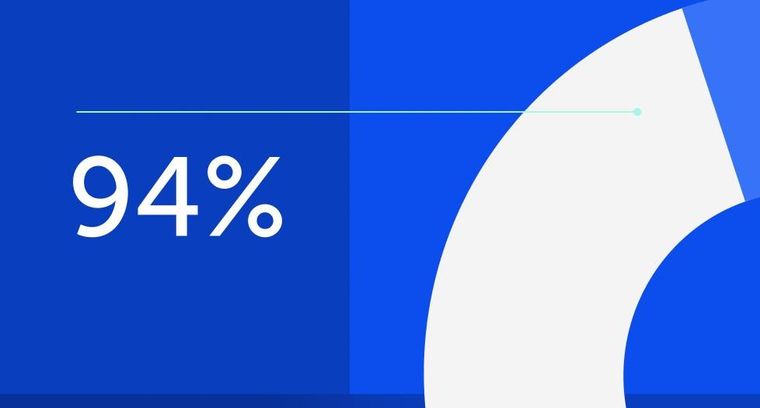
94% of researchers rate our articles as excellent or good
Learn more about the work of our research integrity team to safeguard the quality of each article we publish.
Find out more
REVIEW article
Front. Bioeng. Biotechnol., 27 March 2025
Sec. Nanobiotechnology
Volume 13 - 2025 | https://doi.org/10.3389/fbioe.2025.1566522
This article is part of the Research TopicNanodrug Delivery Strategies for Enhanced Cancer Chemo-ImmunotherapyView all 4 articles
Head and neck cancer (HNC) critically affects patient survival and quality of life, highlighting the need for optimized perioperative interventions. While conventional therapies face limitations in specificity and toxicity, nanotechnology has emerged as a revolutionary approach. Preoperative application of tumor-targeting nanoprobes enables molecular-level lesion identification via biomarker-specific conjugation and spatially resolved fluorescence quantification. Intraoperatively, fluorescent nanomaterials enhance surgical precision through selective tumor accumulation, delineating malignant margins in real time. Postoperatively, engineered nanocarriers improve therapeutic outcomes by delivering drugs with spatial control, minimizing off-target effects, and enabling multimodal synergies. These nanotechnology-driven strategies collectively address critical challenges in HNC management, including diagnostic sensitivity, intraoperative visualization, and postoperative recurrence. Their inherent advantages—precision targeting, reduced systemic toxicity, and multifunctional integration—establish them as cornerstone tools in modern oncology. Future advancements in nanomaterial design and biocompatibility are poised to further refine therapeutic efficacy, survival rates, and patient-centered outcomes.
Head and neck cancer (HNC) represents a major global health burden, with incidence rates steadily increasing worldwide (Stransky et al., 2011; Chow and Longo, 2020; Mody et al., 2021). According to the data collected by the World Health Organization, the annual number of newly diagnosed HNC cases across the globe exceeds 600,000, with approximately 300,000 deaths each year (Bray et al., 2024). The occurrence of HNC is associated with a multitude of factors (Leemans et al., 2018; Cramer et al., 2019; Galizia et al., 2022). These include behaviors such as smoking and excessive drinking, as well as the intake of betel nuts (Galizia et al., 2022). Additionally, infection with the human papillomavirus (HPV) is also identified as a contributing factor (Sabatini and Chiocca, 2019). In China, the incidence of HNC is notably high (Barsouk et al., 2023). This is particularly evident in certain regions, such as Hunan and Guangdong provinces, where the extensive consumption of betel nuts has led to a continually increasing incidence rate (Hu et al., 2017a). HNC has a deep impact on patients. It not only impairs the normal physiological functions of the oral cavity, including mastication, swallowing, and the ability to express speech, but also induces significant changes in patients’ physical appearance (Chen et al., 2014). These consequences collectively subject patients to substantial physical and psychological distress (Borggreven et al., 2005; Chen et al., 2014). Hence, the quest for strategies to improve the therapeutic outcomes of HNC and to enhance the survival prospects and quality of life of affected individuals has emerged as a notable area of focus within the medical research community (Miller and Shuman, 2016; Machiels et al., 2020).
The traditional treatment methods for HNC mainly include surgery, radiotherapy, and chemotherapy, etc (Miller and Shuman, 2016; Lechner et al., 2022; Elaheh Dalir et al., 2023). Surgery is the primary approach for treating HNC, aiming to achieve the therapeutic goal by removing the tumor tissues (Ferris et al., 2016; Burtness et al., 2019; Gillison et al., 2019). However, this approach is constrained by incomplete resection, high recurrence rates, and it can have a significant impact on patients’ oral functions and appearances (Ferris et al., 2016; Burtness et al., 2019; Gillison et al., 2019). Radiotherapy and chemotherapy, as adjuvant therapies for surgery, can improve the treatment outcomes (Gyawali et al., 2016). However, they also come with some side effects (Gyawali et al., 2016; Burtness et al., 2019; Gillison et al., 2019; Lechner et al., 2022). Radiotherapy may lead to oral mucosal damage, dry mouth, and hypogeusia, while chemotherapy can cause nausea, vomiting, hair loss, and bone marrow suppression (Mallick et al., 2015; Zhang et al., 2024). In addition, the treatment effects of traditional methods on some patients with advanced HNC are not satisfactory, and the survival rates and quality of life of these patients remain relatively low (de Roest et al., 2022; Goel et al., 2022; Lee et al., 2024). Given these limitations, there is an urgent need for innovative strategies that can enhance therapeutic precision while minimizing adverse effects. This has driven significant interest in nanomaterials and other advanced approaches, such as bioengineered hydrogels (Adine et al., 2024; Nie et al., 2025), which demonstrate promising potential in improving drug delivery, promoting tissue regeneration, and modulating the tumor microenvironment in HNC (Wu Y. et al., 2023; Zang et al., 2024).
Nanotechnology is an emerging technology that involves the control of substances at the nanoscale (Chaturvedi et al., 2019; Chehelgerdi et al., 2023; Malik et al., 2023). The application of nanomaterials in the medical field has brought new hopes for the treatment of HNC (Xu et al., 2022; Wu Q. et al., 2023; Tang et al., 2024; Wang et al., 2025). The unique advantages of nanomaterials include: (1) The size of nanomaterials generally ranges from 1–100 nm, which is similar to the size of biomolecules and cells (Shang et al., 2014; Navya et al., 2019; Liu R. et al., 2023). Thus, they can more easily penetrate cells and tissues, enabling precise treatment of tumor cells (Sykes et al., 2014; Shi et al., 2020; Lammers, 2024; Wang B. et al., 2024). (2) Nanomaterials have a relatively large specific surface area, which can increase drug loading capacity and the interaction with biomolecules, enhancing the efficacy of drugs (Xu et al., 2022; Tang et al., 2024; Wang et al., 2025). (3) Nanomaterials can be surface-modified with targeting molecules to achieve specific recognition and targeted treatment of tumor cells, thereby reducing damage to normal tissues (Hong et al., 2016; Zhu and Li, 2023). (4) Nanomaterials can be chemically modified and physically modified to endow them with various functions, such as fluorescence imaging, magnetic resonance imaging, photothermal therapy, etc., enabling the integration of tumor diagnosis and treatment (Smith and Gambhir, 2017; Liu et al., 2019; An et al., 2021). During the preoperative phase, nanomaterials enable precise diagnosis and targeted therapy, enhancing treatment efficacy and minimizing adverse effects. During surgery, nanoprobes facilitate real-time imaging and monitoring, assisting surgeons in achieving accurate tumor resection. Postoperatively, nanocarriers can deliver sustained-release drugs locally to prevent recurrence. This review systematically examines the advancements in nanomaterials for the perioperative management of HNC, focusing on three key aspects: preoperative diagnosis, intraoperative imaging, and postoperative recurrence management (Figure 1). By exploring these applications, we aim to provide new perspectives and strategies for improving HNC treatment outcomes and patient prognosis.
Figure 1. The scheme illustrates the application of nanomaterials for the perioperative management of HNC.
Quantum dots (QDs), as a kind of semiconductor nanocrystals, exhibit distinctive optical traits, high fluorescence intensity, excellent stability, and adjustable emission wavelengths (Moon et al., 2019; Gidwani et al., 2021; Shetty et al., 2024). Notably, the fluorescence emission wavelength of QDs is size-dependent, meaning that through exact control of their size, the emission of fluorescence across various wavelengths can be achieved (Fang et al., 2017). These remarkable properties make QDs to be the highly suitable biomarkers for the early detection of HNC (Shetty et al., 2024). The function of QD probes is to attach to the specific markers on the surface of HNC cells, thus contributing to the early diagnosis of this malignancy. For instance, Ya-Dong Li’s group has designed a QD-based probe that has the ability to bind to the epidermal growth factor receptor (EGFR) on the cell surface of HNC (Figure 2) (Yang, 2011). By monitoring the fluorescence signal emitted by the QDs, an early diagnosis of HNC can be effectively achieved. This application offers a novel approach to the early diagnosis of HNC, potentially enhancing the overall diagnostic accuracy and patient outcomes. The application of QD probes holds great promise in the field of HNC diagnosis, opening up new ways for more sensitive and specific detection methods (Xu et al., 2012; Yakavets et al., 2020).
Figure 2. Visible imaging of HNC animal model by QDs probe (A) Visible images of HNC animal model after intravenous injection with QD800-EGFR Ab probe containing 100 pmol equivalent of QD800 (B) Visible images of HNC animal model after intravenous injection with 100 pmol QD800 (C) Visible images of HNC animal model after intravenous injection with 250 µL of EGFR monoclonal antibody (1 mg/mL). Reprinted with permission from Yang (2011). Copyright 2011 Dovepress.
There are many kinds of nanomaterials used for constructing biosensors, such as metal nanoparticles, carbon nanomaterials, QDs, etc (Wong et al., 2020; Eivazzadeh-Keihan et al., 2022; Barhoum et al., 2023). These nanomaterials possess different properties and can be selected and constructed based on specific needs (Wong et al., 2020). For example, metal nanoparticles have excellent electrical conductivity and catalytic performance and can be used to construct electrochemical sensors (Azimzadeh et al., 2016). Carbon nanomaterials have good biocompatibility and electrical conductivity and can be used to build biosensors (Maiti et al., 2019; Mukherjee et al., 2024). Among them, biosensors achieve early diagnosis of HNC by interacting with HNC-related biomolecules to produce signal changes (Xue et al., 2018). For instance, a biosensor based on gold nanoparticles can bind to Rose Bengal (RB), which has been shown to have specificity for oral cancer through inhibition of DNA polymerase of HNC cells (RB-GNR) (Figure 3) (Wang et al., 2013). The RB-GNR platform has been tested on HNC cells, showing its dual functionality as both a sensor and an imaging probe. The sensing assay exhibits enhanced sensitivity and accuracy, while the imaging approach enables rapid, multi-channel detection. Both modes are highly efficient and convenient, highlighting the RB-GNR platform’s significant potential for cost-effective diagnostics in HNC.
Figure 3. Schematic illustration of the synthesis of RB-GNRs. Reprinted with permission from Wang et al. (2013). Copyright 2023 Elsevier.
Targeted nanoparticle imaging requires the selection of targeting molecules that are specific to the markers of HNC cells (Wiechec et al., 2017; Zhao et al., 2019; Chen et al., 2023). These markers include receptors, antigens, enzymes, etc. For example, for EGFR on the surface of HNC cells, anti-EGFR antibodies can be selected as the targeting molecules (Sacco and Cohen, 2015; Wiechec et al., 2017; Bhatia and Burtness, 2023). The enrichment mechanism of targeted nanoparticles in HNC tissues is mainly through the specific binding of the targeting molecules to the markers on the surface of cancer cells (Rahman et al., 2012; Colecchia et al., 2017; Huang et al., 2022). HNC presents significant surgical challenges, as it requires the preservation of healthy structures while ensuring complete tumor resection (Chow and Longo, 2020; Mody et al., 2021). Image-guided surgery has emerged as a promising approach to enhance tumor removal precision (Neilio and Ginat, 2024). Near-infrared (NIR) imaging-guided surgery, in particular, has been successfully applied in both small animal studies and clinical trials (Vahrmeijer et al., 2013; Li et al., 2021). Among the various advancements, gold nanoclusters (AuNCs) have shown great potential as renal-clearable nanoplatforms, offering promising applications in tumor diagnosis and therapy. For example, Cindy Colombé has synthesized AuNCs with different ligands and tested them in vitro and in vivo (Figure 4) (Colombé et al., 2019). They demonstrated that AuNCs exhibit multifunctional potential in various applications, including optical imaging, enhanced radiotherapy, and photothermal therapy. The combination of image-guided surgery with these therapeutic modalities could significantly improve treatment outcomes. Furthermore, the development of AuNCs with short-wave infrared (SWIR) emission and high quantum yield holds promise for further enhancing the contrast between tumor tissue and healthy tissue, potentially revolutionizing precision medicine approaches. These imaging techniques can provide important reference bases for surgical planning and improve the accuracy of surgeries.
Figure 4. Schematic illustration of the designed AuNCs for image-guided surgery. Reprinted with permission from Colombé et al. (2019). Copyright 2019 Elsevier.
The principle of multimodal nanoprobes combining multiple imaging modalities is to integrate probes of different imaging modalities onto a single nanoplatform (Kobayashi et al., 2007; Mallia et al., 2014; Ju et al., 2023). For instance, by integrating MRI probes and optical imaging probes onto one nanoplatform, multimodal imaging combining MRI and optical imaging can be achieved (Jing et al., 2014). Such multimodal imaging can improve the accuracy of defining tumor boundaries through the complementarity of different imaging information (Jing et al., 2014). Multimodal nanoprobes have made significant progress in preclinical studies of HNC(Muhanna et al., 2015). For example, Gang Zheng’s group has developed a multimodal nanoprobe (porphysomes) based on porphyrin-lipid, which can self-assemble into liposome-like nanoparticles (Figure 5) (Muhanna et al., 2015). Porphysomes demonstrate high stability in serum, with partial dissociation generates fluorescence, while the majority of porphysomes remain undegraded, making them highly effective for photoacoustic imaging. In the head and neck mice tumor model and rabbit tumor model, intravenous injection leads to clear visualization of primary tumors and metastatic lymph nodes via fluorescence and photoacoustic imaging 24 h later. Porphysomes enable local photothermal therapy (PTT) at tumor sites, effectively inhibiting tumor growth. With minimal barriers to clinical translation, these nanoparticles hold significant promise for providing precise and effective treatment options for patients with HNC. Its unique properties and multifunctionality position it as a promising candidate for advancing targeted cancer therapies.
Figure 5. Schematic illustration of multifunctional Porphysomes for rabbit HNC. (A) Intravenous administration of porphysomes via the rabbit ear vein. (B) Both fluorescence and photoacoustic imaging were enabled by porphysomes accumulated at 24 h post-injection. (C) Two-step photothermal ablation protocol combining intratumoral and transdermal irradiation. (D) Complete tumor elimination with no recurrence observed. Reprinted with permission from Muhanna et al. (2015). Copyright 2015 Ivyspring International.
Current imaging modalities are largely restricted to preoperative assessment, while intraoperative tumor margin determination still relies heavily on the surgeon’s tactile evaluation and frozen section analysis (Trotta et al., 2011). However, the efficacy of this method remains heavily dependent on the surgeon’s expertise and the tumor’s infiltrative growth pattern. To overcome these limitations, intraoperative imaging technologies, such as fluorescence-guided surgery (FGS) and cone-beam computed tomography (CBCT), are currently under investigation for real-time visualization of tumor margins (Curtin, 2012). FGS enhances detection accuracy of tumor margins through molecular-targeted fluorescence guidance, enabling real-time delineation of tumor boundaries. In contrast, CBCT enables intraoperative acquisition of real-time images, particularly offering advantages in surgeries involving complex bony structures such as the skull base, mandible, and maxilla (Trotta et al., 2011; Curtin, 2012). There exists a variety of fluorescent nanoparticles applicable to surgical navigation, such as persistent luminescent nanoparticles and the NIR fluorescence sensor (Craig et al., 2016; Yin et al., 2024; Zhong et al., 2024). The mechanism of specific accumulation of fluorescent nanoparticles in HNC tissues mainly depends on the specific binding between targeting molecules and surface markers of cancer cells. Under the excitation by near-infrared light, fluorescent nanoparticles emit fluorescent signals, which can be observed using fluorescent microscopes, endoscopes, and other devices. By real-time observation the distribution of fluorescent signals, the surgical resection process can be guided to ensure the complete excision of tumor tissues. Jonathan C. iris constructed a novel multi-modal liposomal contrast agent (CF800) to enable three-dimensional localization and soft-tissue visualization (Figure 6) (Muhanna et al., 2021). Significant enhancement in CBCT images was observed starting 24 h after CF800 administration, peaking at 96 h, and remaining notable at 120 h. The long half-life of CF800 supports its application for multiple intraoperative imaging sessions. Fluorescence-guided surgery enables real-time visualization of tumor boundaries, thereby enhancing surgical precision. Additionally, CF800 demonstrates a high signal-to-noise ratio in ex vivo imaging, which aids in the accurate assessment of surgical margins. These features collectively highlight CF800s potential to improve both intraoperative decision-making and postoperative evaluation in oncological surgeries.
Figure 6. Schematic illustration of dual modality fluorescent/CT contrast liposomal agent (CF800) for surgical navigation. (A) Photographic and 3D reconstructed views of rabbit VX2 buccal carcinoma model. (B) Representation of CF800 dual-modality liposomal nanoparticles co-encapsulating indocyanine green (ICG) for near-infrared fluorescence imaging and iohexol-based computed tomography (CT) contrast. (C) Intraoperative near-infrared fluorescence imaging. (D) Preoperative micro-CT (μCT) and intraoperative cone-beam CT (CBCT). (E) Ex vivo tissue fluorescence quantification. (F) Histopathological validation.Reprinted with permission from (Muhanna et al., 2021) Copyright 2021 Elsevier.
Despite continuous advancements in oncological surgery, microscopic residual disease (MRD) remains a significant factor contributing to cancer recurrence and metastasis (Ferrero et al., 2012; Sano et al., 2012). This issue is particularly common in aggressive cancers such as HNC (Sano et al., 2012). Current clinical methods, including palpation and imaging, lack the sensitivity to detect MRD effectively (Meier et al., 2005). Pathological analysis of surgical margins, while commonly used, is often slow and inaccurate. To eliminate potential MRD, surgeons frequently resort to removing large volumes of normal tissue (Loree and Strong, 1990; de Carvalho et al., 2012). This approach not only fails to guarantee complete tumor removal but also increases patient morbidity, reduces quality of life, and complicates postoperative treatments such as radiotherapy and chemotherapy. Moreover, MRD often develops resistance to these therapies, further diminishing patient survival rates.
Emerging diagnostic technologies have yet to achieve single-cell sensitivity and real-time detection of MRD in solid tissues (Mito et al., 2012). Optical methods, for instance, are limited to detecting larger surface tumors, while photoacoustic techniques lack sensitivity, speed, and specificity in solid tissues. Fluorescence methods lack the necessary sensitivity, and multispectral optoacoustic tomography cannot detect small clusters of cancer cells in real time within solid tissues (Razansky et al., 2011). Even when MRD is identified through frozen section pathology, standard surgical procedures often struggle to remove it without damaging critical organs (Chen and Karlan, 2000). These limitations highlight the critical need for novel nanomaterials enabling precise intraoperative detection and management of MRD.
Ekaterina Y. Lukianova-Hleb and colleagues have developed a plasmonic nanobubble (PNB) surgical technology for the intraoperative management of both resectable and unresectable tumors (Figure 7) (Lukianova-Hleb et al., 2016). This approach involves the local detection and resection of resectable MRD, as well as the mechanical destruction of unresectable MRD using cancer cell-specific PNBs. By combining systemic gold nanoparticle targeting, localized PNB generation with low-energy laser pulses, and acoustic detection, this technology offers a promising solution for real-time, in vivo detection and elimination of MRD during surgery. The high sensitivity and specificity of PNBs, combined with their compatibility with standard surgical protocols, could significantly improve surgical outcomes while minimizing morbidity.
Figure 7. Schematic illustration of a plasmonic nanobubble (PNB) surgical technology for the intraoperative management (A) Systemic delivery of gold conjugates to the tumour via their leaky vasculature (B) The acoustic signal of a PNB (illustrative red time response) reports even a single cancer cell in solid tissue, but not normal cells (illustrative green time response). Reprinted with permission from Lukianova-Hleb et al. (2016). Copyright 2016 Springer Nature.
Currently, researchers are developing advanced imaging technologies to achieve preoperative visualization of HNC(Heidari et al., 2020; Orosz et al., 2020). Among these, MRI is the preferred modality for evaluating primary tumors in the head and neck region due to its high spatial resolution for soft tissue structures (Freihat et al., 2020; Wu et al., 2021). However, the large size of MRI equipment and lengthy acquisition times limit its applicability as an intraoperative imaging guidance instrument (Radtke et al., 2016; Yip and Aerts, 2016; Arnold et al., 2022). In contrast, optical imaging methods offer several advantages, including high sensitivity, relatively low cost, and widespread availability (Gröhl et al., 2021; Park et al., 2023; Zhu et al., 2024). By taking advantage of the strengths of different imaging modalities, nanotechnology enables surgeons to more precisely delineate tumor margins both before and during surgery, thereby improving the completeness of tumor resection, particularly for HNC.
Surface-enhanced Raman scattering (SERS) technology, which uses roughened metallic surfaces as enhancing substrates, can boost Raman signals by a factor of 106 to 1010 compared to traditional Raman techniques (Hu et al., 2017b; Lin et al., 2023; Zhu et al., 2024). In addition to its exceptional signal enhancement, SERS offers multiplexing capabilities, high sensitivity, and enhanced photostability, making it a powerful tool for detecting molecular biomarkers and diagnosing tumors (Qiao et al., 2018; Kaur et al., 2022; Zhang et al., 2022). By employing molecular reporters with absorption energies similar to the incident laser, surface-enhanced resonance Raman spectroscopy (SERRS) further enhances signals by an additional 102 to 103 times (Gao and Weaver, 1987; Zong et al., 2018; Plou et al., 2022). As a result, SERRS not only inherits the advantages of SERS but also achieves significantly higher sensitivity, demonstrating immense potential for guiding tumor resection (Plou et al., 2022). The extremely high sensitivity of SERRS allows for real-time delineation of infiltrative tumor margins, while MRI excels in preoperative tumor localization and preliminary malignancy assessment. The combination of SERRS and MRI thus holds unprecedented potential for guiding tumor resection, such as in gliomas, enabling more precise and complete removal of tumor tissue. Xiaofeng Tao’s group developed a multifunctional MRI-SERRS nanoprobe (AuS-Cy7-Gd) (Sun et al., 2019), which combines the strengths of SERRS and MRI to improve the surgical management of HNC (Figure 8). The AuS-Cy7-Gd specifically accumulates in tumor tissues through the enhanced permeability and retention (EPR) effect, contributing to both preoperative MRI and intraoperative SERRS-guided surgery. SERRS-guided surgery enables precise identification of tumor margins and metastatic lymph nodes, reducing residual tumor tissue and enhancing the completeness of surgical resection, thereby prolonging patient survival.
Figure 8. Schematic illustration of the multifunctional MRI-SERRS nanoprobe (AuS-Cy7-Gd) for the intraoperative guided surgery. Reprinted with permission from Sun et al. (2019). Copyright 2019 Elsevier.
Surgical resection is widely regarded as the primary treatment option for HNC in clinical practice (Hamoir et al., 2018; Cramer et al., 2019; Mody et al., 2021). However, approximately 40%–60% of patients with advanced HNC experience local recurrence postoperatively, primarily due to the difficulty in completely eliminating residual tumor cells at the resection margins (Mahvi et al., 2018). Additionally, postoperative patients may develop localized immunosuppression, further aggravating the risk of recurrence (Mahvi et al., 2018). To reduce postoperative tumor recurrence, conventional chemotherapy and radiotherapy are frequently applied (Qiao et al., 2018; Bu et al., 2019; Petit et al., 2021); however, these approaches are limited by systemic toxicity, drug resistance, and immune system damage (Petit et al., 2021). In recent years, immune checkpoint blockade (ICB) antibody-based immunotherapy has attracted significant attention due to its promising long-term outcomes in preventing tumor recurrence (Ruan et al., 2019; Dolladille et al., 2020). ICB antibodies, such as programmed cell death protein 1 (PD-1) and its ligand programmed death-ligand 1 (PD-L1), have been proposed to alleviate the immunosuppressive tumor microenvironment (TME) (Kurtulus et al., 2019; Schnell et al., 2020). Despite their clinical efficacy, ICB therapies face several challenges, including poor tumor immunogenicity and immune-related systemic side effects (Chen et al., 2019; Kennedy and Salama, 2020; Zhou et al., 2021). Since the effectiveness of ICB therapy largely depends on sufficient tumor-infiltrating T Cells, converting “cold” tumors into immunogenic “hot” tumors represents a promising strategy to enhance immunotherapy outcomes (Chen et al., 2019; Chao et al., 2020). Furthermore, improving methods to leverage the immune system for localized delivery of immunotherapeutic agents may be crucial to stimulate robust antitumor immune responses and prevent local tumor recurrence. Photothermal therapy (PTT) is a non-invasive treatment modality that uses NIR light to irradiate photothermal agents, generating high thermal energy to eliminate tumor cells (Liu R. et al., 2023; Overchuk et al., 2023). Recent studies have demonstrated that PTT ablation can induce immunogenic cell death (ICD) in tumor cells, leading to the release of a wide range of tumor-associated antigens, damage-associated molecular patterns (DAMPs), and pro-inflammatory cytokines (Duan et al., 2018; Ma et al., 2019; Meier et al., 2024). Recent evidence suggests that a newly identified form of ICD, plays a significant role in enhancing antitumor immunity (Wang et al., 2017). Pyroptosis is characterized by cell swelling and the release of cellular contents, which further enhance immune responses (Wang et al., 2017; Xiao et al., 2021; Du et al., 2024). These findings highlight the potential of combining PTT with pyroptosis-inducing strategies to improve therapeutic outcomes by fostering a robust antitumor immune environment.
Our group rationally constructed a versatile implant (TB/αPD-1@AuNCs/OBC) for HNC tumor postoperative treatment (Figure 9) (Zhou et al., 2022). The TB/αPD-1@AuNCs/OBC under NIR irradiation allowed the combination of therapies with remarkable postoperative antitumor immunity to reduce locoregional recurrence and improve survival. We rationally constructed a versatile implant (TB/αPD-1@AuNCs/OBC) for HNC tumor postoperative treatment. The TB/αPD-1@AuNCs/OBC under NIR irradiation allowed the combination of therapies with remarkable postoperative antitumor immunity to reduce locoregional recurrence and improve survival.
Figure 9. Schematic illustration of the versatile OBC-based membrane for achieving a therapeutic effect in antitumor immunotherapy towards HNC postoperative treatment.
Nanomaterials achieves the precise identification of HNC and drug delivery by modifying nanocarriers with targeting molecules (Souza et al., 2019; Zhang et al., 2023; Tang et al., 2024). This precision-targeted therapy can increase the concentration of therapeutic drugs in tumor tissues and reduce damage to normal tissues, thus enhancing the treatment efficacy (Xu et al., 2015; Lee et al., 2018; Souza et al., 2019). In the treatment of HNC, precision-targeted treatment allows drugs to act more concentratedly on tumor cells, strengthening the killing effect on tumor cells while avoiding negative effects on normal tissues (Kitamura et al., 2020). For example, some studies have shown that targeted nano-drug delivery systems can significantly enhance the accumulation of chemotherapy drugs in tumor tissues and improve the uptake efficiency of tumor cells for drugs, thereby increasing the treatment effectiveness rate (Bhirde et al., 2009; Li et al., 2016; Hu et al., 2018; Song et al., 2020). Nanomaterial-mediated multimodal treatment, through the synergy of different treatment mechanisms, can more effectively kill tumor cells, inhibit tumor growth, recurrence, and metastasis, further improving the treatment outcome of HNC(Li et al., 2019; Zhou et al., 2022; Hu et al., 2024). Taking the combination of chemotherapy and photothermal therapy as an example (Nam et al., 2018; Wang et al., 2019; Li et al., 2023), the high temperature generated by photothermal treatment can disrupt the integrity of the tumor cell membrane and increase its permeability, enabling chemotherapy drugs to enter the interior of tumor cells more easily and enhancing the cytotoxic effect of chemotherapy drugs. Meanwhile, chemotherapy drugs can kill tumor cells that are insensitive to photothermal treatment. The two treatments cooperate with each other to form a synergistic effect and improve the killing efficiency of tumor cells. This synergy has been verified in experimental studies on HNC treatment, demonstrating a more superior treatment effect than single treatment modalities (Wang et al., 2019).
Nanomedicine carriers achieve the slow and continuous release of drugs in tumor tissues by controlling the drug release rate and site, which lowers the peak blood drug concentration and mitigates the toxic side effects of drugs on normal tissues throughout the body, thereby improving patients’ tolerance to treatment (Qiu et al., 2018; Moradi Kashkooli et al., 2020; Liu et al., 2024). For example, Poly (lactic-co-glycolic acid) (PLGA) nanoparticles have been extensively used for the delivery of anticancer drugs. However, their application is often limited by challenges such as rapid clearance, burst release, and uncontrolled drug diffusion into surrounding tissues. Chitosan/β-glycerophosphate (CS/GP) thermosensitive hydrogels, known for their biocompatibility and biodegradability, have emerged as a promising platform for drug delivery and tissue engineering. By encapsulating drug-loaded PLGA nanoparticles within CS/GP hydrogels, the solubility and bioavailability of the therapeutic agents can be significantly enhanced. This hybrid system enables sustained and controlled drug release, improves local drug delivery and retention, and consequently enhances the therapeutic efficacy in HNC.
This approach represents a significant advancement in overcoming the limitations of conventional nanoparticle-based drug delivery systems to enhance the treatment effect while reducing the adverse impacts on patients’ bodies.
Nanotechnology contributes to more precise tumor resection and better preservation of oral organ functions by providing real-time and accurate information on tumor boundaries and surgical guidance. It reduces damage to surrounding normal tissues such as nerves and blood vessels (Ghosh et al., 2014; Jin et al., 2016; Zhu et al., 2022). For example, in HNC surgery, the use of fluorescent nanomaterials to mark the tumor boundaries enables surgeons to clearly visualize the extent of the tumor during the operation, preventing accidental resection of normal surrounding tissues (Lukianova-Hleb et al., 2016). This precise surgical operation not only increases the success rate of the surgery but also may decrease the occurrence of postoperative complications. Meanwhile, better preservation of oral organ functions is of great significance for patients’ quality of life. Patients can maintain better masticatory, swallowing, and speech functions after the operation, enhancing their self-care ability and quality of life.
The metabolic pathways and distribution of nanomaterials within the human body are rather complex following administration (Zhao and Castranova, 2011; Navya et al., 2019; Hussain et al., 2020). Some nanomaterials may accumulate in organs such as the liver and spleen, and long-term accumulation might potentially affect the functions of these organs (Hussain et al., 2020). For example, certain metal nanoparticles could trigger an inflammatory response in the liver and impact its normal metabolic function (Noga et al., 2023). In addition, nanomaterials may also enter other tissues and organs through the bloodstream, causing damage to them (Attarilar et al., 2020; Sengul and Asmatulu, 2020). Research has shown that the physicochemical properties of nanomaterials, such as size, shape, and surface charge, can influence their metabolism and distribution in the body (Haripriyaa and Suthindhiran, 2023). Nanomaterials with a smaller size may more easily penetrate biological membranes and enter the interior of cells, thus affecting the physiological functions of cells. Evaluating the safety of nanomaterials demands a deep understanding of their toxicity mechanism (Li et al., 2022). The toxicity of nanomaterials may stem from their interactions with biomolecules, leading to cytotoxicity, inflammatory responses, oxidative stress, etc (Li et al., 2022). For instance, the charge on the surface of nanomaterials may interact with the charge on the cell membrane surface, resulting in an increase in the permeability of the cell membrane, allowing the leakage of intracellular substances and subsequently affecting the normal physiological functions of cells. Moreover, nanomaterials may also react with biomolecules such as intracellular proteins and nucleic acids, interfering with their normal structures and functions. Some nanomaterials may induce cells to generate oxidative stress responses, producing a large amount of reactive oxygen species (ROS) (Li et al., 2014). These ROS will cause damage to the cell’s DNA, proteins, and lipids, leading to cell apoptosis or necrosis (Li et al., 2014).
Currently, there are various methods for preparing nanomaterials, such as physical, chemical, and biological methods (Forsberg, 2011; Abram et al., 2025). Different preparation methods result in variations in the properties of nanomaterials (Forsberg, 2011; Abram et al., 2025). For example, nanomaterials prepared by physical methods may possess good crystallinity and high purity, but the particle size distribution might be relatively wide. Nanomaterials prepared by chemical methods can precisely control the particle size and shape, yet some impurities may be introduced. These differences affect the application efficacy of nanomaterials in the treatment of HNC. Therefore, it is necessary to compare and evaluate different preparation methods to select the most suitable one for the treatment of HNC.
Future multifunctional nanomaterials are expected to combine diagnosis, treatment, and monitoring into a single platform. For instance, nanoplatforms could integrate chemotherapeutic drugs, tumor-targeting ligands, and fluorescence imaging agents to achieve precise diagnosis, personalized therapy, and real-time treatment monitoring for HNC. Such designs align with recent advances in soft monomicelle-directed synthesis, where amphiphilic block copolymers enable precise control over nanoparticle architectures for tailored drug delivery and imaging functionalities. (Wang J. et al., 2024). These platforms could further incorporate redox-responsive linkages, as demonstrated by disulfide-bridged prodrug systems (MTX@LND NPs), which release drugs selectively in tumor microenvironments via glutathione (GSH)-triggered disassembly (Hua et al., 2024). By combining these strategies, multifunctional nanoplatforms can synergize chemotherapy, targeted delivery, and imaging, reducing treatment costs and improving patient outcomes.
Moreover, with the continuous development of gene detection technology, it is anticipated that genotyping will be combined with nanomaterials in the future to provide more personalized treatment protocols for patients with HNC. Genotyping-guided nanomedicine could leverage protein-based nanoparticles, which offer inherent biocompatibility and customizable surfaces for tumor-specific targeting (Liu H. et al., 2023). For example, albumin nanoparticles modified with molecular markers (such as EGFR, CD44) could deliver drug combinations optimized for individual genetic profiles, minimizing off-target effects. This approach mirrors the precision of redox-responsive nanosystems, where stimuli-triggered release enhances therapeutic specificity (Hua et al., 2024). By personalizing nanomedicines, the effectiveness and safety of treatment can be enhanced, and the occurrence of adverse reactions can be reduced.
The profound integration of nanomaterials with medical imaging and bioengineering will bring more innovative solutions to the perioperative treatment of HNC. In the field of imaging, nanomaterials can be used to develop novel nano-imaging probes such as protein-based MRI contrast agents (Hua et al., 2024), enhancing the diagnostic accuracy and visualization of tumors. In the aspect of engineering, nanomaterials can be combined with tissue engineering to develop nanomaterials and scaffolds that are more suitable for the repair and regeneration of HNC issues. The perioperative treatment of HNC involves multiple disciplinary fields, including oral and maxillofacial surgery, medical oncology, radiology, nanomaterials experts, bioengineers, etc. Multidisciplinary team collaboration is essential to optimize nanomaterials applications in HNC perioperative care. Through the close collaboration of interdisciplinary teams, the advantages of each field can be fully used to jointly formulate more scientific and reasonable treatment plans. For instance, oral and maxillofacial surgeons can put forward requirements for nanomaterials based on surgical needs. Nanomaterials experts can design responsive nanomaterials using techniques like photo-reduction synthesis (Hua et al., 2024) or redox-sensitive assembly (Liu H. et al., 2023) according to these requirements. Medical oncologists can propose the design ideas of nano-drugs in combination with the characteristics of chemotherapy drugs. Radiologists can evaluate the treatment effect by using imaging techniques. This multidisciplinary team cooperation model will help accelerate the translation of nanomaterials from basic research to clinical practice and improve the treatment effect of patients with HNC.
In summary, nanomaterials have demonstrated remarkable application potential at every stage of HNC perioperative management (Table 1). In the preoperative diagnosis, the application of nanoprobes in defining tumor boundaries has enhanced the accuracy of diagnosis and the ability to detect tumors at an early stage, providing more precise information for surgical treatment. During the surgical navigation, the use of fluorescent nanomaterials and nanotechnology-assisted surgical instruments has improved the precision and visualization of the surgery, reducing the damage to surrounding normal tissues. In the postoperative adjuvant treatment, the targeted nano drug delivery systems and the combined treatment mediated by nanomaterials have increased the treatment efficacy and decreased the incidence of complications. With the continuous development and innovation of nanomaterials, the future treatment of HNC will show a trend towards more personalized, precise, and efficient approaches. The research and development of new nanomaterials and technologies will offer more options and possibilities for the diagnosis and treatment of HNC. The optimization of the multidisciplinary combined treatment model will integrate the advantageous resources of various fields to provide more comprehensive and integrated treatment services for patients. It is believed that in the near future, nanomaterials will play a more crucial role in the perioperative treatment of HNC, bringing better treatment outcomes and quality of life to patients.
Table 1. Representative nanomaterials summarized in this review for different stages of perioperative HNC treatment.
J-JZ: Writing–original draft, Writing–review and editing. Y-CF: Writing–review and editing. M-LZ: Writing–review and editing. QG: Writing–review and editing. X-BZ: Writing–review and editing.
The author(s) declare that no financial support was received for the research and/or publication of this article.
We wish to thank the help of the FreePik to modify Fig.1.
The authors declare that the research was conducted in the absence of any commercial or financial relationships that could be construed as a potential conflict of interest.
The authors declare that no Generative AI was used in the creation of this manuscript.
All claims expressed in this article are solely those of the authors and do not necessarily represent those of their affiliated organizations, or those of the publisher, the editors and the reviewers. Any product that may be evaluated in this article, or claim that may be made by its manufacturer, is not guaranteed or endorsed by the publisher.
Abram, S. L., Tavernaro, I., Johnston, L. J., Zou, S., and Resch-Genger, U. (2025). Nanoscale reference and test materials for the validation of characterization methods for engineered nanomaterials — current state, limitations, and needs. Anal. Bioanal. Chem. doi:10.1007/s00216-024-05719-6
Adine, C., Fernando, K., Ho, N. C. W., Quah, H. S., Ho, S. S. W., Wu, K. Z., et al. (2024). Bioengineered hydrogels enhance ex vivo preservation of patient-derived tumor explants for drug evaluation. Biomaterials 305, 122460. doi:10.1016/j.biomaterials.2023.122460
An, D., Fu, J., Zhang, B., Xie, N., Nie, G., Ågren, H., et al. (2021). NIR-II responsive inorganic 2D nanomaterials for cancer photothermal therapy: recent advances and future challenges. Adv. Funct. Mater. 31 (32). doi:10.1002/adfm.202101625
Arnold, T. C., Freeman, C. W., Litt, B., and Stein, J. M. (2022). Low-field MRI: clinical promise and challenges. J. Magnetic Reson. Imaging 57 (1), 25–44. doi:10.1002/jmri.28408
Attarilar, S., Yang, J., Ebrahimi, M., Wang, Q., Liu, J., Tang, Y., et al. (2020). The toxicity phenomenon and the related occurrence in metal and metal oxide nanoparticles: a brief review from the biomedical perspective. Front. Bioeng. Biotechnol. 8, 822. doi:10.3389/fbioe.2020.00822
Azimzadeh, M., Rahaie, M., Nasirizadeh, N., Ashtari, K., and Naderi-Manesh, H. (2016). An electrochemical nanobiosensor for plasma miRNA-155, based on graphene oxide and gold nanorod, for early detection of breast cancer. Biosens. Bioelectron. 77, 99–106. doi:10.1016/j.bios.2015.09.020
Barhoum, A., Altintas, Z., Devi, K. S. S., and Forster, R. J. (2023). Electrochemiluminescence biosensors for detection of cancer biomarkers in biofluids: principles, opportunities, and challenges. Nano Today 50, 101874. doi:10.1016/j.nantod.2023.101874
Barsouk, A., Aluru, J. S., Rawla, P., Saginala, K., and Barsouk, A. (2023). Epidemiology, risk factors, and prevention of head and neck squamous cell carcinoma. Med. Sci. 11 (2), 42. doi:10.3390/medsci11020042
Bhatia, A., and Burtness, B. (2023). Treating head and neck cancer in the age of immunotherapy: a 2023 update. Drugs 83 (3), 217–248. doi:10.1007/s40265-023-01835-2
Bhirde, A. A., Patel, V., Gavard, J., Zhang, G. F., Sousa, A. A., Masedunskas, A., et al. (2009). Targeted killing of cancer Cells in Vivo and in vitrowith EGF-directed carbon nanotube-based drug delivery and with EGF-directed carbon nanotube-based drug delivery. ACS Nano 3 (2), 307–316. doi:10.1021/nn800551s
Borggreven, P. A., Verdonck-De Leeuw, I., Langendijk, J. A., Doornaert, P., Koster, M. N., De Bree, R., et al. (2005). Speech outcome after surgical treatment for oral and oropharyngeal cancer: a longitudinal assessment of patients reconstructed by a microvascular flap. Head and Neck 27 (9), 785–793. doi:10.1002/hed.20236
Bray, F., Laversanne, M., Sung, H., Ferlay, J., Siegel, R. L., Soerjomataram, I., et al. (2024). Global cancer statistics 2022: GLOBOCAN estimates of incidence and mortality worldwide for 36 cancers in 185 countries. CA A Cancer J. Clin. 74 (3), 229–263. doi:10.3322/caac.21834
Bu, L. L., Yan, J. J., Wang, Z. J., Ruan, H. T., Chen, Q., Gunadhi, V., et al. (2019). Advances in drug delivery for post-surgical cancer treatment. Biomaterials 219, 119182. doi:10.1016/j.biomaterials.2019.04.027
Burtness, B., Harrington, K. J., Greil, R., Soulières, D., Tahara, M., De Castro, G., et al. (2019). Pembrolizumab alone or with chemotherapy versus cetuximab with chemotherapy for recurrent or metastatic squamous cell carcinoma of the head and neck (KEYNOTE-048): a randomised, open-label, phase 3 study. Lancet 394 (10212), 1915–1928. doi:10.1016/s0140-6736(19)32591-7
Chao, Y., Liang, C., Tao, H. Q., Du, Y. R., Wu, D., Dong, Z. L., et al. (2020). Localized cocktail chemoimmunotherapy after in situ gelation to trigger robust systemic antitumor immune responses. Sci. Adv. 6 (10), eaaz4204. doi:10.1126/sciadv.aaz4204
Chaturvedi, V. K., Singh, A., Singh, V. K., and Singh, M. P. (2019). Cancer nanotechnology: a new revolution for cancer diagnosis and therapy. Curr. Drug Metab. 20 (6), 416–429. doi:10.2174/1389200219666180918111528
Chehelgerdi, M., Chehelgerdi, M., Allela, O. Q. B., Pecho, R. D. C., Jayasankar, N., Rao, D. P., et al. (2023). Progressing nanotechnology to improve targeted cancer treatment: overcoming hurdles in its clinical implementation. Mol. Cancer 22 (1), 169. doi:10.1186/s12943-023-01865-0
Chen, A. M., Daly, M. E., Farwell, D. G., Vazquez, E., Courquin, J., Lau, D. H., et al. (2014). Quality of life among long-term survivors of head and neck cancer treated by intensity-modulated radiotherapy. JAMA Otolaryngology–Head and Neck Surg. 140 (2), 129. doi:10.1001/jamaoto.2013.5988
Chen, L.-M., and Karlan, B. Y. (2000). Recurrent ovarian carcinoma: is there a place for surgery? Seminars Surg. Oncol. 19 (1), 62–68. doi:10.1002/1098-2388(200007/08)19:1<62::Aid-ssu10>3.0.Co;2-o
Chen, Q., Chen, M., and Liu, Z. (2019). Local biomaterials-assisted cancer immunotherapy to trigger systemic antitumor responses. Chem. Soc. Rev. 48 (22), 5506–5526. doi:10.1039/c9cs00271e
Chen, S., Yang, Y., He, S., Lian, M., Wang, R., and Fang, J. (2023). Review of biomarkers for response to immunotherapy in HNSCC microenvironment. Front. Oncol. 13, 1037884. doi:10.3389/fonc.2023.1037884
Chow, L. Q. M., and Longo, D. L. (2020). Head and neck cancer. N. Engl. J. Med. 382 (1), 60–72. doi:10.1056/NEJMra1715715
Colecchia, D., Nicolato, E., Ravagli, C., Faraoni, P., Strambi, A., Rossi, M., et al. (2017). EGFR-targeted magnetic nanovectors recognize, in vivo, head and neck squamous cells carcinoma-derived tumors. ACS Med. Chem. Lett. 8 (12), 1230–1235. doi:10.1021/acsmedchemlett.7b00278
Colombé, C., Le Guével, X., Martin-Serrano, A., Henry, M., Porret, E., Comby-Zerbino, C., et al. (2019). Gold nanoclusters as a contrast agent for image-guided surgery of head and neck tumors. Nanomedicine Nanotechnol. Biol. Med. 20, 102011. doi:10.1016/j.nano.2019.04.014
Craig, S. E. L., Wright, J., Sloan, A. E., and Brady-Kalnay, S. M. (2016). Fluorescent-guided surgical resection of glioma with targeted molecular imaging agents: a literature review. World Neurosurg. 90, 154–163. doi:10.1016/j.wneu.2016.02.060
Cramer, J. D., Burtness, B., Le, Q. T., and Ferris, R. L. (2019). The changing therapeutic landscape of head and neck cancer. Nat. Rev. Clin. Oncol. 16 (11), 669–683. doi:10.1038/s41571-019-0227-z
Curtin, H. D. (2012). Posttreatment CT and MR imaging in head and neck cancer: what the radiologist needs to know commentary. Radiographics 32 (5), 1282–1284. doi:10.1148/Radiographics.32.5.125102
De Carvalho, A. C., Kowalski, L. P., Campos, A. H. J. F. M., Soares, F. A., Carvalho, A. L., and Vettore, A. L. (2012). Clinical significance of molecular alterations in histologically negative surgical margins of head and neck cancer patients. Oral Oncol. 48 (3), 240–248. doi:10.1016/j.oraloncology.2011.10.018
De Roest, R. H., Van Der Heijden, M., Wesseling, F. W. R., De Ruiter, E. J., Heymans, M. W., Terhaard, C., et al. (2022). Disease outcome and associated factors after definitive platinum based chemoradiotherapy for advanced stage HPV-negative head and neck cancer. Radiotherapy Oncol. 175, 112–121. doi:10.1016/j.radonc.2022.08.013
Dolladille, C., Ederhy, S., Sassier, M., Cautela, J., Thuny, F., Cohen, A. A., et al. (2020). Immune checkpoint inhibitor rechallenge after immune-related adverse events in patients with cancer. Jama Oncol. 6 (6), 865–871. doi:10.1001/jamaoncol.2020.0726
Du, G., Healy, L. B., David, L., Walker, C., El-Baba, T. J., Lutomski, C. A., et al. (2024). ROS-dependent S-palmitoylation activates cleaved and intact gasdermin D. Nature 630 (8016), 437–446. doi:10.1038/s41586-024-07373-5
Duan, X., Chan, C., and Lin, W. (2018). Nanoparticle-mediated immunogenic cell death enables and potentiates cancer immunotherapy. Angew. Chem. Int. Ed. 58 (3), 670–680. doi:10.1002/anie.201804882
Eivazzadeh-Keihan, R., Bahojb Noruzi, E., Chidar, E., Jafari, M., Davoodi, F., Kashtiaray, A., et al. (2022). Applications of carbon-based conductive nanomaterials in biosensors. Chem. Eng. J. 442, 136183. doi:10.1016/j.cej.2022.136183
Elaheh Dalir, A., Shahin, A., Sepideh, B., Faezeh Jafarmadar, G., Negar Ghorbani, J., Sepideh, Z. V., et al. (2023). Effect of curcumin on the head and neck squamous cell carcinoma cell line HN5. Curr. Mol. Pharmacol. 16 (3), 374–380. doi:10.2174/1874467215666220414143441
Fang, M., Chen, M., Liu, L. L., and Li, Y. (2017). Applications of quantum dots in cancer detection and diagnosis: a review. J. Biomed. Nanotechnol. 13 (1), 1–16. doi:10.1166/jbn.2017.2334
Ferrero, A., Langella, S., Russolillo, N., Vigano’, L., Lo Tesoriere, R., and Capussotti, L. (2012). Intraoperative detection of disappearing colorectal liver metastases as a predictor of residual disease. J. Gastrointest. Surg. 16 (4), 806–814. doi:10.1007/s11605-011-1810-5
Ferris, R. L., Blumenschein, G., Fayette, J., Guigay, J., Colevas, A. D., Licitra, L., et al. (2016). Nivolumab for recurrent squamous-cell carcinoma of the head and neck. N. Engl. J. Med. 375 (19), 1856–1867. doi:10.1056/NEJMoa1602252
Forsberg, E.-M. (2011). Standardisation in the field of nanotechnology: some issues of legitimacy. Sci. Eng. Ethics 18 (4), 719–739. doi:10.1007/s11948-011-9268-0
Freihat, O., Pinter, T., Kedves, A., Sipos, D., Cselik, Z., Repa, I., et al. (2020). Diffusion-weighted imaging (DWI) derived from PET/MRI for lymph node assessment in patients with head and neck squamous cell carcinoma (HNSCC). Cancer Imaging 20 (1), 56. doi:10.1186/s40644-020-00334-x
Galizia, D., Minei, S., Maldi, E., Chilà, G., Polidori, A., and Merlano, M. C. (2022). How risk factors affect head and neck squamous cell carcinoma (HNSCC) tumor immune microenvironment (TIME): their influence on immune escape mechanisms and immunotherapy strategy. Biomedicines 10 (10), 2498. doi:10.3390/biomedicines10102498
Gao, P., and Weaver, M. J. (1987). Surface-enhanced Raman-spectroscopy as a vibrational probe of electroorganic reaction-mechanisms. Abstr. Pap. Am. Chem. Soc. 194. 250-Coll.
Ghosh, D., Bagley, A. F., Na, Y. J., Birrer, M. J., Bhatia, S. N., and Belcher, A. M. (2014). Deep, noninvasive imaging and surgical guidance of submillimeter tumors using targeted M13-stabilized single-walled carbon nanotubes. Proc. Natl. Acad. Sci. 111 (38), 13948–13953. doi:10.1073/pnas.1400821111
Gidwani, B., Sahu, V., Shukla, S. S., Pandey, R., Joshi, V., Jain, V. K., et al. (2021). Quantum dots: prospectives, toxicity, advances and applications. J. Drug Deliv. Sci. Technol. 61, 102308. doi:10.1016/j.jddst.2020.102308
Gillison, M. L., Trotti, A. M., Harris, J., Eisbruch, A., Harari, P. M., Adelstein, D. J., et al. (2019). Radiotherapy plus cetuximab or cisplatin in human papillomavirus-positive oropharyngeal cancer (NRG Oncology RTOG 1016): a randomised, multicentre, non-inferiority trial. Lancet 393 (10166), 40–50. doi:10.1016/s0140-6736(18)32779-x
Goel, B., Tiwari, A. K., Pandey, R. K., Singh, A. P., Kumar, S., Sinha, A., et al. (2022). Therapeutic approaches for the treatment of head and neck squamous cell carcinoma–An update on clinical trials. Transl. Oncol. 21, 101426. doi:10.1016/j.tranon.2022.101426
Gröhl, J., Schellenberg, M., Dreher, K., and Maier-Hein, L. (2021). Deep learning for biomedical photoacoustic imaging: a review. Photoacoustics 22, 100241. doi:10.1016/j.pacs.2021.100241
Gyawali, B., Shimokata, T., Honda, K., and Ando, Y. (2016). Chemotherapy in locally advanced head and neck squamous cell carcinoma. Cancer Treat. Rev. 44, 10–16. doi:10.1016/j.ctrv.2016.01.002
Hamoir, M., Schmitz, S., Suarez, C., Strojan, P., Hutcheson, K. A., Rodrigo, J. P., et al. (2018). The current role of salvage surgery in recurrent head and neck squamous cell carcinoma. Cancers 10 (8), 267. doi:10.3390/cancers10080267
Haripriyaa, M., and Suthindhiran, K. (2023). Pharmacokinetics of nanoparticles: current knowledge, future directions and its implications in drug delivery. Future J. Pharm. Sci. 9 (1). doi:10.1186/s43094-023-00569-y
Heidari, A. E., Pham, T. T., Ifegwu, I., Burwell, R., Armstrong, W. B., Tjoson, T., et al. (2020). The use of optical coherence tomography and convolutional neural networks to distinguish normal and abnormal oral mucosa. J. Biophot. 13 (3), e201900221. doi:10.1002/jbio.201900221
Hong, E. J., Choi, D. G., and Shim, M. S. (2016). Targeted and effective photodynamic therapy for cancer using functionalized nanomaterials. Acta Pharm. Sin. B 6 (4), 297–307. doi:10.1016/j.apsb.2016.01.007
Hu, H., Wang, X., Zhao, Y., Yan, J., Li, S., Li, C., et al. (2024). Self-Assembled R848/Chlorin e6 Nanoparticles for Combinatorial Immunotherapy of Head and Neck Squamous Cell Carcinoma. ACS Appl. Nano Mater. 7 (7), 7966–7977. doi:10.1021/acsanm.4c00579
Hu, Q., Li, H., Wang, L., Gu, H., and Fan, C. (2018). DNA nanotechnology-enabled drug delivery systems. Chem. Rev. 119 (10), 6459–6506. doi:10.1021/acs.chemrev.7b00663
Hu, Y., Chen, J., Zhong, W., Ling, T., Jian, X., Lu, R., et al. (2017a). Trend analysis of betel nut-associated oral cancer and health burden in China. Chin. J. Dent. Res. 20 (2), 69–78. doi:10.3290/j.cjdr.a38271
Hu, Y., Cheng, H., Zhao, X., Wu, J., Muhammad, F., Lin, S., et al. (2017b). Surface-enhanced Raman scattering active gold nanoparticles with enzyme-mimicking activities for measuring glucose and lactate in living tissues. ACS Nano 11 (6), 5558–5566. doi:10.1021/acsnano.7b00905
Hua, Y., Qin, Z., Gao, L., Zhou, M., Xue, Y., Li, Y., et al. (2024). Protein nanoparticles as drug delivery systems for cancer theranostics. J. Control. Release 371, 429–444. doi:10.1016/j.jconrel.2024.06.004
Huang, Z., Chen, Y., Chen, R., Zhou, B., Wang, Y., Hong, L., et al. (2022). HPV enhances HNSCC chemosensitization by inhibiting SERPINB3 expression to disrupt the fanconi anemia pathway. Adv. Sci. 10 (1), e2202437. doi:10.1002/advs.202202437
Hussain, Z., Thu, H. E., Haider, M., Khan, S., Sohail, M., Hussain, F., et al. (2020). A review of imperative concerns against clinical translation of nanomaterials: unwanted biological interactions of nanomaterials cause serious nanotoxicity. J. Drug Deliv. Sci. Technol. 59, 101867. doi:10.1016/j.jddst.2020.101867
Jin, C. S., Wada, H., Anayama, T., Mcveigh, P. Z., Hu, H. P., Hirohashi, K., et al. (2016). An integrated nanotechnology-enabled transbronchial image-guided intervention strategy for peripheral lung cancer. Cancer Res. 76 (19), 5870–5880. doi:10.1158/0008-5472.Can-15-3196
Jing, L., Ding, K., Kershaw, S. V., Kempson, I. M., Rogach, A. L., and Gao, M. (2014). Magnetically engineered semiconductor quantum dots as multimodal imaging probes. Adv. Mater. 26 (37), 6367–6386. doi:10.1002/adma.201402296
Ju, J., Xu, D., Mo, X., Miao, J., Xu, L., Ge, G., et al. (2023). Multifunctional polysaccharide nanoprobes for biological imaging. Carbohydr. Polym. 317, 121048. doi:10.1016/j.carbpol.2023.121048
Kaur, B., Kumar, S., and Kaushik, B. K. (2022). Recent advancements in optical biosensors for cancer detection. Biosens. Bioelectron. 197, 113805. doi:10.1016/j.bios.2021.113805
Kennedy, L. B., and Salama, A. K. S. (2020). A review of cancer immunotherapy toxicity. Ca-a Cancer J. Clin. 70 (2), 86–104. doi:10.3322/caac.21596
Kitamura, N., Sento, S., Yoshizawa, Y., Sasabe, E., Kudo, Y., and Yamamoto, T. (2020). Current trends and future prospects of molecular targeted therapy in head and neck squamous cell carcinoma. Int. J. Mol. Sci. 22 (1), 240. doi:10.3390/ijms22010240
Kobayashi, H., Koyama, Y., Barrett, T., Hama, Y., Regino, C. a. S., Shin, I. S., et al. (2007). Multimodal nanoprobes for radionuclide and five-color near-infrared optical lymphatic imaging. ACS Nano 1 (4), 258–264. doi:10.1021/nn700062z
Kurtulus, S., Madi, A., Escobar, G., Klapholz, M., Nyman, J., Christian, E., et al. (2019). Checkpoint blockade immunotherapy induces dynamic changes in PD-1−cd8+ tumor-infiltrating T cells. Immunity 50 (1), 181–194.e6. doi:10.1016/j.immuni.2018.11.014
Lammers, T. (2024). Nanomedicine tumor targeting. Adv. Mater. 36 (26), e2312169. doi:10.1002/adma.202312169
Lechner, M., Liu, J., Masterson, L., and Fenton, T. R. (2022). HPV-associated oropharyngeal cancer: epidemiology, molecular biology and clinical management. Nat. Rev. Clin. Oncol. 19 (5), 306–327. doi:10.1038/s41571-022-00603-7
Lee, A. M., Weaver, A. N., Acosta, P., Harris, L., and Bowles, D. W. (2024). Review of current and future medical treatments in head and neck squamous cell carcinoma. Cancers 16 (20), 3488. doi:10.3390/cancers16203488
Lee, Y. T., Tan, Y. J., and Oon, C. E. (2018). Molecular targeted therapy: treating cancer with specificity. Eur. J. Pharmacol. 834, 188–196. doi:10.1016/j.ejphar.2018.07.034
Leemans, C. R., Snijders, P. J. F., and Brakenhoff, R. H. (2018). The molecular landscape of head and neck cancer. Nat. Rev. Cancer 18 (5), 269–282. doi:10.1038/nrc.2018.11
Li, B., Zhang, T., and Tang, M. (2022). Toxicity mechanism of nanomaterials: focus on endoplasmic reticulum stress. Sci. Total Environ. 834, 155417. doi:10.1016/j.scitotenv.2022.155417
Li, H., Kim, D., Yao, Q., Ge, H., Chung, J., Fan, J., et al. (2021). Activity-based NIR enzyme fluorescent probes for the diagnosis of tumors and image-guided surgery. Angew. Chem. Int. Ed. 60 (32), 17268–17289. doi:10.1002/anie.202009796
Li, H., Li, X., Shi, X., Li, Z., and Sun, Y. (2019). Effects of magnetic dihydroartemisinin nano-liposome in inhibiting the proliferation of head and neck squamous cell carcinomas. Phytomedicine 56, 215–228. doi:10.1016/j.phymed.2018.11.007
Li, J., Chang, X., Chen, X., Gu, Z., Zhao, F., Chai, Z., et al. (2014). Toxicity of inorganic nanomaterials in biomedical imaging. Biotechnol. Adv. 32 (4), 727–743. doi:10.1016/j.biotechadv.2013.12.009
Li, J., Chen, L., Liu, N., Li, S. N., Hao, Y. L., and Zhang, X. N. (2016). EGF-coated nano-dendriplexes for tumor-targeted nucleic acid delivery in vivo. Drug Deliv. 23 (5), 1718–1725. doi:10.3109/10717544.2015.1004381
Li, R., Liu, C., Wan, C., Liu, T., Zhang, R., Du, J., et al. (2023). A targeted and pH-responsive nano-graphene oxide nanoparticle loaded with doxorubicin for synergetic chemo-photothermal therapy of oral squamous cell carcinoma. Int. J. Nanomedicine 18, 3309–3324. doi:10.2147/ijn.S402249
Lin, C., Li, Y., Peng, Y., Zhao, S., Xu, M., Zhang, L., et al. (2023). Recent development of surface-enhanced Raman scattering for biosensing. J. Nanobiotechnology 21 (1), 149. doi:10.1186/s12951-023-01890-7
Liu, H., Nie, T., Duan, X., Zhang, X., Zheng, Y., Zhong, W., et al. (2023a). Cerebral delivery of redox-responsive lenalidomide prodrug plus methotrexate for primary central nerve system lymphoma combination therapy. J. Control. Release 359, 132–146. doi:10.1016/j.jconrel.2023.05.040
Liu, J., Cabral, H., and Mi, P. (2024). Nanocarriers address intracellular barriers for efficient drug delivery, overcoming drug resistance, subcellular targeting and controlled release. Adv. Drug Deliv. Rev. 207, 115239. doi:10.1016/j.addr.2024.115239
Liu, J., Lécuyer, T., Seguin, J., Mignet, N., Scherman, D., Viana, B., et al. (2019). Imaging and therapeutic applications of persistent luminescence nanomaterials. Adv. Drug Deliv. Rev. 138, 193–210. doi:10.1016/j.addr.2018.10.015
Liu, R., Luo, C., Pang, Z., Zhang, J., Ruan, S., Wu, M., et al. (2023b). Advances of nanoparticles as drug delivery systems for disease diagnosis and treatment. Chin. Chem. Lett. 34 (2), 107518. doi:10.1016/j.cclet.2022.05.032
Loree, T. R., and Strong, E. W. (1990). Significance of positive margins in oral cavity squamous carcinoma. Am. J. Surg. 160 (4), 410–414. doi:10.1016/S0002-9610(05)80555-0
Lukianova-Hleb, E. Y., Kim, Y.-S., Belatsarkouski, I., Gillenwater, A. M., O'neill, B. E., and Lapotko, D. O. (2016). Intraoperative diagnostics and elimination of residual microtumours with plasmonic nanobubbles. Nat. Nanotechnol. 11 (6), 525–532. doi:10.1038/nnano.2015.343
Ma, Y., Zhang, Y., Li, X., Zhao, Y., Li, M., Jiang, W., et al. (2019). Near-infrared II phototherapy induces deep tissue immunogenic cell death and potentiates cancer immunotherapy. ACS Nano 13 (10), 11967–11980. doi:10.1021/acsnano.9b06040
Machiels, J. P., René Leemans, C., Golusinski, W., Grau, C., Licitra, L., and Gregoire, V. (2020). Squamous cell carcinoma of the oral cavity, larynx, oropharynx and hypopharynx: EHNS–ESMO–ESTRO Clinical Practice Guidelines for diagnosis, treatment and follow-up. Ann. Oncol. 31 (11), 1462–1475. doi:10.1016/j.annonc.2020.07.011
Mahvi, D. A., Liu, R., Grinstaff, M. W., Colson, Y. L., and Raut, C. P. (2018). Local cancer recurrence: the realities, challenges, and opportunities for new therapies. Ca-a Cancer J. Clin. 68 (6), 488–505. doi:10.3322/caac.21498
Maiti, D., Tong, X., Mou, X., and Yang, K. (2019). Carbon-based nanomaterials for biomedical applications: a recent study. Front. Pharmacol. 9, 1401. doi:10.3389/fphar.2018.01401
Malik, S., Muhammad, K., and Waheed, Y. (2023). Emerging applications of nanotechnology in healthcare and medicine. Molecules 28 (18), 6624. doi:10.3390/molecules28186624
Mallia, R. J., Mcveigh, P. Z., Fisher, C. J., Veilleux, I., and Wilson, B. C. (2014). Wide-field multiplexed imaging of EGFR-targeted cancers using topical application of NIR SERS nanoprobes. Nanomedicine 10 (1), 89–101. doi:10.2217/nnm.14.80
Mallick, S., Benson, R., and Rath, G. K. (2015). Radiation induced oral mucositis: a review of current literature on prevention and management. Eur. Archives Oto-Rhino-Laryngology 273 (9), 2285–2293. doi:10.1007/s00405-015-3694-6
Meier, J. D., Oliver, D. A., and Varvares, M. A. (2005). Surgical margin determination in head and neck oncology: current clinical practice. The results of an International American Head and Neck Society Member Survey. Head and Neck 27 (11), 952–958. doi:10.1002/hed.20269
Meier, P., Legrand, A. J., Adam, D., and Silke, J. (2024). Immunogenic cell death in cancer: targeting necroptosis to induce antitumour immunity. Nat. Rev. Cancer 24 (5), 299–315. doi:10.1038/s41568-024-00674-x
Miller, M. C., and Shuman, A. G. (2016). Survivorship in head and neck cancer. JAMA Otolaryngology–Head and Neck Surg. 142 (10), 1002. doi:10.1001/jamaoto.2016.1615
Mito, J. K., Ferrer, J. M., Brigman, B. E., Lee, C. L., Dodd, R. D., Eward, W. C., et al. (2012). Intraoperative detection and removal of microscopic residual sarcoma using wide-field imaging. Cancer 118 (21), 5320–5330. doi:10.1002/cncr.27458
Mody, M. D., Rocco, J. W., Yom, S. S., Haddad, R. I., and Saba, N. F. (2021). Head and neck cancer. Lancet 398 (10318), 2289–2299. doi:10.1016/s0140-6736(21)01550-6
Moon, H., Lee, C., Lee, W., Kim, J., and Chae, H. (2019). Stability of quantum dots, quantum dot films, and quantum dot light-emitting diodes for display applications. Adv. Mater. 31 (34), e1804294. doi:10.1002/adma.201804294
Moradi Kashkooli, F., Soltani, M., and Souri, M. (2020). Controlled anti-cancer drug release through advanced nano-drug delivery systems: static and dynamic targeting strategies. J. Control. Release 327, 316–349. doi:10.1016/j.jconrel.2020.08.012
Muhanna, N., Eu, D., Chan, H. H. L., Daly, M., Fricke, I. B., Douglas, C. M., et al. (2021). Assessment of a liposomal CT/optical contrast agent for image-guided head and neck surgery. Nanomedicine Nanotechnol. Biol. Med. 32, 102327. doi:10.1016/j.nano.2020.102327
Muhanna, N., Jin, C. S., Huynh, E., Chan, H., Qiu, Y., Jiang, W., et al. (2015). Phototheranostic porphyrin nanoparticles enable visualization and targeted treatment of head and neck cancer in clinically relevant models. Theranostics 5 (12), 1428–1443. doi:10.7150/thno.13451
Mukherjee, S., Mukherjee, A., Bytesnikova, Z., Ashrafi, A. M., Richtera, L., and Adam, V. (2024). 2D graphene-based advanced nanoarchitectonics for electrochemical biosensors: applications in cancer biomarker detection. Biosens. Bioelectron. 250, 116050. doi:10.1016/j.bios.2024.116050
Nam, J., Son, S., Ochyl, L. J., Kuai, R., Schwendeman, A., and Moon, J. J. (2018). Chemo-photothermal therapy combination elicits anti-tumor immunity against advanced metastatic cancer. Nat. Commun. 9 (1), 1074. doi:10.1038/s41467-018-03473-9
Navya, P. N., Kaphle, A., Srinivas, S. P., Bhargava, S. K., Rotello, V. M., and Daima, H. K. (2019). Current trends and challenges in cancer management and therapy using designer nanomaterials. Nano Converg. 6 (1), 23. doi:10.1186/s40580-019-0193-2
Neilio, J. M., and Ginat, D. T. (2024). Emerging head and neck tumor targeting contrast agents for the purpose of CT, MRI, and multimodal diagnostic imaging: a molecular review. Diagnostics 14 (15), 1666. doi:10.3390/diagnostics14151666
Nie, T., Fang, Y., Zhang, R., Cai, Y., Wang, X., Jiao, Y., et al. (2025). Self-healable and pH-responsive spermidine/ferrous ion complexed hydrogel Co-loaded with CA inhibitor and glucose oxidase for combined cancer immunotherapy through triple ferroptosis mechanism. Bioact. Mater. 47, 51–63. doi:10.1016/j.bioactmat.2025.01.005
Noga, M., Milan, J., Frydrych, A., and Jurowski, K. (2023). Toxicological aspects, safety assessment, and green toxicology of silver nanoparticles (AgNPs)—critical review: state of the art. Int. J. Mol. Sci. 24 (6), 5133. doi:10.3390/ijms24065133
Orosz, E., Gombos, K., Petrevszky, N., Csonka, D., Haber, I., Kaszas, B., et al. (2020). Visualization of mucosal field in HPV positive and negative oropharyngeal squamous cell carcinomas: combined genomic and radiology based 3D model. Sci. Rep. 10 (1), 40. doi:10.1038/s41598-019-56429-4
Overchuk, M., Weersink, R. A., Wilson, B. C., and Zheng, G. (2023). Photodynamic and photothermal therapies: synergy opportunities for nanomedicine. ACS Nano 17 (9), 7979–8003. doi:10.1021/acsnano.3c00891
Park, J., Bai, B., Ryu, D., Liu, T., Lee, C., Luo, Y., et al. (2023). Artificial intelligence-enabled quantitative phase imaging methods for life sciences. Nat. Methods 20 (11), 1645–1660. doi:10.1038/s41592-023-02041-4
Petit, C., Lacas, B., Pignon, J. P., Le, Q. T., Grégoire, V., Grau, C., et al. (2021). Chemotherapy and radiotherapy in locally advanced head and neck cancer: an individual patient data network meta-analysis. Lancet Oncol. 22 (5), 727–736. doi:10.1016/S1470-2045(21)00076-0
Plou, J., Valera, P. S., García, I., De Albuquerque, C. D. L., Carracedo, A., and Liz-Marzán, L. M. (2022). Prospects of surface-enhanced Raman spectroscopy for biomarker monitoring toward precision medicine. ACS Photonics 9 (2), 333–350. doi:10.1021/acsphotonics.1c01934
Qiao, X. Z., Su, B. S., Liu, C., Song, Q., Luo, D., Mo, G., et al. (2018). Selective surface enhanced Raman scattering for quantitative detection of lung cancer biomarkers in superparticle@MOF structure. Adv. Mater. 30 (5). doi:10.1002/adma.201702275
Qiu, M., Wang, D., Liang, W., Liu, L., Zhang, Y., Chen, X., et al. (2018). Novel concept of the smart NIR-light–controlled drug release of black phosphorus nanostructure for cancer therapy. Proc. Natl. Acad. Sci. 115 (3), 501–506. doi:10.1073/pnas.1714421115
Radtke, J. P., Schwab, C., Wolf, M. B., Freitag, M. T., Alt, C. D., Kesch, C., et al. (2016). Multiparametric magnetic resonance imaging (MRI) and MRI–transrectal ultrasound fusion biopsy for index tumor detection: correlation with radical prostatectomy specimen. Eur. Urol. 70 (5), 846–853. doi:10.1016/j.eururo.2015.12.052
Rahman, M. A., Amin, A. R. M. R., Wang, X., Zuckerman, J. E., Choi, C. H. J., Zhou, B., et al. (2012). Systemic delivery of siRNA nanoparticles targeting RRM2 suppresses head and neck tumor growth. J. Control. Release 159 (3), 384–392. doi:10.1016/j.jconrel.2012.01.045
Razansky, D., Buehler, A., and Ntziachristos, V. (2011). Volumetric real-time multispectral optoacoustic tomography of biomarkers. Nat. Protoc. 6 (8), 1121–1129. doi:10.1038/nprot.2011.351
Ruan, H. T., Bu, L. L., Hu, Q. Y., Cheng, H., Lu, W. Y., and Gu, Z. (2019). Strategies of combination drug delivery for immune checkpoint blockades. Adv. Healthc. Mater. 8 (4), e1801099. doi:10.1002/adhm.201801099
Sabatini, M. E., and Chiocca, S. (2019). Human papillomavirus as a driver of head and neck cancers. Br. J. Cancer 122 (3), 306–314. doi:10.1038/s41416-019-0602-7
Sacco, A. G., and Cohen, E. E. (2015). Current treatment options for recurrent or metastatic head and neck squamous cell carcinoma. J. Clin. Oncol. 33 (29), 3305–3313. doi:10.1200/jco.2015.62.0963
Sano, D., Gule, M. K., Rosenthal, D. I., Bell, D., Yates, J., El-Naggar, A. K., et al. (2012). Early postoperative epidermal growth factor receptor inhibition: safety and effectiveness in inhibiting microscopic residual of oral squamous cell carcinoma in vivo. Head and Neck 35 (3), 321–328. doi:10.1002/hed.22961
Schnell, A., Bod, L., Madi, A., and Kuchroo, V. K. (2020). Author Correction: the yin and yang of co-inhibitory receptors: toward anti-tumor immunity without autoimmunity. Cell. Res. 30 (4), 366. doi:10.1038/s41422-020-0285-x
Sengul, A. B., and Asmatulu, E. (2020). Toxicity of metal and metal oxide nanoparticles: a review. Environ. Chem. Lett. 18 (5), 1659–1683. doi:10.1007/s10311-020-01033-6
Shang, L., Nienhaus, K., and Nienhaus, G. U. (2014). Engineered nanoparticles interacting with cells: size matters. J. Nanobiotechnology 12, 5. doi:10.1186/1477-3155-12-5
Shetty, B., Chauhan, R. S., Tirupathi, S., Krishnapriya, N., Patil, L., and Rathi, N. (2024). Quantum dots in noninvasive imaging of oral squamous cell carcinomas: a scoping literature review. J. Cancer Res. Ther. 20 (3), 745–749. doi:10.4103/jcrt.jcrt_2203_21
Shi, Y., Van Der Meel, R., Chen, X., and Lammers, T. (2020). The EPR effect and beyond: strategies to improve tumor targeting and cancer nanomedicine treatment efficacy. Theranostics 10 (17), 7921–7924. doi:10.7150/thno.49577
Smith, B. R., and Gambhir, S. S. (2017). Nanomaterials for in vivo imaging. Chem. Rev. 117 (3), 901–986. doi:10.1021/acs.chemrev.6b00073
Song, C., Tang, C., Xu, W., Ran, J., Wei, Z., Wang, Y., et al. (2020). Hypoxia-targeting multifunctional nanoparticles for sensitized chemotherapy and phototherapy in head and neck squamous cell carcinoma. Int. J. Nanomedicine 15, 347–361. doi:10.2147/ijn.S233294
Souza, C., Pellosi, D. S., and Tedesco, A. C. (2019). Prodrugs for targeted cancer therapy. Expert Rev. Anticancer Ther. 19 (6), 483–502. doi:10.1080/14737140.2019.1615890
Stransky, N., Egloff, A. M., Tward, A. D., Kostic, A. D., Cibulskis, K., Sivachenko, A., et al. (2011). The mutational landscape of head and neck squamous cell carcinoma. Science 333 (6046), 1157–1160. doi:10.1126/science.1208130
Sun, P., Zhang, Y., Li, K., Wang, C., Zeng, F., Zhu, J., et al. (2019). Image-guided surgery of head and neck carcinoma in rabbit models by intra-operatively defining tumour-infiltrated margins and metastatic lymph nodes. EBioMedicine 50, 93–102. doi:10.1016/j.ebiom.2019.10.055
Sykes, E. A., Chen, J., Zheng, G., and Chan, W. C. W. (2014). Investigating the impact of nanoparticle size on active and passive tumor targeting efficiency. ACS Nano 8 (6), 5696–5706. doi:10.1021/nn500299p
Tang, B., Huang, R., and Ma, W. (2024). Advances in nanotechnology-based approaches for the treatment of head and neck squamous cell carcinoma. RSC Adv. 14 (52), 38668–38688. doi:10.1039/d4ra07193j
Trotta, B. M., Pease, C. S., Rasamny, J. J., Raghavan, P., and Mukherjee, S. (2011). Oral cavity and oropharyngeal squamous cell cancer: key imaging findings for staging and treatment planning. Radiographics 31 (2), 339–354. doi:10.1148/rg.312105107
Vahrmeijer, A. L., Hutteman, M., Van Der Vorst, J. R., Van De Velde, C. J. H., and Frangioni, J. V. (2013). Image-guided cancer surgery using near-infrared fluorescence. Nat. Rev. Clin. Oncol. 10 (9), 507–518. doi:10.1038/nrclinonc.2013.123
Wang, B., Hu, S., Teng, Y., Chen, J., Wang, H., Xu, Y., et al. (2024a). Current advance of nanotechnology in diagnosis and treatment for malignant tumors. Signal Transduct. Target. Ther. 9 (1), 200. doi:10.1038/s41392-024-01889-y
Wang, J., Fan, X., Han, X., Lv, K., Zhao, Y., Zhao, Z., et al. (2024b). Ultrasmall inorganic mesoporous nanoparticles: preparation, functionalization, and application. Adv. Mater. 36 (28), e2312374. doi:10.1002/adma.202312374
Wang, J.-H., Wang, B., Liu, Q., Li, Q., Huang, H., Song, L., et al. (2013). Bimodal optical diagnostics of oral cancer based on Rose Bengal conjugated gold nanorod platform. Biomaterials 34 (17), 4274–4283. doi:10.1016/j.biomaterials.2013.02.012
Wang, Y., Gao, W., Shi, X., Ding, J., Liu, W., He, H., et al. (2017). Chemotherapy drugs induce pyroptosis through caspase-3 cleavage of a gasdermin. Nature 547 (7661), 99–103. doi:10.1038/nature22393
Wang, Y., Han, J., Zhu, Y., Huang, N., and Qu, N. (2025). New advances in the therapeutic strategy of head and neck squamous cell carcinoma: a review of latest therapies and cutting-edge research. Biochimica Biophysica Acta (BBA) - Rev. Cancer 1880 (1), 189230. doi:10.1016/j.bbcan.2024.189230
Wang, Y., Zhang, W., Sun, P., Cai, Y., Xu, W., Fan, Q., et al. (2019). A novel multimodal NIR-II nanoprobe for the detection of metastatic lymph nodes and targeting chemo-photothermal therapy in oral squamous cell carcinoma. Theranostics 9 (2), 391–404. doi:10.7150/thno.30268
Wiechec, E., Hansson, K., Alexandersson, L., Jönsson, J.-I., and Roberg, K. (2017). Hypoxia mediates differential response to anti-EGFR therapy in HNSCC cells. Int. J. Mol. Sci. 18 (5), 943. doi:10.3390/ijms18050943
Wong, X. Y., Sena-Torralba, A., Álvarez-Diduk, R., Muthoosamy, K., and Merkoçi, A. (2020). Nanomaterials for nanotheranostics: tuning their properties according to disease needs. ACS Nano 14 (3), 2585–2627. doi:10.1021/acsnano.9b08133
Wu, J., Liu, J., Lin, B., Lv, R., Yuan, Y., and Tao, X. (2021). Met-targeted dual-modal MRI/NIR II imaging for specific recognition of head and neck squamous cell carcinoma. ACS Biomaterials Sci. and Eng. 7 (4), 1640–1650. doi:10.1021/acsbiomaterials.0c01807
Wu, Q., Chen, L., Huang, X., Lin, J., Gao, J., Yang, G., et al. (2023a). A biomimetic nanoplatform for customized photothermal therapy of HNSCC evaluated on patient-derived xenograft models. Int. J. Oral Sci. 15 (1), 9. doi:10.1038/s41368-022-00211-2
Wu, Y., Chang, X., Yang, G., Chen, L., Wu, Q., Gao, J., et al. (2023b). A physiologically responsive nanocomposite hydrogel for treatment of head and neck squamous cell carcinoma via proteolysis-targeting chimeras enhanced immunotherapy. Adv. Mater. 35 (12), e2210787. doi:10.1002/adma.202210787
Xiao, Y., Zhang, T., Ma, X., Yang, Q. C., Yang, L. L., Yang, S. C., et al. (2021). Microenvironment-responsive prodrug-induced pyroptosis boosts cancer immunotherapy. Adv. Sci. 8 (24), e2101840. doi:10.1002/advs.202101840
Xu, J., Müller, S., Nannapaneni, S., Pan, L., Wang, Y., Peng, X., et al. (2012). Comparison of quantum dot technology with conventional immunohistochemistry in examining aldehyde dehydrogenase 1A1 as a potential biomarker for lymph node metastasis of head and neck cancer. Eur. J. Cancer 48 (11), 1682–1691. doi:10.1016/j.ejca.2011.12.029
Xu, Q., Chen, Y., Jin, Y., Wang, Z., Dong, H., Kaufmann, A. M., et al. (2022). Advanced nanomedicine for high-risk HPV-driven head and neck cancer. Viruses 14 (12), 2824. doi:10.3390/v14122824
Xu, X., Ho, W., Zhang, X., Bertrand, N., and Farokhzad, O. (2015). Cancer nanomedicine: from targeted delivery to combination therapy. Trends Mol. Med. 21 (4), 223–232. doi:10.1016/j.molmed.2015.01.001
Xue, L., Yan, B., Li, Y., Tan, Y., Luo, X., and Wang, M. (2018). Surface-enhanced Raman spectroscopy of blood serum based on gold nanoparticles for tumor stages detection and histologic grades classification of oral squamous cell carcinoma. Int. J. Nanomedicine 13, 4977–4986. doi:10.2147/ijn.S167996
Yakavets, I., Francois, A., Guiot, M., Lequeux, N., Fragola, A., Pons, T., et al. (2020). NIR imaging of the integrin-rich head and neck squamous cell carcinoma using ternary copper indium selenide/zinc sulfide-based quantum dots. Cancers 12 (12), 3727. doi:10.3390/cancers12123727
Yang, K., Zhang, F. J., Tang, H., Zhao, C., Cao, Y. A., Lv, X. Q., et al. (2011). In-vivo imaging of oral squamous cell carcinoma by EGFR monoclonal antibody conjugated near-infrared quantum dots in mice. Int. J. Nanomedicine 6, 1739–1745. doi:10.2147/ijn.S23348
Yin, C., Wei, Z.-J., Long, K., Sun, M., Zhang, Z., Wang, Y., et al. (2024). pH-responsive persistent luminescent nanosystem with sensitized NIR imaging and ratiometric imaging modes for tumor surgery navigation. ACS Appl. Mater. and Interfaces 16 (50), 69071–69085. doi:10.1021/acsami.4c17747
Yip, S. S. F., and Aerts, H. J. W. L. (2016). Applications and limitations of radionics. Phys. Med. Biol. 61 (13), R150–R166. doi:10.1088/0031-9155/61/13/r150
Zang, C., Tian, Y., Tang, Y., Tang, M., Yang, D., Chen, F., et al. (2024). Hydrogel-based platforms for site-specific doxorubicin release in cancer therapy. J. Transl. Med. 22 (1), 879. doi:10.1186/s12967-024-05490-3
Zhang, Y., Chen, R., Liu, F., Miao, P., Lin, L., and Ye, J. (2022). In vivo surface-enhanced transmission Raman spectroscopy under maximum permissible exposure: toward photosafe detection of deep-seated tumors. Small Methods 7 (2), e2201334. doi:10.1002/smtd.202201334
Zhang, Y., Dong, P., and Yang, L. (2023). The role of nanotherapy in head and neck squamous cell carcinoma by targeting tumor microenvironment. Front. Immunol. 14, 1189323. doi:10.3389/fimmu.2023.1189323
Zhang, Y., Wang, Y., Zhu, Y., and Wan, H. (2024). Taste dysfunction symptoms experience in head and neck cancer patients undergoing proton and heavy ion therapy: a qualitative study. Support. Care Cancer 33 (1), 17. doi:10.1007/s00520-024-09040-x
Zhao, J., and Castranova, V. (2011). Toxicology of nanomaterials used in nanomedicine. J. Toxicol. Environ. Health, Part B 14 (8), 593–632. doi:10.1080/10937404.2011.615113
Zhao, M., Hu, X., Xu, Y., Wu, C., Chen, J., Ren, Y., et al. (2019). Targeting of EZH2 inhibits epithelial-mesenchymal transition in head and neck squamous cell carcinoma via regulating the STAT3/VEGFR2 axis. Int. J. Oncol. 55, 1165–1175. doi:10.3892/ijo.2019.4880
Zhong, D., Xiong, S., Zhang, Y., Cui, M., Liu, L., Xu, Y., et al. (2024). H2O2-activated NIR fluorescent probe with tumor targeting for cell imaging and fluorescent-guided surgery. Sensors Actuators B Chem. 418, 136249. doi:10.1016/j.snb.2024.136249
Zhou, J.-J., Li, X.-H., He, P.-Y., Qi, F.-Y., Ullah, M. W., Li, S.-J., et al. (2022). Implantable versatile oxidized bacterial cellulose membrane for postoperative HNSCC treatment via photothermal-boosted immunotherapy. Nano Res. 16 (1), 951–963. doi:10.1007/s12274-022-4811-7
Zhou, X. X., Yao, Z. R., Bai, H., Duan, J. C., Wang, Z. J., Wang, X., et al. (2021). Treatment-related adverse events of PD-1 and PD-L1 inhibitor-based combination therapies in clinical trials: a systematic review and meta-analysis. Lancet Oncol. 22 (9), 1265–1274. doi:10.1016/S1470-2045(21)00333-8
Zhu, F.-Y., Mei, L.-J., Tian, R., Li, C., Wang, Y.-L., Xiang, S.-L., et al. (2024). Recent advances in super-resolution optical imaging based on aggregation-induced emission. Chem. Soc. Rev. 53 (7), 3350–3383. doi:10.1039/d3cs00698k
Zhu, J., Chu, C., Li, D., Zhang, Y., Cheng, Y., Lin, H., et al. (2022). Superior fluorescent nanoemulsion illuminates hepatocellular carcinoma for surgical navigation. Front. Bioeng. Biotechnol. 10, 890668. doi:10.3389/fbioe.2022.890668
Zhu, X., and Li, S. (2023). Nanomaterials in tumor immunotherapy: new strategies and challenges. Mol. Cancer 22 (1), 94. doi:10.1186/s12943-023-01797-9
Keywords: head and neck cancer, perioperative period, nanotechnology, diagnosis, treatment
Citation: Zhou J-J, Feng Y-C, Zhao M-L, Guo Q and Zhao X-B (2025) Nanotechnology in the perioperative treatment of head and neck cancer: application and outlook. Front. Bioeng. Biotechnol. 13:1566522. doi: 10.3389/fbioe.2025.1566522
Received: 06 February 2025; Accepted: 13 March 2025;
Published: 27 March 2025.
Edited by:
Marina Massaro, University of Palermo, ItalyReviewed by:
Umberto M. Musazzi, University of Milan, ItalyCopyright © 2025 Zhou, Feng, Zhao, Guo and Zhao. This is an open-access article distributed under the terms of the Creative Commons Attribution License (CC BY). The use, distribution or reproduction in other forums is permitted, provided the original author(s) and the copyright owner(s) are credited and that the original publication in this journal is cited, in accordance with accepted academic practice. No use, distribution or reproduction is permitted which does not comply with these terms.
*Correspondence: Jun-Jie Zhou, emhvdWp1bmppZUB3aHUuZWR1LmNu
Disclaimer: All claims expressed in this article are solely those of the authors and do not necessarily represent those of their affiliated organizations, or those of the publisher, the editors and the reviewers. Any product that may be evaluated in this article or claim that may be made by its manufacturer is not guaranteed or endorsed by the publisher.
Research integrity at Frontiers
Learn more about the work of our research integrity team to safeguard the quality of each article we publish.