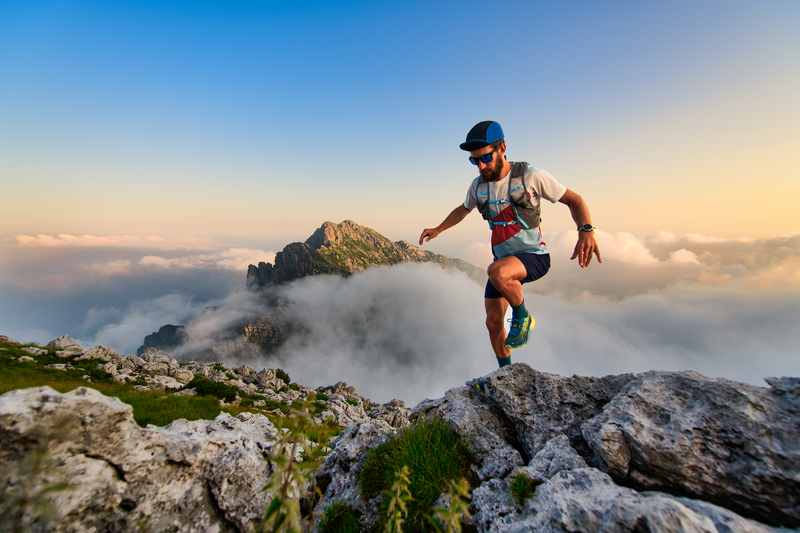
95% of researchers rate our articles as excellent or good
Learn more about the work of our research integrity team to safeguard the quality of each article we publish.
Find out more
ORIGINAL RESEARCH article
Front. Bioeng. Biotechnol. , 20 March 2025
Sec. Biomaterials
Volume 13 - 2025 | https://doi.org/10.3389/fbioe.2025.1566946
This article is part of the Research Topic Advanced Functional Materials for Disease Diagnosis, Drug Delivery and Tissue Repair View all 16 articles
Introduction: Sonodynamic therapy (SDT) is an innovative way to treat tumors by activating sonosensitizers via ultrasound (US). The development of sonosensitizers with high sonodynamic activity is the key to promote the clinical application of SDT.
Methods: In this study, a novel sonosensitizer, La2(WO4)3/CuWO4 composite LC-10, was prepared by two-step hydrothermal method and characterized. In addition, the sonodynamic antitumor activity of La2(WO4)3/CuWO4 composite LC-10 was investigated using u251 glioma cells as a model.
Results and Discussion: The results showed that compared with La2(WO4)3 and CuWO4, La2(WO4)3/CuWO4 composite had better sonodynamic antitumor activity, and LC-10 had good biosafety at concentrations below 50 μg/mL. After La2(WO4)3 and CuWO4 formed La2(WO4)3/CuWO4 composite, the recombination of electron-hole (e−–h+) pairs were effectively inhibited, and more strongly oxidizing ROS was produced, inducing apoptosis of u251 glioma cells. In which, singlet oxygen (1O2) and hydroxyl radical (·OH), especially the production of ⋅OH, played an important role in the La2(WO4)3/CuWO4 composite mediated SDT antitumor process. The results of this study would offer a foundation for the design of CuWO4 base nano-sonosensitizers and its further clinical application in SDT antitumor. In addition, it also provided a new strategy for the design and development of novel nano-sonosensitizers with excellent sonodynamic activity.
Sonodynamic therapy (SDT) is an emerging non-invasive cancer treatment derived from photodynamic therapy (PDT) (Canavese et al., 2018). SDT uses the focusing of ultrasound (US) and strong penetration of biological tissues to enrich tumor sites of sonosensitizers with certain frequency and intensity of US, and activates the sonosensitizers to produce cytotoxicity, thus producing antitumor effects (Rosenthal et al., 2004). In 1993, Umemura et al. (Umemura et al., 1993) named this method of combining sonosensitizer with US for tumor treatment as SDT according to the name of PDT. This method not only gets rid of the disadvantage of PDT’s poor tissue permeability (Hachimine et al., 2007a), but also shows unique advantages in the non-invasive treatment of deep tumors due to its local cytotoxic effect, which can minimize the damage of normal tissues around the tumor site (Yang et al., 2021). Therefore, SDT has attracted wide attention since it was proposed (Han et al., 2018; Wu et al., 2022).
According to the action mechanisms of SDT, the performance of sonosensitizers plays a crucial role in the SDT process. The traditional organic sonosensitizers used in early studies mainly include hematoporphyrin (Hp) (Umemura et al., 1989) and its derivatives such as ATX-70 (Sasaki et al., 1998), DCPH-P-Na(I) (Hachimine et al., 2007b), haematoporphyrin monomethyl ether (HMME) (Li et al., 2008) and protoporphyrin IX (PPIX) (Liu et al., 2006). In addition, some antitumor drugs and small molecule drugs such as curcumin (Jiang et al., 2020), acridine orange (Xing et al., 2021), methylene blue (He et al., 2015a), promethazine hydrochloride (He et al., 2011a), dioxypromethazine hydrochloride (He et al., 2011b), eosin B (He et al., 2015b), brilliant cresyl blue (Wang et al., 2018), toluidine blue and azure A (Qian et al., 2024) have also been proved to have good sonodynamic activity. The advantages of these organic sonosensitizers are that they all have clear chemical structures and excellent biodegradation rates. However, most of them have disadvantages such as greater hydrophobicity and phototoxicity, as well as lower tissue selectivity and stability when applied in vivo (Wu et al., 2022; Xing et al., 2021).
In recent decades, the research of inorganic nanoparticle based sonosensitizers such as titanium dioxide (TiO2) has made great progress (Yang et al., 2021). Compared with organic small molecule drugs, inorganic nanomaterials have the advantages of excellent physical and chemical properties, easy manufacturing, low phototoxicity, good biocompatibility and stability (Yang et al., 2021; Li et al., 2023). At the same time, various inorganic nanomaterials such as TiH1.924 (Gong et al., 2020), BaTiO3 (Zhu et al., 2020) and Bi2MoO6 (Dong et al., 2021) were proved to have good sonodynamic activity. These inorganic nano-sonosensitizers absorb the energy generated by the US cavitation effect (thermal and sonoluminescence) and excite the sonosensitizers to produce electron-hole (e−–h+) separation. The separated e− and h+ migrate to the surface of the sonosensitizer to produce corresponding reduction and oxidation reactions, resulting in a large number of reactive oxygen species (ROS) generation and antitumor effects (Li et al., 2023). However, due to the rapid recombination of e− and h+ in the band structure, the efficiency of ROS generation of single-component sonosensitizer is relatively low, which affects the effect of SDT (Li et al., 2023; Ping et al., 2023). Therefore, the researchers overcome the recombination of carriers by forming oxygen defect layers on the surface of sonosensitizer (Zhou et al., 2022; Wang Y. et al., 2023), deposition of precious metals (Zhang et al., 2021), ion doping (Cheng et al., 2024), construction of heterojunction (Chen et al., 2022; Kang et al., 2022) and a combination of these strategies (Zhang et al., 2023a; Song et al., 2022), thus further improving the efficiency of SDT.
Because of the effective conversion of light energy into thermal and chemical energy, tungstate nanomaterials are often used as photoresponsive materials in the field of photocatalytic degradation of organic pollutants (Kumar et al., 2022), photothermal therapy (Xiao et al., 2016), PDT and radiotherapy (Zhang et al., 2018). CuWO4 is an important semiconductor material with a band gap of about 2.60 eV (Liu et al., 2023). Compared with other tungstate structures, CuWO4 exhibits stronger absorption in the near infrared region, indicating that CuWO4 is a good candidate for PDT. For example, Cui et al. (2021) prepared a single original nanostructured CuWO4 nanodots, and introduced the nanodots into tumor tissue to generate ROS to generate PDT under 808 nm light irradiation, and released copper ions into the acidic tumor microenvironment to promote Fenton-like reaction and generate chemodynamic therapy, which can effectively inhibit tumor tissue growth. Since most sonosensitizers are derived from photosensitizers, CuWO4 nanomaterials may be a candidate sonosensitizer for SDT applications.
The construction of heterojunction can effectively inhibit the e−–h+ pairs recombination, promote the generation of ROS, and significantly improve the effect of SDT (Ping et al., 2023; Chen et al., 2022; Kang et al., 2022). Therefore, in this paper, La2(WO4)3/CuWO4 composites were prepared by two-step hydrothermal method, and the microstructure, morphology and elemental composition of La2(WO4)3/CuWO4 composites were analyzed by X-ray diffraction (XRD), scanning electron microscope (SEM), energy dispersive X-ray spectroscopy (EDX) and X-ray photoelectron spectroscopy (XPS). Using u251 glioma cells as model, the sonodynamic anti-glioma activity of La2(WO4)3/CuWO4 composites was investigated by MTT method. Finally, the sonodynamic antitumor mechanism of La2(WO4)3/CuWO4 composites was discussed based on the optical properties and electrochemical characteristics of La2(WO4)3/CuWO4 composites and ROS probe experimental results. It is expected that the results of this study will provide the research basis for the further development and application of CuWO4 based sonosensitizers in SDT antitumor.
NaWO4·2H2O (AR), 1, 3-diphenylisobenzofuran (DPBF, AR) and terephthalic acid (TA, AR) were purchased from Tianjin Hengxing Chemical reagent manufacturing Co., LTD., (China). NaOH (AR) was purchased from Tianjin Yongda Chemical reagent Co., LTD., (China). Anhydrous ethanol (AR), Cu(NO3)2·3H2O and La(NO3)3·6H2O were purchased from Tianjin Damao chemical reagent factory (China). Phosphate buffer (PBS, BR), thiazole blue (AR), RPMI Medium 1640 (BR), superior fetal bovine serum (BR), 0.25% trypsin/EDTA digestion solution (BR), serum-free cryopreservation (BR), dimethyl sulfoxide (DMSO, BR), 3-(4,5-dimethylthiazol-2-yl)-2,5 diphenyl-tetrazolium bromide (MTT, BR) and AO/EB Kit (LR) were purchased from Beijing Solarbio Technology Co., LTD., (China). Human glioma u251 cells were purchased from Cell Resource Center, Institute of Basic Medicine, Chinese Academy of Medical Sciences.
1.0872 g Cu(NO3)2·3H2O and 0.2165 g La(NO3)3·6H2O were dissolved together in 40 mL deionized water. 2.3090 g Na2WO4·2H2O was weight and dissolved in 20 mL deionized water. Then, under the condition of magnetic stirring, the Na2WO4 solution was added to the mixed solution of Cu(NO3)2 and La(NO3)3 drop by drop, and continued to stir for 30 min. Then the mixed solution was transferred to the polytetrafluoroethylene inner tank of 100 mL stainless steel reactor and reacted in a constant temperature drying oven at 180°C for 24 h. The reaction products were washed with deionized water and anhydrous ethanol three times respectively, and dried at 60°C for 8 h to obtain La2(WO4)3/CuWO4 composite (LC-10) sample. According to the above experimental methods, CuWO4 sample was prepared without adding La(NO3)3·6H2O in the process of synthesis. Similarly, La2(WO4)3 sample was prepared under the above experimental conditions without adding Cu(NO3)2·3H2O in the process of synthesis. Detailed information on the various instruments used in the paper could be found in Supplementary Material.
The cytotoxicity of nano-sonosensitizers and nano-sonosensitizers-mediated sonodynamic action on u251 glioma cells was tested by the typical MTT assay. The u251 glioma cells were plated in 96-well microplates (6 × 103 cells per well) and incubated at 37°C for 24 h. After removing the culture medium, 50 μL of base medium containing various concentrations of La2(WO4)3, CuWO4 and LC-10 samples (10, 20, 50, 100 and 200 μg/mL) were added. After incubation at 37°C for 24 h, 20 μL of medium containing MTT (0.5 mg/mL diluted with medium) was added to each well and continued incubation at 37°C for 4 h. Then, the supernatant in the well was discarded, 150 μL of DMSO was added to each well, and the optical density (OD) was measured at 490 nm using a microplate reader instrument. The cell viability was calculated by Graphpad Pism software. In the experiment on sonodynamic damage to u251 glioma cells, various concentrations of La2(WO4)3, CuWO4 and LC-10 samples (0, 10, 20, 30, 40 and 50 μg/mL) were added. After incubation at 37°C for 24 h, the cells were irradiated with a 1.0 MHz US probe for 1 min, and incubated at 37°C for 24 h. MTT assay was used to detect cell viability.
In order to detect the formation of singlet oxygen (1O2) in the SDT process, DPBF was used as a probe, which could react with 1O2 to decompose DPBF into 1, 2-diphenyl-benzene, resulting in a decrease in the absorption intensity of its characteristic absorption peak at 410 nm (Zhou et al., 2024; Zhang et al., 2023b). The specific experimental method was as follows: 8 mL of 75% ethanol solution containing DPBF (8 mg/L) and sonosensitizer (20 μg/mL) were taken and placed in the US device for 5 min. Then centrifuged at 15,000 rpm for 10 min to remove the sonosensitizer. The absorption spectra of the solution near 410 nm were measured with an ultraviolet-visible (UV-Vis) spectrophotometer.
In order to detect the formation of hydroxyl radical (⋅OH) in the SDT process, TA was used as a probe, which could react with ⋅OH to produce 2-hydroxyterephthalic acid, resulting in a characteristic fluorescence emission peak generated around 430 nm (Pan et al., 2018). Typically, 8 mL 0.5 mmol/L TA solution containing 20 μg/mL of sonosensitizer was taken, then the solution was placed in US bath for US irradiation for 5 min, and centrifugated at 15,000 rpm for 10 min to remove the sonosensitizer. The emission spectrum of the supernatant near 425 nm was measured by a fluorescence spectrophotometer with excitation wavelength of 315 nm.
All data were expressed as mean ± SD, and one-way ANOVA was performed by Graphpad Pism software. When P was less than 0.05, it was statistically significant.
The XRD patterns of the prepared La2(WO4)3, CuWO4 and LC-10 composite were shown in Figure 1. As shown in Figure 1, the XRD patterns of CuWO4 showed that the diffraction peaks were at 13.96°, 22.73°, 28.17°, 35.46°, 36.43° and 40.52°, which belonged to the (010), (110), (−1–11), (0–21), (021) and (−102) planes of CuWO4, respectively. For La2(WO4)3, the diffraction peaks at 18.20°, 28.08°, 30.64°, 33.44°, 38.34°, 45.98°, 47.98°, 52.92°, 56.52°, 58.02° and 74.20° were related to the planes (−112), (−132), (040), (−204), (−134), (060), (−116), (063), (−354), (−264) and (191) of La2(WO4)3. For LC-10 composite, a series of characteristic peaks of CuWO4 and La2(WO4)3 appeared. The XRD diffraction peaks at 22.52°, 35.40°, 36.32° and 40.50° were attributed to the (110), (0-21), (021) and (−102) crystal planes of CuWO4. The XRD diffraction peaks at 18.22°, 28.10°, 30.68°, 33.44°, 38.32°, 45.98°, 47.98°, 52.90°, 56.54°, 58.02° and 74.20° were attributed to the (−112), (−132), (040), (−204), (−134), (060), (−116), (063), (−354), (−264) and (191) crystal planes of La2(WO4)3. The above results confirmed the successful preparation of La2(WO4)3/CuWO4 composite.
The morphologies of the prepared La2(WO4)3, CuWO4 and LC-10 samples were analyzed by SEM, and the results were shown in Figure 2. It could be seen that the prepared La2(WO4)3 sample had an irregular lamellar structure (Figure 2A), and the prepared CuWO4 sample had a nanoparticle shape (Figure 2B). It could be seen from the SEM image of the prepared LC-10 sample (Figure 2C) that CuWO4 nanoparticles were evenly distributed on the lamellar structure of La2(WO4)3, which proved the successful composite of La2(WO4)3 and CuWO4. The EDX diagrams of Cu, La, W and O elements in the synthesized LC-10 sample were shown in Figure 3. It could be seen that Cu, La, W and O elements were evenly distributed on the surface of the synthesized LC-10 sample.
The elemental compositions and chemical valence states of synthesized La2(WO4)3, CuWO4 and LC-10 samples were analyzed by XPS measurement. As shown in Figure 4A, the three peaks of La2(WO4)3 sample at 832.2, 32.6 and 527.7 eV were attributed to La 3d, W 4f and O 1s, respectively. As shown in Figure 4B, the three peaks of the CuWO4 sample at 932.2, 31.1 and 528.1 eV were attributed to Cu 2p, W 4f and O 1s, respectively. As shown in Figure 4C, the four peaks of LC-10 composite at 833.3, 932.5, 33.7 and 528.1 eV were attributed to La 3d, Cu 2p, W 4f and O 1s, respectively.
The high-resolution XPS spectra of Cu 2p, W 4f, O 1s and La 3d in the synthesized CuWO4, La2(WO4)3 and LC-10 samples were shown in Figure 5. The two peaks with binding energies of 933.12 and 952.90 eV in the spectrum of Cu 2p of CuWO4 (Figure 5A) belonged to Cu 2p3/2 and Cu 2p2/1, respectively, which suggested that the presence of Cu2+ and the capture of photogenerated electrons by Cu2+ may cause the valence state change from Cu(II) to Cu(I) (Wang X. et al., 2023; Wan et al., 2013). Compared with CuWO4, the two fitted peaks of Cu 2p of LC-10 composite sample shifted slightly to the higher energy, located at 933.57 and 953.36 eV, respectively. In the high resolution XPS spectrum of W 4f of CuWO4 (Figure 5B), the two peaks at the binding energies of 33.83 and 35.97 eV belonged to W 4f7/2 and W 4f5/2, respectively, suggesting the existence of W6+ in the synthesized sample (Wang et al., 2021; Xu et al., 2023). The two fitted peaks of W 4f of La2(WO4)3 were located at 33.73 and 35.89 eV, respectively. Compared with CuWO4 and La2(WO4)3, the two fitted peaks of W 4f of LC-10 composite sample shifted slightly to the higher energy, located at 34.07 and 36.18 eV, respectively. The high-resolution XPS spectra of O 1s of CuWO4 shown in Figure 5C displayed two fitted peaks at 528.73 and 529.83 eV, respectively, indicating the presence of the lattice oxygen and O-H bonds absorbed on surface of the synthesized sample (He et al., 2024; Wei et al., 2023). The two fitted peaks of O 1s of La2(WO4)3 were located at 528.85 and 531.16 eV, respectively. Compared with CuWO4 and La2(WO4)3, the two fitted peaks of O 1s of LC-10 composite sample shifted slightly to the higher energy or lower energy, located at 529.01 and 530.29 eV, respectively. The high-resolution XPS spectrum of La 3d of La2(WO4)3 shown in Figure 5D displayed the spin–orbit splitting: La 3d5/2 (833.51 and 836.97 eV) and La 3d3/2 (850.28 and 854.00 eV), which were in accordance with the standard XPS peaks of La3+ (Baby et al., 2024). Compared with La2(WO4)3, the fitted peaks of La 3d of LC-10 composite sample also shifted slightly. The above results indicated that the two substances, CuWO4 and La2(WO4)3 interacted with each other, and the heterojunction of La2(WO4)3/CuWO4 formed.
Figure 5. High-resolution XPS spectra of Cu 2p (A), W 4f (B), O 1s (C) and La 3d (D) in synthesized CuWO4, La2(WO4)3 and LC-10 samples.
The UV-Vis diffuse reflectance spectra (DRS) of CuWO4, La2(WO4)3 and LC-10 were measured using a UV-Vis spectrophotometer in the wavelength range of 200–800 nm, and the results were shown in Figure 6. It could be seen that CuWO4 had a strong light absorption capacity in the entire 200–800 nm range, indicating that CuWO4 had a good light response ability to both UV and Vis light. La2(WO4)3 had an obvious absorption boundary near 340 nm, which indicated that La2(WO4)3 had a strong absorption capacity for UV light. It could be seen from the DRS of LC-10 that La2(WO4)3/CuWO4 composite had a good light response to both UV and Vis light. Based on the above DRS results, the band gap energy (Eg) of CuWO4 and La2(WO4)3 could be obtained by the Kubelka-Munk formula (Wang et al., 2021). The correlation curves of (Ahν)2 vs. hν of CuWO4 and La2(WO4)3 were shown in Figures 7A, B, the Eg of CuWO4 and La2(WO4)3 were obtained as 2.99 and 2.54 eV, respectively.
First, the cytotoxicity of prepared CuWO4, La2(WO4)3 and LC-10 samples to u251 cells was investigated. In the presence of different concentrations (10, 20, 50, 100 and 200 μg/mL) prepared CuWO4, La2(WO4)3 and LC-10 samples, the cell viability of u251 cells during logarithmic growth was measured by MTT method. Figure 8A displayed the cytotoxicity test results of CuWO4. When CuWO4 concentration was 10, 20, 50, 100 and 200 μg/mL, the cell viability was 105.45% ± 5.05%, 103.01% ± 2.57%, 96.31% ± 3.71%, 93.50% ± 6.46% and 86.65% ± 8.08%, respectively. Figure 8B showed the cytotoxicity test results of La2(WO4)3. When the concentration of La2(WO4)3 was 10, 20, 50, 100 and 200 μg/mL, the cell viability was 97.72% ± 4.54%, 93.66% ± 4.80%, 90.84% ± 2.78%, 89.88% ± 4.15% and 85.65% ± 6.49%, respectively. The results showed that CuWO4 and La2(WO4)3 did not cause obvious cytotoxicity to u251 cells when the concentrations of CuWO4 and La2(WO4)3 were below 200 μg/mL. Figure 8C showed the cytotoxicity test results of LC-10 sample. When the concentration of LC-10 was 10, 20, 50, 100 and 200 μg/mL, the cell viability was 95.17% ± 4.35%, 94.40% ± 6.70%, 84.11% ± 5.31%, 79.26% ± 4.38% and 71.05% ± 6.12%, respectively. These results showed that with the increase of LC-10 concentration, the cell survival rate decreased gradually. When the concentration of LC-10 was higher than 50 μg/mL, it had a certain toxic effect on cells.
Figure 8. Cytotoxicity of CuWO4 (A), La2(WO4)3 (B) and LC-10 (C) to u251 cells (*p < 0.05,**p < 0.01,***p < 0.001,****p < 0.0001).
Next, u251 cells were treated differently to evaluate the sonodynamic therapeutic effect of La2(WO4)3/CuWO4 composite on tumor cells. It could be seen from Figure 9A that the cell viability decreased slightly under US irradiation alone. This was because the energy generated by the cavitation effect of US could lead to the cracking of water molecules, resulting in a strong oxidizing ·OH. The effects of different concentrations of LC-10 on the cell viability of u251 cells under US irradiation were shown in Supplementary Figures S1–S5. It could be clearly observed that the cell viability of u251 cells treated with SDT in the presence of LC-10 was significantly lower than that treated with LC-10 alone and US alone at any concentration of LC-10. These results suggested that La2(WO4)3/CuWO4 composite had excellent sonodynamic antitumor performance. The cell inhibition rate of u251 cells after SDT in the presence of different concentrations of CuWO4, La2(WO4)3 and LC-10 were shown in Figure 9B. It could be seen that the cell inhibition rate of u251 cells after SDT in the presence of LC-10 at any concentration was significantly higher than that in the presence of CuWO4 and La2(WO4)3 at same concentration. These results indicated that La2(WO4)3/CuWO4 composite had higher sonodynamic antitumor performance. In addition, it was also shown that the construction of heterojunction was an effective means to improve the sonodynamic antitumor performance of nano-sonosensitizers.
Figure 9. Cell viability of u251 cells under US irradiation (A) and cell inhibition rate of u251 cells after SDT in the presence of different concentrations of CuWO4, La2(WO4)3 and LC-10 (B) (*p < 0.05).
Subsequently, sonodynamic-induced cancer cell apoptosis was further evaluated using AO/EB staining. As shown in Figure 10, under the same experimental conditions, cells were divided into control group (A), US group (B), LC-10 group (C), and LC-10+US group (D). In the control group (Figure 10A), the quantity and intensity of green fluorescence were high, while the orange fluorescence was negligible, indicating good growth of cells. As shown in Figures 10B, C, the US group and the LC-10 group showed weak orange fluorescence, and most of the cells showed green fluorescence, indicating that most of the cells had good viability. Figure 10D showed a large amount of orange fluorescence in the LC-10+US group, indicating that LC-10 produced a large number of ROS inducing apoptosis under US irradiation, and the experimental results were consistent with the above MTT results.
Figure 10. Confocal laser scanning microscopy images of u251 cells stained with AO/EB after various treatments. (A) control group, (B) US group, (C) LC-10 group, and (D) LC-10+US group.
It could be clearly seen from the above results that the combined use of US and sonosensiizer LC-10 had a much higher inhibitory effect on the growth of u251 glioma cells than that of US alone and LC-10 alone. The combined use of US and sonosensiizer LC-10 also significantly inhibited the growth of u251 glioma cells compared with the combined effect of US and La2(WO4)3 and the combined effect of US and CuWO4, indicating that LC-10 had better sonodynamic antitumor activity. The essence of the good sonodynamic activity of the nano-sonosensitizer was that it could become a ROS generator under US irradiation (Duan et al., 2023). In order to study the ability of La2(WO4)3/CuWO4 composite as a sonosensitizer to produce ROS and the corresponding sonodynamic mechanism, specific ROS probes were used to verify the types of ROS produced during SDT process. The 1O2 yields of La2(WO4)3/CuWO4 composite produced under US irradiation were evaluated using DPBF as a probe. It could be seen from Figure 11A, under US irradiation, the absorption peak of DPBF at 410 nm decreased in the presence of LC-10 compared with the control group of water, suggesting the generation of 1O2 in the system. Similarly, the capture of ⋅OH was tracked by TA. It could be seen from Figure 11B, the combined use of US and LC-10 remarkably increased the fluorescence intensity of the solution around 430 nm. The results showed that La2(WO4)3/CuWO4 composite had good ·OH production ability under US irradiation.
The photoluminescence spectra of CuWO4 and La2(WO4)3/CuWO4 composite LC-10 were measured with a fluorescence spectrophotometer in the wavelength range of 200–600 nm, and the results were shown in Figure 12. It could be seen that under the excitation condition of 269 nm wavelength, CuWO4 had an obvious emission peak near 520 nm. Similarly, the maximum emission wavelength of the emission peak of LC-10 was also around 520 nm, but its fluorescence intensity was significantly lower than that of CuWO4, indicating that after La2(WO4)3 and CuWO4 formed a composite, carrier separation could be effectively realized in the catalyst, and the recombination rate of e−-h+ pairs was significantly reduced (Liu et al., 2024). The production of ROS of the catalyst under US irradiation was improved significantly, thus displayed excellent sonodynamic antitumor performance.
The type of semiconductor material could be determined by whether the slope of the tangent in the Mott-Schottky curves was positive or negative. A positive slope indicated an n-type semiconductor and a negative slope indicated a p-type semiconductor (Chang et al., 2023; Chang et al., 2024). As seen in Figure 13, The slopes of tangent lines of the Mott-Schottky curves of La2(WO4)3 and CuWO4 were both positive. The results showed that all the prepared La2(WO4)3 and CuWO4 samples had n-type semiconductor characteristics. Additionally, the flat band potential (Ef) values of the prepared La2(WO4)3 and CuWO4 samples could be obtained from the intercept of the tangent with the X-axis (Huang et al., 2024a; Chang et al., 2025). Therefore, the Ef values of La2(WO4)3 and CuWO4 samples were determined to be −0.55 V and −0.30 V vs saturated calomel electrode (SCE), respectively. Accordingly, the Ef values of La2(WO4)3 and CuWO4 samples could be calculated as −0.31 V and −0.06 V vs standard hydrogen electrode (NHE), considering the correction value between SCE and NHE was 0.24 V (Lu et al., 2025; Huang et al., 2024b). It was known that for most n-type semiconductors, the minimum potential of the conduction band (ECB) was about 0.1–0.3 V lower than the Ef value, and the middle value of 0.2 V was used here (Huang et al., 2024c; Chen et al., 2024). Therefore, the ECB values of La2(WO4)3 and CuWO4 samples were calculated as −0.51 V and −0.26 V vs. NHE, respectively. According to the Eg values of La2(WO4)3 and CuWO4 samples were 2.54 and 2.99 eV, respectively, and the relationship between the maximum potential of valence band (EVB), ECB and Eg (EVB = ECB + Eg) (Han et al., 2025), EVB values of La2(WO4)3 and CuWO4 samples relative to NHE could be calculated as 2.03 V and 2.73 V, respectively. Based on the above calculation, the schematic diagram of the band structure corresponding to La2(WO4)3/CuWO4 composite was shown in Figure 14. It could be seen that there are obvious differences in the band and energy level structure between La2(WO4)3 and CuWO4 in La2(WO4)3/CuWO4 composite, and a hypothesis that La2(WO4)3/CuWO4 n–n heterojunction formed was proposed.
Combined with the results of the above study and related literature reports, the main mechanism of LC-10-mediated SDT antitumor was proposed (as shown in Figure 14). Firstly, in the process of US irradiation, the tiny bubbles in the liquid were activated by US, resulting in a series of dynamic processes such as expansion, contraction, expansion and collapse, that was, the cavitation effect (Rosenthal et al., 2004; Teng et al., 2024). The cavitation effect (transient cavitation) generated by US could form local hot spots of high temperature and pressure, and its energy could cause water molecules to crack and produced ·OH (Rosenthal et al., 2004), which would cause damage to tumor cells. Secondly, the addition of La2(WO4)3/CuWO4 composite nanoparticles could provide more active sites for the nucleation process and the formation of cavitation microbubbles (Qiu et al., 2018), which could generate more active free radicals, and then caused more serious damage to tumor cells. Thirdly, in the process of US irradiation, the cavitation effect of US would lead to sonoluminescence (Xu et al., 2025). The light energy generated by sonoluminescence and the heat energy from local hot spots could activate the sonosensitizer molecule to transition from the ground state to the excited state (Qian et al., 2024). In the process of the sonosensitizer molecule returning from the excited state to the ground state, the released energy was transferred to the water and oxygen molecules in the medium. ROS with strong oxidizing properties such as 1O2 and ·OH were produced (Qian et al., 2024), thus causing damage to tumor cells. Finally, the La2(WO4)3/CuWO4 composite formed by La2(WO4)3 and CuWO4 was stimulated by the light generated by sonoluminescence and heat from local hot spots. When the light energy and the heat energy were equal to or exceeded the Eg of La2(WO4)3 and CuWO4 semiconductor, the photothermal generated e− would produce on the VB of La2(WO4)3 and CuWO4, and then transferred to the CB, forming h+ on the VB of La2(WO4)3 and CuWO4, respectively (Xu et al., 2025). Due to obvious differences in the band and energy level structure between La2(WO4)3 and CuWO4 in La2(WO4)3/CuWO4 composite, the photothermal generated e− on the CB of La2(WO4)3 would transfer to the CB of CuWO4, and photothermal generated h+ on the VB of CuWO4 would transfer to the VB of La2(WO4)3. This could effectively inhibit the photothermal-generated e−–h+ pairs recombination in La2(WO4)3/CuWO4 composite. Because the EVB oxidation potential (2.03 V) of La2(WO4)3 was higher than that of ⋅OH/OH− (1.99 V) (He et al., 2025; Sun et al., 2025), h+ on VB could oxidize OH− to ⋅OH, leading to oxidative damage of tumor cells. In addition, the CB reduction potential (−0.26 V) of CuWO4 was not negative than that of O2/⋅O2− (−0.33 V) (Wang et al., 2024; Sun et al., 2024), which suggested that the e− on the CB of CuWO4 could not reduce O2 to superoxide anion radical (⋅O2−). In summary, the production of ROS such as 1O2 and ·OH, especially the production of ⋅OH, played an important role in the La2(WO4)3/CuWO4 composite mediated SDT antitumor process, which was consistent with the results of previous ROS probe experiments.
In summary, La2(WO4)3/CuWO4 composites were prepared by two-step hydrothermal method and characterized by XRD, SEM, XPS, EDX and DRS. On this basis, using u251 glioma cells as model, the sonodynamic antitumor activity of La2(WO4)3/CuWO4 composite LC-10 was investigated by MTT method and AO/EB staining. The results showed that compared with La2(WO4)3 and CuWO4, La2(WO4)3/CuWO4 composite had better sonodynamic antitumor activity, and LC-10 had good biosafety at concentrations below 50 μg·mL−1. After La2(WO4)3 and CuWO4 formed La2(WO4)3/CuWO4 composite, the photo-thermal generated e− on the CB of La2(WO4)3 would be transferred to the CB of CuWO4, and the generated h+ on the VB of CuWO4 would be transferred to the VB of La2(WO4)3 during SDT. The recombination of e−–h+ pairs was effectively inhibited, and more strongly oxidizing ROS was produced, inducing apoptosis of u251 glioma cells. In which, 1O2 and ·OH, especially the production of ⋅OH, played an important role in the La2(WO4)3/CuWO4 composite mediated SDT antitumor process. In general, the results of this study would lay a foundation for the design of CuWO4 base nano-sonosensitizer and its further clinical application in the study of sonodynamic antitumor. At the same time, it also provided a new strategy for the design and development of novel nano-sonosensitizers with excellent sonodynamic activity.
The original contributions presented in the study are included in the article/Supplementary Material, further inquiries can be directed to the corresponding author.
Ethical approval was not required for the studies on humans in accordance with the local legislation and institutional requirements because only commercially available established cell lines were used.
F-YL: Data curation, Investigation, Writing–original draft. XW: Conceptualization, Methodology, Project administration, Writing–review and editing. Y-FL: Funding acquisition, Project administration, Writing–review and editing.
The author(s) declare that financial support was received for the research, authorship, and/or publication of this article. This work was financially supported by the Medical and Industrial Cross Joint Fund of Liaoning Cancer Hospital and Institute and Dalian University of Technology (LD202212).
The authors declare that the research was conducted in the absence of any commercial or financial relationships that could be construed as a potential conflict of interest.
The author(s) declare that no Generative AI was used in the creation of this manuscript.
All claims expressed in this article are solely those of the authors and do not necessarily represent those of their affiliated organizations, or those of the publisher, the editors and the reviewers. Any product that may be evaluated in this article, or claim that may be made by its manufacturer, is not guaranteed or endorsed by the publisher.
The Supplementary Material for this article can be found online at: https://www.frontiersin.org/articles/10.3389/fbioe.2025.1566946/full#supplementary-material
Baby, B., Thomas, S., Jose, J., Thomas, K., Punathil, P., Unnikrishnan, N. V., et al. (2024). Luminescence studies and Judd Ofelt parameterization of Dy3+ activated La2(WO4)3 yellow phosphor. Inorg. Chem. Commun. 165, 112550. doi:10.1016/j.inoche.2024.112550
Canavese, G., Ancona, A., Racca, L., Canta, M., Dumontel, B., Barbaresco, F., et al. (2018). Nanoparticle-assisted ultrasound: a special focus on sonodynamic therapy against cancer. Chem. Eng. J. 340, 155–172. doi:10.1016/j.cej.2018.01.060
Chang, F., Bao, W., Li, K., Bai, W., Shi, Z., Liu, D.-g., et al. (2024). Augmented photocatalytic NO removal by the S-scheme Bi7O9I3/Bi2S3 heterojunctions with surface oxygen vacancies: experimental analyses and theoretical calculations. J. Environ. Manag. 370, 122390. doi:10.1016/j.jenvman.2024.122390
Chang, F., Lei, Y., Li, J., Li, S., Liu, D.-g., and Kong, Y. (2023). Externally modified Bi12SiO20 with BiOI: n-p heterojunctions for effectually photocatalytic degradation of bisphenol A. Sep. Purif. Technol. 323, 124516. doi:10.1016/j.seppur.2023.124516
Chang, F., Li, J., Kou, Y., Bao, W., Shi, Z., Zhu, G., et al. (2025). The intense charge migration and efficient photocatalytic NO removal of the S-scheme heterojunction composites Bi7O9I3-BiOBr. Sep. Purif. Technol. 353, 128402. doi:10.1016/j.seppur.2024.128402
Chen, H., Sun, M., Zhao, J., Huang, X., Teng, H., Gao, Y., et al. (2024). In-situ assembling novel N-Ti3C2/BiOClxBr1−x composites with enhanced photocatalytic degradation and nitrogen reduction activity. Spectrochimica Acta Part A Mol. Biomol. Spectrosc. 316, 124331. doi:10.1016/j.saa.2024.124331
Chen, L., Mao, Z., Wang, Y., Kang, Y., Wang, Y., Mei, L., et al. (2022). Edge modification facilitated heterogenization and exfoliation of two-dimensional nanomaterials for cancer catalytic therapy. Sci. Adv. 8, eabo7372. doi:10.1126/sciadv.abo7372
Cheng, M., Liu, Y., You, Q., Lei, Z., Ji, J., Zhang, F., et al. (2024). Metal-doping strategy for carbon-based sonosensitizer in sonodynamic therapy of glioblastoma. Adv. Sci. 11, 2404230. doi:10.1002/advs.202404230
Cui, Y., Chen, X., Cheng, Y., Lu, X., Meng, J., Chen, Z., et al. (2021). CuWO4 Nanodots for NIR-induced photodynamic and chemodynamic synergistic therapy. ACS Appl. Mater. and Interfaces 13, 22150–22158. doi:10.1021/acsami.1c00970
Dong, Y., Dong, S., Liu, B., Yu, C., Liu, J., Yang, D., et al. (2021). 2D piezoelectric Bi2MoO6 nanoribbons for GSH-enhanced sonodynamic therapy. Adv. Mater. 33, 2106838. doi:10.1002/adma.202106838
Duan, Y., Yu, Y., Liu, P., Gao, Y., Dai, X., Zhang, L., et al. (2023). Reticular chemistry-enabled sonodynamic activity of covalent organic frameworks for nanodynamic cancer therapy. Angew. Chem. Int. Ed. 62, e202302146. doi:10.1002/anie.202302146
Gong, F., Cheng, L., Yang, N., Gong, Y., Ni, Y., Bai, S., et al. (2020). Preparation of TiH1.924 nanodots by liquid-phase exfoliation for enhanced sonodynamic cancer therapy. Nat. Commun. 11, 3712. doi:10.1038/s41467-020-17485-x
Hachimine, K., Shibaguchi, H., Kuroki, M., Yamada, H., Kinugasa, T., Nakae, Y., et al. (2007a). Sonodynamic therapy of cancer using a novel porphyrin derivative, DCPH-P-Na(I), which is devoid of photosensitivity. Cancer Sci. 98, 916–920. doi:10.1111/j.1349-7006.2007.00468.x
Hachimine, K., Shibaguchi, H., Kuroki, M., Yamada, H., Kinugasa, T., Nakae, Y., et al. (2007b). Sonodynamic therapy of cancer using a novel porphyrin derivative, DCPH-P-Na(I), which is devoid of photosensitivity. Cancer Sci. 98, 916–920. doi:10.1111/j.1349-7006.2007.00468.x
Han, X., Huang, J., Jing, X., Yang, D., Lin, H., Wang, Z., et al. (2018). Oxygen-deficient black titania for synergistic/enhanced sonodynamic and photoinduced cancer therapy at near infrared-II biowindow. ACS Nano 12, 4545–4555. doi:10.1021/acsnano.8b00899
Han, Y., Qi, X.-X., Liu, Y.-C., Wang, Y., Xiang, Z., and Wang, X. (2025). Preparation of CuWO4/Bi2WO6 composite and its sonocatalytic removal of tetracycline by combined persulfate. J. Alloys Compd. 1010, 177505. doi:10.1016/j.jallcom.2024.177505
He, L.-L., Li, S., Qi, S., Wang, N., Sun, M.-T., Su, C., et al. (2024). Synthesis of three-dimensional flower-like BiFeO3 with enhanced sonocatalytic performance for the removal of methylene blue. Inorg. Chem. Commun. 166, 112649. doi:10.1016/j.inoche.2024.112649
He, L.-L., Wang, N., Qi, S., Sun, M.-T., Kou, X.-W., Su, C., et al. (2025). Preparation of CuWO4/CaWO4 n–n heterojunction with enhanced sonocatalytic performance: characterization, sonocatalytic mechanism and degradation pathways of organic pollutant. Inorg. Chem. Commun. 171, 113564. doi:10.1016/j.inoche.2024.113564
He, L.-L., Wang, X., Liu, B., Wang, J., Sun, Y.-G., and Xu, S.-K. (2011a). Study on the sonodynamic activity and mechanism of promethazine hydrochloride by multi-spectroscopic techniques. Spectrochimica Acta Part A Mol. Biomol. Spectrosc. 81, 698–705. doi:10.1016/j.saa.2011.07.006
He, L.-L., Wang, X., Liu, B., Wang, J., Sun, Y.-G., and Xu, S.-K. (2011b). Spectroscopic investigation on the synergistic effects of ultrasound and dioxopromethazine hydrochloride on protein. J. Fluoresc. 21, 1847–1856. doi:10.1007/s10895-011-0879-2
He, L.-L., Wang, X., Wu, X.-X., Wang, Y.-X., Kong, Y.-M., Wang, X., et al. (2015a). Protein damage and reactive oxygen species generation induced by the synergistic effects of ultrasound and methylene blue. Spectrochimica Acta Part A Mol. Biomol. Spectrosc. 134, 361–366. doi:10.1016/j.saa.2014.06.121
He, L.-L., Wu, X.-X., Wang, Y.-X., Liu, X.-P., Song, Y.-L., Yang, Y.-J., et al. (2015b). Spectroscopic investigation on the sonodynamic damage to protein in the presence of eosine B. Ultrason. Sonochemistry 26, 93–98. doi:10.1016/j.ultsonch.2015.02.002
Huang, X., Chen, H., Sun, M., Zhao, J., Teng, H., Gao, Y., et al. (2024c). N-graphyne surrounded Bi2S3/BiOBr composites: in-situ ultrasound-assisted synthesis and superior photocatalytic activity. Fuel 375, 132613. doi:10.1016/j.fuel.2024.132613
Huang, X., Sun, M., Humayun, M., Li, S., Zhao, J., Chen, H., et al. (2024b). In-situ synthesis of efficient N-graphyne/Bi/BiOBr photocatalysts for contaminants removal and nitrogen fixation. J. Alloys Compd. 976, 173025. doi:10.1016/j.jallcom.2023.173025
Huang, X., Sun, M., Sun, W., Li, Z., Chen, H., and Zhao, J. (2024a). One-step hydrothermal formation of porous N-graphyne decorated TiO2/Ti3C2 composites with enhanced photocatalytic activity. Int. J. Hydrogen Energy 55, 581–591. doi:10.1016/j.ijhydene.2023.11.203
Jiang, L., Wang, J., Jiang, J., Zhang, C., Zhao, M., Chen, Z., et al. (2020). Sonodynamic therapy in atherosclerosis by curcumin nanosuspensions: preparation design, efficacy evaluation, and mechanisms analysis. Eur. J. Pharm. Biopharm. 146, 101–110. doi:10.1016/j.ejpb.2019.12.005
Kang, Y., Mao, Z., Wang, Y., Pan, C., Ou, M., Zhang, H., et al. (2022). Design of a two-dimensional interplanar heterojunction for catalytic cancer therapy. Nat. Commun. 13, 2425. doi:10.1038/s41467-022-30166-1
Kumar, G., Kumar, J., Bag, M., and Kumar Dutta, R. (2022). Solar light induced photocatalytic process for reduction of hexavalent chromium and degradation of tetracycline and methylene blue by heterostructures made of SnS2 nanoplates surface modified by ZnWO4 nanorods. Sep. Purif. Technol. 292, 121040. doi:10.1016/j.seppur.2022.121040
Li, J.-H., Song, D.-Y., Xu, Y.-G., Huang, Z., and Yue, W. (2008). In vitro study of haematoporphyrin monomethyl ether-mediated sonodynamic effects on C6 glioma cells. Neurol. Sci. 29, 229–235. doi:10.1007/s10072-008-0972-8
Li, R., Wang, X., Shi, J., Kang, Y., and Ji, X. (2023). Sonocatalytic cancer therapy: theories, advanced catalysts and system design. Nanoscale 15, 19407–19422. doi:10.1039/d3nr04505f
Liu, Q., Wang, X., Wang, P., Qi, H., Zhang, K., and Xiao, L. (2006). Sonodynamic effects of protoporphyrin IX disodium salt on isolated sarcoma 180 cells. Ultrasonics 45, 56–60. doi:10.1016/j.ultras.2006.06.063
Liu, Y.-C., Ge, Y.-D., An, H.-L., Ju, W.-T., Zhou, X.-Y., Xing, M.-Y., et al. (2024). Synthesis of a novel double Z-scheme BiVO4/Cu2O/g-C3N4 sonocatalyst and research on sonocatalytic degradation of tetracycline. J. Environ. Chem. Eng. 12, 113668. doi:10.1016/j.jece.2024.113668
Liu, Y.-C., Liu, X., Wang, X., Li, Z.-H., Chen, C.-L., and Xiang, Z. (2023). Hybrid persulfate/sonocatalysis for degradation of acid orange 7 in the presence of Ag2O/CuWO4 composite: operating parameters and sonocatalytic mechanism. J. Clean. Prod. 394, 136287. doi:10.1016/j.jclepro.2023.136287
Lu, X., Li, J., and Chang, F. (2025). Sonocatalytic evaluation of binary composites Bi12GeO20/GO in degradation of tetracycline hydrochloride. Mater. Sci. Semicond. Process. 185, 108964. doi:10.1016/j.mssp.2024.108964
Pan, X., Bai, L., Wang, H., Wu, Q., Wang, H., Liu, S., et al. (2018). Metal–organic-framework-derived carbon nanostructure augmented sonodynamic cancer therapy. Adv. Mater. 30, 1800180. doi:10.1002/adma.201800180
Ping, J., Du, J., Ouyang, R., Miao, Y., and Li, Y. (2023). Recent advances in stimuli-responsive nano-heterojunctions for tumor therapy. Colloids Surfaces B Biointerfaces 226, 113303. doi:10.1016/j.colsurfb.2023.113303
Qian, M.-Q., Xiang, Z., and Wang, X. (2024). Sonodynamic inactivation of gram-negative and gram-positive bacteria in the presence of phenothiazine compounds toluidine blue and azurin A. Biochimica Biophysica Acta (BBA) - General Subj. 1868, 130711. doi:10.1016/j.bbagen.2024.130711
Qiu, P., Park, B., Choi, J., Thokchom, B., Pandit, A. B., and Khim, J. (2018). A review on heterogeneous sonocatalyst for treatment of organic pollutants in aqueous phase based on catalytic mechanism. Ultrason. Sonochemistry 45, 29–49. doi:10.1016/j.ultsonch.2018.03.003
Rosenthal, I., Sostaric, J. Z., and Riesz, P. (2004). Sonodynamic therapy––a review of the synergistic effects of drugs and ultrasound. Ultrason. Sonochemistry 11, 349–363. doi:10.1016/j.ultsonch.2004.03.004
Sasaki, K., Yumita, N., Nishigaki, R., and Umemura, S. (1998). Antitumor effect sonodynamically induced by focused ultrasound in combination with Ga-porphyrin complex. Jpn. J. Cancer Res. 89, 452–456. doi:10.1111/j.1349-7006.1998.tb00584.x
Song, K., Du, J., Wang, X., Zheng, L., Ouyang, R., Li, Y., et al. (2022). Biodegradable bismuth-based nano-heterojunction for enhanced sonodynamic oncotherapy through charge separation engineering. Adv. Healthc. Mater. 11, 2102503. doi:10.1002/adhm.202102503
Sun, L., He, X., Liu, B., Zhang, S., Xiang, Z., Wang, X., et al. (2025). Synergistic adsorption-photocatalytic degradation of tetracycline by S-scheme InVO4/ZnIn2S4 heterojunction: mechanism, toxicity assessment, and potential applications. Sep. Purif. Technol. 353, 128515. doi:10.1016/j.seppur.2024.128515
Sun, M.-T., Qi, Q., Wang, X., and He, L.-L. (2024). Synthesis of Ag2O/CaMoO4 S-type heterojunction with enhanced sonocatalytic performance to remove crystal violet in dye wastewater. Inorg. Chem. Commun. 167, 112778. doi:10.1016/j.inoche.2024.112778
Teng, J., Xiong, S., Li, F., Wang, S., and Li, T. (2024). Sonocatalytic performance of Bi2WO6 nanoparticles for degradation of tetracycline antibiotics. Appl. Catal. O Open 191, 206957. doi:10.1016/j.apcato.2024.206957
Umemura, S., Yumita, N., Nishigaki, R., and Umemura, K. (1989). “Sonochemical activation of hematoporphyrin: a potential modality for cancer treatment,” in Ultrasonics symposium, proceedings (IEEE), 955–960.
Umemura, S.-i., Yumita, N., and Nishigaki, R. (1993). Enhancement of ultrasonically induced cell damage by a gallium-porphyrin complex, ATX-70. Jpn. J. Cancer Res. 84, 582–588. doi:10.1111/j.1349-7006.1993.tb00179.x
Wan, L., Sheng, J., Chen, H., and Xu, Y. (2013). Different recycle behavior of Cu2+ and Fe3+ ions for phenol photodegradation over TiO2 and WO3. J. Hazard. Mater. 262, 114–120. doi:10.1016/j.jhazmat.2013.08.002
Wang, X., He, L.-L., Liu, B., Wang, X.-F., Xu, L., and Sun, T. (2018). Reactive oxygen species generation and human serum albumin damage induced by the combined effects of ultrasonic irradiation and brilliant cresyl blue. Int. J. Biol. Macromol. 120, 1865–1871. doi:10.1016/j.ijbiomac.2018.10.002
Wang, X., He, X.-S., Li, C.-Y., Liu, S.-L., Lu, W., Xiang, Z., et al. (2023b). Sonocatalytic removal of tetracycline in the presence of S-scheme Cu2O/BiFeO3 heterojunction: operating parameters, mechanisms, degradation pathways and toxicological evaluation. J. Water Process Eng. 51, 103345. doi:10.1016/j.jwpe.2022.103345
Wang, X., Yu, S., Li, Z.-H., He, L.-L., Liu, Q.-L., Hu, M.-Y., et al. (2021). Fabrication Z-scheme heterojunction of Ag2O/ZnWO4 with enhanced sonocatalytic performances for meloxicam decomposition: increasing adsorption and generation of reactive species. Chem. Eng. J. 405, 126922. doi:10.1016/j.cej.2020.126922
Wang, Y., Gong, F., Han, Z., Lei, H., Zhou, Y., Cheng, S., et al. (2023a). Oxygen-deficient molybdenum oxide nanosensitizers for ultrasound-enhanced cancer metalloimmunotherapy. Angew. Chem. Int. Ed. 62, e202215467. doi:10.1002/anie.202215467
Wang, Y., Li, F., and Li, T. (2024). Facile synthesis of BiOI/BaNbO3 composite for rapid sonocatalytic degradation of tetracycline hydrochloride. J. Solid State Chem. 333, 124644. doi:10.1016/j.jssc.2024.124644
Wei, J., Li, T., and Li, F. (2023). Synthesis and sonocatalytic performance of Dy2Sn2O7/Sepiolite nanocomposite. J. Solid State Chem. 328, 124368. doi:10.1016/j.jssc.2023.124368
Wu, N., Fan, C.-H., and Yeh, C.-K. (2022). Ultrasound-activated nanomaterials for sonodynamic cancer theranostics. Drug Discov. Today 27, 1590–1603. doi:10.1016/j.drudis.2022.02.025
Xiao, Z., Peng, C., Jiang, X., Peng, Y., Huang, X., Guan, G., et al. (2016). Polypyrrole-encapsulated iron tungstate nanocomposites: a versatile platform for multimodal tumor imaging and photothermal therapy. Nanoscale 8, 12917–12928. doi:10.1039/c6nr03336a
Xing, X., Zhao, S., Xu, T., Huang, L., Zhang, Y., Lan, M., et al. (2021). Advances and perspectives in organic sonosensitizers for sonodynamic therapy. Coord. Chem. Rev. 445, 214087. doi:10.1016/j.ccr.2021.214087
Xu, L., Ge, Y.-D., Zhou, X.-Y., Xing, M.-Y., Wu, X.-Q., Wang, Y., et al. (2025). Study on the sonocatalytic removal of tetracycline by an type-II heterojunction CuS/FeWO4. J. Alloys Compd. 1015, 178826. doi:10.1016/j.jallcom.2025.178826
Xu, L., Wu, X.-Q., Li, C.-Y., Liu, N.-P., An, H.-L., Ju, W.-T., et al. (2023). Sonocatalytic degradation of tetracycline by BiOBr/FeWO4 nanomaterials and enhancement of sonocatalytic effect. J. Clean. Prod. 394, 136275. doi:10.1016/j.jclepro.2023.136275
Yang, Y., Wang, X., Qian, H., and Cheng, L. (2021). Titanium-based sonosensitizers for sonodynamic cancer therapy. Appl. Mater. Today 25, 101215. doi:10.1016/j.apmt.2021.101215
Zhang, M., Cui, Z., Song, R., Lv, B., Tang, Z., Meng, X., et al. (2018). SnWO4-based nanohybrids with full energy transfer for largely enhanced photodynamic therapy and radiotherapy. Biomaterials 155, 135–144. doi:10.1016/j.biomaterials.2017.11.013
Zhang, S., Xia, S., Chen, L., Chen, Y., and Zhou, J. (2023b). Covalent organic framework nanobowls as activatable nanosensitizers for tumor-specific and ferroptosis-augmented sonodynamic therapy. Adv. Sci. 10, 2206009. doi:10.1002/advs.202206009
Zhang, S., Zhang, L., Hu, J., He, X., Geng, B., Pan, D., et al. (2023a). Trienzyme-like Co3O4@TiO2-x nanozymes for heterojunction-enhanced nanocatalytic-sonodynamic tumor therapy. Chem. Eng. J. 458, 141485. doi:10.1016/j.cej.2023.141485
Zhang, T., Zheng, Q., Fu, Y., Xie, C., Fan, G., Wang, Y., et al. (2021). α-Fe2O3@Pt heterostructure particles to enable sonodynamic therapy with self-supplied O2 and imaging-guidance. J. Nanobiotechnology 19, 358. doi:10.1186/s12951-021-01105-x
Zhou, Y., Cao, Z., Jiang, L., Chen, Y., Cui, X., Wu, J., et al. (2024). Magnetically actuated sonodynamic nanorobot collectives for potentiated ovarian cancer therapy. Front. Bioeng. Biotechnol. 12, 1374423. doi:10.3389/fbioe.2024.1374423
Zhou, Y., Yang, N., Gong, F., Wang, Y., Yang, X., Dai, Y., et al. (2022). Oxygen-deficient tungsten oxide (WOx) nanobelts with pH-sensitive degradation for enhanced sonodynamic therapy of cancer. ACS Nano 16, 17242–17256. doi:10.1021/acsnano.2c07903
Keywords: La2(WO4)3/CuWO4 composite, nano-sonosensitizer, sonodynamic therapy (SDT), sonodynamic antitumor activity, reactive oxygen species (ROS)
Citation: Liu F-Y, Wang X and Liu Y-F (2025) Preparation of La2(WO4)3/CuWO4 composite nanomaterials with enhanced sonodynamic anti-glioma activity. Front. Bioeng. Biotechnol. 13:1566946. doi: 10.3389/fbioe.2025.1566946
Received: 26 January 2025; Accepted: 05 March 2025;
Published: 20 March 2025.
Edited by:
Xianzhi Zhang, Yale University, United StatesReviewed by:
Fei Chang, University of Shanghai for Science and Technology, ChinaCopyright © 2025 Liu, Wang and Liu. This is an open-access article distributed under the terms of the Creative Commons Attribution License (CC BY). The use, distribution or reproduction in other forums is permitted, provided the original author(s) and the copyright owner(s) are credited and that the original publication in this journal is cited, in accordance with accepted academic practice. No use, distribution or reproduction is permitted which does not comply with these terms.
*Correspondence: Ye-Fu Liu, aHk4MTUxQDE2My5jb20=
Disclaimer: All claims expressed in this article are solely those of the authors and do not necessarily represent those of their affiliated organizations, or those of the publisher, the editors and the reviewers. Any product that may be evaluated in this article or claim that may be made by its manufacturer is not guaranteed or endorsed by the publisher.
Research integrity at Frontiers
Learn more about the work of our research integrity team to safeguard the quality of each article we publish.