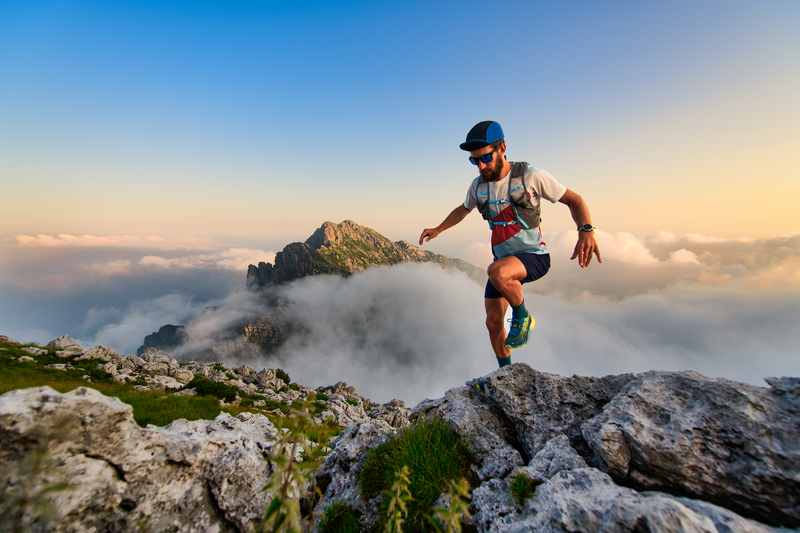
95% of researchers rate our articles as excellent or good
Learn more about the work of our research integrity team to safeguard the quality of each article we publish.
Find out more
MINI REVIEW article
Front. Bioeng. Biotechnol. , 12 March 2025
Sec. Nanobiotechnology
Volume 13 - 2025 | https://doi.org/10.3389/fbioe.2025.1563701
This article is part of the Research Topic Advances in Nanomedicine: Revolutionizing Healthcare with Nanoscale Innovations View all 4 articles
Mitochondria play a significant role in several cellular activities and their function in health and disease has become an important area of research. Since the brain is a high-energy-demanding organ, it is particularly vulnerable to mitochondrial dysfunction. This has been implicated in several brain disorders including neurodegenerative, psychiatric and neurological disorders, e.g., Parkinson’s disease and schizophrenia. Significant efforts are underway to develop mitochondria-targeting pharmaceutical interventions. However, the complex mitochondrial membrane network restricts the entry of therapeutic compounds into the mitochondrial matrix. Nanoparticles (NPs) present a novel solution to this limitation, while also increasing the stability of the therapeutic moieties and improving their bioavailability. This article provides a detailed overview of studies that have investigated the treatment of mitochondrial dysfunction in brain disorders using either targeted or non-targeted NPs as drug delivery systems. All the NPs showed improved mitochondrial functioning including a reduction in reactive oxygen species (ROS) production, an improvement in overall mitochondrial respiration and a reversal of toxin-induced mitochondrial damage. However, the mitochondrial-targeted NPs showed an advantage over the non-targeted NPs as they were able to improve or rescue mitochondrial dynamics and biogenesis, and they required a lower concentration of the in vivo therapeutic dosage of the drug load to show an effect. Consequently, mitochondria-targeted NPs are a promising therapeutic approach. Future studies should exploit advances in nanotechnology, neuroscience and chemistry to design NPs that can cross the blood-brain barrier and selectively target dysfunctional mitochondria, to improve treatment outcomes.
Mitochondrial function is important for numerous cellular activities, including β-oxidation of fatty acids, modification of phospholipids, metabolism of amino acids, the urea cycle, reactive oxygen species (ROS) generation, calcium (Ca2+) homeostasis, intracellular signaling pathways, as well as cellular ageing, survival and death (Bagheri-Mohammadi et al., 2023). Disruption of these cellular activities can impair mitochondrial function and contribute to diseases like Parkinson’s, Alzheimer’s, Huntington’s, schizophrenia, and ischemic stroke (Alqahtani et al., 2023; Sultana et al., 2023). While the term “mitochondrial dysfunction” is often misinterpreted or misunderstood, it can be defined as i) the inability of mitochondria to sustain adenosine triphosphate (ATP) production that is sufficient at supporting cellular demands, or ii) the failure of mitochondria to adapt sufficiently to pathologic conditions (Maarman, 2022). Mitochondrial dysfunction is frequently considered to involve collective changes in the electron transfer system (ETS), ROS production, antioxidant enzyme activity, mitochondrial gene/protein expression, mitochondrial membrane potential, and/or fission and fusion rates (Maarman, 2022).
Based on the importance of functional mitochondria and its vulnerability to dysfunction, significant efforts are underway to develop mitochondria-targeting pharmaceutical interventions. However, directly targeting therapeutics to mitochondria is challenging. One of the main barriers to molecules intracellularly reaching mitochondria is its complex membrane network. While therapeutic moieties are capable of passive diffusion through the outer mitochondrial membrane, phospholipid cardiolipin and the highly negative membrane potential make it nearly impossible for therapeutics to pass through the other mitochondrial membranes (Abdel-Mageed et al., 2021). Other disadvantages limiting the effect of some drugs include their poor stability and bioavailability, low solubility, and non-selective biodistribution.
Nanoparticles (NPs) present a novel solution to overcome several of these challenges by improving the bioavailability and enhancing the efficacy of therapeutic moieties (Abdel-Mageed et al., 2021). Notably, while many diverse types of NPs exist, all can be designed to target organelles including mitochondria, thereby facilitating precise disease treatment. In this mini-review, we discuss features of mitochondrial dysfunction in brain disorders and provide a detailed overview of studies that have investigated the treatment of mitochondrial dysfunction using targeted and non-targeted NPs as a drug delivery system. The goal of this mini-review is to highlight the potential of mitochondria-targeted NPs for the treatment of brain disorders, while emphasizing that several knowledge gaps need to be explored before the field is ready for translational medicine applications.
The regulation and maintenance of cellular metabolism are a critical challenge for the nervous system. Due to the highly complex morphology of neurons, their regulated transmembrane ion gradients and the constant activity of synapses, cellular metabolism of performing and maintaining neural functions is excessively high (Rangaraju et al., 2019). With the human brain being one of the most energy-demanding organs, accounting for 20% of the body’s total energy expenditure (Rangaraju et al., 2019), it is to be expected that mitochondrial dysfunction is implicated in the pathogenesis of several brain disorders (Clemente-Suárez et al., 2023).
The exact roles that mitochondria play in these disorders varies, however, evidence suggests that the progression of brain diseases and mitochondria dysfunction have an interchanging relationship. For example, mitochondrial DNA mutations that disrupt normal cellular function have been implicated in neurodegenerative disease development (Clemente-Suárez et al., 2023). Other examples include neuronal damage and cell death caused by abnormal mitochondrial dynamics, reduced neuronal viability caused by Ca2+ dysregulation, and neuronal damage and neurodegeneration because of excessive ROS production and redox homeostasis imbalance (Clemente-Suárez et al., 2023).
Other cases where brain disorders can exhibit mitochondrial dysfunction include inflammatory mediator-induced mitochondrial dysfunction resulting from the production of nitric oxide (NO) and ROS causing damage to mitochondrial lipids, proteins, and DNA (Clemente-Suárez et al., 2023; Missiroli et al., 2020). Moreover, inflammatory signaling pathways can activate pro-apoptotic proteins, leading to mitochondrial outer membrane permeabilization and subsequent release of cytochrome c which further propagates the inflammatory response (Clemente-Suárez et al., 2023; Teleanu et al., 2022). Alterations in Ca2+ homeostasis due to inflammation can also promote mitochondrial fragmentation via mitochondrial dynamic disruption (Clemente-Suárez et al., 2023). For example, Ca2+ influx into mitochondria can trigger excessive mitochondrial fission, impairing mitochondrial function and energy deficits. Other evidence suggests that mitochondrial dysfunction can influence neurotransmitter metabolism and signaling in psychiatric disorders (Daniels et al., 2020). More specifically, the balance of neurotransmitters can be disrupted through multiple mechanisms, such as impaired oxidative phosphorylation (OXPHOS) and reduced ATP production which affects the synthesis, release and reuptake of neurotransmitters such as serotonin, dopamine, and glutamate (Teleanu et al., 2022).
Given the critical role of mitochondria in brain disorders, many therapeutic strategies have been explored to identify biomarkers and protect and restore mitochondrial function (Clemente-Suárez et al., 2023). Mitochondrial protective agents, such as antioxidants and mitochondrial-targeted compounds, have shown promise in preclinical and clinical studies (Jiang et al., 2020). Moreover, recent advances in nanotechnology have opened possibilities for restoring mitochondrial function in brain diseases. Making use of nanomaterials and nanotechnology as novel therapeutic approaches to protect mitochondrial health can improve treatment strategies in brain disorders. With this approach, knowledge of underlying mechanisms of mitochondrial dysfunction can be explored and possible therapeutics can be developed to repair mitochondrial defects and mitigate disease progression while improving patient outcomes.
Nanoparticles (NPs) have a comprehensive range of applications and are multifunctional depending on their size and surface functionalities (Najahi-Missaoui et al., 2020). NPs typically range between 1 and 100 nm in size, in at least one dimension and are classified into three classes (Najahi-Missaoui et al., 2020) (Supplementary Figure S1). They can be used to achieve a wide range of medical benefits such as prevention and treatment by utilizing them as a drug delivery system (Abdel-Mageed et al., 2021) (Supplementary Material). For therapeutic applications, NPs require specific characterizations, for example,; size determines the interaction between the NP and biological systems (Hoshyar et al., 2016), and surface charge indicates NP stability and absorption into the cell membrane (Abdel-Mageed et al., 2021; Joudeh and Linke, 2022) (Supplementary Material). Drug loading of NPs can be achieved via adsorption/absorption through drug incubation or by chemical conjugation (Singh and Lillard, 2009; Zashikhina et al., 2023) (Supplementary Material). By applying functionalized groups on the surface of NPs, their application in drug delivery can be personalized according to disease-specific needs by targeting specific cells and intracellular compartments, giving them an advantage over current therapeutic moieties (Kumar et al., 2023). Additionally, nanomedicine has the capability of overcoming the disadvantages of therapeutic moieties by improving drug stability, absorption across biological membranes and consequently bioavailability, targeting intracellular components such as the nucleus, lysosomes or mitochondria, enhancing efficacy, and extending half-life, and reducing cytotoxicity (Abuelezz et al., 2020). Moreover, nanomedicines are actively able to co-target therapeutic moieties to the brain parenchyma and brain lesions by crossing the blood-brain barrier (BBB) and entering cells and intracellular compartments to achieve the delivery of multiple cargos with therapeutic, diagnostic, and theranostic properties (Faria et al., 2023).
Consequently, nanomedicines are becoming more accessible in the market and others in various stages of clinical translation (Shan et al., 2022). To date, there are ∼100 nanomedicines on the market and 563 in clinical process or other stages for a variety of diseases (Shan et al., 2022). However, most of these nanomedicines are focused on cancers and infectious disease treatments with very few for brain disorders (Shan et al., 2022).
Globally, neurological conditions are the leading cause of ill health and disability according to data from the World Health Organization (WHO) and the 2021 Global Burden of Disease, Injuries, and Risk Factor Study (GBD) (Faria et al., 2023; Markowicz-Piasecka et al., 2022; Steinmetz et al., 2024). At present, there are several treatment strategies that target mitochondrial dysfunction in brain disorders including pharmacological treatments, mitotherapy (transplantation/transfer of mitochondria), photobiomodulation and gene therapy (Alshial et al., 2023). While there has been significant effort in the field of mitochondrial medicine, the development of mitochondria-targeted NP therapeutics for brain disorders is hindered. This is due to several translational challenges such as the genetic complexity of brain disorders and of the mitochondrion itself, as well as the heterogeneous clinical presentations of patients (Alshial et al., 2023). Currently, there are four Food and Drug Administration (FDA)-approved nanocrystals for the treatment of schizophrenia (Jia et al., 2023), however, no mitochondria-targeting specific NPs are on the market or in clinical translation. Factors which hinder the clinical application of NPs include the enhanced permeability and retention discrepancies between animal models and humans, unclear in vivo fate, and NP toxicity (Jia et al., 2023). The substantial properties of NPs in not only improving the delivery of biopharmaceuticals (Jia et al., 2023), but also its ability to personalize disease treatment with surface modifiers has made them an attractive therapeutic option for the treatment of brain disorders.
To identify and summarize published work in the area concerning NPs and mitochondrial dysfunction in brain disorders to date, we conducted a literature search of the PubMed database (on 12 December 2023). The following search terms were used: “nanoparticles” AND “mitochondrial” AND “dysfunction” AND “brain” AND “disorders”, and 80 articles were retrieved. After all review articles, book chapters, and other nonrelevant literature were removed and a date selection of the past decade (2013–2023) was applied, 52 articles were returned. These articles were further filtered by removing all articles that did not focus on the terms: “mitochondria dysfunction” or “brain disorders”. After all filters were applied, 36 original research articles were identified and summarized into two tables (Tables 1, 2). More detailed versions of these tables, which include NP characterization and properties of the loaded drug, are provided in Supplementary Tables S1, S2, respectively.
Table 1. In vitro and in vivo studies of non-targeted nanoparticles and their effect on mitochondrial functioning.
Table 2. In vitro and in vivo studies of mitochondria-targeted nanoparticles and their effect on mitochondrial functioning.
Of the 36 research articles, 27 used NPs that did not target mitochondria and are summarized in Table 1 with a more detailed version in Supplementary Table S1. In the non-targeted NP studies, a wide variety of NP types were used. The choice of the type of NP intended for use in drug delivery systems is based on the physiochemical properties, characteristics, benefits and applications of the NP type. These include size, shape, surface functionalization, core stability, biocompatibility, release kinetics, targeting, and accumulation (Yusuf et al., 2023).
Across the studies, a common improvement was reported in; i) mitochondrial respiration particularly in basal respiration, complex I activity, ATP levels, and leak respiration, and ii) the reduction in ROS production (5, 18, 20, 23, 26 in Table 1; Supplementary Table S1). An effect on i) the reduction in superoxide and hydrogen peroxide levels indicating a lowering of ROS production, ii) the improvement in ATP production, PGC1α, NRF-1 and Tfam mRNA levels, thereby indicating an improvement in OXPHOS and mitochondrial biogenesis, and iii) the normalization of the mitochondrial membrane potential and cytochrome c oxidase activity was also reported (6, 12, 13, 15, 16, 19, 21, and 24 in Table 1; Supplementary Table S1). Lastly, i) an improvement in complex II activity, mitochondrial membrane potential and ATP levels, ii) a reduction in brain mitochondrial ROS production and cytochrome c release, iii) an improvement in basal respiration, superoxide dismutase (SOD) activity, and catalase activity, indicating a decrease in ROS production (Saxena et al., 2023), iv) a reduction in proton leak and caspase-3 protein expression, indicating a lowering of apoptotic activation and prevention of cell atrophy (Brentnall et al., 2013), and v) the restoration of the response to injury-induced glutamate signaling, implying an improvement of OXPHOS and glycolysis (Hillen and Heine, 2020) (9, 11, 14, 17–20, 22, 23 in Table 1; Supplementary Table S1), was observed.
Although the function of the NPs in these studies was not to specifically target mitochondria, they all proved to affect mitochondria functioning. The two most common drugs used in these studies were curcumin (1, 3, 8, 9, and 20 in Table 1) and resveratrol (7 and 10 in Table 1). Curcumin and resveratrol are strong antioxidants, and this property is evident with the NPs loaded with these therapeutic moieties showing a marked improvement in antioxidant functioning and reduction in ROS production.
The concentration of the therapeutic moieties loaded in/on the NPs, however, should be noted. The therapeutic dosage of the two common drugs in Table 1, specifically in vivo studies, ranges from 5–50 mg/kg body weight and 10–40 mg/kg body weight for curcumin and resveratrol, respectively. These values far exceed the Allowable Daily Intake (ADI) of both drugs, which could lead to cellular toxicity. According to the European Food Safety Authority (EFSA) reports, the ADI of curcumin is 0–3 mg/kg body weight (Hewlings and Kalman, 2017) and the ADI of resveratrol is 7.5 mg/kg body weight (Louis Bresson et al., 2016).
The mitochondrial membrane complex structure and its highly negative membrane potential inhibit the entry of anionic drugs and molecules into the mitochondrial matrix (Tabish and Hamblin, 2021). Non-targeted NPs enter the cell via the endocytosis process through the direct coating of the NPs with plasma proteins, which allows entry of the NPs through the plasma membrane (Oh and Park, 2014). However, due to the plasma membrane being selectively permeable, entry of the NPs into the cells is size, shape and surface chemistry dependent, which ultimately determines their systemic elimination and toxicity (Oh and Park, 2014). Consequently, the need to design and develop target-specific NPs is of growing interest. Of the 36 research articles, nine used mitochondria-targeted NPs and are summarized in Table 2 and Supplementary Table S2.
There are currently two approaches to achieve mitochondrial targeting of NPs: active and passive targeting. Active targeting involves the surface functionalization of NPs with lipophilic cations (mitochondrial-specific ligands), while passive targeting involves the tuning of the NP characteristics to improve their specificity (Tabish and Hamblin, 2021). There are a variety of mitochondrial-specific moieties that can be attached to either drug-loaded or unloaded NPs. These ligands include triphenylphosphonium (TPP+), tetramethylrhodamine-5-isothiocyanate, mitochondrial peptides, dequalinium, and natural products (such as hypericin and glycyrrhetinic), to name a few (Tabish and Hamblin, 2021). TPP+ is a widely studied mitochondrial-targeting ligand used in the preparation of non-toxic NPs. The conjugation of TPP+ to NPs increases the lipophilicity and enables the NPs to freely cross the mitochondrial membrane bilayers (Tabish and Hamblin, 2021).
The common effects of in vivo studies include; i) the normalization of superoxide levels, SOD and GSH activity, imbalance of MFN1 and DRP1, and the mitochondrial membrane potential and mitochondrial morphology, indicating the normalization of OXPHOS and ROS levels as well as mitochondrial dynamic balance, ii) reduction in ROS production and MDA levels, and iii) the improvement of PGC-1α levels, SOD activity, and ATP production, suggesting an increase in mitochondrial biogenesis, ROS reduction and OXPHOS (2, 5 and 7 in Table 2; Supplementary Table S2). Other common effects in vivo and in vitro are the reduction in ROS, MDA and SOD activity, and cell apoptosis (3 and 4 in Table 2; Supplementary Table S2). The common effects of the study which aimed to induce glioma cell death include the induction of mitochondrial membrane depolarization, ROS production, caspase-3 overexpression and apoptosis in vitro. These results indicate the successful abrogation of glioblastoma proliferation through the activation of mitochondrial destruction, thereby highlighting the dual property of organelle-targeted NPs (6 in Table 2; Supplementary Table S2). The other effects included apoptotic rescue and the improvement in mitochondrial membrane potential (8 in Table 2; Supplementary Table S2).
As with the studies in Table 1, the most common drug used in these studies was curcumin (3 and 9 in Table 2). However, in contrast to the in vivo therapeutic dosage of curcumin seen in Table 1. (5–50 mg/kg body weight), the concentrations of curcumin in vivo in Table 2, were much lower (2 and 5 mg/kg body weight). While this concentration range is still higher than the ADI of curcumin (0–3 mg/kg body weight (Hewlings and Kalman, 2017), the dosage is significantly less than those used in non-targeted NPs. This is important as it highlights that targeted NPs decrease the therapeutic threshold dosage, meaning that lower therapeutic concentrations are needed to provide optimal treatment outcomes while minimizing side effects (Tian et al., 2022).
Overall, the results of the mitochondrial-targeted NPs demonstrated a direct effect of the NPs on mitochondrial functioning as shown by the recovery/improvement of mitochondrial morphology, and structure as well as crucial proteins involved in mitochondrial maintenance. It is important to note that, unlike the untargeted NPs, mitochondrial-targeted NPs were able to improve or rescue mitochondrial dynamics and biogenesis. The predominant effects of mitochondria-targeted NPs were the recovery of mitochondria cristae, mitochondrial membrane potential, and the overall morphology of mitochondria, the reduction of mitochondrial ROS production, MDA and superoxide levels, and cell apoptosis, as well as the improvement in PGC-1α, SIRT1, and TFAM levels, all of which are necessary for proper mitochondrial functioning.
Mitochondria-targeted NPs hold potential for the treatment of various brain disorders, with an illustrated summary of the potential effects mitochondria-targeted NPs have on mitochondrial functioning shown in Supplementary Figure S2. However, several knowledge gaps in this area of research need to be addressed before advancements in the field can be made. While there is evidence to support the implication of mitochondrial dysfunction in brain disorders (as shown in Tables 1, 2), the specific mechanisms that lead to mitochondrial dysfunction need to be further elucidated. Consequently, more research is needed to understand exactly how the ETS of mitochondria and other aspects of mitochondrial function are involved in pathogenesis. This could be done using high-resolution respirometry (HRR), specifically the Oroboros Oxygraph (O2k), to measure mitochondrial respiratory control relative to specific tissue/cell types across various metabolic states (Walsh et al., 2023). Additionally, the design of NPs to specifically cross the BBB and accumulate in mitochondria of brain cells need to be further optimized and developed to produce an efficient drug delivery system. Achieving specificity and selectivity for mitochondria is necessary to avoid off-target effects and potential toxicity. Therefore, a crucial aspect of target-specific NPs is to design NPs that selectively target dysfunctional mitochondria in diseased cells while avoiding healthy mitochondria. Furthermore, while there is promising evidence of NPs from preclinical studies, translating the NP’s effectiveness to clinical settings requires more evaluation of their safety and efficacy in vivo and ultimately in human trials. Moreover, additional studies on the long-term effects and stability of NPs need to be performed to assess the feasibility of mitochondria-targeted NPs as therapeutic agents. Factors such as NP degradation, potential accumulation in non-target tissues, and immune response need to be investigated thoroughly.
To address these knowledge gaps and drive the field forward, future research should focus on collaborative interdisciplinary research involving researchers from different fields to develop novel therapeutic mitochondria-targeted NPs. Conducting well-designed clinical trials to evaluate the safety, tolerability, and efficacy of mitochondria-targeted NPs in patients with various brain disorders, while focusing on patient stratification and personalised medicine approaches, are an essential part of the process. By addressing these and other challenges, while exploiting advances in nanotechnology, neuroscience, and medicine, the field of mitochondria-targeted NPs holds great potential for transforming brain disorder treatment and improving patient outcomes in the future.
All the NPs that were tested in the reviewed studies showed improved mitochondrial functioning. These features included a reduction in ROS production, an improvement in overall mitochondrial respiration and a reversal of toxin-induced mitochondrial damage. However, one of the major limitations to these studies was the use of the ROS assay to measure mitochondrial function and dysfunction in vivo. While ROS assays are useful for understanding how ROS levels impact health and disease, the use of HRR, such as the Oroboros O2k, can help identify the exact cause of the mitochondrial dysfunction by measuring different components of mitochondrial bioenergetics. This does not only aid in the identification of the underlying mechanisms that cause mitochondrial dysfunction, but may assist with developing more effective treatment modalities. Although the field of nanomedicine shows promise, several technical challenges, as stated above, need to be addressed before this technology can be used to treat patients.
In conclusion, the improvement and refinement of nanotechnologies may play a critical role in the way mitochondrial dysfunction in brain disorders is successfully treated in future, by delivering drugs and other agents directly to the site of the disease. This should lead to less toxicity, better adherence and ultimately improved treatment outcomes.
AB: Conceptualization, Investigation, Resources, Visualization, Writing–original draft, Writing–review and editing. GM: Project administration, Supervision, Writing–review and editing, Conceptualization, Resources. AD: Project administration, Supervision, Writing–review and editing, Conceptualization, Resources. SB: Conceptualization, Funding acquisition, Investigation, Project administration, Resources, Supervision, Writing–review and editing.
The author(s) declare that financial support was received for the research, authorship, and/or publication of this article. This work is based on the research supported in part by the National Research Foundation of South Africa (grant numbers 129429 (S.B), PMDS22070532523 (A.B) and CSUR23042095435) and by the South African Medical Research Council (Self-Initiated Research Grant).
We also acknowledge the support of the Centre for Tuberculosis Research (CTR) of the South African Medical Research Council (SAMRC). All figures in the document were created using BioRender.com.
The authors declare that the research was conducted in the absence of any commercial or financial relationships that could be construed as a potential conflict of interest.
The author(s) declare that no Generative AI was used in the creation of this manuscript.
All claims expressed in this article are solely those of the authors and do not necessarily represent those of their affiliated organizations, or those of the publisher, the editors and the reviewers. Any product that may be evaluated in this article, or claim that may be made by its manufacturer, is not guaranteed or endorsed by the publisher.
The Supplementary Material for this article can be found online at: https://www.frontiersin.org/articles/10.3389/fbioe.2025.1563701/full#supplementary-material
Abdel-Mageed, H. M., AbuelEzz, N. Z., Radwan, R. A., and Mohamed, S. A. (2021). Nanoparticles in nanomedicine: a comprehensive updated review on current status, challenges and emerging opportunities. J. Microencapsul. 38 (6), 414–436. doi:10.1080/02652048.2021.1942275
Abuelezz, N. Z., Shabana, M. E., Abdel-Mageed, H. M., Rashed, L., and Morcos, G. N. B. (2020). Nanocurcumin alleviates insulin resistance and pancreatic deficits in polycystic ovary syndrome rats: insights on PI3K/AkT/mTOR and TNF-α modulations. Life Sci. 256, 118003. doi:10.1016/j.lfs.2020.118003
Alqahtani, T., Deore, S. L., Kide, A. A., Shende, B. A., Sharma, R., Dadarao Chakole, R., et al. (2023). Mitochondrial dysfunction and oxidative stress in Alzheimer’s disease, and Parkinson’s disease, Huntington’s disease and Amyotrophic Lateral Sclerosis -An updated review. Mitochondrion 71, 83–92. doi:10.1016/j.mito.2023.05.007
Alshial, E. E., Abdulghaney, M. I., Wadan, A. H. S., Abdellatif, M. A., Ramadan, N. E., Suleiman, A. M., et al. (2023). Mitochondrial dysfunction and neurological disorders: a narrative review and treatment overview. Life Sci. 334, 122257. doi:10.1016/J.LFS.2023.122257
Babylon, L., Grewal, R., Stahr, P.-L., Eckert, R. W., Keck, C. M., and Eckert, G. P. (2021). Hesperetin nanocrystals improve mitochondrial function in a cell model of early alzheimer disease. Antioxidants 10 (7), 1003. doi:10.3390/antiox10071003
Bagheri-Mohammadi, S., Farjami, M., Suha, A. J., Zarch, S. M. A., Najafi, S., and Esmaeili, A. (2023). The mitochondrial calcium signaling, regulation, and cellular functions: a novel target for therapeutic medicine in neurological disorders. J. Cell. Biochem. 124 (5), 635–655. doi:10.1002/jcb.30414
Bailey, Z. S., Nilson, E., Bates, J. A., Oyalowo, A., Hockey, K. S., Sajja, V. S. S. S., et al. (2020). Cerium oxide nanoparticles improve outcome after in vitro and in vivo mild traumatic brain injury. J. Neurotrauma 37 (12), 1452–1462. doi:10.1089/neu.2016.4644
Battaglini, M., Carmignani, A., Martinelli, C., Colica, J., Marino, A., Doccini, S., et al. (2022). In vitro study of polydopamine nanoparticles as protective antioxidant agents in fibroblasts derived from ARSACS patients. Biomaterials Sci. 10 (14), 3770–3792. doi:10.1039/d2bm00729k
Brentnall, M., Rodriguez-Menocal, L., De Guevara, R. L., Cepero, E., and Boise, L. H. (2013). Caspase-9, caspase-3 and caspase-7 have distinct roles during intrinsic apoptosis. BMC Cell Biol. 14 (1), 32–39. doi:10.1186/1471-2121-14-32
Chiang, M.-C., and Nicol, C. J. B. (2022). GSH-AuNP anti-oxidative stress, ER stress and mitochondrial dysfunction in amyloid-beta peptide-treated human neural stem cells. Free Radic. Biol. & Med. 187, 185–201. doi:10.1016/j.freeradbiomed.2022.05.025
Chiang, M.-C., Nicol, C. J. B., Cheng, Y.-C., Yen, C., Lin, C.-H., Chen, S.-J., et al. (2020). Nanogold neuroprotection in human neural stem cells against amyloid-beta-induced mitochondrial dysfunction. Neuroscience 435, 44–57. doi:10.1016/j.neuroscience.2020.03.040
Choi, S. G., Shin, J., Lee, K. Y., Park, H., Kim, S. I., Yi, Y. Y., et al. (2023). PINK1 siRNA-loaded poly(lactic-co-glycolic acid) nanoparticles provide neuroprotection in a mouse model of photothrombosis-induced ischemic stroke. Glia 71 (5), 1294–1310. doi:10.1002/glia.24339
Clemente-Suárez, V. J., Redondo-Flórez, L., Beltrán-Velasco, A. I., Ramos-Campo, D. J., Belinchón-deMiguel, P., Martinez-Guardado, I., et al. (2023). Mitochondria and brain disease: a comprehensive review of pathological mechanisms and therapeutic opportunities. Biomedicines 11 (9), 2488. doi:10.3390/BIOMEDICINES11092488
Daniels, T. E., Olsen, E. M., and Tyrka, A. R. (2020). Stress and psychiatric disorders: the role of mitochondria. Annu. Rev. Clin. Psychol. 16, 165–186. doi:10.1146/ANNUREV-CLINPSY-082719-104030
dos Santos Tramontin, N., da Silva, S., Arruda, R., Ugioni, K. S., Canteiro, P. B., de Bem Silveira, G., et al. (2020). Gold nanoparticles treatment reverses brain damage in Alzheimer’s disease model. Mol. Neurobiol. 57 (2), 926–936. doi:10.1007/s12035-019-01780-w
Faria, P., Pacheco, C., Moura, P., Sarmento, B., and Martins, C. (2023). Multifunctional nanomedicine strategies to manage brain diseases. Drug Deliv. Transl. Res. 13, 1322–1342. doi:10.1007/s13346-022-01256-w
Gao, C., Wang, Y., Sun, J., Han, Y., Gong, W., Li, Y., et al. (2020). Neuronal mitochondria-targeted delivery of curcumin by biomimetic engineered nanosystems in Alzheimer’s disease mice. Acta Biomater. 108, 285–299. doi:10.1016/j.actbio.2020.03.029
Hammerschmidt, T. G., Donida, B., Raabe, M., Faverzani, J. L., de Fátima Lopes, F., Machado, A. Z., et al. (2023). Evidence of redox imbalance and mitochondrial dysfunction in Niemann-Pick type C 1 patients: the in vitro effect of combined therapy with antioxidants and β-cyclodextrin nanoparticles. Metab. Brain Dis. 38 (2), 507–518. doi:10.1007/s11011-022-01128-9
Han, Y., Chu, X., Cui, L., Fu, S., Gao, C., Li, Y., et al. (2020). Neuronal mitochondria-targeted therapy for Alzheimer’s disease by systemic delivery of resveratrol using dual-modified novel biomimetic nanosystems. Drug Deliv. 27 (1), 502–518. doi:10.1080/10717544.2020.1745328
Hao, C., Qu, A., Xu, L., Sun, M., Zhang, H., Xu, C., et al. (2019). Chiral molecule-mediated porous CuxO nanoparticle clusters with antioxidation activity for ameliorating Parkinson’s disease. J. Am. Chem. Soc. 141 (2), 1091–1099. doi:10.1021/jacs.8b11856
Hewlings, S. J., and Kalman, D. S. (2017). Curcumin: a review of its effects on human health. Foods 6 (10), 92. doi:10.3390/FOODS6100092
Hillen, A. E. J., and Heine, V. M. (2020). Glutamate carrier involvement in mitochondrial dysfunctioning in the brain white matter. Front. Mol. Biosci. 7, 151. doi:10.3389/FMOLB.2020.00151
Hoshyar, N., Gray, S., Han, H., and Bao, G. (2016). The effect of nanoparticle size on in vivo pharmacokinetics and cellular interaction. Nanomedicine 11 (6), 673–692. doi:10.2217/NNM.16.5
Jia, Y., Jiang, Y., He, Y., Zhang, W., Zou, J., Magar, K. T., et al. (2023). Approved nanomedicine against diseases. Pharmaceutics 15 (3), 774. doi:10.3390/PHARMACEUTICS15030774
Jia, Z., Yuan, X., Wei, J., Guo, X., Gong, Y., Li, J., et al. (2021). A functionalized octahedral palladium nanozyme as a radical scavenger for ameliorating Alzheimer’s disease. ACS Appl. Mater. & Interfaces 13 (42), 49602–49613. doi:10.1021/acsami.1c06687
Jiang, Q., Yin, J., Chen, J., Ma, X., Wu, M., Liu, G., et al. (2020). Mitochondria-targeted antioxidants: a step towards disease treatment. Oxidative Med. Cell. Longev. 2020, 1–18. doi:10.1155/2020/8837893
Joudeh, N., and Linke, D. (2022). Nanoparticle classification, physicochemical properties, characterization, and applications: a comprehensive review for biologists. J. Nanobiotechnology 20 (1), 262. doi:10.1186/s12951-022-01477-8
Kaundal, B., Karmakar, S., and Roy Choudhury, S. (2022). Mitochondria-targeting nano therapy altering IDH2-mediated EZH2/EZH1 interaction as precise epigenetic regulation in glioblastoma. Biomaterials Sci. 1 (18), 5301–5317. doi:10.1039/d1bm02006d
Kumar, Y., Sinha, A. S. K., Nigam, K. D. P., Dwivedi, D., and Sangwai, J. S. (2023). Functionalized nanoparticles: tailoring properties through surface energetics and coordination chemistry for advanced biomedical applications. Nanoscale 15, 6075–6104. doi:10.1039/d2nr07163k
Kundu, P., Das, M., Tripathy, K., and Sahoo, S. K. (2016). Delivery of dual drug loaded lipid based nanoparticles across the blood-brain barrier impart enhanced neuroprotection in a rotenone induced mouse model of Parkinson’s disease. ACS Chem. Neurosci. 7 (12), 1658–1670. doi:10.1021/ACSCHEMNEURO.6B00207
Kwon, H. J., Cha, M.-Y., Kim, D., Kim, D. K., Soh, M., Shin, K., et al. (2016). Mitochondria-targeting ceria nanoparticles as antioxidants for Alzheimer’s disease. ACS Nano 10 (2), 2860–2870. doi:10.1021/acsnano.5b08045
Li, W., Peng, X., Mei, X., Dong, M., Li, Y., and Dong, H. (2023). Multifunctional DNA tetrahedron for Alzheimer’s disease mitochondria-targeted therapy by MicroRNA regulation. ACS Appl. Mater. & Interfaces 15 (19), 22977–22984. doi:10.1021/acsami.3c03181
Louis Bresson, J., Burlingame, B., Dean, T., Fairweather-Tait, S., Heinonen, M., Ildico Hirsch-Ernst, K., et al. (2016). Safety of synthetic trans-resveratrol as a novel food pursuant to Regulation (EC) No 258/97. EFSA J. 14 (1), 4368. doi:10.2903/J.EFSA.2016.4368
Maarman, G. J. (2022). Reviewing the suitability of mitochondrial transplantation as therapeutic approach for pulmonary hypertension in the era of personalized medicine. Am. J. Physiology. Lung Cell. Mol. Physiology 322 (5), L641–L646. doi:10.1152/ajplung.00484.2021
Markowicz-Piasecka, M., Markiewicz, A., Darłak, P., Sikora, J., Adla, S. K., Bagina, S., et al. (2022). Current chemical, biological, and physiological views in the development of successful brain-targeted pharmaceutics. Neurotherapeutics 19 (3), 942–976. doi:10.1007/S13311-022-01228-5
Missiroli, S., Genovese, I., Perrone, M., Vezzani, B., Vitto, V. A. M., and Giorgi, C. (2020). The role of mitochondria in inflammation: from cancer to neurodegenerative disorders. J. Clin. Med. 9 (3), 740. doi:10.3390/JCM9030740
Mukherjee, A., Sarkar, S., Jana, S., Swarnakar, S., and Das, N. (2019). Neuro-protective role of nanocapsulated curcumin against cerebral ischemia-reperfusion induced oxidative injury. Brain Res. 1704, 164–173. doi:10.1016/j.brainres.2018.10.016
Muller, A. P., Ferreira, G. K., Pires, A. J., de Bem Silveira, G., de Souza, D. L., Brandolfi, J. de A., et al. (2017). Gold nanoparticles prevent cognitive deficits, oxidative stress and inflammation in a rat model of sporadic dementia of Alzheimer’s type. Mater. Sci. & Eng. C 77, 476–483. doi:10.1016/j.msec.2017.03.283
Najahi-Missaoui, W., Arnold, R. D., and Cummings, B. S. (2020). Safe nanoparticles: are we there yet? Int. J. Mol. Sci. 22 (1), 385. doi:10.3390/ijms22010385
Naserzadeh, P., Hafez, A. A., Abdorahim, M., Abdollahifar, M. A., Shabani, R., Peirovi, H., et al. (2018). Curcumin loading potentiates the neuroprotective efficacy of Fe3O4 magnetic nanoparticles in cerebellum cells of schizophrenic rats. Biomed. & Pharmacother. 108, 1244–1252. doi:10.1016/j.biopha.2018.09.106
Oh, N., and Park, J.-H. (2014). Endocytosis and exocytosis of nanoparticles in mammalian cells. Int. J. Nanomedicine 9 (1), 51–63. doi:10.2147/ijn.s26592
Palle, S., and Neerati, P. (2018). Improved neuroprotective effect of resveratrol nanoparticles as evinced by abrogation of rotenone-induced behavioral deficits and oxidative and mitochondrial dysfunctions in rat model of Parkinson’s disease. Naunyn-Schmiedeberg’s Archives Pharmacol. 391 (4), 445–453. doi:10.1007/s00210-018-1474-8
Petronilho, F., Tenfen, L., Della Giustina, A., Joaquim, L., Novochadlo, M., de Oliveira Junior, A. N., et al. (2020). Gold nanoparticles potentiates N-acetylcysteine effects on neurochemicals alterations in rats after polymicrobial sepsis. J. Drug Target. 28 (4), 428–436. doi:10.1080/1061186X.2019.1678168
Qian, K., Bao, X., Li, Y., Wang, P., Guo, Q., Yang, P., et al. (2022). Cholinergic neuron targeting nanosystem delivering hybrid peptide for combinatorial mitochondrial therapy in Alzheimer’s disease. ACS Nano 16 (7), 11455–11472. doi:10.1021/acsnano.2c05795
Ramachandran, S., and Thangarajan, S. (2016). A novel therapeutic application of solid lipid nanoparticles encapsulated thymoquinone (TQ-SLNs) on 3-nitroproponic acid induced Huntington’s disease-like symptoms in wistar rats. Chemico-Biological Interact. 256, 25–36. doi:10.1016/J.CBI.2016.05.020
Ramires Júnior, O. V., Alves, B. da S., Barros, P. A. B., Rodrigues, J. L., Ferreira, S. P., Monteiro, L. K. S., et al. (2021). Nanoemulsion improves the neuroprotective effects of curcumin in an experimental model of Parkinson’s disease. Neurotox. Res. 39 (3), 787–799. doi:10.1007/s12640-021-00362-w
Rangaraju, V., Lewis, T. L., Hirabayashi, Y., Bergami, M., Motori, E., Cartoni, R., et al. (2019). Pleiotropic mitochondria: the influence of mitochondria on neuronal development and disease. J. Neurosci. 39 (42), 8200–8208. doi:10.1523/JNEUROSCI.1157-19.2019
Rodrigues, M. S., de Paula, G. C., Duarte, M. B., de Rezende, V. L., Possato, J. C., Farias, H. R., et al. (2021). Nanotechnology as a therapeutic strategy to prevent neuropsychomotor alterations associated with hypercholesterolemia. Colloids Surfaces, B, Biointerfaces 201, 111608. doi:10.1016/j.colsurfb.2021.111608
Samir, M., Abdelkader, R. M., Boushehri, M. S., Mansour, S., Lamprecht, A., and Tammam, S. N. (2023). Enhancement of mitochondrial function using NO releasing nanoparticles; a potential approach for therapy of Alzheimer’s disease. Eur. J. Pharm. Biopharm. 184, 16–24. doi:10.1016/j.ejpb.2023.01.006
Sandhir, R., Yadav, A., Mehrotra, A., Sunkaria, A., Singh, A., and Sharma, S. (2014). Curcumin nanoparticles attenuate neurochemical and neurobehavioral deficits in experimental model of Huntington’s disease. NeuroMolecular Med. 16 (1), 106–118. doi:10.1007/S12017-013-8261-Y
Saxena, A., Lakshmi, J., Bhattacharjya, R., Singh, P. K., Mishra, B., and Tiwari, A. (2023). The role of antioxidant enzymes in diatoms and their therapeutic role. Mar. Antioxidants Prep. Syntheses, Appl., 89–118. doi:10.1016/B978-0-323-95086-2.00019-9
Shan, X., Gong, X., Li, J., Wen, J., Li, Y., and Zhang, Z. (2022). Current approaches of nanomedicines in the market and various stage of clinical translation. Acta Pharm. Sin. B 12 (7), 3028–3048. doi:10.1016/J.APSB.2022.02.025
Sharma, A., Liaw, K., Sharma, R., Zhang, Z., Kannan, S., and Kannan, R. M. (2018). Targeting mitochondrial dysfunction and oxidative stress in activated microglia using dendrimer-based therapeutics. Int. Publ. Theranostics 8 (20), 5529–5547. doi:10.7150/thno.29039
Singh, R., and Lillard, J. W. (2009). Nanoparticle-based targeted drug delivery. Exp. Mol. Pathology 86 (3), 215–223. doi:10.1016/J.YEXMP.2008.12.004
Srivastava, A. K., Yadav, S. S., Mishra, S., Yadav, S. K., Parmar, D., and Yadav, S. (2020). A combined microRNA and proteome profiling to investigate the effect of ZnO nanoparticles on neuronal cells. Nanotoxicology 14 (6), 757–773. doi:10.1080/17435390.2020.1759726
Steinmetz, J. D., Seeher, K. M., Schiess, N., Nichols, E., Cao, B., Servili, C., et al. (2024). Global, regional, and national burden of disorders affecting the nervous system, 1990–2021: a systematic analysis for the Global Burden of Disease Study 2021. Lancet Neurology 23 (4), 344–381. doi:10.1016/S1474-4422(24)00038-3
Sultana, M. A., Hia, R. A., Akinsiku, O., and Hegde, V. (2023). Peripheral mitochondrial dysfunction: a potential contributor to the development of metabolic disorders and Alzheimer’s disease. Biology 12 (Issue 7), 1019. doi:10.3390/biology12071019
Surnar, B., Shah, A. S., Park, M., Kalathil, A. A., Kamran, M. Z., Ramirez Jaime, R., et al. (2021). Brain-accumulating nanoparticles for assisting astrocytes to reduce human immunodeficiency virus and drug abuse-induced neuroinflammation and oxidative stress. ACS Nano 15 (10), 15741–15753. doi:10.1021/acsnano.0c09553
Tabish, T. A., and Hamblin, M. R. (2021). Mitochondria-targeted nanoparticles (mitoNANO): an emerging therapeutic shortcut for cancer. Biomaterials Biosyst. 3, 100023. doi:10.1016/j.bbiosy.2021.100023
Teleanu, R. I., Niculescu, A. G., Roza, E., Vladâcenco, O., Grumezescu, A. M., and Teleanu, D. M. (2022). Neurotransmitters—key factors in neurological and neurodegenerative disorders of the central nervous system. Int. J. Mol. Sci. 23 (11), 5954. doi:10.3390/IJMS23115954
Tian, H., Zhang, T., Qin, S., Huang, Z., Zhou, L., Shi, J., et al. (2022). Enhancing the therapeutic efficacy of nanoparticles for cancer treatment using versatile targeted strategies. J. Hematol. Oncol. 15 (1), 132. doi:10.1186/s13045-022-01320-5
Trapani, A., Guerra, L., Corbo, F., Castellani, S., Sanna, E., Capobianco, L., et al. (2021). Cyto/biocompatibility of dopamine combined with the antioxidant grape seed-derived polyphenol compounds in solid lipid nanoparticles. Mol. Basel, Switz. 26 (4), 916. doi:10.3390/molecules26040916
Walsh, M. A., Musci, R. V., Jacobs, R. A., and Hamilton, K. L. (2023). A practical perspective on how to develop, implement, execute, and reproduce high-resolution respirometry experiments: the physiologist’s guide to an Oroboros O2k. FASEB J. 37 (12), e23280. doi:10.1096/FJ.202301644RR
Wong, L. R., and Ho, P. C. (2017). Role of serum albumin as a nanoparticulate carrier for nose-to-brain delivery of R-flurbiprofen: implications for the treatment of Alzheimer’s disease. J. Pharm. Pharmacol. 70 (1), 59–69. doi:10.1111/JPHP.12836
Xu, Z. R., Wang, W. F., Liang, X. F., Liu, Z. H., Liu, Y., Lin, L., et al. (2015). Protective effects of poly (butyl) cyanoacrylate nanoparticles containing vasoactive intestinal peptide against 6-hydroxydopamine-induced neurotoxicity in vitro. J. Mol. Neurosci. 55 (4), 854–864. doi:10.1007/S12031-014-0438-9
Yadav, A., Sunkaria, A., Singhal, N., and Sandhir, R. (2018). Resveratrol loaded solid lipid nanoparticles attenuate mitochondrial oxidative stress in vascular dementia by activating Nrf2/HO-1 pathway. Neurochem. Int. 112, 239–254. doi:10.1016/j.neuint.2017.08.001
Yusuf, A., Almotairy, A. R. Z., Henidi, H., Alshehri, O. Y., and Aldughaim, M. S. (2023). Nanoparticles as drug delivery systems: a review of the implication of nanoparticles’ physicochemical properties on responses in biological systems. Polymers 15 (7), 1596. doi:10.3390/polym15071596
Zashikhina, N., Gladnev, S., Sharoyko, V., Korzhikov-Vlakh, V., Korzhikova-Vlakh, E., and Tennikova, T. (2023). Synthesis and characterization of nanoparticle-based dexamethasone-polypeptide conjugates as potential intravitreal delivery systems. Int. J. Mol. Sci. 24 (4), 3702. doi:10.3390/ijms24043702
Zhang, L., Zhao, P., Yue, C., Jin, Z., Liu, Q., Du, X., et al. (2019). Sustained release of bioactive hydrogen by Pd hydride nanoparticles overcomes Alzheimer’s disease. Biomaterials 197, 393–404. doi:10.1016/j.biomaterials.2019.01.037
Keywords: brain disorders, mitochondrial dysfunction, nanomedicine, mitochondria-targeted nanoparticles, therapy
Citation: Buck AC, Maarman GJ, Dube A and Bardien S (2025) Mitochondria targeted nanoparticles for the treatment of mitochondrial dysfunction-associated brain disorders. Front. Bioeng. Biotechnol. 13:1563701. doi: 10.3389/fbioe.2025.1563701
Received: 20 January 2025; Accepted: 24 February 2025;
Published: 12 March 2025.
Edited by:
Kexiao Yu, Chongqing Hospital of Traditional Chinese Medicine, ChinaReviewed by:
Chiara Martinelli, Politecnico di Milano, ItalyCopyright © 2025 Buck, Maarman, Dube and Bardien. This is an open-access article distributed under the terms of the Creative Commons Attribution License (CC BY). The use, distribution or reproduction in other forums is permitted, provided the original author(s) and the copyright owner(s) are credited and that the original publication in this journal is cited, in accordance with accepted academic practice. No use, distribution or reproduction is permitted which does not comply with these terms.
*Correspondence: Soraya Bardien, c2JhcmRpZW5Ac3VuLmFjLnph
Disclaimer: All claims expressed in this article are solely those of the authors and do not necessarily represent those of their affiliated organizations, or those of the publisher, the editors and the reviewers. Any product that may be evaluated in this article or claim that may be made by its manufacturer is not guaranteed or endorsed by the publisher.
Research integrity at Frontiers
Learn more about the work of our research integrity team to safeguard the quality of each article we publish.