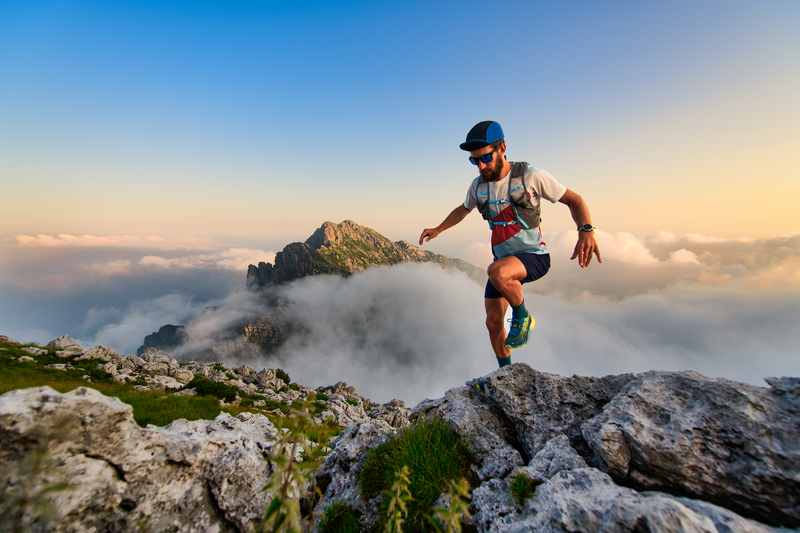
95% of researchers rate our articles as excellent or good
Learn more about the work of our research integrity team to safeguard the quality of each article we publish.
Find out more
REVIEW article
Front. Biomater. Sci. , 03 November 2022
Sec. Biomaterials Science for Regenerative Therapies
Volume 1 - 2022 | https://doi.org/10.3389/fbiom.2022.1046123
Bio-glues are gaining ground in medical research to close wounds and fight infections. Among them, the most promising bio-glue is the one prepared from natural materials (fibrin, gelatin, polysaccharides, etc.). Most of these materials are components of the extracellular matrix (ECM) and possess excellent biocompatibility, biodegradability and mechanical strength, which facilitate wound repair. However, there are no studies that utilize the decellularized materials to prepare bio-glues. Outside the wound sealants, approaches that utilize the ECM scaffold to promote tissue repair show tremendous potential. Experimentally, it is unknown if ECM can be successfully transformed to the bio-glue, either alone or in combination with nature biomaterials. In this review, we outline the first attempts at the potential of using ECM to prepare bio-glue for wound repair during the surgery.
Skin wounds or late closure of postoperative wounds can cause severe complications. For instance, wound infections, electrolyte imbalances, inflammatory reactions, and scarring in the body which will place a huge burden on patients and society (Zhao et al., 2016; Dhaliwal and Lopez, 2018; Yang et al., 2020). The common interventions for wound closure are surgical sutures (Bush and Bayat, 2007; Prabha et al., 2021). Although surgical sutures have achieved satisfactory results in the wound repair process, they are increasingly unable to meet the needs of modern clinical practice with the development of medicine. For example, wounds cannot be closed immediately with traditional closure method, which may cause secondary injury to the wound and aggravate infection during the stitch removal. Additionally, those injuries in relatively hidden locations cannot be closed effectively, which highly requires the physician’s surgical skills. Different experimental treatment strategies are under investigation to promote recovery after trauma, with bio-glue as the most common approach (Suzuki et al., 2014; Wang et al., 2018; Pandey et al., 2020; Lu et al., 2021).
Biomedical materials are used in trauma approaches to replace or restore the anatomic structure and function of damaged or missing tissues following any injury or disease by combining the topographical cues of biomaterials with cells or bioactive molecules (Tan et al., 2021). Bio-glue intended for trauma repair are often derived from particular extracellular matrix (ECM) molecules [e.g., fibrin (Kalsi et al., 2017), collagen (Schiele et al., 1992), hyaluronic acid (Luo et al., 2020)], or other natural polymers [e.g., gelatin/alginate (Song et al., 2022), chitosan (Lu et al., 2018)] or synthetic polymers [e.g., cyanoacrylate (Carvalho et al., 2021), polyurethane (Zhao et al., 2021), polyethylene glycol (Privett et al., 2021)]. While both natural and synthetic polymers are widely utilized as tissue adhesives, the use of ECM bio-glue is rarely seen (Nishiguchi and Taguchi, 2021; Yazdanpanah et al., 2022). However, ECM materials used in tissue engineering [e.g., peripheral nerves (Kim et al., 2004), lung (Ohata and Ott, 2020), kidney (Sobreiro-Almeida et al., 2021), liver (Hussein et al., 2020), skin (Wolf et al., 2012)] have shown great prospect.
In this paper, we describe the developmental trends of some tissue adhesives in the medical field and focus on the adhesion mechanism of these tissue adhesives. At the same time, we discuss the feasibility to prepare bio-glue with ECM obtained by the process of decellularization and the possibility to promote wound repair.
The ideal bio-glue is supposed to possess advantages of biocompatibility, biodegradability, fantastic mechanical strength, and adhesive strength (Peng and Shek, 2010). In recent years, researchers have developed several bio-glues using natural or synthetic materials such as fibrin adhesives, gelatin adhesives, polysaccharide adhesives, cyanoacrylate adhesives, polyethylene glycol adhesives, polyurethane adhesives and so on.
Fibrin, as a soluble glycoprotein in plasma, has been extensively studied in surgical hemostatic agents (Mandell and Gibran, 2014), tissue engineering (Li et al., 2015; Noori et al., 2017), and drug delivery systems (Spicer and Mikos, 2010) due to its unique biological performance. The typical fibrin glue consists of fibrinogen, coagulation factor XIII, calcium-containing thrombin, and antifibrinolytic components (e.g., peptides). The principle of gelation simulates the final step in the clotting cascade (Mankad and odispoti, 2001; Mooney et al., 2009) and can be summarized as follows: in the presence of thrombin, fibrinogen is converted to fibrin, and coagulation factor XIII is converted to XIIIa. Lastly, the fibrin is cross-linked in the presence of coagulation factor XIIIa.
Fibrin sealants have been used for decades as tissue adhesives for hemostasis in surgery since the first purification and preparation in the 1940s. Currently, Food and Drug Administration (FDA) has license TISSEEL®, ARTISS®, EVARREST®, FloSeal® and TachoSiL® (Alston et al., 2008; Guzzo et al., 2009; Hester et al., 2013; Kuschel et al., 2013; DeAnglis et al., 2017). Those fibrin glues are often used in combination with traditional sutures to treat severe bleeding in cardiovascular, gynecologic, urological, and orthopedic operations. In general, these products are safe and effective, and few adverse effects have been reported.
Fibrin adhesives are widely used in clinical practice, but there are still some problems that need to be solved (Beudert et al., 2022). First, they are isolated from human plasma, which makes them more expensive than synthetic materials. Second, the potential spread of diseases associated with blood products may bring severe safety risks. Moreover, fibrin adhesives with poor adhesion and insufficient mechanical strength are only used in surface wound closure, rather than in the moist physiological environment filled with blood and tissue fluid. Therefore, fibrin adhesives can only be as an adjunct in traditional wound closure methods such as sutures or staples in many surgical procedures (Mandell and Gibran, 2014).
Gelatin, a kind of macromolecule hydrophilic colloid, is the product of partial hydrolysis of collagen (an important part of ECM). At present, it has been wildly applied in bio-glue (Thirupathi Kumara Raja et al., 2013), wound dressings (Gaspar-Pintiliescu et al., 2019), and tissue engineering (Agarwal et al., 2016) because of its good biocompatibility. When prepared as a biological glue, gelatin must form a network structure through cross-linking to keep stable in a physiological environment. For instance, after reacting with aldehydes, it turns to be more cohesive.
Gelatine-Resorcine-Formaline (GRF) is one of the medical tissue adhesives based on gelatin, which consists of two parts: 1) Gelatin/resorcinol mixture; 2) Formaldehyde or formaldehyde/glutaraldehyde mixture. It is formed by the condensation polymerization of the amino of gelatin chains and aldehyde groups on resorcinol. Meanwhile, the aldehyde group is chemically bonded to the tissue surface, forming a firm connection (Elvin et al., 2010). However, the toxicity of formaldehyde limits its clinical application (Fukunaga et al., 1999; Tanaka et al., 2017).
For above shortcomings, researchers have improved the biocompatibility of gelatin glue by photo-crosslinking (Shin et al., 2016). Gelatin methacryloyl (GelMA) hydrogels are prepared by modifying gelatin with methacryloyl groups, whose adhesion performance can be optimized by adjusting the degree of substitution, prepolymer concentration, photo-initiator concentration, which are superior to fibrin glue, polyethylene glycol glue (Assmann et al., 2017). Zhao et al. (Zhao et al., 2022) developed a photocurable bio-adhesive hydrogel which was composed of gelatin methacryloyl and oxidized dextran for suture-less keratoplasty. Seung Bae Ryue et al. (Ryu et al., 2022) investigated the effect of different concentrations of graphene oxide (GO) on the physicochemical properties of gelatin hydrogels, especially on the strength of tissue adhesion, by enzyme-catalyed cross-linking of gelatin-based hydrogels. Liwei Yan et al. (Yan et al., 2022) developed a mussel-inspired tough and viscous polydopamine/gelatin-polyacrylic acid (PDA/Gel-PAA) composite hydrogel for cartilage regeneration. The hydrogel obtained a high compressive strength of up to 0.67 MPa and a toughness of 420 J/m2 with good biofunctionality.
Polysaccharide is a class of carbohydrates whose molecules contain chains of monosaccharide molecules including chitosan, chondroitin sulfate, and hyaluronic acid (Francis Suh and Matthew, 2000). The main driving forces for the polysaccharide hydrogels gelation are the intermolecular hydrogen bond and intermolecular interactions. In the preparation of polysaccharide glue, researchers tend to modify the functional groups on the chain to increase the adhesion of hydrogels because their chemical structures determine their adhesive mechanisms.
Chitosan is insoluble in neutral, alkaline pH solutions and organic solvents. To improve solubility, the method is to chemically modify chitosan to obtain derivatives such as carboxymethyl chitosan, glycol chitosan, and chitosan ester. Chitosan and its derivatives have attracted extensive attention in the field of tissue adhesives because of their excellent antibacterial properties (Jayakumar et al., 2011; Amoozgar et al., 2012; Lima et al., 2018). Wahid et al. (Wahid et al., 2017) reported a metal ion crosslinked carboxymethyl chitosan supramolecular hydrogel with good antibacterial activity against Escherichia coli and Staphylococcus aureus. Moreover, Chitosan and its derivatives also promote tissue repair (Tao et al., 2013). Glycol chitosan is modified by 3-(4-hydroxyphenyl) propionic acid to form phenolic groups and is cross-linked by blue light irradiation under the catalysis of ruthenium (Ru) to form a gel, which has the characteristic of tissue adhesion and wound repair (Lu et al., 2018). Qingcong Wei et al. (Wei et al., 2022) designed and prepared a series of good performance photoinduced Schiff base cross-linked hydrogels by Diels–Alder (DA) reaction between functional group grafted carboxymethyl chitosan (CMCS) and photosensitive polyethylene glycol (PEG) cross-linker.
Chondroitin sulfate (CS) is a sulfated polysaccharide that is widely distributed in the extracellular matrix and cell surface of animal tissues. The main chains of CS have multiple reaction sites and can be chemically modified to introduce functional groups. Researchers have developed several medical tissue adhesives based on chondroitin sulfate (Strehin et al., 2010; Han et al., 2018; Shin et al., 2021). CS is the main component of the cartilage extracellular matrix, which can maintain the functions of chondrocytes and is therefore widely used in cartilage repair. Inspired by mussel chemistry, Han et al. (Han et al., 2018) designed a polydopamine-chondroitin sulfate-polyacrylamide (PDA-CS-PAM) hydrogel with tissue adhesiveness and superb mechanical properties for growth-factor-free cartilage regeneration. Han Fu et al. (Fu et al., 2021) described a simple double cross-linking strategy for the preparation of ECM mimetic hydrogels with good overall performance by reversible borate ester bonding based on chondroitin sulfate and gelatin as a single cross-linked hydrogel. Photopolymerization with thiol-containing polyethylene glycol (PEG) crosslinkers was further enhanced by Michael addition crosslinking reactions or visible light.
Hyaluronic acid (HA), the only non-sulfated polysaccharide, is an essential component of the ECM and plays an important role in wound healing, cell motility, angiogenesis, and cell signaling (Allison and Grande-Allen, 2006). As a biomaterial, it has been studied for several decades in hemostasis dressing and anti-Inflammatory dressing (Sakoda et al., 2018; Bao et al., 2020; Kim et al., 2020; Hu et al., 2021). In the application of hemostasis, Sakoda et al. (Sakoda et al., 2018) have developed a novel hydrogel hemostat conjugated with inorganic polyphosphate (PolyP). The HA-PolyP hemostatic hydrogel was formed rapidly by mixing aldehyde-modified HA and hydrazide-modified HA conjugated with PolyP. As for its anti-inflammatory feature, Kim et al. (Kim et al., 2020) reported a tissue adhesive and anti-inflammatory hydrogel formed by high-affinity enzymatic crosslinking by polyphenolic epigallocatechin gallates (EGCGs), which may provide a robust tissue adhesive platform for clinical applications. In particular, HA hydrogel successfully closed the skin wound and displayed insignificant host tissue responses. Furthermore, the HA hydrogel exhibited the highest tissue adhesiveness both in wet and dry conditions.
Silk fibroin (SF) is a natural polymeric fibrous protein extracted from silk, containing 18 kinds of amino acids, among which glycine (Gly), alanine (Ala) and serine (Ser) account for more than 80% of the total composition. Silk fibroin has good mechanical and physicochemical properties, such as good flexibility and tensile strength, permeability and moisture, slow release, etc., and different forms like fiber, solution, powder, film and gel can be obtained after didderent treatments (Zheng and Zuo, 2021). Silk proteins have been prepared as bio-glues for clinical applications. Rumeysa Tutar et al. (Tutar et al., 2022) synthesized a biocompatible and photocross-linked ocular tissue adhesive composite hydrogel from silk fibroin for corneal injury. Zichu Yin et al. (Yin et al., 2021) prepared a dopamine-modified SF-based bioadhesive using genipin as a cross-linking agent.
Cyanoacrylate is a liquid tissue adhesive in surgery for over 60 years. It rapidly polymerizes to form sturdy chemical bonds in seconds when contacting with proteins (Bao et al., 2020). Its heat released during the polymerization process can easily cause secondary damage. Notably, the initial cyanoacrylates, mainly including methyl 2-cyanoacrylate and ethyl 2-cyanoacrylate, exhibit cytotoxicity while exhibiting strong tissue adhesion (Evans et al., 1999; Setlik et al., 2005; Sheikh, 2007; Cannata et al., 2009; Habib et al., 2013). To solve this problem, researchers improved the biocompatibility by optimizing cyanoacrylate so that it could be further used in clinical practice. At present, cyanoacrylate adhesives commonly used in clinics are octyl 2-cyanoacrylate and butyl 2-cyanoacrylate (García Cerdá et al., 2015; Lim and Kim, 2015; Kiaii et al., 2022).
The synthesis of polyurethane (PU) adhesives is based on the unique chemical properties of isocyanates, featuring high elasticity and strong adhesion. Isocyanates form strong peptide bonds by reacting with amino groups of tissue proteins (Mehdizadeh and Yang, 2013). The polymerization of N, N′ carbonylbis (caprolactam) (CBC) and polyol are catalyzed by alcoholate to form cross-linked poly (ester-urea)s through ring-opening addition reaction. Mechanical properties, biocompatibility, and degradation behavior of the CBC/polyol-based polymers make them widely used in biomedical applications (Zimmermann et al., 2004; Lin et al., 2013). Zhou et al. (Zhou et al., 2015) developed a catechol-functional polyurethane copolymer (PEUs) for sturdy adhesion and biodegradability. When they performed lap shear tests after bonding aluminum materials together, they detected a bond strength close to 1 MPa. With further addition of oxidative crosslinker, the lap shear bond strength increases to 2.4 MPa. What’s more, they are non-toxic in vitro and in vivo (Stakleff et al., 2013). Zhao et al. (Zhao et al., 2021) prepared a rapid in situ gelling of mussel polyurethane adhesives (MPUAs) under mild conditions, which showed good biocompatibility and strong adhesion to complex wounds. Faxing Zou et al. (Zou et al., 2022) prepared a novel tissue adhesive composed of L-arginine, degradable polyurethane (DLPU) and GelMA for wound repair. As far as it goes, the first FDA-approved PU medical tissue adhesive TissuGlu® is prepared from trimethylolpropane and lysine ethyl diisocyanate, which has no toxic side effects and has been used in clinical practice (Gilbert et al., 2008).
PEG tissue adhesive has the advantages of fast curing, strong adhesion, and almost no inflammatory reaction (Li et al., 2014). However, the clinical applications of PEG bio-glue are greatly limited. On the one hand, due to the high equilibrium swelling rate, it tends to cause wound dehiscence. On the other hand, the anti-protein properties of PEG itself are not conducive to cell adhesion and thus hinder wound repair (Chahal et al., 2018).
Currently, PEG tissue adhesives consist of chemically modified linear or branched PEG molecules. Bu et al. (Bu et al., 2019), introduced cyclized succinate groups into PEG branches to control the swelling rate of PEG hydrogels, while also exhibiting rapid degradation and excellent biocompatibility. Apart from this, the hydrogel has fine hemostatic ability even under anticoagulant conditions. These results reveal that the optimized hydrogel may be a facile, effective, and safe sealant for hemorrhage control in vivo (Tan et al., 2011). Matthew N George et al. (George et al., 2022) developed hydrogels with tunable expansion rate, porosity and cross-linking density by cross-linking catechol functional groups and conjugation of the synthesized oligomer oligo [poly (ethylene glycol) fumarate] (OPF).
Tissue adhesives are usually a liquid or semi-liquid materials. When applied to a tissue surface, they form a strong interfacial interaction with the tissue, thereby tightly bonding two substrate surfaces together. To date, scientists have proposed several mechanisms to explain the adhesion process of tissue adhesives. The adhesion mechanism mainly includes the formation of chemical bonds, mechanical interlocking, Gecko-inspired adsorption, electrostatic interaction, and hydrogen bond. In the actual adhesion process, most tissue adhesives mainly rely on chemical cross-linking, supplemented by other adhesion mechanisms to facilitate wound healing.
The formation of chemical bonds is an important mechanism by which bio-glues adhere to tissues. There are a large number of amino acid residues (such as mercaptan and amino) on the surface of the tissues. Once the glue is injected onto the tissue surface, various chemical bonds (such as covalent bonds, ionic bonds, metallic bonds) are formed at the interface, thereby closing the wound. Common chemical reactions between tissue and glue include Schiff base reaction and active ester reaction.
Schiff base reaction is a process in which an aldehyde group and an amino group react to form a dynamic bond (Figure 1). Researchers have designed several tissue adhesives for wound sealing by Schiff base reaction (Yang et al., 2016, 2017; Xu et al., 2018). Currently, bio-adhesives based on Schiff base reaction are usually composite bio-glue. Because composite bio-glue can compensate for the non-uniform distribution of the hydrogel network caused by Schiff base reaction.
FIGURE 1. Schiff base reaction of a schematic diagram of cross-linking mechanism. Reprinted with permission from Macromolecular Bioscience, Mohammadreza Mehdizadeh, Jian Yang, Design strategies and applications of tissue bioadhesives, Pages No. 271–288. Copyright (2013) John wiley and Sons.
Unal et al. (Unal et al., 2021) used elastic polypeptide (mELP) and methacryloyl gelatin (GelMA) to form a composite elastic tissue binder (Figures 2A,B). The Michael addition reaction between the methacryloyl olefin in the adhesive and the primary amine on the surface of the surrounding vascular tissue allow the hydrogel to adhere strongly to the skin surface (Figure 2C). The elastic GelMA/mELP composite glue attained desirable mechanical properties and adhesion to the blood vessel, while the pure GelMA glue exhibited superior adhesion at the cost of increased stiffness and reduced extensibility (Figures 2D,E). Pang et al. (Pang et al., 2020) combined chitin nanowhiskers (CtNWs) with carboxymethyl chitosan and dextran dialdehyde hydrogels by Schiff base reaction to design a mechanically enhanced tissue adhesive. What’s more, the addition of CtNWs significantly increased the tissue adhesion of the composite hydrogel.
FIGURE 2. Ex vivo endovascular anastomosis model and shear test. (A) Experimental setup. (B) 3D schematic of the proposed two glue-based systems. (C) Two carotid segments were glued together; the anastomosis procedure was complete, and a catheter was inserted (left). The glue sealed the area surrounding the anastomosis and prevented fluid leakage; the arteries could not be pulled apart with ease (center). Using this ex vivo anastomosis model, both arteries expanded up to four to six times in diameter before the glue system failed and burst (right). (D) Shear strength of the applied glues on porcine carotid arteries. (E) The maximal intra-arterial pressures were achieved with the ex vivo endovascular anastomosis model, in comparison to human systolic blood pressures at various stages. Reprinted with permission from Bioengineering & Translational Medicine, Gokberk Unal, Jesse Jones, Sevana Baghdasarian, Naoki Kaneko, Ehsan Shirzaei Sani, Sohyung Lee, Shima Gholizadeh, Satoshi Tateshima, Nasim Annabi, Engineering Elastic Sealants Based on Gelatin and Elastin-like Polypeptides for Endovascular Anastomosis. Copyright (2021) John wiley and Sons.
The active ester reaction refers to the carboxyl group on the side chain of the polymer, which reacts with the primary amine group on the tissue surface to form an amide bond after activated by N-hydroxysuccinimide (NHS) (Figure 3H).
FIGURE 3. CS-NHS reacts with primary amines of both PEG-(NH2)6 and proteins of tissue to form a covalently bound hydrogel to tissue. (A–D) Solutions of PEG-(NH2)6 (colored with blue dye) and CS-NHS (clear solution) are mixed to form a hydrogel. (E) The same hydrogel without dye (star) in a cartilage defect after 11 days of swelling in PBS. The NHS activated carboxyl groups of CS-NHS (F) react with the primary amines of PEG-(NH2)6 (G) and the primary amines of proteins in tissue (H, reactive groups circled). The new bonds that form are amide bonds. (H) The material in contact with tissue before (left) and after (right) it has reacted with primary amines. NHS = N-Hydroxysuccinimide; PEG = Poly (ethylene glycol); CS = Chondroitin Sulfate. Reprinted with permission from Biomaterials, Iossif Strehin, Zayna Nahas, Karun Arora, Thao Nguyen, Jennifer Elisseeff, A versatile pH sensitive chondroitin sulfate-PEG tissue adhesive and hydrogel, Pages No. 2788–2797. Copyright Elsevier.
Strehin et al. (Strehin et al., 2010) used NHS to activate the carboxyl group on the side chain of chondroitin sulfate (CS) to obtain CS-NHS. Then, using polyethylene glycol (PEG (NH2)6) as a cross-linking agent, a hydrogel adhesive (CS-PEG) covalently bound to proteins in tissues through amide bonds (Figures 3A–G). The adhesion strength of the material to cartilage tissue is about 10 times higher than that of fibrin adhesive and has many potential biomedical applications. Moreover, Sun et al. (Sun et al., 2020) designed a PEG/TA multifunctional adhesive that has the characteristics of injectability, instant self-healing, and durable strong adhesion, which is of great significance to wound healing.
Physical forces involve applying tissue glue to the surface of the tissues and then slowly immersing the polymer carrying water into the tissue of the object. When the macromolecules in the glue solidify, the tissues are tightly adhered together. This process can be roughly divided into three forces, van der Waals forces, hydrogen bonds, and electrostatic forces.
Mechanical interlocking means that the adhesive material penetrates through the surface and mechanically interlocks with the microscopic pores or irregularities on the substrate surface (Figure 4). Jeon et al. (Jeon et al., 2019) prepared a bilayer bonded microneedle (MN) patch in the form of a hydrogel, which consists of swollen mussel adhesion protein (MAP) and non-swollen silk fibronectin (Figure 4A). Microneedle technology can be used to fix tissues in a minimally invasive manner with a large contact area with the tissue (Figures 4D,E) (Yang et al., 2013; Ma and Wu, 2017). In experiments, smooth conical needles can be inserted into tissue in a dry (rigid) state to minimize the force required to penetrate tissues. After contacting with water, the tip expands, and the cross-sectional area increases rapidly, resulting in the mechanical interlocking and deformation of local tissue (Figures 4B,C). MN patches are adhesive to various moist tissues both in vitro and in vivo.
FIGURE 4. Fabrication of double-layered adhesive MN patches with different contents of swellable layer and tissue insertion capability. (A) Bright-field, fluorescence, and merged micrographs for various DL-MN patches that were fabricated by the double-casting method with different filling times for MAP/HA prehydrogel solution under the backside vacuum. To discriminate between the two layers, rhodamine B was loaded into the SF prehydrogel solution. Scale bar = 500 μm. (B) Compression force-displacement curves and fracture points (indicated by an arrow) for the MAP/HA SL-MN patch and 60% DL-MN patch. (C) Ultimate MN fracture forces of the MAP/HA SL-MN patch and 60% DL-MN patch obtained by the compression tests (n ≥ 5). (D) punctured rat skin after inserting the 60% DL-MN patch and exposing a red tissue-marking dye and (E) sharpness of MN tips of the 60% DL-MN patch. Scale bar = 1 mm. (For interpretation of the references to color in this figure legend, the reader is referred to the Web version of this article). Reprinted with permission from Biomaterials, Eun Young Jeon, Jungho Lee, Bum Ju Kim, Kye Il Joo, Ki Hean Kim, Geunbae Lim, Hyung Joon Cha, Bio-inspired swellable hydrogel-forming double-layered adhesive microneedle protein patch for regenerative internal/external surgical closure. Copyright (2019) Elsevier.
The gecko’s ability to quickly climb vertical surfaces inspired the researchers to uncover the underlying mechanisms behind its reversible adhesion. Experimental evidences confirm (Autumn et al., 2000; Kwak et al., 2011) that the gecko’s adhesion strategy relies on a footpad composed of setae and that the adhesion force generated by the contact between the footpad and the relative surface (caused by van der Waals forces) is sufficient for the gecko to adhere to a vertical or inverted surface. This adhesion is strong but temporary, so the gecko’s feet can quickly separate and adhere during movement. Lee et al. (Lee et al., 2007) created a hybrid tissue adhesive consisting of nanofabricated polymer arrays covered with a thin layer of synthetic polymer that mimics the mussel adhesion proteins. The adhesion of nanostructured polymer arrays can be increased nearly 15-fold in humid conditions. The system has maintained strong adhesion in the performance tests for more than 1000 contact cycles in both dry and wet conditions. It combines the characteristics of gecko and mussel adhesives for reversible adhesion to a variety of surfaces in any environment.
The molecular composition of biological tissue surfaces, with a large number of positively or negatively charges, polar or nonpolar groups is very complicated. Therefore, positively or negatively charged tissue surfaces tend to attract oppositely charged adhesives. Chitosan is a cationic polysaccharide that interacts with negatively charged tissue surfaces through electrostatic forces, but the force is very weak (Ryu et al., 2011). Based on this adhesion mechanism, scientists have improved its property by adding other polymers and then invented a composite adhesive based on chitosan.
Jung et al. (Jung et al., 2021) created chitosan/polyethylene glycol hydrogels with improved mechanical properties in situ using horseradish peroxidase (HRP) reaction. The effect of PEG on the physicochemical properties of chitosan hydrogels was revealed by different contents, molecular weights, and geometry (linear, four-armed) of PEG derivatives. Balakrishnan et al. (Balakrishnan et al., 2017) developed an in situ cured binder based on the bio-polymers chitosan and dextran. When blending the two components, the gel showed good adhesion and was non-cytotoxic. Zhou et al. (Zhou et al., 2016) used a hydrogel composed of chitosan and ε-poly-lysine to bind to neural tissues (Figures 5A,B). The hydrogel mimicked the polysaccharide/protein structure of the natural outer membrane matrix, thus enhancing compatibility with nerves (Figures 5C,D).
FIGURE 5. Schematic diagram. (A) Gelation Mechanism through Michael Addition. (B) Formation of Hydrogel Network. (C) Schematic of Interactions inside the Adhesives. (D) Schematic of Nerve Anastomosis Test. Reprinted with permission from Biomacromolecules, Yalin Zhou, Jin Zhao, Xiaolei Sun, Sidi Li, Xin Hou, Xubo Yuan, Xiaoyan Yuan, Rapid Gelling Chitosan/Polylysine Hydrogel with Enhanced Bulk Cohesive and Interfacial Adhesive Force: Mimicking Features of Epineurial Matrix for Peripheral Nerve Anastomosis, Pages No. 622–630. Copyright (2016) American Chemical Society.
Marine mussels, such as purple mussel, thick shell mussel, and halcyon mussel, fix themselves to multiple solid surfaces. Their adhesive proteins secreted by byssus can form hydrophobic bonds to resist erosion brought by wind and waves. Adhesive proteins Mfp-3 and Mfp-5 are composed of 3, 4-dihydroxyphenylalanine (Dopa) (Cha et al., 2008; Maier et al., 2015; Han et al., 2017; Lo Presti et al., 2021). Dopa is considered to be a key factor for clipping adhesion. After understanding the underwater adhesion mechanism of mussels, the researchers introduced catechol groups into the polymer to prepare bio-glue (Mehdizadeh et al., 2012; Fan et al., 2016; Ji et al., 2016; Zhu et al., 2017). Lu et al. (Lu et al., 2020) designed a bio-glue composed of citric acid, PEG-PPG-PEG diol, and dopamine. Zeng et al. (Zeng et al., 2021) achieved skin wound healing effect by incorporating polydopamine nanoparticles (PDA) into xanthan gum and konjac glucomannan. However, when Dopa is exposed to oxidants such as O2, it inevitably oxidizes to quinones, resulting in loss of adhesion. Therefore, Xu et al. (Xu et al., 2020) designed a hydrogel based on thiourea-catechol reaction to protect catechol groups from oxidation through thiourea. Compared with traditional tissue adhesives, thiourea-catechol hydrogels have higher mechanical properties, shorter curing time, less environmental PH independence, and lower oxidation concentration in the treatment of gastric ulcers. At the same time, the application of hydrogel to the ulcer site increased the content of pro-regeneration growth factors, reduced the exposure to external catabolic factors, and contributed to the cure of gastric ulcers.
The complexity of the wound and its response to injury dictates that the latest advances in bioengineering and biological materials have to be implemented in the design of bio-glue intended to promote wound tissue repair. Researchers have developed multi-therapeutic strategies, combining various elements to promote repair (Zhang et al., 2016; Gong et al., 2017; Hong et al., 2018; Saiz-Poseu et al., 2019). Bio-glue are well-positioned to deliver such combinatorial approaches for wound repair, the cell-active factor has been used in many of the strategies (Zhao et al., 2014; Guo et al., 2016; Liang et al., 2019a, 2019b; Han et al., 2019; Chen et al., 2020; Zhang et al., 2020). Biodegradability, mechanical strength, porosity, and capability of cell adhesion are some of the parameters that affect the regenerative capacity of a given design. Many issues need to be considered for the choice of biomaterial and the design of bio-glue, including:
1, Biocompatibility, minimizing adverse tissue reactions in vivo;
2, Biological function, promote wound healing in vivo;
3, Cytocompatibility, promote cell proliferation and adhesion in vivo;
4, Physical properties resemble those of the target tissues (e.g., elasticity, strength, tenacity);
5, Biodegradable and the degradation products have no cytotoxicity.
In conclusion, many tissue adhesives have been developed by researchers. Their advantages and disadvantages are summarized in Table 1.
One important point when designing bio-glue is that it is supposed to mimic the biological properties of host tissues. Soft hydrogels with high water content are becoming increasingly versatile as materials and significant in vivo data support their utilizations. For example, Hong et al. (Hong et al., 2019) laboratory developed a hydrogel with hybrid hyaluronic acid, gelatin, glycosaminoglycan to mimic a tissue ECM structure. This biomacromolecule-based matrix hydrogel can gel rapidly and adhere tightly to seal bleeding arteries and cardiac walls after UV light irradiation. These repairs can withstand up to 290 mmHg blood pressure, significantly higher than common blood pressures (systolic BP 60–160 mmHg).
Hence, the direct use of ECM by decellularization for the preparation of bio-glue may be a strategic option since the extracellular matrix is structurally similar to the tissues. It is a highly competitive bioactive material with a higher potential value than tissue adhesives of single protein or component. The ECM is mainly composed of two major groups of macromolecules: proteoglycans and fibrous proteins. The main fibrous ECM proteins are collagen, elastin, fibronectin, etc. Proteoglycans fill most of the extracellular space in the tissue in the form of hydration gel, mainly including hyaluronic acid, chondroitin sulfate, and dermal sulfate (Frantz et al., 2010). Collagen is the most abundant fibrous protein within the interstitial ECM and is most abundant in skin tissue (Kisling et al., 2019). Elastin is highly elastic and is found in connective tissues, allowing many tissues in the body to regain their original shape after stretching or contracting. It is particularly abundant in large elastic vessels such as the aorta (Kuzan et al., 2018). Hyaluronic acid is a glycosaminoglycan composed of the basic structure of disaccharides (D-glucuronic acid and N-acetylglucosamine). The molecular weight of hyaluronic acid ranges from 50 to 20 million daltons in the body. Hyaluronic acid is widely found in connective, epithelial and nervous tissues. With its unique molecular structure and physical and chemical properties, hyaluronic acid shows a variety of important physiological functions in the body, such as lubricating joints, regulating the permeability of blood vessel walls, regulating the diffusion and operation of proteins, water and electrolytes, and promoting wound healing. (Salwowska et al., 2016; Agarwal et al., 2020; Marinho et al., 2021). Chondroitin sulfate (CS) is a class of glycosaminoglycans that are covalently linked to proteins to form proteoglycans. The glycan chains are composed of alternating polymerization of glucuronic acid and N-acetylgalactosamine and are usually derived from animal cartilage (Valcarcel et al., 2017). Actually, there are many researchers on the preparation of bio-glues using ECM single components, but less reports on the preparation of bio-glues using composite ECM components. Yi Liu et al. (Liu et al., 2019) choose gelatin as the backbone of adhesive. Firstly, catechol-modified gelatin (Gel-Cat) and phenol-modified gelatin (Gel-Ph) were synthesized by conjugating dopamine and phloretic acid to the gelatin backbone via an EDC and NHS chemistry. This gelatin-based adhesives demonstrate great potential for wound closure and healing. In addition, a two-component tissue adhesive were prepared based on oxidized urethane dextran (Dex-U-AD) and gelatin by photo-crosslinking under UV irradiation (Wang et al., 2012). Hyaluronic acid and Chondroitin sulfate in extracellular matrix can also be reacted with catechol to prepare tissue glues (Zhou Y. et al., 2020; Shin et al., 2021; Zhang et al., 2021).
Bio-glue, derived from natural or synthetic materials, can be implanted into the injured tissue (Mankad M.B.B.S and odispoti, 2001; Carvalho et al., 2021; Song et al., 2022). Natural materials like fibrin, gelatin, and polysaccharides, most of which are polymers, are the main components of ECM (Frantz et al., 2010). However, there are no studies on preparation of bio-glue with ECM obtained by the process of decellularization. ECM materials that can treat wounds and promote regeneration are known as ECM-bio-glue. In this section, we will discuss the application of ECM materials to prepare glue for wound repair. So far, this natural biomaterial is of tremendous potential as a scaffold for cell growth, differentiation and tissue development, and has been applied to blood vessels (Conklin et al., 2002), skin (Chen et al., 2004), bone (Woods and Gratzer, 2005), cartilage (Cheng et al., 2009), kidney (Yi et al., 2017; Sobreiro-Almeida et al., 2021), lung (Cortiella et al., 2010), heart (Rieder et al., 2004), and peripheral nerves (Karabekmez et al., 2009) in research and industry.
Extracellular matrix is a fundamental structural support for cells, so derived or functionalized natural ECM plays a crucial role in regenerative medicine (Badylak et al., 2009). The ECM is not only a supporting material, but also a regulator of cellular behaviors such as cell survival, proliferation, morphogenesis, and differentiation (Giancotti and Ruoslahti, 1999; Nie and Wang, 2018; Urbanczyk et al., 2020). In addition, ECM can regulate signal transduction activated by a variety of biologically active molecules including growth factors and cytokines (Comoglio et al., 2003). ECM molecules and networks are able to change their compositions, structures, and biomechanical properties according to different tissues and organs (Rauch, 2007). Ideally, ECM materials for wound repair can not only provide the same or similar local microenvironment in vivo, but also recruit cells and even induce cell-directed differentiation. In the process of damage repair, ECM degradation should reach a balance with tissue regeneration.
Traditional bio-glues have been widely used, but they do not fully mimic ECM structures. In addition, the component of traditional glue is single, while the ECM is a combination of several proteins, growth factors, and other cytokines, with a wide range of biological functions. Therefore, ECM seems to have potential in the preparation of bio-glues.
According to some of the adhesion mechanisms mentioned in Part 3 (Schiff base reactions, active ester reactions, and hydrogen bonds), natural adhesives can chemically and physically interact with tissue surfaces to form tight bonds. Among these, the glycoproteins in the ECM are composed of core proteins and polysaccharide chains (glycosaminoglycans) (Frantz et al., 2010), which consist of a series of disaccharide repeating units (acetyl-galactosamine, glucuronic acid, iduronic acid, galactose, etc.). The disaccharide repeating units can be further divided into sulfates (chondroitin sulfate, heparin sulfate, and keratin sulfate) and hyaluronic acid. At present, researchers have prepared adhesive hydrogels by modified chitosan, hyaluronic acid, and other polysaccharides substances (Jayakumar et al., 2011; Lu et al., 2018; Kim et al., 2020). Hence, these polysaccharide chains can be chemically modified (Dopa, catechol group) to endow the ECM with adhesion function. In addition, elastin is abundant in tissues (e.g., blood vessel, lung, heart, skin, bladder) and can be chemically modified to give it adhesion properties. Brennan et al. (Brennan et al., 2017) designed an elastin bio-glue that was prokaryotically expressed from E. coli. The main ingredient, ELY16, can aggregate under the influence of environmental factors such as temperature, pH, and salinity. Besides, dopamine modifies collagen, endowing the decellularized extracellular matrix (dECM) hydrogel with adhesion function. Catia Correia et al. (Correia et al., 2022) described the development of a bio-adhesive membrane using a Marine renewable biomaterial, collagen extracted from fish skin. Collagen was functionalized with catechol group (Col-Cat), which made the membrane have good adhesion property in wet environment, and was mixed with chitosan, which improved the mechanical properties of the membrane.
By way of conclusion, the dECM can be chemically modified with catechol group/oxidized urethane dextran/lysine and imparted with adhesion properties (Matsumura et al., 2014; Ma et al., 2021). Hence, it is feasible to prepare ECM-bio-glue by chemically (Dopa, catechol group) modifying (Figures 6A,B). It also exhibits features such as antibacterial properties, high mechanical strength, and cytocompatibility, overall making it a very promising tissue adhesive. However, the ECM derived from a variety of tissue types is composed of multiple proteins, growth factors and cytokines (Frantz et al., 2010; Theocharis et al., 2016). The decellularization protocols lack specificity in view of the complexity of healing (Choudhury et al., 2020). It is difficult to remove growth-inhibiting molecules while retaining growth-promoting molecules. What’s more, it is very difficult to do the precise chemical modifications of ECM. These reasons above restrict the development of ECM-Bio-glue.
FIGURE 6. Model diagram of ECM preparation of biological glue. (A) ECM is chemically modified by DOPA. (B) ECM bio-glue is used for wound repair.
The ECM is composed of non-cellular components of tissue that provide a complex three-dimensional network environment for cell survival. Recent studies have shown that ECM also provides tissue-specific biochemical and biophysical cues that are required for tissue morphogenesis and homeostasis (Nelson and Bissell, 2006; Badylak et al., 2009; Karamanos, 2019). In the field of regenerative medicine, dECM matrices have played an important role as scaffolds for tissue engineering. The dECM scaffolds are obtained by treating tissues with detergents to removing cells and immunogenicity, subsequently refilling the dECM scaffold with host cells and allowing them to be transplanted into the host (Yi et al., 2017). For example (Tian et al., 2021), prepared porcine articular cartilage derived ECM, could achieve good cartilage regeneration in a rat knee osteochondral defect model. Biomaterials have played a key role in our current understanding of the contribution of matrix properties to cellular responses. Previous studies have focused on decellularized materials to promote wound repair. Interestingly, most researchers believe that maintaining a moist environment on the wound surface is crucial to promoting wound healing. And hydrogel, a highly hydrophilic material, is an ideal choice. Among different forms of biomaterials, beyond dECM scaffolds, the dECM hydrogels have been widely used, because they provide a highly hydrated, cytocompatible environment and superior printability (Sobreiro-Almeida et al., 2021). Currently, scientists are already trying to exploit this advantage by mimicking the structure of the ECM with natural materials. Many researchers have used natural substances (hyaluronic acid/gelatin/glycosaminoglycans) to simulate the structure/composition of the dECM to produce bio-glues or 3D-printed artificial tissues/organs, but this does not fully mimic the structure and composition of the dECM (Pati et al., 2014; Huang et al., 2016; Hong et al., 2019). dECM has a very powerful biological function and plays a positive role in wound repair. Its advantages are unquestionable. However, is dECM available for the preparation of bio-glue? The main components of ECM include collagen, hyaluronic acid, chondroitin sulfate and so on. These substances are the main components in the preparation of natural bio-glues, so the dECM is possible to be chemically modified to endow it an adhesive function. The scope of application of chemically modified dECM has been expanded. In the future, it is believed that ECM-bio-glue can show their favorable prospect. The finding that these ECM materials can be dissolved and subsequently form hydrogels expands their potential for use in vitro and in vivo. In addition to this, hydrogels are also beneficial to filling irregular shaped defects (Saldin et al., 2017).
ECM hydrogels are considered the most promising alternative materials due to their excellent swelling properties and similarity to soft tissues. Various strategies have been applied to synthesize bionic hydrogels whose biophysical and biochemical properties have been tuned to suit cell differentiation (Huang et al., 2017; Boso et al., 2020). Recently, a variety of polymers have been used alone or in combination to create hydrogels designed for biomedical applications in the treatment of wounds (Ge et al., 2020; Stoica et al., 2020). However, there is no research on using ECM to prepare bio-glue for damage repair. Present studies investigated the potential of ECM hydrogels (e.g., laminin, fibronectin, and collagen), which promote recovery after injury. However, all of these strategies are early attempts and only give a hint on the potential of ECM-bio-glue. If we can endow ECM hydrogels with adhesive properties, it will greatly expand the scope of applications of ECM, for example, artificial skin. At present, the materials commonly used in the preparation of artificial skin include gelatin (Wang et al., 2006), gelatin/chitosan (Chang et al., 2008), gelatin/alginate (Yan et al., 2005), gelatin/fibrinogen (Xu et al., 2007), alginate (Fedorovich et al., 2012). Fei-fei Zhou et al. (Zhou F. et al., 2020) developed a biomimetic bioink composed of GelMA and HA-NB with phenyl Li-2,4,6 trimethylbenzoyl phosphate (LAP) as polymerization initiator for DLP-based 3D printing. GelMA/HA-NB/LAP bioink was selected in part because of its rapid gelation properties and structural similarity to native ECM, which can provide a beneficial microenvironment for cell growth and tissue regeneration. In addition, GelMA/HA-NB/LAP hydrogel can also be used as an adhesive to “immobilize” living organs during living transplants, as it can bind tightly to tissues through the Schiff base reaction. However, there are some concerns about the results of these studies, such as the use of harsh cross-linking agents, like glutaraldehyde (Chang et al., 2008), which increase cytotoxicity. Furthermore, these materials do not fully mimic the complexity of native ECMs and are therefore insufficient to reconstruct the microenvironment of organization. As a result, the cells in these hydrogels cannot exhibit the morphology and function inherent to living tissues. Consequently, it is ideal to provide cells with a natural microenvironment similar to their natural tissues. dECM is the best choice to achieve this goal, as no natural or man-made material can reproduce all the features of a natural ECM. However, dECM materials also have disadvantages, such as inability to cure quickly/poor mechanical properties/lack of adhesion, which require chemical modification to compensate.
Compared with traditional wound treatment technology, medical tissue adhesives have many advantages, such as simple operation, non-invasive adhesion, rapid hemostasis, and effective shortening of surgery time. However, there are still many challenges to be overcome for the development of medical tissue adhesives. ECM is a complex three-dimensional network structure, which plays a vital role in the treatment of wound repair and provides new targets for clinical research. In the past decades, studies of ECM macromolecules and intertwined networks have made great progress. From a physiological and pathological perspective, ECM plays a regulatory role in tissue integrity, cell signal transduction, gene expression, and cell behavior. A number of ECM materials have been successfully commercialized for the treatment of various diseases.
In this review, we provide some clues whether ECM can be used to prepare bio-glue. It is believed that with the continuous development of materials science and the unremitting efforts of researchers, the future of medical tissue adhesives will be brighter. Research on novel ECM biomaterials deserves more attention.
Writing—original draft preparation, YZ; writing—review and editing, LT; project administration, JM and ST. All authors have read and agreed to the published version of the manuscript.
This research was funded by the National Natural Science Foundation of China (Grant NO. 81970653), and National key R&D Program of China (Grant NO. 2021YFF0700300).
The authors declare that the research was conducted in the absence of any commercial or financial relationships that could be construed as a potential conflict of interest.
All claims expressed in this article are solely those of the authors and do not necessarily represent those of their affiliated organizations, or those of the publisher, the editors and the reviewers. Any product that may be evaluated in this article, or claim that may be made by its manufacturer, is not guaranteed or endorsed by the publisher.
Agarwal, G., Agiwal, S., and Srivastava, A. (2020). Hyaluronic acid containing scaffolds ameliorate stem cell function for tissue repair and regeneration. Int. J. Biol. Macromol. 165, 388–401. doi:10.1016/j.ijbiomac.2020.09.107
Agarwal, T., Narayan, R., Maji, S., Behera, S., Kulanthaivel, S., Maiti, T. K., et al. (2016). Gelatin/Carboxymethyl chitosan based scaffolds for dermal tissue engineering applications. Int. J. Biol. Macromol. 93, 1499–1506. doi:10.1016/j.ijbiomac.2016.04.028
Allison, D. D., and Grande-Allen, K. J. (2006). Review. Hyaluronan: A powerful tissue engineering tool. Tissue Eng. 12, 2131–2140. doi:10.1089/ten.2006.12.2131
Alston, S. M., Solen, K. A., Sukavaneshvar, S., and Fazal Mohammad, S. (2008). In vivo efficacy of a new autologous fibrin sealant. J. Surg. Res. 146, 143–148. doi:10.1016/j.jss.2007.08.006
Amoozgar, Z., Rickett, T., Park, J., Tuchek, C., Shi, R., and Yeo, Y. (2012). Semi-interpenetrating network of polyethylene glycol and photocrosslinkable chitosan as an in-situ-forming nerve adhesive. Acta Biomater. 8, 1849–1858. doi:10.1016/j.actbio.2012.01.022
Assmann, A., Vegh, A., Ghasemi-Rad, M., Bagherifard, S., Cheng, G., Sani, E. S., et al. (2017). A highly adhesive and naturally derived sealant. Biomaterials 140, 115–127. doi:10.1016/j.biomaterials.2017.06.004
Autumn, K., Liang, Y. A., Hsieh, S. T., Zesch, W., Chan, W. P., Kenny, T. W., et al. (2000). Adhesive force of a single gecko foot-hair. Nature 405, 681–685. doi:10.1038/35015073
Badylak, S., Freytes, D., and Gilbert, T. (2009). Extracellular matrix as a biological scaffold material: Structure and function. Acta Biomater. 5, 1–13. doi:10.1016/j.actbio.2008.09.013
Balakrishnan, B., Soman, D., Payanam, U., Laurent, A., Labarre, D., and Jayakrishnan, A. (2017). A novel injectable tissue adhesive based on oxidized dextran and chitosan. Acta Biomater. 53, 343–354. doi:10.1016/j.actbio.2017.01.065
Bao, Z., Gao, M., Sun, Y., Nian, R., and Xian, M. (2020). The recent progress of tissue adhesives in design strategies, adhesive mechanism and applications. Mater. Sci. Eng. C 111, 110796. doi:10.1016/j.msec.2020.110796
Beudert, M., Gutmann, M., Lühmann, T., and Meinel, L. (2022). Fibrin sealants: Challenges and solutions. ACS Biomater. Sci. Eng. 8, 2220–2231. doi:10.1021/acsbiomaterials.1c01437
Boso, D., Maghin, E., Carraro, E., Giagante, M., Pavan, P., and Piccoli, M. (2020). Extracellular matrix-derived hydrogels as biomaterial for different skeletal muscle tissue replacements. Materials 13, 2483. doi:10.3390/ma13112483
Brennan, M. J., Kilbride, B. F., Wilker, J. J., and Liu, J. C. (2017). A bioinspired elastin-based protein for a cytocompatible underwater adhesive. Biomaterials 124, 116–125. doi:10.1016/j.biomaterials.2017.01.034
Bu, Y., Zhang, L., Sun, G., Sun, F., Liu, J., Yang, F., et al. (2019). Tetra‐PEG based hydrogel sealants for in vivo visceral hemostasis. Adv. Mat. 31, 1901580. doi:10.1002/adma.201901580
Bush, J., and Bayat, A. (2007). Surgical instruments, sutures and suturing techniques. Br. J. Hosp. Med. (Lond). 68, M142–M145. doi:10.12968/hmed.2007.68.Sup8.24511
Cannata, A., Taglieri, C., Russo, C. F., Bruschi, G., and Martinelli, L. (2009). Use of CoSeal in a patient with a left ventricular assist device. Ann. Thorac. Surg. 87, 1956–1958. doi:10.1016/j.athoracsur.2008.10.042
Carvalho, C., Marinho, A. S., Barbosa-Sequeira, J., Correia, M. R., Carvalho, F., Banquart-Leitão, J., et al. (2021). Pediatric burns with cyanoacrylate glue: An inconspicuous danger. J. Burn Care Res. 42, 1047–1049. doi:10.1093/jbcr/irab063
Cha, H. J., Hwang, D. S., and Lim, S. (2008). Development of bioadhesives from marine mussels. Biotechnol. J. 3, 631–638. doi:10.1002/biot.200700258
Chahal, A. S., Schweikle, M., Heyward, C. A., and Tiainen, H. (2018). Attachment and spatial organisation of human mesenchymal stem cells on poly(ethylene glycol) hydrogels. J. Mech. Behav. Biomed. Mater. 84, 46–53. doi:10.1016/j.jmbbm.2018.04.025
Chang, R., Nam, J., and Sun, W. (2008). Effects of dispensing pressure and nozzle diameter on cell survival from solid freeform fabrication–based direct cell writing. Tissue Eng. Part A 14, 41–48. doi:10.1089/ten.a.2007.0004
Chen, R.-N., Ho, H.-O., Tsai, Y.-T., and Sheu, M.-T. (2004). Process development of an acellular dermal matrix (ADM) for biomedical applications. Biomaterials 25, 2679–2686. doi:10.1016/j.biomaterials.2003.09.070
Chen, Y., Wu, L., Li, P., Hao, X., Yang, X., Xi, G., et al. (2020). Polysaccharide based hemostatic strategy for ultrarapid hemostasis. Macromol. Biosci. 20, 1900370. doi:10.1002/mabi.201900370
Cheng, N.-C., Estes, B. T., Awad, H. A., and Guilak, F. (2009). Chondrogenic differentiation of adipose-derived adult stem cells by a porous scaffold derived from native articular cartilage extracellular matrix. Tissue Eng. Part A 15, 231–241. doi:10.1089/ten.tea.2008.0253
Choudhury, D., Yee, M., Sheng, Z. L. J., Amirul, A., and Naing, M. W. (2020). Decellularization systems and devices: State-of-the-art. Acta Biomater. 115, 51–59. doi:10.1016/j.actbio.2020.07.060
Comoglio, P. M., Boccaccio, C., and Trusolino, L. (2003). Interactions between growth factor receptors and adhesion molecules: Breaking the rules. Curr. Opin. Cell Biol. 15, 565–571. doi:10.1016/S0955-0674(03)00096-6
Conklin, B. S., Richter, E. R., Kreutziger, K. L., Zhong, D.-S., and Chen, C. (2002). Development and evaluation of a novel decellularized vascular xenograft. Med. Eng. Phys. 24, 173–183. doi:10.1016/S1350-4533(02)00010-3
Correia, C., Sousa, R. O., Vale, A. C., Peixoto, D., Silva, T. H., Reis, R. L., et al. (2022). Adhesive and biodegradable membranes made of sustainable catechol-functionalized marine collagen and chitosan. Colloids Surfaces B Biointerfaces 213, 112409. doi:10.1016/j.colsurfb.2022.112409
Cortiella, J., Niles, J., Cantu, A., Brettler, A., Pham, A., Vargas, G., et al. (2010). Influence of acellular natural lung matrix on murine embryonic stem cell differentiation and tissue formation. Tissue Eng. Part A 16, 2565–2580. doi:10.1089/ten.tea.2009.0730
DeAnglis, A. P., Nur, I., Gorman, A. J., and Meidler, R. (2017). A method to measure thrombin activity in a mixture of fibrinogen and thrombin powders. Blood Coagul. Fibrinolysis 28, 134–138. doi:10.1097/MBC.0000000000000560
Dhaliwal, K., and Lopez, N. (2018). Hydrogel dressings and their application in burn wound care. Br. J. Community Nurs. 23, S24–S27. doi:10.12968/bjcn.2018.23.Sup9.S24
Elvin, C. M., Vuocolo, T., Brownlee, A. G., Sando, L., Huson, M. G., Liyou, N. E., et al. (2010). A highly elastic tissue sealant based on photopolymerised gelatin. Biomaterials 31, 8323–8331. doi:10.1016/j.biomaterials.2010.07.032
Evans, C. E., Lees, G. C., and Trail, I. A. (1999). Cytotoxicity of cyanoacrylate adhesives to cultured tendon cells. J. Hand Surg. 24, 658–661. doi:10.1054/JHSB.1999.0279
Fan, C., Fu, J., Zhu, W., and Wang, D.-A. (2016). A mussel-inspired double-crosslinked tissue adhesive intended for internal medical use. Acta Biomater. 33, 51–63. doi:10.1016/j.actbio.2016.02.003
Fedorovich, N. E., Schuurman, W., Wijnberg, H. M., Prins, H.-J., van Weeren, P. R., Malda, J., et al. (2012). Biofabrication of osteochondral tissue equivalents by printing topologically defined, cell-laden hydrogel scaffolds. Tissue Eng. Part C. Methods 18, 33–44. doi:10.1089/ten.tec.2011.0060
Francis Suh, J.-K., and Matthew, H. W. T. (2000). Application of chitosan-based polysaccharide biomaterials in cartilage tissue engineering: A review. Biomaterials 21, 2589–2598. doi:10.1016/S0142-9612(00)00126-5
Frantz, C., Stewart, K. M., and Weaver, V. M. (2010). The extracellular matrix at a glance. J. Cell Sci. 123, 4195–4200. doi:10.1242/jcs.023820
Fu, H., Yu, C., Li, X., Bao, H., Zhang, B., Chen, Z., et al. (2021). Facile engineering of ECM-mimetic injectable dual crosslinking hydrogels with excellent mechanical resilience, tissue adhesion, and biocompatibility. J. Mat. Chem. B 9, 10003–10014. doi:10.1039/D1TB01914G
Fukunaga, S., Karck, M., Harringer, W., Cremer, J., Rhein, C., and Haverich, A. (1999). The use of gelatin-resorcin-formalin glue in acute aortic dissection type Aq. Thorac. Surg. 7.
García Cerdá, D., Ballester, A. M., Aliena-Valero, A., Carabén-Redaño, A., and Lloris, J. M. (2015). Use of cyanoacrylate adhesives in general surgery. Surg. Today 45, 939–956. doi:10.1007/s00595-014-1056-4
Gaspar-Pintiliescu, A., Stanciuc, A.-M., and Craciunescu, O. (2019). Natural composite dressings based on collagen, gelatin and plant bioactive compounds for wound healing: A review. Int. J. Biol. Macromol. 138, 854–865. doi:10.1016/j.ijbiomac.2019.07.155
Ge, B., Wang, H., Li, J., Liu, H., Yin, Y., Zhang, N., et al. (2020). Comprehensive assessment of nile Tilapia skin (Oreochromis niloticus) collagen hydrogels for wound dressings. Mar. Drugs 18, 178. doi:10.3390/md18040178
George, M. N., Liu, X., Miller, A. L., Zuiker, E., Xu, H., and Lu, L. (2022). Injectable pH-responsive adhesive hydrogels for bone tissue engineering inspired by the underwater attachment strategy of marine mussels. Biomater. Adv. 133, 112606. doi:10.1016/j.msec.2021.112606
Giancotti, F. G., and Ruoslahti, E. (1999). Integrin signaling. Science 285, 1028–1033. doi:10.1126/science.285.5430.1028
Gilbert, T. W., Badylak, S. F., Gusenoff, J., Beckman, E. J., Clower, D. M., Daly, P., et al. (2008). Lysine-derived urethane surgical adhesive prevents seroma formation in a canine abdominoplasty model. Plastic Reconstr. Surg. 122, 95–102. doi:10.1097/PRS.0b013e31817743b8
Gong, C., Shan, M., Li, B., and Wu, G. (2017). Injectable dual redox responsive diselenide-containing poly(ethylene glycol) hydrogel: Injectable dual redox responsive diselenide. J. Biomed. Mat. Res. 105, 2451–2460. doi:10.1002/jbm.a.36103
Guo, J., Wang, W., Hu, J., Xie, D., Gerhard, E., Nisic, M., et al. (2016). Synthesis and characterization of anti-bacterial and anti-fungal citrate-based mussel-inspired bioadhesives. Biomaterials 85, 204–217. doi:10.1016/j.biomaterials.2016.01.069
Guzzo, T. J., Pollock, R. A., Forney, A., Aggarwal, P., Matlaga, B. R., and Allaf, M. E. (2009). Safety and efficacy of a surgeon-prepared gelatin hemostatic agent compared with FloSeal for hemostasis in laparoscopic partial nephrectomy. J. Endourology 23, 279–282. doi:10.1089/end.2008.0535
Habib, A., Mehanna, A., and Medra, A. (2013). Cyanoacrylate: A handy tissue glue in maxillofacial surgery: Our experience in alexandria, Egypt. J. Maxillofac. Oral Surg. 12, 243–247. doi:10.1007/s12663-012-0433-z
Han, L., Lu, X., Liu, K., Wang, K., Fang, L., Weng, L.-T., et al. (2017). Mussel-inspired adhesive and tough hydrogel based on nanoclay confined dopamine polymerization. ACS Nano 11, 2561–2574. doi:10.1021/acsnano.6b05318
Han, L., Wang, M., Li, P., Gan, D., Yan, L., Xu, J., et al. (2018). Mussel-inspired tissue-adhesive hydrogel based on the polydopamine–chondroitin sulfate complex for growth-factor-free cartilage regeneration. ACS Appl. Mat. Interfaces 10, 28015–28026. doi:10.1021/acsami.8b05314
Han, X., Meng, G., Wang, Q., Cui, L., Wang, H., Wu, J., et al. (2019). Mussel-inspired in situ forming adhesive hydrogels with anti-microbial and hemostatic capacities for wound healing. J. Biomater. Appl. 33, 915–923. doi:10.1177/0885328218810552
Hester, T. R., Shire, J. R., Nguyen, D. B., Gerut, Z. E., Chen, A. H., Diamond, J., et al. (2013). Randomized, controlled, phase 3 study to evaluate the safety and efficacy of fibrin sealant VH S/D 4 s-apr (artiss) to improve tissue adherence in subjects undergoing rhytidectomy. Aesthet. Surg. J. 33, 487–496. doi:10.1177/1090820X13479969
Hong, S. H., Kim, S., Park, J. P., Shin, M., Kim, K., Ryu, J. H., et al. (2018). Dynamic bonds between boronic acid and alginate: Hydrogels with stretchable, self-healing, stimuli-responsive, remoldable, and adhesive properties. Biomacromolecules 19, 2053–2061. doi:10.1021/acs.biomac.8b00144
Hong, Y., Zhou, F., Hua, Y., Zhang, X., Ni, C., Pan, D., et al. (2019). A strongly adhesive hemostatic hydrogel for the repair of arterial and heart bleeds. Nat. Commun. 10, 2060. doi:10.1038/s41467-019-10004-7
Hu, M., Yang, J., and Xu, J. (2021). Structural and biological investigation of chitosan/hyaluronic acid with silanized-hydroxypropyl methylcellulose as an injectable reinforced interpenetrating network hydrogel for cartilage tissue engineering. Drug Deliv. 28, 607–619. doi:10.1080/10717544.2021.1895906
Huang, Q., Zou, Y., Arno, M. C., Chen, S., Wang, T., Gao, J., et al. (2017). Hydrogel scaffolds for differentiation of adipose-derived stem cells. Chem. Soc. Rev. 46, 6255–6275. doi:10.1039/C6CS00052E
Huang, S., Yao, B., Xie, J., and Fu, X. (2016). 3D bioprinted extracellular matrix mimics facilitate directed differentiation of epithelial progenitors for sweat gland regeneration. Acta Biomater. 32, 170–177. doi:10.1016/j.actbio.2015.12.039
Hussein, K. H., Park, K-M., Yu, L., Kwak, H.-H., and Woo, H.-M. (2020). Decellularized hepatic extracellular matrix hydrogel attenuates hepatic stellate cell activation and liver fibrosis. Mater. Sci. Eng. C 116, 111160. doi:10.1016/j.msec.2020.111160
Jayakumar, R., Prabaharan, M., Sudheesh Kumar, P. T., Nair, S. V., and Tamura, H. (2011). Biomaterials based on chitin and chitosan in wound dressing applications. Biotechnol. Adv. 29, 322–337. doi:10.1016/j.biotechadv.2011.01.005
Jeon, E. Y., Lee, J., Kim, B. J., Joo, K. I., Kim, K. H., Lim, G., et al. (2019). Bio-inspired swellable hydrogel-forming double-layered adhesive microneedle protein patch for regenerative internal/external surgical closure. Biomaterials 222, 119439. doi:10.1016/j.biomaterials.2019.119439
Ji, Y., Ji, T., Liang, K., and Zhu, L. (2016). Mussel-inspired soft-tissue adhesive based on poly(diol citrate) with catechol functionality. J. Mat. Sci. Mat. Med. 27, 30. doi:10.1007/s10856-015-5649-2
Jung, H. Y., Le Thi, P., HwangBo, K.-H., Bae, J. W., and Park, K. D. (2021). Tunable and high tissue adhesive properties of injectable chitosan based hydrogels through polymer architecture modulation. Carbohydr. Polym. 261, 117810. doi:10.1016/j.carbpol.2021.117810
Kalsi, P., Thom, M., and Choi, D. (2017). Histological effects of fibrin glue and synthetic tissue glues on the spinal cord: Are they safe to use? Br. J. Neurosurg. 31, 695–700. doi:10.1080/02688697.2017.1359491
Karabekmez, F. E., Duymaz, A., and Moran, S. L. (2009). Early clinical outcomes with the use of decellularized nerve allograft for repair of sensory defects within the hand. Hand (New York, N. Y.) 4, 245–249. doi:10.1007/s11552-009-9195-6
Karamanos, N. K. (2019). Extracellular matrix: Key structural and functional meshwork in health and disease. FEBS J. 286, 2826–2829. doi:10.1111/febs.14992
Kiaii, B., Johnston, S. S., Jang, S. R., Elangovanraaj, N., Tewari, P., and Chen, B. P.-H. (2022). Clinical and economic outcomes after sternotomy for cardiac surgery with skin closure through 2-octyl cyanoacrylate plus polymer mesh tape versus absorbable sutures plus waterproof wound dressings: A retrospective cohort study. J. Cardiothorac. Surg. 17, 212. doi:10.1186/s13019-022-01956-x
Kim, B.-S., Yoo, J. J., and Atala, A. (2004). Peripheral nerve regeneration using acellular nerve grafts. J. Biomed. Mat. Res. 68A, 201–209. doi:10.1002/jbm.a.10045
Kim, S.-H., Kim, K., Kim, B. S., An, Y.-H., Lee, U.-J., Lee, S.-H., et al. (2020). Fabrication of polyphenol-incorporated anti-inflammatory hydrogel via high-affinity enzymatic crosslinking for wet tissue adhesion. Biomaterials 242, 119905. doi:10.1016/j.biomaterials.2020.119905
Kisling, A., Lust, R. M., and Katwa, L. C. (2019). What is the role of peptide fragments of collagen I and IV in health and disease? Life Sci. 228, 30–34. doi:10.1016/j.lfs.2019.04.042
Kuschel, T. J., Gruszka, A., Hermanns-Sachweh, B., Elyakoubi, J., Sachweh, J. S., Vázquez-Jiménez, J. F., et al. (2013). Prevention of postoperative pericardial adhesions with TachoSil. Ann. Thorac. Surg. 95, 183–188. doi:10.1016/j.athoracsur.2012.08.057
Kuzan, A., Chwiłkowska, A., Maksymowicz, K., Bronowicka-Szydełko, A., Stach, K., Pezowicz, C., et al. (2018). Advanced glycation end products as a source of artifacts in immunoenzymatic methods. Glycoconj. J. 35, 95–103. doi:10.1007/s10719-017-9805-4
Kwak, M. K., Jeong, H.-E., and Suh, K. Y. (2011). Rational design and enhanced biocompatibility of a dry adhesive medical skin patch. Adv. Mat. 23, 3949–3953. doi:10.1002/adma.201101694
Lee, H., Lee, B. P., and Messersmith, P. B. (2007). A reversible wet/dry adhesive inspired by mussels and geckos. Nature 448, 338–341. doi:10.1038/nature05968
Li, C., Wang, T., Hu, L., Wei, Y., Liu, J., Mu, X., et al. (2014). Photocrosslinkable bioadhesive based on dextran and PEG derivatives. Mater. Sci. Eng. C 35, 300–306. doi:10.1016/j.msec.2013.10.032
Li, Y., Meng, H., Liu, Y., and Lee, B. P. (2015). Fibrin gel as an injectable biodegradable scaffold and cell carrier for tissue engineering. Sci. World J. 2015, 1–10. doi:10.1155/2015/685690
Liang, Y., Zhao, X., Hu, T., Chen, B., Yin, Z., Ma, P. X., et al. (2019a). Adhesive hemostatic conducting injectable composite hydrogels with sustained drug release and photothermal antibacterial activity to promote full‐thickness skin regeneration during wound healing. Small 15, 1900046. doi:10.1002/smll.201900046
Liang, Y., Zhao, X., Hu, T., Han, Y., and Guo, B. (2019b). Mussel-inspired, antibacterial, conductive, antioxidant, injectable composite hydrogel wound dressing to promote the regeneration of infected skin. J. Colloid Interface Sci. 556, 514–528. doi:10.1016/j.jcis.2019.08.083
Lim, J. I., and Kim, J. H. (2015). Enhanced biocompatibility and adhesive properties of modified allyl 2-cyanoacrylate-based elastic bio-glues. Colloids Surfaces B Biointerfaces 133, 19–23. doi:10.1016/j.colsurfb.2015.05.004
Lima, D. B., Almeida, R. D., Pasquali, M., Borges, S. P., Fook, M. L., and Lisboa, H. M. (2018). Physical characterization and modeling of chitosan/peg blends for injectable scaffolds. Carbohydr. Polym. 189, 238–249. doi:10.1016/j.carbpol.2018.02.045
Lin, F., Yu, J., Tang, W., Zheng, J., Defante, A., Guo, K., et al. (2013). Peptide-functionalized oxime hydrogels with tunable mechanical properties and gelation behavior. Biomacromolecules 14, 3749–3758. doi:10.1021/bm401133r
Liu, Y., Cheong Ng, S., Yu, J., and Tsai, W.-B. (2019). Modification and crosslinking of gelatin-based biomaterials as tissue adhesives. Colloids Surfaces B Biointerfaces 174, 316–323. doi:10.1016/j.colsurfb.2018.10.077
Lo Presti, M., Rizzo, G., Farinola, G. M., and Omenetto, F. G. (2021). Bioinspired biomaterial composite for all‐water‐based High2010Performance adhesives. Adv. Sci. (Weinh). 8, 2004786. doi:10.1002/advs.202004786
Lu, M., Liu, Y., Huang, Y.-C., Huang, C.-J., and Tsai, W.-B. (2018). Fabrication of photo-crosslinkable glycol chitosan hydrogel as a tissue adhesive. Carbohydr. Polym. 181, 668–674. doi:10.1016/j.carbpol.2017.11.097
Lu, X., Shi, S., Li, H., Gerhard, E., Lu, Z., Tan, X., et al. (2020). Magnesium oxide-crosslinked low-swelling citrate-based mussel-inspired tissue adhesives. Biomaterials 232, 119719. doi:10.1016/j.biomaterials.2019.119719
Lu, Y., Dai, W., Huang, J., Chen, X., and Yao, Y. (2021). A biomimetic glue protein modulates hepatic gene expression. Macromol. Biosci. 21, 2000303. doi:10.1002/mabi.202000303
Luo, J., Liu, C., Wu, J., Zhao, D., Lin, L., Fan, H., et al. (2020). In situ forming gelatin/hyaluronic acid hydrogel for tissue sealing and hemostasis. J. Biomed. Mat. Res. 108, 790–797. doi:10.1002/jbm.b.34433
Ma, C., Sun, J., Li, B., Feng, Y., Sun, Y., Xiang, L., et al. (2021). Ultra-strong bio-glue from genetically engineered polypeptides. Nat. Commun. 12, 3613. doi:10.1038/s41467-021-23117-9
Ma, G., and Wu, C. (2017). Microneedle, bio-microneedle and bio-inspired microneedle: A review. J. Control. Release 251, 11–23. doi:10.1016/j.jconrel.2017.02.011
Maier, G. P., Rapp, M. V., Waite, J. H., Israelachvili, J. N., and Butler, A. (2015). Adaptive synergy between catechol and lysine promotes wet adhesion by surface salt displacement. SCIENCE 349, 628–632. doi:10.1126/science.aab0556
Mandell, S. P., and Gibran, N. S. (2014). Fibrin sealants: Surgical hemostat, sealant and adhesive. Expert Opin. Biol. Ther. 14, 821–830. doi:10.1517/14712598.2014.897323
Mankad, P. S., and odispoti, M. C. (2001). The role of fibrin sealants in hemostasis. Am. J. Surg. 182, S21–S28. doi:10.1016/S0002-9610(01)00773-5
Marinho, A., Nunes, C., and Reis, S. (2021). Hyaluronic acid: A key ingredient in the therapy of inflammation. Biomolecules 11, 1518. doi:10.3390/biom11101518
Matsumura, K., Nakajima, N., Sugai, H., and Hyon, S.-H. (2014). Self-degradation of tissue adhesive based on oxidized dextran and poly-l-lysine. Carbohydr. Polym. 113, 32–38. doi:10.1016/j.carbpol.2014.06.073
Mehdizadeh, M., Weng, H., Gyawali, D., Tang, L., and Yang, J. (2012). Injectable citrate-based mussel-inspired tissue bioadhesives with high wet strength for sutureless wound closure. Biomaterials 33, 7972–7983. doi:10.1016/j.biomaterials.2012.07.055
Mehdizadeh, M., and Yang, J. (2013). Design strategies and applications of tissue bioadhesives. Macromol. Biosci. 13, 271–288. doi:10.1002/mabi.201200332
Mooney, E., Loh, C., and Pu, L. L. Q. (2009). The use of fibrin glue in plastic surgery. Plastic Reconstr. Surg. 124, 989–992. doi:10.1097/PRS.0b013e3181b039a3
Nelson, C. M., and Bissell, M. J. (2006). Of extracellular matrix, scaffolds, and signaling: Tissue architecture regulates development, homeostasis, and cancer. Annu. Rev. Cell Dev. Biol. 22, 287–309. doi:10.1146/annurev.cellbio.22.010305.104315
Nie, X., and Wang, D.-A. (2018). Decellularized orthopaedic tissue-engineered grafts: Biomaterial scaffolds synthesised by therapeutic cells. Biomater. Sci. 6, 2798–2811. doi:10.1039/C8BM00772A
Nishiguchi, A., and Taguchi, T. (2021). A pH-driven genipin gelator to engineer decellularized extracellular matrix-based tissue adhesives. Acta Biomater. 131, 211–221. doi:10.1016/j.actbio.2021.06.033
Noori, A., Ashrafi, S. J., Vaez-Ghaemi, R., Hatamian-Zaremi, A., and Webster, T. J. (2017). A review of fibrin and fibrin composites for bone tissue engineering. Int. J. Nanomedicine 12, 4937–4961. doi:10.2147/IJN.S124671
Ohata, K., and Ott, H. C. (2020). Human-scale lung regeneration based on decellularized matrix scaffolds as a biologic platform. Surg. Today 50, 633–643. doi:10.1007/s00595-020-02000-y
Pandey, N., Soto-Garcia, L. F., Liao, J., Zimmern, P., Nguyen, K. T., and Hong, Y. (2020). Mussel-inspired bioadhesives in healthcare: Design parameters, current trends, and future perspectives. Biomater. Sci. 8, 1240–1255. doi:10.1039/C9BM01848D
Pang, J., Bi, S., Kong, T., Luo, X., Zhou, Z., Qiu, K., et al. (2020). Mechanically and functionally strengthened tissue adhesive of chitin whisker complexed chitosan/dextran derivatives based hydrogel. Carbohydr. Polym. 237, 116138. doi:10.1016/j.carbpol.2020.116138
Pati, F., Jang, J., Ha, D.-H., Won Kim, S., Rhie, J.-W., Shim, J.-H., et al. (2014). Printing three-dimensional tissue analogues with decellularized extracellular matrix bioink. Nat. Commun. 5, 3935. doi:10.1038/ncomms4935
Peng, H. T., and Shek, P. N. (2010). Novel wound sealants: Biomaterials and applications. Expert Rev. Med. Devices 7, 639–659. doi:10.1586/erd.10.40
Prabha, S., Sowndarya, J., Ram, P. J. V. S., Rubini, D., Hari, B. N. V., Aruni, W., et al. (2021). Chitosan-coated surgical sutures prevent adherence and biofilms of mixed microbial communities. Curr. Microbiol. 78, 502–512. doi:10.1007/s00284-020-02306-7
Privett, B. J., Perini, M. V., Weinberg, L., Fink, M. A., Muralidharan, V., Lee, E., et al. (2021). Reduction in post‐operative pancreatic fistula with polyethylene glycol and recombinant human albumin sealant following stapled distal pancreatectomy. ANZ J. Surg. 91, 2459–2465. doi:10.1111/ans.17181
Rauch, U. (2007). Brain matrix: Structure, turnover and necessity. Biochem. Soc. Trans. 35, 656–660. doi:10.1042/BST0350656
Rieder, E., Kasimir, M.-T., Silberhumer, G., Seebacher, G., Wolner, E., Simon, P., et al. (2004). Decellularization protocols of porcine heart valves differ importantly in efficiency of cell removal and susceptibility of the matrix to recellularization with human vascular cells. J. Thorac. Cardiovasc. Surg. 127, 399–405. doi:10.1016/j.jtcvs.2003.06.017
Ryu, J. H., Lee, Y., Kong, W. H., Kim, T. G., Park, T. G., and Lee, H. (2011). Catechol-functionalized chitosan/pluronic hydrogels for tissue adhesives and hemostatic materials. Biomacromolecules 12, 2653–2659. doi:10.1021/bm200464x
Ryu, S. B., Park, K. M., and Park, K. D. (2022). In situ graphene oxide-gelatin hydrogels with enhanced mechanical property for tissue adhesive and regeneration. Biochem. Biophysical Res. Commun. 592, 24–30. doi:10.1016/j.bbrc.2022.01.010
Saiz-Poseu, J., Mancebo-Aracil, J., Nador, F., Busqué, F., and Ruiz-Molina, D. (2019). The chemistry behind catechol-based adhesion. Angew. Chem. Int. Ed. 58, 696–714. doi:10.1002/anie.201801063
Sakoda, M., Kaneko, M., Ohta, S., Qi, P., Ichimura, S., Yatomi, Y., et al. (2018). Injectable hemostat composed of a polyphosphate-conjugated hyaluronan hydrogel. Biomacromolecules 19, 3280–3290. doi:10.1021/acs.biomac.8b00588
Saldin, L. T., Cramer, M. C., Velankar, S. S., White, L. J., and Badylak, S. F. (2017). Extracellular matrix hydrogels from decellularized tissues: Structure and function. Acta Biomater. 49, 1–15. doi:10.1016/j.actbio.2016.11.068
Salwowska, N. M., Bebenek, K. A., Żądło, D. A., and Wcisło-Dziadecka, D. L. (2016). Physiochemical properties and application of hyaluronic acid: A systematic review. J. Cosmet. Dermatol. 15, 520–526. doi:10.1111/jocd.12237
Schiele, U., Kuntz, G., and Riegler, A. (1992). Haemostyptic preparations on the basis of collagen alone and as fixed combination with fibrin glue. Clin. Mater. 9, 169–177. doi:10.1016/0267-6605(92)90097-D
Setlik, D. E., Seldomridge, D. L., Adelman, R. A., Semchyshyn, T. M., and Afshari, N. A. (2005). The effectiveness of isobutyl cyanoacrylate tissue adhesive for the treatment of corneal perforations. Am. J. Ophthalmol. 140, 920–921. doi:10.1016/j.ajo.2005.04.062
Sheikh, B. Y. (2007). Efficacy of acrylate tissue adhesive as vascular repair and hemostatic material. Ann. Vasc. Surg. 21, 56–60. doi:10.1016/j.avsg.2006.08.001
Shin, J., Kang, E. H., Choi, S., Jeon, E. J., Cho, J. H., Kang, D., et al. (2021). Tissue-adhesive chondroitin sulfate hydrogel for cartilage reconstruction. ACS Biomater. Sci. Eng. 7, 4230–4243. doi:10.1021/acsbiomaterials.0c01414
Shin, S. R., Zihlmann, C., Akbari, M., Assawes, P., Cheung, L., Zhang, K., et al. (2016). Reduced graphene oxide‐GelMA hybrid hydrogels as scaffolds for cardiac tissue engineering. Small 12, 3677–3689. doi:10.1002/smll.201600178
Sobreiro-Almeida, R., Gómez-Florit, M., Quinteira, R., Reis, R. L., Gomes, M. E., and Neves, N. M. (2021). Decellularized kidney extracellular matrix bioinks recapitulate renal 3D microenvironment in vitro. Biofabrication 13, 045006. doi:10.1088/1758-5090/ac0fca
Song, Y., Xu, L., Xu, L., and Deng, L. (2022). Radiation cross-linked gelatin/sodium alginate/carboxymethylcellulose sodium hydrogel for the application as debridement glue paste. Polym. Bull. 79, 725–742. doi:10.1007/s00289-020-03525-5
Spicer, P. P., and Mikos, A. G. (2010). Fibrin glue as a drug delivery system. J. Control. Release 148, 49–55. doi:10.1016/j.jconrel.2010.06.025
Stakleff, K. S., Lin, F., Smith Callahan, L. A., Wade, M. B., Esterle, A., Miller, J., et al. (2013). Resorbable, amino acid-based poly(ester urea)s crosslinked with osteogenic growth peptide with enhanced mechanical properties and bioactivity. Acta Biomater. 9, 5132–5142. doi:10.1016/j.actbio.2012.08.035
Stoica, A. E., Chircov, C., and Grumezescu, A. M. (2020). Hydrogel dressings for the treatment of burn wounds: An up-to-date overview. Materials 13, 2853. doi:10.3390/ma13122853
Strehin, I., Nahas, Z., Arora, K., Nguyen, T., and Elisseeff, J. (2010). A versatile pH sensitive chondroitin sulfate–PEG tissue adhesive and hydrogel. Biomaterials 31, 2788–2797. doi:10.1016/j.biomaterials.2009.12.033
Sun, F., Bu, Y., Chen, Y., Yang, F., Yu, J., and Wu, D. (2020). An injectable and instant self-healing medical adhesive for wound sealing. ACS Appl. Mat. Interfaces 12, 9132–9140. doi:10.1021/acsami.0c01022
Suzuki, S., Masuda, M., and Imoto, K. (2014). The use of surgical glue in acute type A aortic dissection. Gen. Thorac. Cardiovasc. Surg. 62, 207–213. doi:10.1007/s11748-013-0343-0
Tan, C., Utley, M., Paschalides, C., Pilling, J., Robb, J. D., Harrison-Phipps, K. M., et al. (2011). A prospective randomized controlled study to assess the effectiveness of CoSeal® to seal air leaks in lung surgery. Eur. J. Cardiothorac. Surg. 40, 304–308. doi:10.1016/j.ejcts.2010.11.079
Tan, S., Wang, Y., Du, Y., Xiao, Y., and Zhang, S. (2021). Injectable bone cement with magnesium-containing microspheres enhances osteogenesis via anti-inflammatory immunoregulation. Bioact. Mater. 6, 3411–3423. doi:10.1016/j.bioactmat.2021.03.006
Tanaka, Y., Kitamura, T., Miyata, Y., and Miyaji, K. (2017). Mitral valve necrosis after cardiac surgery using gelatin-resorcinol-formaldehyde glue. Ann. Thorac. Surg. 103, e435–e436. doi:10.1016/j.athoracsur.2016.10.011
Tao, S., Gao, S., Zhou, Y., Cao, M., Xie, W., Zheng, H., et al. (2013). Preparation of carboxymethyl chitosan sulfate for improved cell proliferation of skin fibroblasts. Int. J. Biol. Macromol. 54, 160–165. doi:10.1016/j.ijbiomac.2012.12.009
Theocharis, A. D., Skandalis, S. S., Gialeli, C., and Karamanos, N. K. (2016). Extracellular matrix structure. Adv. Drug Deliv. Rev. 97, 4–27. doi:10.1016/j.addr.2015.11.001
Thirupathi Kumara Raja, S., Thiruselvi, T., Sailakshmi, G., Ganesh, S., and Gnanamani, A. (2013). Rejoining of cut wounds by engineered gelatin–keratin glue. Biochimica Biophysica Acta (BBA) - General Subj. 1830, 4030–4039. doi:10.1016/j.bbagen.2013.04.009
Tian, G., Jiang, S., Li, J., Wei, F., Li, X., Ding, Y., et al. (2021). Cell-free decellularized cartilage extracellular matrix scaffolds combined with interleukin 4 promote osteochondral repair through immunomodulatory macrophages: In vitro and in vivo preclinical study. Acta Biomater. 127, 131–145. doi:10.1016/j.actbio.2021.03.054
Tutar, R., Yüce-Erarslan, E., İzbudak, B., and Bal-Öztürk, A. (2022). Photocurable silk fibroin-based tissue sealants with enhanced adhesive properties for the treatment of corneal perforations. J. Mat. Chem. B 10, 2912–2925. doi:10.1039/D1TB02502C
Unal, G., Jones, J., Baghdasarian, S., Kaneko, N., Shirzaei Sani, E., Lee, S., et al. (2021). Engineering elastic sealants based on gelatin and elastin‐like polypeptides for endovascular anastomosis. Bioeng. Transl. Med. 6, e10240. doi:10.1002/btm2.10240
Urbanczyk, M., Layland, S. L., and Schenke-Layland, K. (2020). The role of extracellular matrix in biomechanics and its impact on bioengineering of cells and 3D tissues. Matrix Biol. 85 (86), 1–14. doi:10.1016/j.matbio.2019.11.005
Valcarcel, J., Novoa-Carballal, R., Pérez-Martín, R. I., Reis, R. L., and Vázquez, J. A. (2017). Glycosaminoglycans from marine sources as therapeutic agents. Biotechnol. Adv. 35, 711–725. doi:10.1016/j.biotechadv.2017.07.008
Wahid, F., Wang, H.-S., Zhong, C., and Chu, L.-Q. (2017). Facile fabrication of moldable antibacterial carboxymethyl chitosan supramolecular hydrogels cross-linked by metal ions complexation. Carbohydr. Polym. 165, 455–461. doi:10.1016/j.carbpol.2017.02.085
Wang, T., Nie, J., and Yang, D. (2012). Dextran and gelatin based photocrosslinkable tissue adhesive. Carbohydr. Polym. 90, 1428–1436. doi:10.1016/j.carbpol.2012.07.011
Wang, X., Yan, Y., Pan, Y., Xiong, Z., Liu, H., Cheng, J., et al. (2006). Generation of three-dimensional hepatocyte/gelatin structures with rapid prototyping system. Tissue Eng. 12, 83–90. doi:10.1089/ten.2006.12.83
Wang, Y., Fu, Y., Li, J., Mu, Y., Zhang, X., Zhang, K., et al. (2018). Multifunctional chitosan/dopamine/diatom-biosilica composite beads for rapid blood coagulation. Carbohydr. Polym. 200, 6–14. doi:10.1016/j.carbpol.2018.07.065
Wei, Q., Wang, Y., Wang, H., Qiao, L., Jiang, Y., Ma, G., et al. (2022). Photo-induced adhesive carboxymethyl chitosan-based hydrogels with antibacterial and antioxidant properties for accelerating wound healing. Carbohydr. Polym. 278, 119000. doi:10.1016/j.carbpol.2021.119000
Wolf, M. T., Daly, K. A., Brennan-Pierce, E. P., Johnson, S. A., Carruthers, C. A., D’Amore, A., et al. (2012). A hydrogel derived from decellularized dermal extracellular matrix. Biomaterials 33, 7028–7038. doi:10.1016/j.biomaterials.2012.06.051
Woods, T., and Gratzer, P. F. (2005). Effectiveness of three extraction techniques in the development of a decellularized bone–anterior cruciate ligament–bone graft. Biomaterials 26, 7339–7349. doi:10.1016/j.biomaterials.2005.05.066
Xu, W, Wang, X, Yan, Y, Zheng, W, Xiong, Z, Lin, F, et al. (2007). Rapid prototyping three-dimensional cell/gelatin/fibrinogen constructs for medical regeneration. J. Bioact. Compatible Polym. 22, 363–377. doi:10.1177/0883911507079451
Xu, X., Xia, X., Zhang, K., Rai, A., Li, Z., Zhao, P., et al. (2020). Bioadhesive hydrogels demonstrating pH-independent and ultrafast gelation promote gastric ulcer healing in pigs. Sci. Transl. Med. 12, eaba8014. doi:10.1126/scitranslmed.aba8014
Xu, Y., Li, Y., Chen, Q., Fu, L., Tao, L., and Wei, Y. (2018). Injectable and self-healing chitosan hydrogel based on imine bonds: Design and therapeutic applications. Int. J. Mol. Sci. 19, 2198. doi:10.3390/ijms19082198
Yan, L., Zhou, T., Ni, R., Jia, Z., Jiang, Y., Guo, T., et al. (2022). Adhesive gelatin-catechol complex reinforced poly(acrylic acid) hydrogel with enhanced toughness and cell affinity for cartilage regeneration. ACS Appl. Bio Mat. 5 4366–4377. doi:10.1021/acsabm.2c00533
Yan, Y., Wang, X., Xiong, Z., Liu, H., Liu, F., Lin, F., et al. (2005). Direct construction of a three-dimensional structure with cells and hydrogel. J. Bioact. Compatible Polym. 20, 259–269. doi:10.1177/0883911505053658
Yang, S. Y., O’Cearbhaill, E. D., Sisk, G. C., Park, K. M., Cho, W. K., Villiger, M., et al. (2013). A bio-inspired swellable microneedle adhesive for mechanical interlocking with tissue. Nat. Commun. 4, 1702. doi:10.1038/ncomms2715
Yang, X., Ren, H., Guo, X., Hu, C., and Fu, J. (2020). Radiation-induced skin injury: Pathogenesis, treatment, and management. aging 12, 23379–23393. doi:10.18632/aging.103932
Yang, Y., Liu, X., Li, Y., Wang, Y., Bao, C., Chen, Y., et al. (2017). A postoperative anti-adhesion barrier based on photoinduced imine-crosslinking hydrogel with tissue-adhesive ability. Acta Biomater. 62, 199–209. doi:10.1016/j.actbio.2017.08.047
Yang, Y., Zhang, J., Liu, Z., Lin, Q., Liu, X., Bao, C., et al. (2016). Tissue-integratable and biocompatible photogelation by the imine crosslinking reaction. Adv. Mat. 28, 2724–2730. doi:10.1002/adma.201505336
Yazdanpanah, G., Shen, X., Nguyen, T., Anwar, K. N., Jeon, O., Jiang, Y., et al. (2022). A light‐curable and tunable extracellular matrix hydrogel for in situ Suture2010Free corneal repair. Adv. Funct. Mat. 32, 2113383. doi:10.1002/adfm.202113383
Yi, S., Ding, F., Gong, L., and Gu, X. (2017). Extracellular matrix scaffolds for tissue engineering and regenerative medicine. Curr. Stem Cell Res. Ther. 12, 233–246. doi:10.2174/1574888X11666160905092513
Yin, Z., Liu, H., Lin, M., Xie, W., Yang, X., and Cai, Y. (2021). Controllable performance of a dopamine-modified silk fibroin-based bio-adhesive by doping metal ions. Biomed. Mat. 16, 045025. doi:10.1088/1748-605X/ac0087
Zeng, Q., Qian, Y., Huang, Y., Ding, F., Qi, X., and Shen, J. (2021). Polydopamine nanoparticle-dotted food gum hydrogel with excellent antibacterial activity and rapid shape adaptability for accelerated bacteria-infected wound healing. Bioact. Mater. 6, 2647–2657. doi:10.1016/j.bioactmat.2021.01.035
Zhang, D., Xu, Z., Li, H., Fan, C., Cui, C., Wu, T., et al. (2020). Fabrication of strong hydrogen-bonding induced coacervate adhesive hydrogels with antibacterial and hemostatic activities. Biomater. Sci. 8, 1455–1463. doi:10.1039/C9BM02029B
Zhang, W., Bao, B., Jiang, F., Zhang, Y., Zhou, R., Lu, Y., et al. (2021). Promoting oral mucosal wound healing with a hydrogel adhesive based on a phototriggered S‐nitrosylation coupling reaction. Adv. Mat. 33, 2105667. doi:10.1002/adma.202105667
Zhang, Y., Zhang, J., Chen, M., Gong, H., Thamphiwatana, S., Eckmann, L., et al. (2016). A bioadhesive nanoparticle–hydrogel hybrid system for localized antimicrobial drug delivery. ACS Appl. Mat. Interfaces 8, 18367–18374. doi:10.1021/acsami.6b04858
Zhao, P., Deng, C., Xu, H., Tang, X., He, H., Lin, C., et al. (2014). Fabrication of photo-crosslinked chitosan- gelatin scaffold in sodium alginate hydrogel for chondrocyte culture. Biomed. Mat. Eng. 24, 633–641. doi:10.3233/BME-130851
Zhao, R., Liang, H., Clarke, E., Jackson, C., and Xue, M. (2016). Inflammation in chronic wounds. Int. J. Mol. Sci. 17, 2085. doi:10.3390/ijms17122085
Zhao, X., Li, S., Du, X., Li, W., Wang, Q., He, D., et al. (2022). Natural polymer-derived photocurable bioadhesive hydrogels for sutureless keratoplasty. Bioact. Mater. 8, 196–209. doi:10.1016/j.bioactmat.2021.07.001
Zhao, X., Ming, H., Wang, Y., Luo, F., Li, Z., Li, J., et al. (2021). Mussel-inspired, injectable polyurethane tissue adhesives demonstrate in situ gel formation under mild conditions. ACS Appl. Bio Mat. 4, 5352–5361. doi:10.1021/acsabm.1c00451
Zheng, H., and Zuo, B. (2021). Functional silk fibroin hydrogels: Preparation, properties and applications. J. Mat. Chem. B 9, 1238–1258. doi:10.1039/D0TB02099K
Zhou, F., Hong, Y., Liang, R., Zhang, X., Liao, Y., Jiang, D., et al. (2020a). Rapid printing of bio-inspired 3D tissue constructs for skin regeneration. Biomaterials 258, 120287. doi:10.1016/j.biomaterials.2020.120287
Zhou, J., Defante, A. P., Lin, F., Xu, Y., Yu, J., Gao, Y., et al. (2015). Adhesion properties of catechol-based biodegradable amino acid-based poly(ester urea) copolymers inspired from mussel proteins. Biomacromolecules 16, 266–274. doi:10.1021/bm501456g
Zhou, Y., Kang, L., Yue, Z., Liu, X., and Wallace, G. G. (2020b). Composite tissue adhesive containing catechol-modified hyaluronic acid and poly- l -lysine. ACS Appl. Bio Mat. 3, 628–638. doi:10.1021/acsabm.9b01003
Zhou, Y., Zhao, J., Sun, X., Li, S., Hou, X., Yuan, X., et al. (2016). Rapid gelling chitosan/polylysine hydrogel with enhanced Bulk cohesive and interfacial adhesive force: Mimicking features of epineurial matrix for peripheral nerve anastomosis. Biomacromolecules 17, 622–630. doi:10.1021/acs.biomac.5b01550
Zhu, W., Peck, Y., Iqbal, J., and Wang, D.-A. (2017). A novel DOPA-albumin based tissue adhesive for internal medical applications. Biomaterials 147, 99–115. doi:10.1016/j.biomaterials.2017.09.016
Zimmermann, J., Loontjens, T., Scholtens, B. J. R., and Mülhaupt, R. (2004). The formation of poly(ester–urea) networks in the absence of isocyanate monomers. Biomaterials 25, 2713–2719. doi:10.1016/j.biomaterials.2003.09.110
Keywords: bio-glues, extracellular matrix (ECM), decellularized materials, wound repair, surgery
Citation: Zhou Y, Tang L, Mei J and Tao S (2022) Using extracellular matrix as the bio-glue for wound repair in the surgery. Front. Front. Biomater. Sci. 1:1046123. doi: 10.3389/fbiom.2022.1046123
Received: 21 September 2022; Accepted: 24 October 2022;
Published: 03 November 2022.
Edited by:
Shabir Hassan, Khalifa University, United Arab EmiratesReviewed by:
Chengchen Guo, Westlake University, ChinaCopyright © 2022 Zhou, Tang, Mei and Tao. This is an open-access article distributed under the terms of the Creative Commons Attribution License (CC BY). The use, distribution or reproduction in other forums is permitted, provided the original author(s) and the copyright owner(s) are credited and that the original publication in this journal is cited, in accordance with accepted academic practice. No use, distribution or reproduction is permitted which does not comply with these terms.
*Correspondence: Jin Mei, bWVpamluQHdtdS5lZHUuY24=; Shengxiang Tao, em4tdGFvc2hlbmd4aWFuZ0AxNjMuY29t
†These authors have contributed equally to this work
Disclaimer: All claims expressed in this article are solely those of the authors and do not necessarily represent those of their affiliated organizations, or those of the publisher, the editors and the reviewers. Any product that may be evaluated in this article or claim that may be made by its manufacturer is not guaranteed or endorsed by the publisher.
Research integrity at Frontiers
Learn more about the work of our research integrity team to safeguard the quality of each article we publish.