08152
Wang, Y., Pei, Z., Feng, W., and Pei, Y. (2019). Stimuli-responsive Supramolecular Nano-Systems Based on Pillar[n]arenes and Their Related Applications. J. Mat. Chem. B 7 (48), 7656–7675. doi:10.1039/c9tb01913h
Wooster, T. J., Acquistapace, S., Mettraux, C., Donato, L., and Dekkers, B. L. (2019). Hierarchically Structured Phase Separated Biopolymer Hydrogels Create Tailorable Delayed Burst Release during Gastrointestinal Digestion. J. Colloid Interface Sci. 553, 308–319. doi:10.1016/j.jcis.2019.06.033
Wu, J., Zheng, Z., Chong, Y., Li, X., Pu, L., Tang, Q., et al. (2018). Immune Responsive Release of Tacrolimus to Overcome Organ Transplant Rejection. Adv. Mater 30 (45), e1805018. doi:10.1002/adma.201805018
Keywords: drug delivery system, enzyme-mediated controlled release, hydrogel scaffold, enzyme sensitive linker, pharmacokinetic, injectable hydrogels
Citation: Minehan RL and Del Borgo MP (2022) Controlled Release of Therapeutics From Enzyme-Responsive Biomaterials. Front. Front. Biomater. Sci. 1:916985. doi: 10.3389/fbiom.2022.916985
Received: 10 April 2022; Accepted: 31 May 2022;
Published: 27 June 2022.
Edited by:
Regine Willumeit-Römer, Helmholtz Center Hereon, GermanyReviewed by:
Nuria Oliva, Imperial College London, United KingdomJennifer Patterson, Instituto IMDEA Materiales, Spain
Copyright © 2022 Minehan and Del Borgo. This is an open-access article distributed under the terms of the Creative Commons Attribution License (CC BY). The use, distribution or reproduction in other forums is permitted, provided the original author(s) and the copyright owner(s) are credited and that the original publication in this journal is cited, in accordance with accepted academic practice. No use, distribution or reproduction is permitted which does not comply with these terms.
*Correspondence: Mark P. Del Borgo, TWFyay5kZWxib3Jnb0Btb25hc2guZWR1