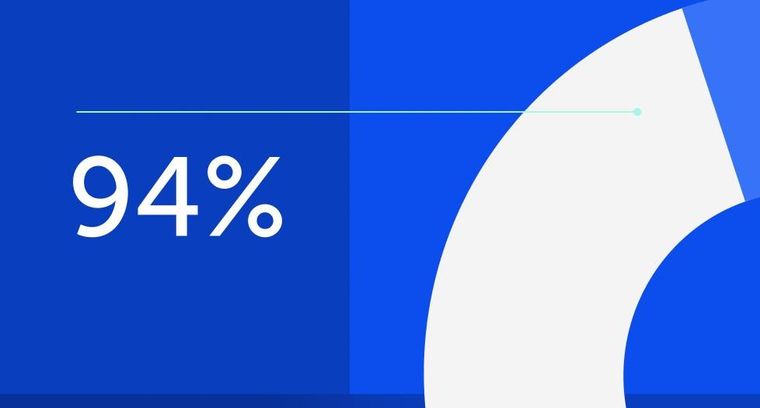
94% of researchers rate our articles as excellent or good
Learn more about the work of our research integrity team to safeguard the quality of each article we publish.
Find out more
REVIEW article
Front. Bioeng. Biotechnol., 21 March 2025
Sec. Nanobiotechnology
Volume 13 - 2025 | https://doi.org/10.3389/fbioe.2025.1565999
This article is part of the Research TopicThe Road toward Nano-Based Diagnostics for Health and DiseaseView all 9 articles
The early diagnosis rate of gastric cancer is low, and most patients are already at an advanced stage by the time they are diagnosed, posing significant challenges for treatment and exhibiting high recurrence rates, which notably diminish patients’ survival time and quality of life. Therefore, there is an urgent need to identify methods that can enhance treatment efficacy. Nanomedicine, distinguished by its small size, high targeting specificity, and strong biological compatibility, is particularly well-suited to address the toxic side effects associated with current diagnostic and therapeutic approaches for gastric cancer. Consequently, the application of nanomedicine and delivery systems in the diagnosis and treatment of gastric cancer has garnered increasing interest from researchers. This review provides an overview of recent advancements in the use of nanomaterials as drugs or drug delivery systems in gastric cancer research, encompassing their applications in diagnosis, chemotherapy, radiotherapy, surgery, and phototherapy, and explores the promising prospects of nanomedicine in the treatment of gastric cancer.
In 2022, there were over 960 thousand new cases of gastric cancer globally, resulting in approximately 660 thousand deaths, both figures ranking fifth worldwide (Siegel et al., 2022). Projections indicate that by 2050, annual cancer incidences will reach 35 million, marking a 77% increase from 2022 levels (Bray et al., 2024). Gastric cancer, characterized by high incidence and mortality rates, places a substantial burden on healthcare systems and the World Health Organization due to its extensive consumption of medical resources. Gastric cancer has a low accuracy in early diagnosis, suboptimal treatment outcomes, high recurrence rates, and a 5-year survival rate that remains below 20% (Liu Q. et al., 2023). Most gastric cancer patients are diagnosed at advanced stages because early symptoms are often absent or nonspecific, leading to delayed detection (Liu N. et al., 2022; Thrift and El-Serag, 2020; Tian et al., 2020). Current primary treatment modalities include chemotherapy, radiotherapy, surgery, immunotherapy, and phototherapy (Li et al., 2023). While these treatments can effectively eliminate gastric cancer cells to varying degrees, they frequently result in drug resistance and multiple adverse effects such as vomiting and hair loss (Zhu T. et al., 2024). Nano-drug delivery systems, as a novel drug delivery approach, offer the advantage of precisely targeting tumor cells while causing relatively small damage to healthy tissues and cells (Moradian and Mohammadzadeh, 2023).
Nanomaterials, typically ranging from 1 to 100 nm in size (Jeevanandam et al., 2018), exhibit excellent inclusivity, targeting capabilities, and structural stability (Li et al., 2021; Yang et al., 2022; Yao et al., 2020).In recent years, novel nanotechnology has led to remarkable advancements in nanomedicines, which have made extraordinary achievements in cancer diagnosis and treatment (Bor et al., 2019). Research indicates that nanomaterials hold substantial promise in areas such as early cancer diagnosis, targeted chemotherapy drug delivery, radiation sensitization, surgical tracing, and phototherapy (He et al., 2015). Furthermore, nanomedicine demonstrates strong compatibility with vaccines and 3D printing technologies, opening up extensive prospects for improving the diagnosis and treatment of gastric cancer (Alsharabasy and Pandit, 2024; Ke et al., 2022; Liu Y. et al., 2023).In the diagnosis domain, nanosensors play a pivotal role in detecting cancer at earlier stages, as exemplified by the use of nano-probes (Li et al., 2018). The principle of “early detection and early treatment” has a vital meaning for extending patient survival and enhancing quality of life. (Figure 1).
Figure 1. Application of nanomedicine and delivery systems in the diagnosis and treatment of gastric cancer.
Imaging techniques and endoscopic examinations represent a currently prevalent approach for diagnosing gastric cancer. However, these approaches have certain limitations, including blind spots and difficulties in accurate characterization (Li et al., 2021; Yang et al., 2022). Nanotechnology-based diagnostic methods can be divided into direct and indirect approaches. Direct diagnosis involves the use of nanomaterials conjugated with fluorescent substances to form tracers, enabling improved visualization of tumor locations. This provides more precise guidance for subsequent surgical procedures, radiotherapy, and chemotherapy. Indirect diagnosis refers to the integration of nanomaterials into existing diagnostic technologies, such as computed tomography (CT), surface-enhanced Raman scattering (SERS), and endoscopy, to complement existing testing techniques in confirming the diagnosis of gastric cancer.
The application of nanocarriers in the diagnosis of gastric cancer involves the use of nanoscale materials or structures as delivery vehicles to precisely transport diagnostic agents or markers to gastric tissues. This approach facilitates early detection, localization, and assessment of gastric cancer (Kwak et al., 2024). By integrating the benefits of nanotechnology and molecular biology, this technology aims to improve diagnostic sensitivity and specificity, reduce the need for invasive procedures, and enhance patient comfort (Mollasalehi and Shajari, 2021). Key technical requirements include: Size and Permeability: Nanomaterials must be capable of penetrating biological barriers while avoiding clearance by macrophages; Targeting and Loading Capacity: Nanomaterials should be able to conjugate with specific ligands to deliver sufficient diagnostic agents to the target sites; Regulatory Compliance: The design and manufacturing of nanocarriers must comply with relevant pharmaceutical regulations and safety standards, undergoing rigorous safety and efficacy testing (Ruan et al., 2012; Wang et al., 2011). Zhang et al. (2025) devised perovskite-based probes, designated as AZI-PQDs, tailored specifically for the identification of gastric cancer tumors. These probes demonstrated accurate detection of gastric cancer cells in both in vitro and in vivo fluorescence imaging experiments while ensuring minimal immunogenicity and toxicity. This approach showcases substantial promise for the diagnosis of gastric cancer. Furthermore, Ma et al. (2024) introduced an electrochemical biosensor, termed MoS2-Au@Pt, which exhibits remarkable sensitivity and selectivity. Notably, this biosensor can detect miR-19b-3p at exceedingly low concentrations—down to 0.7 a.m.—thus effectively differentiating between normal individuals and those afflicted with gastric cancer. Wu et al. (2021) established a sensitive single-nucleotide polymorphism (SNP) detection system that demonstrated high selectivity and sensitivity for detecting mutations in the p53 gene. This system has the ability to identify early gastric cancer. Zhang C. et al. (2022) developed an electrochemical cell sensor named GO-AuNSs@rBSA-FA for analyzing MGC-803 cells. This sensor quantitatively detects cells in the range of 3 × 102 to 7 × 106 cells/mL, offering the potential for early gastric cancer detection in clinical settings. Shi et al. (2019) introduced a nanoprobe named T-MAN, which targets tumor cells and activates matrix metalloproteinase 2 (MMP-2), enabling the precise in situ detection of gastric tumors in a mouse model during surgery for the first time (Wang et al., 2014).
Nanomaterials can be integrated with existing diagnostic techniques to detect gastric cancer cells. It can be combined with existing CT, SERS, or endoscopy for the diagnosis of gastric cancer. By precisely delivering diagnostic agents via nanocarriers and integrating multiple imaging techniques, this approach enables early diagnosis and comprehensive evaluation of gastric cancer (Cai et al., 2023). Specific criteria cover aspects such as the design of nanocarriers, the performance of imaging techniques, and the ease of operation, ensuring the safety and effectiveness of this method in clinical applications. This not only enhances the accuracy of gastric cancer diagnosis but also provides patients with more treatment options and opportunities.
CT imaging can accurately depict the size and location of gastric cancer cells and is commonly used for preoperative planning and surgical risk assessment. CT offers several advantages, including non-invasiveness (Ma et al., 2022), high image resolution, and the ability to perform three-dimensional reconstruction. However, it has certain limitations, such as reduced sensitivity in detecting small lesions in the early stages (Wei et al., 2024), and radiation exposure from CT scans can pose risks to patients. When combined with CT, nanocarriers should efficiently load CT contrast agents to ensure sufficient contrast during CT scans (Yin et al., 2021). Additionally, CT should have high resolution to visualize the anatomical structures of the stomach and potential lesion areas, facilitating accurate analysis and diagnosis by medical professionals. Shi et al. (2018) investigated a fluorescent copper sulfide nanoparticle (RGD-CuS-Cy5.5), which, when combined with CT, enabled in vivo imaging and detection of sentinel lymph node (SLN) metastasis in gastric cancer. Xuan et al. (2019) developed a bismuth nanoparticle cluster probe with enhanced permeability and retention properties. These particles, measuring approximately 25–55 nm, can easily penetrate tumor cells and assist CT in the accurate diagnosis of gastric cancer. Huang et al. (2017) created a nanoparticle composite, Au-BSA-DOX-FA, as a CT contrast agent. This material improves CT imaging clarity and enhances the efficacy of targeted gastric cancer treatment, making it a promising new nanocarrier for drug delivery in gastric cancer diagnosis. Shi et al. (2018) devised a nanoparticle named RGD-CuS-Cy5.5, which targets gastric cancer cells in lymph nodes for non-invasive multimodal imaging, allowing for precise identification of SLN metastasis. Kukreja et al. (2017) developed a porous-shell nanoparticle, AuFe NPs, which exhibits T2 contrast effects and reduces X-ray radiation, making it suitable for CT imaging (Zhang et al., 2013).
SERS has emerged as a promising technique for diagnosing gastric cancer by detecting specific biomarkers in bodily fluids (Zhai et al., 2022). Nanocarriers typically utilize gold or silver nanoparticles as SERS substrates (Xie et al., 2024), which can specifically bind to gastric cancer cells or related molecules, thereby enhancing Raman signals and improving the sensitivity and specificity of the detection (Wei et al., 2016; Xie et al., 2024). However, it remains in the research phase and has not yet been extensively adopted in clinical practice. Consequently, advancing research on SERS-based diagnostic methods for gastric cancer is imperative. For instance, Chen et al. (2016) successfully differentiated between early- and late-stage gastric cancer patients and healthy individuals by analyzing volatile organic compounds in exhaled breath, achieving an accuracy rate exceeding 83%. This approach demonstrates potential as an effective preliminary screening tool. Similarly, Pan et al. (2022) developed a sensor utilizing a nanocomposite material (MoS2-AuNSs) to detect exosomes, which carry tumor-specific molecular information, thereby facilitating early cancer diagnosis. Huang et al. (2022) designed an Ag@ZIF-67 sensor that energizes plasmonic nanoparticles, enabling non-invasive, rapid, and user-friendly gastric cancer screening with an accuracy rate of up to 89.83%. Shao et al. (2023) introduced Au/SiNUA, which enhances SERS performance with detection times as short as 18 min, while also exhibiting ultra-sensitivity and specificity, making it advantageous for precise gastric cancer screening. Additionally, Cao et al. (2023) developed a microarray chip based on a gold nanohexagon substrate to construct a SERS spectral recognition model, achieving an accuracy rate exceeding 97.5% in gastric cancer diagnosis, thus highlighting its potential as a clinical diagnostic technology.
Endoscopy is a primary diagnostic method for gastric cancer, offering speed and convenience. However, it has notable limitations, including the inability to detect early-stage lesions below its resolution threshold (Han et al., 2020) and risks such as perforation and bleeding. When integrated with endoscopy, nanocarriers should exhibit excellent integration and real-time capabilities. This means that the endoscope must be compatible with nanocarriers and imaging technologies, enabling multimodal imaging while providing real-time imaging data to assist doctors in making rapid diagnoses. To address these challenges, Luo et al. (2023) developed a nanoprobe named M-1, which enables imaging under blue laser endoscopy, simplifying procedures and reducing the risk of errors. In animal experiments, the M-1 probe demonstrated exceptional capability in identifying gastric cancer cells, marking a significant advancement in the visualization of blood vessels for early gastric cancer diagnosis. Harmsen et al. (2019) integrated SERS nanoparticles into endoscopy, successfully detecting gastrointestinal tumor cells in animal models. Furthermore, Du et al. (2018) designed a nanoprobe based on dye-labeled human heavy-chain ferritin, which enhances the accuracy of early gastric cancer cell detection and aids in the endoscopic resection of tumor cells.
In clinical practice, the primary treatment modalities for gastric cancer include chemotherapy, radiotherapy, surgery, and phototherapy (Li et al., 2024; Wang G. et al., 2024). However, these approaches are often associated with drug resistance and adverse effects such as hair loss and vomiting. (Rai et al., 2021). By utilizing nanoparticles, liposomes, polymers, nano-micelles, and hydrogels as carriers (Cabral et al., 2018; Che et al., 2020; Salapa et al., 2020), nanomedicines can be delivered precisely to tumor cells, minimizing drug loss and increasing drug concentration within the Tumor Microenvironment (TME) (Kaur et al., 2022; Zhu et al., 2022). When combined with existing therapies, nanomedicines have the potential to enhance clinical efficacy in the treatment of gastric cancer.
Chemotherapy remains one of the primary treatment modalities for gastric cancer. However, conventional chemotherapeutic agents often exhibit suboptimal tumor cell targeting and inflict significant collateral damage to normal cells (Zheng et al., 2020a). The side effects of chemotherapy are not only limited to hair loss and gastrointestinal disturbances but also include cardiovascular and liver-kidney dysfunction. Notably, anthracyclines, a class of chemotherapeutic drugs, are associated with severe cardiotoxicity (Geerts et al., 2024), which can manifest as myocardial ischemia in mild cases and heart failure in severe cases, thereby severely compromising the patient’s quality of life. To address these challenges, researchers have focused on developing various nanomaterials to serve either as chemotherapeutic agents or as drug delivery systems. These nanomaterials enhance the precision of drug delivery through mechanisms such as active targeting and passive targeting while being engineered to account for critical factors like pH, temperature, light, and magnetism (Yu et al., 2022). These factors significantly influence drug efficacy and delivery, paving the way for safer and more precise chemotherapy in the treatment of gastric cancer.
Active targeting represents one of the primary strategies for delivering nanomedicine to tumor cells (Garcia-Pinel et al., 2019). This mechanism involves tethering tumor-specific molecular recognition ligands to the surface of nanomedicine, thereby enabling targeted delivery to tumor cell surfaces. The number, spatial distribution, and other parameters of the targeting ligands on the nanomaterials directly influence the targeting efficiency of the nanomedicine. Typically, tumor cells overexpress a repertoire of surface receptors that can bind to these ligands, leading to endocytosis. Kong et al. (2024) developed a multifunctional pH-responsive nano platform named CPP10-PEG@Cur@FT, which delivers curcumin (Cur) to gastric cancer cells through a synergistic combination of chemotherapy and PDT. This platform significantly enhanced the cytotoxicity of Cur against gastric cancer cells. Zhang et al. (2020) demonstrated that Salidroside potentiates the chemosensitivity of apatinib in gastric cancer, and their engineered nano drug delivery system, iVR1-NPs-Apa/Sal, precisely targets tumor cells. Alam et al. (2022) synthesized curcumin-loaded poly (lactic-co-glycolic acid) nanoparticles (Cur-NPs) using a single-emulsion solvent evaporation method. Experimental results revealed that, compared to cells treated with free Cur, a higher number of apoptotic cells were observed after 72 h of Cur-NPs treatment, highlighting the therapeutic potential of Cur-NPs in gastric cancer.
Passive targeting of nanomedicine to tumor cells is based on the enhanced permeability and retention effect (Obata and Hirohara, 2023). Research has shown that paclitaxel (PTX) synergizes with the AKT inhibitor capivasertib to exert potent antitumor effects, demonstrating significant potential in advanced gastric cancer treatment (Song et al., 2024). Jian et al. (2020) engineered GX1-modified nanoliposomes named GX1-PTX-NLCs, which not only significantly reduced PTX toxicity but also enhanced its antitumor efficacy, making it a promising nano-drug delivery system. Diniz et al. (Diniz et al., 2023) created “Foretinib”-loaded nanoparticles containing tyrosine kinase inhibitors, which exhibited the ability to slow tumor progression and inhibit cell proliferation in vivo studies, underscoring their potential as a therapeutic strategy for gastric cancer. Zhang A. et al. (2022) developed dual-loaded DNA micelles (Cur@affi-F/GQs) by incorporating Cur and 5-fluorodeoxyuridine into affi-F/GQs micelles. In vitro studies revealed that Cur@affi-F/GQs significantly enhanced the activity of apoptosis-related proteins in the Bcl-2/Bax-caspase eight and 9-caspase three pathways, exerting robust cytotoxic effects on N87 cells. Abedi et al. (2023) synthesized poly (ethylene glycol) -coated nanoparticles that retained 39% of the drug payload after 72 h, demonstrating superior drug retention and advancing the application of nanomedicine in chemotherapy. Zheng et al. (2020b) developed PLGA-NPs co-delivering doxorubicin (DOX) and nitrofurazone, which inhibited gastric cancer cell proliferation by downregulating STAT3 pathway phosphorylation. Chen et al. (2023) engineered a nano-micelle based on carboxymethyl alginate, which, in both in vitro and in vivo studies, activated cytotoxic T lymphocyte (CTL) activity and polarized M2 phenotype to M1 phenotype, phenotype thereby exerting synergistic antitumor effects and extending the overall survival of gastric cancer animal models. Cur, a traditional Chinese medicine monomer, not only acts as an effective radiosensitizer but also holds promise as a therapeutic agent for gastric cancer. Yaghoubi et al. (2021) targeted human gastric adenocarcinoma cell lines using a combination of Cur and DOX loaded onto carboxylated graphene oxide (APT-CGO), which demonstrated significantly higher inhibitory effects compared to CGO-based drugs (Figure 2).
Radiotherapy is a crucial method for treating malignant tumors, effectively eradicating tumor cells. However, it can also damage normal cells, leading to side effects such as hair loss, gastrointestinal reactions, and bone marrow suppression. Given these side effects, the development of more benign and precisely localized nanomedicines for radiotherapy is crucial. Current nanotherapeutic agents, characterized by reduced toxicity, can be primarily categorized into two major types: organic and inorganic nanoparticles (Wei et al., 2022).
Organic nanoparticles are typically used as radio-sensitizers to enhance the sensitivity of tumor cells to radiation. Numerous studies have confirmed that Cur is an effective organic nanoparticle, significantly improving radiation efficiency. Cur exhibits several beneficial properties, including inhibiting tumor cell proliferation, inducing apoptosis in gastric cancer cells, regulating the cell cycle to arrest cells in the radiation-sensitive G2/M phase, and displaying antioxidant effects by scavenging oxidative free radicals generated during radiotherapy. These effects reduce damage to normal cells (Liu Z. et al., 2022). Nosrati et al. (2022) developed a metal-semiconductor heterojunction nanoparticle (Bi2S3@BSA-Au-BSA-MTX-Cur) loaded with Cur and methotrexate (MTX). This nanoparticle, when administered in a single dose of combined chemoradiotherapy with X-rays, completely eradicated tumors in animal models within approximately 20 days, demonstrating an extremely effective anti-tumor approach. Delahunty et al. (2022) demonstrated that 7-dehydrocholesterol can act as a sensitizer to induce reactive oxygen species (ROS) responses. Encapsulating 7-dehydrocholesterol into nanoparticles (7-DHC@PLGANP) significantly enhances the efficacy of radiotherapy.
A hypoxic microenvironment (HME) can reduce the sensitivity of tumor cells to radiotherapy. Inorganic nanomaterials are generally used directly in radiotherapy, converting light energy into heat to enhance local radiation or improving the HME of tumor tissues by generating ROS to enhance radiotherapy effects. Unlike traditional radiotherapy, inorganic nanomaterials exhibit excellent biocompatibility and safety, can stably exist within the human body without easy degradation, and have significantly lower toxicity compared to existing radiotherapy methods. Tang et al. (2021) developed an oxygen-generating nanomotor (pHPFON-NO/O2) based on hybrid semiconductor organic silicon, which can alleviate HME to enhance the efficacy of radiotherapy. Broekgaarden et al. (2020) demonstrated that the surface chemical design of ultra-small gold nanoclusters (AuNC) enabled high-sensitivity near-infrared and short-wave infrared imaging. They found that combining high-content imaging assays significantly enhances the effectiveness of radiotherapy. Chen et al. (2021) combined Au-OMV with radiotherapy, which not only increased radio-sensitivity but also modulated immune function. In animal experiments, mice treated with Au-OMV in combination with radiotherapy had longer survival times. Wang Z. et al. (2024) designed a tungsten-based nano radiosensitizer (PWAI) to address immune suppression caused by severe MYC upregulation due to radiotherapy. PWAI significantly enhanced the anti-tumor immune response to radiotherapy, reducing the cytotoxicity of T lymphocytes in animal models. Wang H. et al. (2023) developed a nanosystem called MON@pG, which enhanced the radio-sensitivity of gastric cancer cells through ROS-mediated effects, induced mitochondrial dysfunction, and ferroptosis, thereby improving the effectiveness of radiotherapy for gastric cancer.
Surgery remains a crucial treatment for gastric cancer. However, it also has drawbacks such as significant bodily trauma and a long postoperative recovery period (Skapars et al., 2023). Nanomedicine and delivery systems can precisely target tumor cells, reduce incisions, and shorten recovery times. Peritoneal metastatic nodules in gastric cancer, characterized by focal diffusion, small size, and close contact with adjacent organs, are often challenging to identify and completely remove during surgery, frequently leading to surgical failure and cancer recurrence (Guo et al., 2023). Indocyanine green (ICG)-mediated fluorescence imaging is widely used in gastrointestinal surgery. ICG’s excellent permeability and retention properties enable precise and personalized radical surgery (Sun et al., 2024). Wang et al. (2018) synthesized a novel gold nanoshell conjugated with ICG (I-GSN) to overcome the weak targeting ability and poor tissue penetration of chemotherapeutic drugs, as well as incomplete tumor clearance. I-GSN-mediated near-infrared imaging provides sufficient optical contrast for preoperative guidance and intraoperative tumor resection. Wang H. et al. (2023) developed a near-infrared fluorophore methylene blue probe to assist surgeons in clearly visualizing resection margins during gastric cancer surgery. Liposomes, primarily composed of phospholipids, are one of the most commonly used nanocarriers. Encapsulation in liposomes can improve the solubility and stability of anti-cancer drugs (Zhou et al., 2023). Seo et al. (2023) demonstrated that liposome-encapsulated ICG can remain in gastric cancer tissue for a longer period, aiding in lesion marking and SLN identification. Wang et al. (2022) developed a hollow virus-mimetic MnO2 nanoshell, which, when used to create the nanoprobe PbS@CdS, can adhere to tumor cells and assist in detailed tumor model investigations under NIR-IIb fluorescence imaging, guiding tumor surgery.
Photodynamic therapy (PDT) induces apoptosis in target cells by disrupting the structure and function of organelles through the use of photosensitizers and laser irradiation at specific wavelengths (Tanaka et al., 2021). Due to its high selectivity, minimal invasiveness, and high safety, PDT is widely applied in tumor treatment (Shen et al., 2022). Nanomedicine can enhance the targeted penetration of photosensitizers in PDT, making its integration into PDT a viable option. Current research indicates that PDT has been explored for gastric cancer treatment (Zhang Y. et al., 2023). Yang et al. (2019) demonstrated that the nanoparticle CM/SLN/Ce6 releases its loaded drug in a pH-dependent manner, showing superior anti-gastric cancer effects compared to the free drug in both in vitro and in vivo studies. (Zhu T. et al. (2024) found that IR780-mediated PDT significantly increases ROS levels, effectively inhibits tumor neutrophil ferroptosis, remodels the immunosuppressive TME, and suppresses gastric cancer cell growth. Xin et al. (2022) revealed that AuS-mediated phototherapy enhances photothermal and vapor effects by increasing ROS, rapidly inducing apoptosis, and improving the clinical efficacy of gastric cancer treatment. Mao et al. (2018) developed 5-fluorouracil-doped silk fibroin nanoparticles, which demonstrated ideal tumor-targeting properties when combined with PDT in vivo studies, making it a potential option for cancer treatment. Liao et al. (2021) used a two-photon photosensitizer (Ir-OH) to disrupt mitochondrial redox homeostasis. After irradiation with a 730 nm two-photon laser, they observed enhanced phototoxicity of Ir-OH against human gastric adenocarcinoma due to reduced glutathione levels.
Photothermal therapy (PTT) and PDT are both forms of phototherapy used in the clinical treatment of gastric cancer (Ouyang et al., 2022; Xie et al., 2020). PTT primarily involves materials with high photothermal conversion efficiency to convert light energy into heat, thereby killing tumor cells (Xiong et al., 2020). PTT is known for its high safety, low toxicity, and short treatment duration, and it can be combined with chemotherapy to enhance efficacy and reduce side effects (Sun et al., 2021; Yan et al., 2020). The integration of nanomedicine into PTT has introduced several advantages, such as improved photothermal conversion efficiency, and light-controlled drug release. Yang et al. (2024) synthesized nanoliposomes encapsulating IR780 and EN4, termed Nano-EN-IR@Lip. These nanoliposomes were shown to mediate PTT, which rapidly kills cancer cells, indicating that this nanoliposome system could potentially function as a novel nano-drug delivery platform for the diagnosis and treatment of gastric cancer. Sang et al. (2021) developed a hydrogel named OSA/AHA/BP/PTX, which exhibited excellent photothermal conversion effects at temperatures close to body temperature in both in vitro and in vivo experiments. The hydrogel, when combined with chemotherapy, demonstrated a significantly enhanced anti-tumor efficacy. Xia et al. (2023) designed extracellular vesicles modified with CDH17 nanobodies, which, when irradiated with PTT, polarized macrophages from the M2 to the M1 phenotype, exerted synergistic anti-tumor effects and presented a promising treatment method for gastric cancer. Chen et al. (2022) developed a platform named PP@MnNPs, which combines magnetic resonance imaging with chemodynamic therapy/PTT, aligning with the trend of integrated diagnosis and treatment. Both in vitro and in vivo studies demonstrated that this platform could exert anti-tumor effects and induce ferroptosis. Guo et al. (2020) prepared a novel nanotheranostic agent named HMON@CuS/Gd, which selectively induces mild phototherapy in the TME, inhibiting gastric cancer cell growth while ensuring safety. Wu et al. (2016) synthesized CuInS2/ZnS nanocrystals using PEGylated liposomes as linkers, which exhibited lower toxicity than pure liposomes and could be used for PTT and PDT in cancer treatment (Table 1).
Table 1. Application of nanomedicine and drug delivery systems in gastric cancer radiotherapy, surgery, PDT, and PTT.
Nanomaterials exhibit broad prospects for application in the biomedical field, particularly in the diagnosis and treatment of gastric cancer, due to their unique physicochemical properties. However, the potential toxicity of nanomaterials to healthy tissues is a critical aspect that cannot be overlooked, as it is essential for evaluating their biocompatibility and safety (Deng et al., 2022). When nanomaterials enter a biological system, they can affect healthy tissues through various mechanisms, including physical damage, chemical toxicity, and immune responses (Ling et al., 2023). In clinical practice, a comprehensive evaluation of the biocompatibility and safety of nanomaterials requires consideration of their physicochemical properties, the routes of exposure to the biological system, and the mechanisms of biological responses (Souto et al., 2024). Firstly, nanomaterials can penetrate cell membranes, leading to cell membrane damage, which is the most direct form of physical toxicity (Su et al., 2018). Nanomaterials can also interact with intracellular structures, causing organelle dysfunction. Additionally, variations in the physicochemical properties of nanomaterial surfaces can result in the formation of protein coronas, which may alter the biodistribution, clearance rates, and interactions of nanoparticles with cells, thereby affecting their toxicity. Secondly, chemical toxicity is another significant aspect of the impact of nanomaterials on healthy tissues. Active functional groups or components on the surface of nanomaterials can undergo chemical reactions in the body, producing ROS that induce oxidative stress and cell damage (Lerida-Viso et al., 2023). For example, gold nanoparticles and silver nanoparticles can release metal ions that react with thiol groups within cells, causing cytotoxic effects. Lastly, nanomaterials can also elicit immune responses in the human body, including both immunostimulatory and immunosuppressive effects (Qiu et al., 2017). The size, shape, charge, and surface modifications of nanoparticles can serve as signals for immune cell recognition, leading to the activation or suppression of the immune system, which can result in inflammatory and allergic reactions that impact healthy tissues (Qian et al., 2017). For instance, nanomaterials can activate macrophages and dendritic cells, promoting the release of immune cell factors and triggering localized or systemic inflammation.
Assessing the long-term biocompatibility of nanomaterials with living organisms is a central challenge, especially in their biomedical applications for gastric cancer treatment. Extended exposure to nanomaterials within biological systems can induce a range of biological effects. Ensuring the safety and efficacy of these materials for gastric cancer treatment necessitates systematic optimization and evaluation to mitigate potential risks associated with prolonged exposure (Parvin et al., 2024). Firstly, biodistribution and metabolic kinetics are critical determinants of the long-term biocompatibility of nanomaterials (Andoh et al., 2024). However, clearance mechanisms can vary depending on material properties, leading to prolonged retention within the body and an increased risk of chronic toxicity (Bizeau and Mertz, 2021). For instance, the degradation and stability of surface modifications influence biocompatibility; unstable modifications may lead to excessive release of degradation products, accumulating toxicity over time. Secondly, prolonged exposure to nanomaterials poses genotoxicity risks (Tang et al., 2024). These materials can directly interact with DNA or induce oxidative stress, leading to DNA damage and genetic mutations. Moreover, sustained contact with certain nanomaterials may alter cellular microenvironments, promoting cancer cell proliferation and metastasis, which negatively impacts gastric cancer therapy. Furthermore, the accumulation of nanomaterials within the body during prolonged exposure is a critical concern. Some nanomaterials may accumulate in specific tissues or organs, increasing their burden (Mahajan and Cho, 2022). For example, metal-based nanomaterials can damage the liver and kidneys through the release of metal ions, whereas polymeric nanomaterials may exhibit long-term toxicity via the release of degradation products (Pan et al., 2023) (Figure 3).
Biomimetic nanoparticles are nanoscale carriers constructed by mimicking naturally occurring substances or cellular structures in the biological world. These carriers exhibit excellent biocompatibility and biodegradability, effectively reducing non-targeted effects and enhancing drug delivery efficiency (Anitha et al., 2024). The design inspiration for biomimetic nanoparticles comes from biological structures such as cell membranes, exosomes, and viral particles. By simulating the characteristics of these biological structures, the targeting, biocompatibility, and therapeutic efficacy of nano-drugs can be significantly improved (Xu et al., 2016). Wang et al. (2021) developed a drug delivery tool called the RP (P/T) erythrocyte membrane biomimetic nanosystem. This emerging drug delivery tool, which co-loads PTX and triptolide, can prolong the circulation time of the drug in the blood and evade immune surveillance, making it a promising therapy for gastric cancer. Lei et al. (2023) synthesized a new nano-drug called GIC@HM·NPs with a biomimetic coating, which has the functions of PTT and chemotherapy. This nano-drug not only improves biocompatibility but also allows for more precise drug delivery to tumor sites, enhancing drug utilization efficiency and providing a safer, more efficient, and more precise method for gastric cancer treatment. Zhang L. et al. (2023) designed a co-delivery system called M@BP. In a mouse model of orthotopic gastric cancer, M@BP can effectively target and accumulate in gastric cancer cells, blocking the activation of CDK9 and BRD4 and impeding the growth of gastric cancer cells.
Nanotheranostics is an integrated nanotechnology that combines diagnosis and therapy (Arshad et al., 2021), aiming to construct a multifunctional platform capable of simultaneously achieving disease monitoring and treatment. By co-loading diagnostic molecules, such as fluorescent dyes, magnetic nanoparticles, or radioactive isotopes, and therapeutic molecules, such as chemotherapeutic agents, proteins, or nucleic acids, within biomimetic nanoparticles, it is possible to achieve precise targeting and real-time tracking of gastric cancer, as well as dynamic monitoring of its microenvironment, while simultaneously performing effective targeted therapy (Kumari and Sunil, 2021). Bao et al. (2016) developed a functionalized gold nanoprism named AuNprs, which can be used simultaneously for in situ photoacoustic imaging, angiography, and localized thermal therapy. This represents a highly sensitive in vivo nanotheranostic platform for detecting gastric cancer tumors and performing targeted therapy. In vivo studies have demonstrated that it can reduce tumor size and improve survival rates in mice following localized thermal therapy. Wu et al. (2019) developed a theranostic gold nanoparticle-boron cage assembly, named B-AuNPs, for evaluating the feasibility of boron neutron capture therapy. This study advanced the integrative diagnosis and treatment of gastric cancer by creating detectable boron-containing gold nanoparticles. Zhang et al. (2023a) reported a series of nanobody-derived CD47-targeted agents, among which [68Ga]Ga-NOTA-C2 and [89Zr]Zr-DFO-ABDC2 exhibited longer circulation times. The study suggests that the optimization of CD47-targeted theranostic approaches may provide new strategies for the treatment of CD47-targeted solid tumors (Figure 4 and Table 2).
Gastric cancer, one of the most common types of cancer, continues to have high incidence and mortality rates, placing significant pressure on healthcare systems. While existing treatments have achieved some success, issues such as drug resistance, toxic side effects, and recurrence remain significant challenges. In terms of diagnosis, nanoprobes, due to their large surface area, can easily bind to gastric cancer cells, allowing for the detection of early-stage gastric cancer using fluorescence techniques. The introduction of nanotechnology into gastric cancer diagnosis has the potential to develop more specific and sensitive probes, enhancing diagnostic accuracy. By leveraging the unique physical and chemical properties of nanomaterials, future advancements could integrate optical imaging, magnetic resonance imaging, and ultrasound imaging to achieve multimodal diagnostic fusion, aiding in the accurate staging of tumors. In recent years, significant efforts have been directed toward the integration of diagnosis and treatment in cancer research. A variety of nanomaterials capable of both diagnosing and treating cancer have been developed. These materials enable the initiation of personalized treatment plans based on diagnostic outcomes, thereby minimizing the physical burden on patients associated with multiple diagnostic and therapeutic procedures. In the realm of treatment, nanomedicine has demonstrated considerable promise in several areas, including the targeted delivery of small molecules to tumor cells, the reduction of toxicity, the enhancement of the efficacy of existing treatments, and the improvement of patient quality of life. Nano-drug delivery systems can precisely target tumor cells, increasing drug concentration within the TME, prolonging drug circulation time in the bloodstream, and thereby inhibiting tumor growth, which leads to improved clinical outcomes. Furthermore, nanomedicine can augment the therapeutic effects of PDT and PTT by enhancing the efficiency of light-to-heat and light-to-kinetic energy conversion, aiming to optimize clinical results.
Looking ahead, the development of intelligent nanomedicine and delivery systems represents a key direction for future research. These intelligent systems can monitor changes in the TME in real-time through integrated sensors and adjust drug release accordingly. For instance, temperature- and pH-sensitive nano-drug delivery systems can release drugs under specific TME conditions, thereby achieving targeted therapeutic effects. As nanotechnology continues to evolve, researchers will focus on the development of novel nanocarriers. For example, the use of biocompatible materials such as chitosan as carrier materials can lead to the creation of nanomedicines with higher bioavailability. The integration of novel nanomedicines with advanced drug delivery systems can address diverse treatment needs. The application of nanotechnology in gastric cancer diagnosis and treatment is very promising, but its clinical translation still faces many challenges. For instance, the relationship between the preparation methods, structure, composition, and efficacy of nanomedicines is not yet fully understood; development costs are high, posing significant economic challenges; and many drugs and carriers are still in the research and development phase, not yet meeting clinical standards. Most existing research is still confined to animal experimentation, and the stability of these technologies in the human physiological environment remains uncertain. Researchers should strengthen collaboration with fields such as materials science, chemistry, biology, and engineering, integrating cutting-edge technologies from various disciplines to drive the innovation and development of nanotechnology. For instance, artificial intelligence algorithms can be utilized to optimize the design of nanomedicines, and microfluidic technology can enable high-throughput preparation and screening of nanomedicines. Currently, the most pressing issue to address is the lack of systematic preclinical research and clinical trial data for nanomedicines. Researchers should intensify preclinical studies on nanomedicines, including pharmacokinetic, pharmacodynamic, and toxicological evaluations, to ensure their safety and efficacy in animal models. Additionally, multi-center, large-sample clinical trials are needed to evaluate the efficacy, tolerability, and long-term safety of nanomedicines in gastric cancer patients. Accelerating the clinical translation process of nanomedicines can be achieved through the establishment of standardized clinical trial protocols and data-sharing platforms. Despite the numerous challenges in applying nanotechnology to the clinical diagnosis and treatment of gastric cancer, the continuous advancement of nanotechnology and the deepening of interdisciplinary collaboration are expected to make nanomedicine and delivery systems powerful tools for diagnosing and treating gastric cancer, offering new hope to patients.
SW: Writing–original draft, Writing–review and editing, Conceptualization. JL: Writing–review and editing, Supervision, Conceptualization. ZhZ: Writing–review and editing, Resources. SC: Writing–review and editing. ZiZ: Writing–review and editing. YB: Writing–review and editing. YX: Writing–review and editing. CM: Funding acquisition, Writing–review and editing.
The author(s) declare that financial support was received for the research, authorship, and/or publication of this article. This work is supported by the Henan Province Administration of Traditional Chinese Medicine (NO: 2022ZY1089) (NO: 2024ZY1013), Leading Talents of Henan Province (NO: (2021)-8), and Henan Provincial Hospital of Traditional Chinese Medicine Doctoral Fund (NO: 2024BSJJ10).
The authors declare that the research was conducted in the absence of any commercial or financial relationships that could be construed as a potential conflict of interest.
The author(s) declare that no Generative AI was used in the creation of this manuscript.
All claims expressed in this article are solely those of the authors and do not necessarily represent those of their affiliated organizations, or those of the publisher, the editors and the reviewers. Any product that may be evaluated in this article, or claim that may be made by its manufacturer, is not guaranteed or endorsed by the publisher.
The Supplementary Material for this article can be found online at: https://www.frontiersin.org/articles/10.3389/fbioe.2025.1565999/full#supplementary-material
Abedi, C. H. Z., Ghanbarikondori, P., Mortazavi, M. E., Hheidari, A., Saberian, E., Mozaffari, E., et al. (2023). Characteristics and cytotoxic effects of nano-liposomal paclitaxel on gastric cancer cells. Asian Pac J. Cancer Prev. 24, 3291–3296. doi:10.31557/APJCP.2023.24.9.3291
Alam, J., Dilnawaz, F., Sahoo, S. K., Singh, D. V., Mukhopadhyay, A. K., Hussain, T., et al. (2022). Curcumin encapsulated into biocompatible co-polymer plga nanoparticle enhanced anti-gastric cancer and anti-helicobacter pylori effect. Asian Pac J. Cancer Prev. 23, 61–70. doi:10.31557/APJCP.2022.23.1.61
Alsharabasy, A. M., and Pandit, A. (2024). Hyaluronan-based hydrogels for 3d modeling of tumor tissues. Tissue Eng. Part C Methods 30, 452–499. doi:10.1089/ten.tec.2024.0271
Andoh, V., Ocansey, D., Naveed, H., Wang, N., Chen, L., Chen, K., et al. (2024). The advancing role of nanocomposites in cancer diagnosis and treatment. Int. J. Nanomedicine 19, 6099–6126. doi:10.2147/IJN.S471360
Anitha, K., Chenchula, S., Surendran, V., Shvetank, B., Ravula, P., Milan, R., et al. (2024). Advancing cancer theranostics through biomimetics: a comprehensive review. Heliyon 10, e27692. doi:10.1016/j.heliyon.2024.e27692
Arshad, R., Fatima, I., Sargazi, S., Rahdar, A., Karamzadeh-Jahromi, M., Pandey, S., et al. (2021). Novel perspectives towards rna-based nano-theranostic approaches for cancer management. Nanomater. (Basel) 11, 3330. doi:10.3390/nano11123330
Bao, C., Conde, J., Pan, F., Li, C., Zhang, C., Tian, F., et al. (2016). Gold nanoprisms as a hybrid in vivo cancer theranostic platform for in situ photoacoustic imaging, angiography, and localized hyperthermia. Nano Res. 9, 1043–1056. doi:10.1007/s12274-016-0996-y
Bizeau, J., and Mertz, D. (2021). Design and applications of protein delivery systems in nanomedicine and tissue engineering. Adv. Colloid Interface Sci. 287, 102334. doi:10.1016/j.cis.2020.102334
Bor, G., Azmi, I. D. M., and Yaghmur, A. (2019). Nanomedicines for cancer therapy: current status, challenges and future prospects. Ther. Deliv. 10, 113–132. doi:10.4155/tde-2018-0062
Bray, F., Laversanne, M., Sung, H., Ferlay, J., Siegel, R. L., Soerjomataram, I., et al. (2024). Global cancer statistics 2022: globocan estimates of incidence and mortality worldwide for 36 cancers in 185 countries. Ca Cancer J. Clin. 74, 229–263. doi:10.3322/caac.21834
Broekgaarden, M., Bulin, A., Porret, E., Musnier, B., Chovelon, B., Ravelet, C., et al. (2020). Surface functionalization of gold nanoclusters with arginine: a trade-off between microtumor uptake and radiotherapy enhancement. Nanoscale 12, 6959–6963. doi:10.1039/d0nr01138j
Cabral, H., Miyata, K., Osada, K., and Kataoka, K. (2018). Block copolymer micelles in nanomedicine applications. Chem. Rev. 118, 6844–6892. doi:10.1021/acs.chemrev.8b00199
Cai, Y., Huang, L., Sun, W., Xu, C., Ren, X., Ye, Y., et al. (2023). Noninvasive analysis of exhaled breath for gastric cancer diagnosis using paper-based smartphone nano-optoelectronic noses. Sens. Actuators B Chem. 381, 133411. doi:10.1016/j.snb.2023.133411
Cao, D., Lin, H., Liu, Z., Qiu, J., Ge, S., Hua, W., et al. (2023). Pca-tlnn-based sers analysis platform for label-free detection and identification of cisplatin-treated gastric cancer. Sens. Actuators B Chem. 375, 132903. doi:10.1016/j.snb.2022.132903
Che, J., Najer, A., Blakney, A. K., McKay, P. F., Bellahcene, M., Winter, C. W., et al. (2020). Neutrophils enable local and non-invasive liposome delivery to inflamed skeletal muscle and ischemic heart. Adv. Mater 32, e2003598. doi:10.1002/adma.202003598
Chen, J., Liu, X., Zhao, S., Chen, H., Lu, T., Wang, J., et al. (2023). Carboxymethylated alginate-resiquimod micelles reverse the immunosuppressive tumor microenvironment and synergistically enhance the chemotherapy and immunotherapy for gastric cancer. Acs Appl. Mater Interfaces 15, 35999–36012. doi:10.1021/acsami.3c06828
Chen, M., Liu, T., Chen, Y., and Chen, M. (2021). Combining augmented radiotherapy and immunotherapy through a nano-gold and bacterial outer-membrane vesicle complex for the treatment of glioblastoma. Nanomater. (Basel) 11, 1661. doi:10.3390/nano11071661
Chen, Y., Zhang, Y., Pan, F., Liu, J., Wang, K., Zhang, C., et al. (2016). Breath analysis based on surface-enhanced Raman scattering sensors distinguishes early and advanced gastric cancer patients from healthy persons. Acs Nano 10, 8169–8179. doi:10.1021/acsnano.6b01441
Chen, Z., Li, Z., Li, C., Huang, H., Ren, Y., Li, Z., et al. (2022). Manganese-containing polydopamine nanoparticles as theranostic agents for magnetic resonance imaging and photothermal/chemodynamic combined ferroptosis therapy treating gastric cancer. Drug Deliv. 29, 1201–1211. doi:10.1080/10717544.2022.2059124
Delahunty, I., Li, J., Jiang, W., Lee, C., Yang, X., Kumar, A., et al. (2022). 7-dehydrocholesterol encapsulated polymeric nanoparticles as a radiation-responsive sensitizer for enhancing radiation therapy. Small 18, e2200710. doi:10.1002/smll.202200710
Deng, S., Gu, J., Jiang, Z., Cao, Y., Mao, F., Xue, Y., et al. (2022). Application of nanotechnology in the early diagnosis and comprehensive treatment of gastrointestinal cancer. J. Nanobiotechnology 20, 415. doi:10.1186/s12951-022-01613-4
Diniz, F., Lamas, S., Osorio, H., Aguiar, P., Freitas, D., Gartner, F., et al. (2023). Nanoparticles targeting sialyl-tn for efficient tyrosine kinase inhibitor delivery in gastric cancer. Acta Biomater. 170, 142–154. doi:10.1016/j.actbio.2023.08.014
Du, Y., Fan, K., Zhang, H., Li, L., Wang, P., He, J., et al. (2018). Endoscopic molecular imaging of early gastric cancer using fluorescently labeled human h-ferritin nanoparticle. Nanomedicine 14, 2259–2270. doi:10.1016/j.nano.2018.07.007
Garcia-Pinel, B., Porras-Alcala, C., Ortega-Rodriguez, A., Sarabia, F., Prados, J., Melguizo, C., et al. (2019). Lipid-based nanoparticles: application and recent advances in cancer treatment. Nanomater. (Basel) 9, 638. doi:10.3390/nano9040638
Geerts, J. F. M., van der Zijden, C. J., van der Sluis, P. C., Spaander, M. C. W., Nieuwenhuijzen, G. A. P., Rosman, C., et al. (2024). Perioperative chemotherapy for gastro-esophageal or gastric cancer: anthracyclin triplets versus flot. Cancers (Basel) 16, 1291. doi:10.3390/cancers16071291
Guo, W., Chen, Z., Chen, J., Feng, X., Yang, Y., Huang, H., et al. (2020). Biodegradable hollow mesoporous organosilica nanotheranostics (hmon) for multi-mode imaging and mild photo-therapeutic-induced mitochondrial damage on gastric cancer. J. Nanobiotechnology 18, 99. doi:10.1186/s12951-020-00653-y
Guo, W., Ren, Y., Chen, Z., Shen, G., Lu, Y., Zhou, H., et al. (2023). Targeted magnetic resonance imaging/near-infrared dual-modal imaging and ferroptosis/starvation therapy of gastric cancer with peritoneal metastasis. Adv. Funct. Mater 33. doi:10.1002/adfm.202213921
Han, J., Wang, Y., Zhu, L., Cui, Y., Li, L., Zeng, Z., et al. (2020). Preventing the spread of covid-19 in digestive endoscopy during the resuming period: meticulous execution of screening procedures. Gastrointest. Endosc. 92, 445–447. doi:10.1016/j.gie.2020.03.3855
Harmsen, S., Rogalla, S., Huang, R., Spaliviero, M., Neuschmelting, V., Hayakawa, Y., et al. (2019). Detection of premalignant gastrointestinal lesions using surface-enhanced resonance Raman scattering-nanoparticle endoscopy. Acs Nano 13, 1354–1364. doi:10.1021/acsnano.8b06808
He, C., Chan, C., Weichselbaum, R. R., Fleming, G. F., Yamada, S. D., and Lin, W. (2015). Nanomedicine for combination therapy of cancer. Ebiomedicine 2, 366–367. doi:10.1016/j.ebiom.2015.05.013
Huang, H., Yang, D., Liu, M., Wang, X., Zhang, Z., Zhou, G., et al. (2017). Ph-sensitive au-bsa-dox-fa nanocomposites for combined ct imaging and targeted drug delivery. Int. J. Nanomedicine 12, 2829–2843. doi:10.2147/IJN.S128270
Huang, L., Zhu, Y., Xu, C., Cai, Y., Yi, Y., Li, K., et al. (2022). Noninvasive diagnosis of gastric cancer based on breath analysis with a tubular surface-enhanced Raman scattering sensor. Acs Sens. 7, 1439–1450. doi:10.1021/acssensors.2c00146
Jeevanandam, J., Barhoum, A., Chan, Y. S., Dufresne, A., and Danquah, M. K. (2018). Review on nanoparticles and nanostructured materials: history, sources, toxicity and regulations. Beilstein J. Nanotechnol. 9, 1050–1074. doi:10.3762/bjnano.9.98
Jian, Y., Zhao, M., Cao, J., Fan, T., Bu, W., Yang, Y., et al. (2020). A gastric cancer peptide gxi-modified nano-lipid carriers encapsulating paclitaxel: design and evaluation of anti-tumor activity. Drug Des. Dev. Ther. 14, 2355–2370. doi:10.2147/DDDT.S233023
Kaur, J., Gulati, M., Jha, N. K., Disouza, J., Patravale, V., Dua, K., et al. (2022). Recent advances in developing polymeric micelles for treating cancer: breakthroughs and bottlenecks in their clinical translation. Drug Discov. Today 27, 1495–1512. doi:10.1016/j.drudis.2022.02.005
Ke, Y., Zhu, J., Chu, Y., Cen, L., Fu, Y., Fan, X., et al. (2022). Bifunctional fusion membrane-based hydrogel enhances antitumor potency of autologous cancer vaccines by activating dendritic cells. Adv. Funct. Mater 32. doi:10.1002/adfm.202201306
Kong, J., Cai, M., Zhu, R., Zhang, Y., Du, Y., Jing, X., et al. (2024). Cpp10-targeted photoactivatable mof nanosystem for combined photodynamic therapy-chemotherapy of cancer. J. Sci. 9, 100761. doi:10.1016/j.jsamd.2024.100761
Kukreja, A., Kang, B., Kim, H., Jang, E., Son, H. Y., Huh, Y., et al. (2017). Preparation of gold core-mesoporous iron-oxide shell nanoparticles and their application as dual mrct contrast agent in human gastric cancer cells. J. Ind. Eng. Chem. 48, 56–65. doi:10.1016/j.jiec.2016.12.020
Kumari, R., and Sunil, D. (2021). A mechanistic insight into benefits of aggregation induced emissive luminogens in cancer. J. Drug Target 29, 592–608. doi:10.1080/1061186X.2020.1868479
Kwak, M. H., Yun, S. K., Yang, S. M., Myeong, S., and Park, J. M. (2024). Gastric cancer specific drug delivery with hydrophilic peptide probe conjugation. Biomater. Sci. 12, 440–452. doi:10.1039/d3bm01590d
Lei, Z., Fan, J., Li, X., Chen, Y., Shi, D., Xie, H., et al. (2023). Biomimetic graphene oxide quantum dots nanoparticles targeted photothermal-chemotherapy for gastric cancer. J. Drug Target 31, 320–333. doi:10.1080/1061186X.2022.2162060
Lerida-Viso, A., Estepa-Fernandez, A., Garcia-Fernandez, A., Marti-Centelles, V., and Martinez-Manez, R. (2023). Biosafety of mesoporous silica nanoparticles; towards clinical translation. Adv. Drug Deliv. Rev. 201, 115049. doi:10.1016/j.addr.2023.115049
Li, B., Ashrafizadeh, M., and Jiao, T. (2024). Biomedical application of metal-organic frameworks (mofs) in cancer therapy: stimuli-responsive and biomimetic nanocomposites in targeted delivery, phototherapy and diagnosis. Int. J. Biol. Macromol. 260, 129391. doi:10.1016/j.ijbiomac.2024.129391
Li, D., Fan, Y., Liu, M., Huang, S., and Wang, S. (2023). The effect of using albumin-perfluorohexane/cisplatin-magnetite nanoparticles produced by hydrothermal method against gastric cancer cells through combination therapy. Arab. J. Chem. 16, 104758. doi:10.1016/j.arabjc.2023.104758
Li, J., Yao, M., Shao, Y., and Yao, D. (2018). The application of bio-nanotechnology in tumor diagnosis and treatment: a view. Nanotechnol. Rev. 7, 257–266. doi:10.1515/ntrev-2018-0011
Li, X., Ai, S., Lu, X., Liu, S., and Guan, W. (2021). Nanotechnology-based strategies for gastric cancer imaging and treatment. Rsc Adv. 11, 35392–35407. doi:10.1039/d1ra01947c
Liao, X., Shen, J., Wu, W., Kuang, S., Lin, M., Karges, J., et al. (2021). A mitochondrial-targeting iridium(iii) complex for h 2 o 2 -responsive and oxidative stress amplified two-photon photodynamic therapy. Inorg. Chem. Front. 8, 5045–5053. doi:10.1039/d1qi01038g
Ling, L., Ouyang, Y., and Hu, Y. (2023). Research trends on nanomaterials in gastric cancer: a bibliometric analysis from 2004 to 2023. J. Nanobiotechnology 21, 248. doi:10.1186/s12951-023-02033-8
Liu, N., Liu, M., Fu, S., Wang, J., Tang, H., Isah, A. D., et al. (2022a). Ang2-targeted combination therapy for cancer treatment. Front. Immunol. 13, 949553. doi:10.3389/fimmu.2022.949553
Liu, Q., Chu, Y., Shao, J., Qian, H., Yang, J., Sha, H., et al. (2023a). Benefits of an immunogenic personalized neoantigen nanovaccine in patients with high-risk gastric/gastroesophageal junction cancer. Adv. Sci. (Weinh) 10, e2203298. doi:10.1002/advs.202203298
Liu, Y., Li, D., Chen, Y., Liu, Y., Lin, Y., Huang, X., et al. (2023b). Integrated bioinformatics analysis for conducting a prognostic model and identifying immunotherapeutic targets in gastric cancer. Bmc Bioinforma. 24, 191. doi:10.1186/s12859-023-05312-1
Liu, Z., Wang, K., Wang, T., Wang, Y., and Ge, Y. (2022b). Copper nanoparticles supported on polyethylene glycol-modified magnetic fe 3 o 4 nanoparticles: its anti-human gastric cancer investigation. Arab. J. Chem. 15, 103523. doi:10.1016/j.arabjc.2021.103523
Luo, Q., Fan, C., Ying, W., Peng, X., Hu, Y., Luan, Z., et al. (2023). In vivo anchoring bis-pyrene probe for molecular imaging of early gastric cancer by endoscopic techniques. Adv. Sci. (Weinh) 10, e2203918. doi:10.1002/advs.202203918
Ma, D., Zhang, Y., Shao, X., Wu, C., and Wu, J. (2022). Pet/ct for predicting occult lymph node metastasis in gastric cancer. Curr. Oncol. 29, 6523–6539. doi:10.3390/curroncol29090513
Ma, J., Yao, Q., Lv, S., Yi, J., Zhu, D., Zhu, C., et al. (2024). Integrated triple signal amplification strategy for ultrasensitive electrochemical detection of gastric cancer-related microrna utilizing mos 2 -based nanozyme, hybridization chain reaction, and horseradish peroxidase. J. Nanobiotechnology 22, 596. doi:10.1186/s12951-024-02848-z
Mahajan, H., and Cho, S. (2022). Novel au nanorod/cu(2)o composite nanoparticles for a high-performance supercapacitor. Rsc Adv. 12, 9112–9120. doi:10.1039/d2ra00812b
Mao, B., Liu, C., Zheng, W., Li, X., Ge, R., Shen, H., et al. (2018). Cyclic crgdfk peptide and chlorin e6 functionalized silk fibroin nanoparticles for targeted drug delivery and photodynamic therapy. Biomaterials 161, 306–320. doi:10.1016/j.biomaterials.2018.01.045
Mollasalehi, H., and Shajari, E. (2021). A colorimetric nano-biosensor for simultaneous detection of prevalent cancers using unamplified cell-free ribonucleic acid biomarkers. Bioorg Chem. 107, 104605. doi:10.1016/j.bioorg.2020.104605
Moradian, F., and Mohammadzadeh, S. (2023). Evaluation of the effect of nano-encapsulated lactoferrin on the expression of bak and bax genes in gastric cancer cell line ags and study of the molecular docking of lactoferrin with these proteins. Gene 866, 147355. doi:10.1016/j.gene.2023.147355
Nosrati, H., Attari, E., Abhari, F., Barsbay, M., Ghaffarlou, M., Mousazadeh, N., et al. (2022). Complete ablation of tumors using synchronous chemoradiation with bimetallic theranostic nanoparticles. Bioact. Mater 7, 74–84. doi:10.1016/j.bioactmat.2021.05.015
Obata, M., and Hirohara, S. (2023). Raft synthesis and characterization of poly(butyl-co -2-(n, n -dimethylamino)ethyl acrylates)- block -poly(polyethylene glycol monomethyl ether acrylate) as a photosensitizer carrier for photodynamic therapy. Mater. (Basel) 16, 4192. doi:10.3390/ma16114192
Ouyang, J., Xie, A., Zhou, J., Liu, R., Wang, L., Liu, H., et al. (2022). Minimally invasive nanomedicine: nanotechnology in photo-/ultrasound-/radiation-/magnetism-mediated therapy and imaging. Chem. Soc. Rev. 51, 4996–5041. doi:10.1039/d1cs01148k
Pan, H., Dong, Y., Gong, L., Zhai, J., Song, C., Ge, Z., et al. (2022). Sensing gastric cancer exosomes with mos2-based sers aptasensor. Biosens. Bioelectron. 215, 114553. doi:10.1016/j.bios.2022.114553
Pan, Y., Wu, M., Shi, M., Shi, P., Zhao, N., Zhu, Y., et al. (2023). An overview to molecularly imprinted electrochemical sensors for the detection of bisphenol a. Sensors (Basel) 23, 8656. doi:10.3390/s23208656
Parvin, N., Kumar, V., Joo, S. W., and Mandal, T. K. (2024). Emerging trends in nanomedicine: carbon-based nanomaterials for healthcare. Nanomater. (Basel) 14, 1085. doi:10.3390/nano14131085
Qian, X., Gu, Z., and Chen, Y. (2017). Two-dimensional black phosphorus nanosheets for theranostic nanomedicine. Mater Horiz. 4, 800–816. doi:10.1039/c7mh00305f
Qiu, H., Min, Y., Rodgers, Z., Zhang, L., and Wang, A. Z. (2017). Nanomedicine approaches to improve cancer immunotherapy. Wiley Interdiscip. Rev. Nanomed Nanobiotechnol 9. doi:10.1002/wnan.1456
Rai, A., Noor, S., Ahmad, S. I., Alajmi, M. F., Hussain, A., Abbas, H., et al. (2021). Recent advances and implication of bioengineered nanomaterials in cancer theranostics. Med. Kaunas. 57, 91. doi:10.3390/medicina57020091
Ruan, J., Ji, J., Song, H., Qian, Q., Wang, K., Wang, C., et al. (2012). Fluorescent magnetic nanoparticle-labeled mesenchymal stem cells for targeted imaging and hyperthermia therapy of in vivo gastric cancer. Nanoscale Res. Lett. 7, 309. doi:10.1186/1556-276X-7-309
Salapa, J., Bushman, A., Lowe, K., and Irudayaraj, J. (2020). Nano drug delivery systems in upper gastrointestinal cancer therapy. Nano Converg. 7, 38. doi:10.1186/s40580-020-00247-2
Sang, S., Jiang, Z., Xie, N., Rao, H., Liao, K., Hu, Q., et al. (2021). Black phosphorus nanosheets and paclitaxel encapsulated hydrogel for synergistic photothermal-chemotherapy. Nanophotonics 10, 2625–2637. doi:10.1515/nanoph-2021-0089
Seo, K., Zhang, Y., Toyota, T., Hayashi, H., Hirata, S., Yamaguchi, T., et al. (2023). Release of liposomally formulated near-infrared fluorescent probes included in giant cluster vesicles by ultrasound irradiation. Ultrasonics 134, 107102. doi:10.1016/j.ultras.2023.107102
Shao, P., Dong, D., Huang, Y., Wang, Y., Pan, L., Zhu, Q., et al. (2023). Aptamer-based functionalized sers biosensor for rapid and ultrasensitive detection of gastric cancer-related biomarkers. Int. J. Nanomedicine 18, 7523–7532. doi:10.2147/IJN.S434854
Shen, W., Hu, T., Liu, X., Zha, J., Meng, F., Wu, Z., et al. (2022). Defect engineering of layered double hydroxide nanosheets as inorganic photosensitizers for nir-iii photodynamic cancer therapy. Nat. Commun. 13, 3384. doi:10.1038/s41467-022-31106-9
Shi, H., Sun, Y., Yan, R., Liu, S., Zhu, L., Liu, S., et al. (2019). Magnetic semiconductor gd-doping cus nanoparticles as activatable nanoprobes for bimodal imaging and targeted photothermal therapy of gastric tumors. Nano Lett. 19, 937–947. doi:10.1021/acs.nanolett.8b04179
Shi, H., Yan, R., Wu, L., Sun, Y., Liu, S., Zhou, Z., et al. (2018). Tumor-targeting cus nanoparticles for multimodal imaging and guided photothermal therapy of lymph node metastasis. Acta Biomater. 72, 256–265. doi:10.1016/j.actbio.2018.03.035
Siegel, R. L., Miller, K. D., Fuchs, H. E., and Jemal, A. (2022). Cancer statistics, 2022. Ca Cancer J. Clin. 72, 7–33. doi:10.3322/caac.21708
Skapars, R., Gasenko, E., Broza, Y. Y., Sivins, A., Polaka, I., Bogdanova, I., et al. (2023). Breath volatile organic compounds in surveillance of gastric cancer patients following radical surgical management. Diagn. (Basel) 13, 1670. doi:10.3390/diagnostics13101670
Song, X., Cai, H., Shi, Z., Li, Z., Zheng, X., Yang, K., et al. (2024). Enzyme-responsive branched glycopolymer-based nanoassembly for co-delivery of paclitaxel and akt inhibitor toward synergistic therapy of gastric cancer. Adv. Sci. (Weinh) 11, e2306230. doi:10.1002/advs.202306230
Souto, E. B., Blanco-Llamero, C., Krambeck, K., Kiran, N. S., Yashaswini, C., Postwala, H., et al. (2024). Regulatory insights into nanomedicine and gene vaccine innovation: safety assessment, challenges, and regulatory perspectives. Acta Biomater. 180, 1–17. doi:10.1016/j.actbio.2024.04.010
Su, H., Wang, Y., Gu, Y., Bowman, L., Zhao, J., and Ding, M. (2018). Potential applications and human biosafety of nanomaterials used in nanomedicine. J. Appl. Toxicol. 38, 3–24. doi:10.1002/jat.3476
Sun, H., Zhang, Q., Li, J., Peng, S., Wang, X., and Cai, R. (2021). Near-infrared photoactivated nanomedicines for photothermal synergistic cancer therapy. Nano Today 37, 101073. doi:10.1016/j.nantod.2020.101073
Sun, L., Meng, C., Zhang, Z., Luo, Y., Yang, Z., and Yao, H. (2024). Opportunities and challenges of indocyanine green in gastrointestinal cancers for intraoperative and nano-medicine application. Cancer Nanotechnol. 15, 12. doi:10.1186/s12645-024-00251-9
Tanaka, M., Sasaki, M., Suzuki, T., Nishie, H., and Kataoka, H. (2021). Combination of talaporfin photodynamic therapy and poly (adp-ribose) polymerase (parp) inhibitor in gastric cancer. Biochem. Biophys. Res. Commun. 539, 1–7. doi:10.1016/j.bbrc.2020.12.073
Tang, W., Yang, Z., He, L., Deng, L., Fathi, P., Zhu, S., et al. (2021). A hybrid semiconducting organosilica-based o 2 nanoeconomizer for on-demand synergistic photothermally boosted radiotherapy. Nat. Commun. 12, 523. doi:10.1038/s41467-020-20860-3
Tang, W., Zhang, X., Hong, H., Chen, J., Zhao, Q., and Wu, F. (2024). Computational nanotoxicology models for environmental risk assessment of engineered nanomaterials. Nanomater. (Basel) 14, 155. doi:10.3390/nano14020155
Thrift, A. P., and El-Serag, H. B. (2020). Burden of gastric cancer. Clin. Gastroenterol. Hepatol. 18, 534–542. doi:10.1016/j.cgh.2019.07.045
Tian, S., Peng, P., Li, J., Deng, H., Zhan, N., Zeng, Z., et al. (2020). Serpinh1 regulates emt and gastric cancer metastasis via the wnt/β-catenin signaling pathway. Aging-Us 12, 3574–3593. doi:10.18632/aging.102831
Wang, G., Yan, J., Tian, H., Li, B., Yu, X., Feng, Y., et al. (2024a). Dual-epigenetically relieving the myc-correlated immunosuppression via an advanced nano-radiosensitizer potentiates cancer immuno-radiotherapy. Adv. Mater 36, e2312588. doi:10.1002/adma.202312588
Wang, H., Niu, H., Luo, X., Zhu, N., Xiang, J., He, Y., et al. (2023a). Radiosensitizing effects of pyrogallol-loaded mesoporous or-ganosilica nanoparticles on gastric cancer by amplified ferroptosis. Front. Bioeng. Biotechnol. 11, 1171450. doi:10.3389/fbioe.2023.1171450
Wang, K., Ruan, J., Qian, Q., Song, H., Bao, C., Zhang, X., et al. (2011). Brcaa1 monoclonal antibody conjugated fluorescent magnetic nanoparticles for in vivo targeted magnetofluorescent imaging of gastric cancer. J. Nanobiotechnology 9, 23. doi:10.1186/1477-3155-9-23
Wang, P., Li, J., Wei, M., Yang, R., Lou, K., Dang, Y., et al. (2022). Tumor-microenvironment triggered signal-to-noise boosting nanoprobes for nir-iib fluorescence imaging guided tumor surgery and nir-ii photothermal therapy. Biomaterials 287, 121636. doi:10.1016/j.biomaterials.2022.121636
Wang, Q., Zhang, C., Shen, G., Liu, H., Fu, H., and Cui, D. (2014). Fluorescent carbon dots as an efficient sirna nanocarrier for its interference therapy in gastric cancer cells. J. Nanobiotechnology 12, 58. doi:10.1186/s12951-014-0058-0
Wang, S., Chi, C., Cheng, H., Pan, X., Li, S., Zhang, F., et al. (2018). Photothermal adjunctive cytoreductive surgery for treating peritoneal metastasis of gastric cancer. Small Methods 2. doi:10.1002/smtd.201700368
Wang, S. W., Jiang, H., Wang, J., Wu, H. S., Wu, T., Ni, M. N., et al. (2021). Superior in vitro anticancer effect of biomimetic paclitaxel and triptolide co-delivery system in gastric cancer. J. Biomed. Res. 35, 327–338. doi:10.7555/JBR.35.20210102
Wang, Z., Mei, L., Yang, X., Jiang, T., Sun, T., Su, Y., et al. (2023b). Near-infrared fluorophores methylene blue for targeted imaging of the stomach in intraoperative navigation. Front. Bioeng. Biotechnol. 11, 1172073. doi:10.3389/fbioe.2023.1172073
Wang, Z., Pang, S., Liu, X., Dong, Z., Tian, Y., Ashrafizadeh, M., et al. (2024b). Chitosan- and hyaluronic acid-based nanoarchitectures in phototherapy: combination cancer chemotherapy, immunotherapy and gene therapy. Int. J. Biol. Macromol. 273, 132579. doi:10.1016/j.ijbiomac.2024.132579
Wei, X., Wang, J., Liang, M., and Song, M. (2022). Development of functional nanomedicines for tumor associated macrophages-focused cancer immunotherapy. Theranostics 12, 7821–7852. doi:10.7150/thno.78572
Wei, Y., Cai, J., Wang, L., Yang, J., Li, Y., Li, X., et al. (2024). Dynamic change in the peritoneal cancer index based on ct after chemotherapy in the overall survival prediction of gastric cancer patients with peritoneal metastasis. J. Cancer Res. Clin. Oncol. 150, 222. doi:10.1007/s00432-024-05707-4
Wei, Y., Zhu, Y., and Wang, M. (2016). Surface-enhanced Raman spectroscopy of gastric cancer serum with gold nanoparticles/silicon nanowire arrays. Opt. (Stuttg) 127, 7902–7907. doi:10.1016/j.ijleo.2016.05.146
Wu, C., Lin, J., Chang, W., Hsieh, C., Wu, C., Chen, H., et al. (2019). Development of theranostic active-targeting boron-containing gold nanoparticles for boron neutron capture therapy (bnct). Colloids Surf. B Biointerfaces 183, 110387. doi:10.1016/j.colsurfb.2019.110387
Wu, L. Z., Ye, Y., Wang, Z. X., Ma, D., Li, L., Xi, G. H., et al. (2021). Sensitive detection of single-nucleotide polymorphisms by solid nanopores integrated with dna probed nanoparticles. Front. Bioeng. Biotechnol. 9, 690747. doi:10.3389/fbioe.2021.690747
Wu, Q., Chu, M., Shao, Y., Wo, F., and Shi, D. (2016). Reduced graphene oxide conjugated with cuins 2/zns nanocrystals with low toxicity for enhanced photothermal and photodynamic cancer therapies. Carbon N. Y. 108, 21–37. doi:10.1016/j.carbon.2016.06.070
Xia, P., Yuan, H., Tian, M., Zhong, T., Hou, R., Xu, X., et al. (2023). Surface-engineered extracellular vesicles with cdh17 nanobodies to efficiently deliver imaging probes and chemo-photothermal drugs for gastric cancer theragnostic. Adv. Funct. Mater 33. doi:10.1002/adfm.202209393
Xie, X., Yu, W., Wang, L., Yang, J., Tu, X., Liu, X., et al. (2024). Sers-based ai diagnosis of lung and gastric cancer via exhaled breath. Spectrochim. Acta a Mol. Biomol. Spectrosc. 314, 124181. doi:10.1016/j.saa.2024.124181
Xie, Z., Fan, T., An, J., Choi, W., Duo, Y., Ge, Y., et al. (2020). Emerging combination strategies with phototherapy in cancer nanomedicine. Chem. Soc. Rev. 49, 8065–8087. doi:10.1039/d0cs00215a
Xin, J., Fu, L., Wang, J., Wang, S., Zhang, L., Zhang, Z., et al. (2022). Influence of parameters on the death pathway of gastric cells induced by gold nanosphere mediated phototherapy. Nanomater. (Basel) 12, 646. doi:10.3390/nano12040646
Xiong, X., Xu, Z., Huang, H., Wang, Y., Zhao, J., Guo, X., et al. (2020). A nir light triggered disintegratable nanoplatform for enhanced penetration and chemotherapy in deep tumor tissues. Biomaterials 245, 119840. doi:10.1016/j.biomaterials.2020.119840
Xu, R., Zhang, G., Mai, J., Deng, X., Segura-Ibarra, V., Wu, S., et al. (2016). An injectable nanoparticle generator enhances delivery of cancer therapeutics. Nat. Biotechnol. 34, 414–418. doi:10.1038/nbt.3506
Xuan, Y., Yang, X., Song, Z., Zhang, R., Zhao, D., Hou, X., et al. (2019). High-security multifunctional nano-bismuth-sphere-cluster prepared from oral gastric drug for ct/pa dual-mode imaging and chemo-photothermal combined therapy in vivo. Adv. Funct. Mater 29. doi:10.1002/adfm.201900017
Yaghoubi, F., Naghib, S. M., Motlagh, N. S. H., Haghiralsadat, F., Jaliani, H. Z., Tofighi, D., et al. (2021). Multiresponsive carboxylated graphene oxide-grafted aptamer as a multifunctional nanocarrier for targeted delivery of chemotherapeutics and bioactive compounds in cancer therapy. Nanotechnol. Rev. 10, 1838–1852. doi:10.1515/ntrev-2021-0110
Yan, Z., Wang, M., Shi, M., He, Y., Zhang, Y., Qiu, S., et al. (2020). Amphiphilic bodipy dye aggregates in polymeric micelles for wavelength-dependent photo-induced cancer therapy. J. Mater Chem. B 8, 6886–6897. doi:10.1039/d0tb00609b
Yang, J., Teng, Y., Fu, Y., and Zhang, C. (2019). Chlorins e6 loaded silica nanoparticles coated with gastric cancer cell membrane for tumor specific photodynamic therapy of gastric cancer. Int. J. Nanomedicine 14, 5061–5071. doi:10.2147/IJN.S202910
Yang, L., Du, Y., Liao, F., Huang, T., Liu, Y., Liu, J., et al. (2024). A novel combined therapeutic strategy of nano-en-ir@lip mediated photothermal therapy and stem cell inhibition for gastric cancer. Biomed. Pharmacother. 174, 116486. doi:10.1016/j.biopha.2024.116486
Yang, Z., Wang, D., Zhang, C., Liu, H., Hao, M., Kan, S., et al. (2022). The applications of gold nanoparticles in the diagnosis and treatment of gastrointestinal cancer. Front. Oncol. 11, 819329. doi:10.3389/fonc.2021.819329
Yao, Y., Zhou, Y., Liu, L., Xu, Y., Chen, Q., Wang, Y., et al. (2020). Nanoparticle-based drug delivery in cancer therapy and its role in overcoming drug resistance. Front. Mol. Biosci. 7, 193. doi:10.3389/fmolb.2020.00193
Yin, J., Xin, B., Zhang, M., Hui, X., Chai, N., Hu, H., et al. (2021). 68ga-labeled gx1 dimer: a novel probe for pet/cerenkov imaging targeting gastric cancer. Front. Oncol. 11, 750376. doi:10.3389/fonc.2021.750376
Yu, Z., Cao, W., Han, C., Wang, Z., Qiu, Y., Wang, J., et al. (2022). Biomimetic metal-organic framework nanoparticles for synergistic combining of sdt-chemotherapy induce pyroptosis in gastric cancer. Front. Bioeng. Biotechnol. 10, 796820. doi:10.3389/fbioe.2022.796820
Zhai, J., Li, X., Zhang, J., Pan, H., Peng, Q., Gan, H., et al. (2022). Sers/electrochemical dual-mode biosensor based on multi-functionalized molybdenum disulfide nanosheet probes and sers-active ag nanorods array electrodes for reliable detection of cancer-related mirna. Sens. Actuators B Chem. 368, 132245. doi:10.1016/j.snb.2022.132245
Zhang, A., Liu, Q., Huang, Z., Zhang, Q., Wang, R., and Cui, D. (2022a). Electrochemical cytosensor based on a gold nanostar-decorated graphene oxide platform for gastric cancer cell detection. Sensors (Basel) 22, 2783. doi:10.3390/s22072783
Zhang, C., Fu, S., Zhang, F., Han, M., Wang, X., Du, J., et al. (2022b). Affibody modified g-quadruplex dna micelles incorporating polymeric 5-fluorodeoxyuridine for targeted delivery of curcumin to enhance synergetic therapy of her2 positive gastric cancer. Nanomater. (Basel) 12, 696. doi:10.3390/nano12040696
Zhang, C., Zhou, Z., Qian, Q., Gao, G., Li, C., Feng, L., et al. (2013). Glutathione-capped fluorescent gold nanoclusters for dual-modal fluorescence/x-ray computed tomography imaging. J. Mater Chem. B 1, 5045–5053. doi:10.1039/c3tb20784f
Zhang, D., Wang, H., Chen, C., Lu, G., Yin, Y., Ren, M., et al. (2025). Preparation and identification of a fluorescent probe with cspbbr 3 perovskite quantum dots and cd44v6 specific peptide for gastric cancer imaging. Nanotechnology 36, 02LT02. doi:10.1088/1361-6528/ad86c7
Zhang, L., Li, X., Yue, G., Guo, L., Hu, Y., Cui, Q., et al. (2023a). Nanodrugs systems for therapy and diagnosis of esophageal cancer. Front. Bioeng. Biotechnol. 11, 1233476. doi:10.3389/fbioe.2023.1233476
Zhang, Y., Zhang, Di, An, S., Liu, Q., Liang, C., Li, J., et al. (2023b). Development and characterization of nanobody-derived cd47 theranostic pairs in solid tumors. Research 6, 0077. doi:10.34133/research.0077
Zhang, Z., Yang, W., Ma, F., Ma, Q., Zhang, B., Zhang, Y., et al. (2020). Enhancing the chemotherapy effect of apatinib on gastric cancer by co-treating with salidroside to reprogram the tumor hypoxia micro-environment and induce cell apoptosis. Drug Deliv. 27, 691–702. doi:10.1080/10717544.2020.1754528
Zhang, Z., Zhang, X., Ren, Z., Wu, X., Qiao, H., Huang, X., et al. (2023c). Biomimetic black phosphorus nanosheets codeliver cdk9 and brd4 inhibitors for gastric cancer targeted therapy. J. Mater Chem. B 11, 6131–6140. doi:10.1039/d3tb00046j
Zheng, H., Chen, Z., Cai, A., Lin, X., Jiang, X., Zhou, B., et al. (2020a). Nanoparticle mediated codelivery of nifuratel and doxorubicin for synergistic anticancer therapy through stat3 inhibition. Colloids Surf. B Biointerfaces 193, 111109. doi:10.1016/j.colsurfb.2020.111109
Zheng, H., Chen, Z., Cai, A., Lin, X., Jiang, X., Zhou, B., et al. (2020b). Nanoparticle mediated codelivery of nifuratel and doxorubicin for synergistic anticancer therapy through stat3 inhibition. Colloids Surf. B Biointerfaces 193, 111109. doi:10.1016/j.colsurfb.2020.111109
Zhou, Q., Cai, X., Huang, Y., and Zhou, Y. (2023). Pluronic f127-liposome-encapsulated curcumin activates nrf2/keap1 signaling pathway to promote cell migration of hacat cells. Mol. Cell Biochem. 478, 241–247. doi:10.1007/s11010-022-04481-6
Zhu, T., Liang, D., Zhang, Q., Sun, W., and Shen, X. (2024a). Curcumin-encapsulated fish gelatin-based microparticles from microfluidic electrospray for postoperative gastric cancer treatment. Int. J. Biol. Macromol. 254, 127763. doi:10.1016/j.ijbiomac.2023.127763
Zhu, X., Su, T., Wang, S., Zhou, H., and Shi, W. (2022). New advances in nano-drug delivery systems: helicobacter pylori and gastric cancer. Front. Oncol. 12, 834934. doi:10.3389/fonc.2022.834934
Keywords: gastric cancer, nanomedicine, nanomaterials, nano drug delivery systems, diagnosis and treatment
Citation: Wang S, Li J, Zhang Z, Cao S, Zhang Z, Bian Y, Xu Y and Ma C (2025) Advances in nanomedicine and delivery systems for gastric cancer research. Front. Bioeng. Biotechnol. 13:1565999. doi: 10.3389/fbioe.2025.1565999
Received: 24 January 2025; Accepted: 05 March 2025;
Published: 21 March 2025.
Edited by:
Gymama Slaughter, Old Dominion University, United StatesCopyright © 2025 Wang, Li, Zhang, Cao, Zhang, Bian, Xu and Ma. This is an open-access article distributed under the terms of the Creative Commons Attribution License (CC BY). The use, distribution or reproduction in other forums is permitted, provided the original author(s) and the copyright owner(s) are credited and that the original publication in this journal is cited, in accordance with accepted academic practice. No use, distribution or reproduction is permitted which does not comply with these terms.
*Correspondence: Chunzheng Ma, bWN6aG5zenl5NjY2QDEyNi5jb20=
†These authors have contributed equally to this work
Disclaimer: All claims expressed in this article are solely those of the authors and do not necessarily represent those of their affiliated organizations, or those of the publisher, the editors and the reviewers. Any product that may be evaluated in this article or claim that may be made by its manufacturer is not guaranteed or endorsed by the publisher.
Research integrity at Frontiers
Learn more about the work of our research integrity team to safeguard the quality of each article we publish.