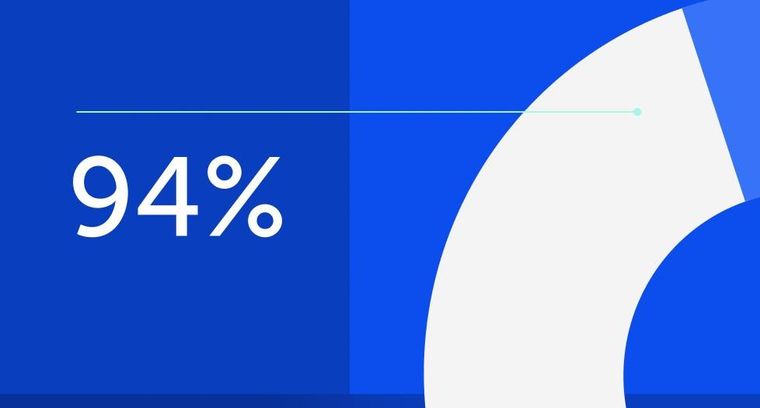
94% of researchers rate our articles as excellent or good
Learn more about the work of our research integrity team to safeguard the quality of each article we publish.
Find out more
ORIGINAL RESEARCH article
Front. Bioeng. Biotechnol., 28 March 2025
Sec. Nanobiotechnology
Volume 13 - 2025 | https://doi.org/10.3389/fbioe.2025.1548025
This article is part of the Research TopicIn vivo Applications of NanozymesView all articles
Background: Bacterial resistance remains a significant challenge, necessitating the development of new antibacterial strategies. This study introduces a rapidly synthesized L-histidine- Fe3O4 (L-His-Fe3O4) nanozyme with enhanced peroxidase (POD)-like activity, designed to improve antibacterial efficacy and accelerate the healing of bacteria-infected wounds.
Methods: We successfully synthesized L-His-Fe3O4 using an ultrafast, room-temperature synthesis method, and observed its anti-infection effect and explored its anti-infection mechanism through in vivo and in vitro antibacterial experiments.
Results: We produced L-His-Fe3O4 cost-effectively while preserving L-His, which was essential for its catalytic and antibacterial functions. The resulting nanozyme demonstrated exceptional antibacterial activity against both Gram-positive (S. aureus) and Gram-negative (E. coli) bacteria. In vivo experiments revealed that L-His-Fe3O4 outperformed vancomycin in reducing bacterial viability and effectively promoting wound healing, all while maintaining excellent biosafety with no adverse effects on blood or liver functions.
Discussion: These findings highlight the potential of L-His-Fe3O4 for large-scale production and practical use in treating bacterial infections, offering a promising approach to combating antibiotic-resistant pathogens.
The misuse and overuse of antibiotics have led to an alarming rise in multidrug-resistant bacteria, posing a severe threat to global public health (Brown and Wright, 2016; Pogue et al., 2015). Infections such as tuberculosis and gonorrhea are increasingly challenging to treat due to this resistance (Alim-Marvasti et al., 2016; Lee et al., 2020). Unfortunately, the development of new antibiotics has lagged behind the rapid emergence of bacterial resistance (Theuretzbacher et al., 2020; Brussow, 2024; Burki, 2018). The World Health Organization (WHO) predicts that by 2050, antibiotic resistance could result in up to 10 million deaths annually, identifying it as one of the top ten global threats to humanity (He et al., 2014). To address bacterial resistance, researchers have explored various novel antibacterial strategies (Panda et al., 2018; Gupta et al., 2019; Li et al., 2019; Makabenta et al., 2021), including antimicrobial peptides, bacteriophages, probiotics, and plant extracts; however, much of this research remains in its early stages.
In recent years, advancements in nanotechnology have led to the application of nanomaterials in medicine (Fan et al., 2021; Wang et al., 2018; Seaberg et al., 2023; Gao et al., 2007). In 2007, the Yan group demonstrated that Fe3O4 possesses peroxidase-like activity (Gao et al., 2024; Xie et al., 2024), paving the way for the development of nanozymes-nanomaterials with enzyme-like catalytic properties (Jiang et al., 2019; Sheng et al., 2024). Due to their adjustable physicochemical properties and unique catalytic activities, nanozymes hold significant promise for antibacterial applications (Liu et al., 2024). They can effectively kill bacteria by generating reactive oxygen species (ROS), such as hydroxyl radicals (·OH) and superoxide anions (·O2−) (Song et al., 2024; Trujillo-Alonso et al., 2019). Numerous studies have reported various nanozymes for antibacterial purposes, with Fe3O4 receiving FDA approval for biomedical applications, highlighting its high biosafety profile (Wei et al., 2021). Consequently, Fe3O4 emerges as a particularly promising candidate in combating bacterial infections. However, the primary synthesis methods for Fe3O4 like hydrothermal and solvothermal approaches are often tedious, time-consuming, expensive, and high temperature, which hinders large-scale production (Dong et al., 2022; Liu et al., 2021). Thus, there is an urgent need to improve Fe3O4 synthesis methods to accelerate the development of new antibacterial agents.
In this work, we present a low-cost and ultrafast method for synthesizing Fe3O4 to enhance its antibacterial properties. The synthesis of Fe3O4 at room temperature can preserve the structure and biological function of the modified histidine (L-His), thereby enhancing its antibacterial properties. In detail, by mixing ferrous chloride and L-His in an ammonium hydroxide solution for only 1 minute, we successfully synthesized L-His-Fe3O4. This method leverages histidine’s ability to enhance peroxidase (POD)-like activity through its activation of hydrogen peroxide reduction reactions (Yang et al., 2019; Du et al., 2022). Notably, this reaction occurs at room temperature, preserving the functional integrity of L-His. The improved POD-like activity of L-His-Fe3O4 catalyzed the production of hydroxyl radicals (·OH), which disrupt bacterial cell membranes and degrade biofilms. Both in vitro and in vivo antibacterial tests demonstrated the strong antimicrobial efficacy of L-His-Fe3O4. In wound healing studies, L-His-Fe3O4 exhibited a superior capacity to promote healing compared with vancomycin. Additionally, L-His-Fe3O4 showed excellent biosafety, reinforcing its potential as a novel antibacterial agent. Moreover, the synthesis strategy of L-His-Fe3O4 at room temperature provide a new pathway for complete modification of different functional groups of Fe3O4.
Ferrous chloride (FeCl2) and L-His were purchased from Sigma and Beyotime, respectively. Ammonium hydroxide was obtained from J&K Scientific. MRSA (ATCC 43300) and S. aureus (ATCC 29213) were sourced from the American Type Culture Collection (ATCC), and E. coli (CCUG58541) was obtained from the BeNa Culture Collection (BNCC). Balb/c mice were purchased from Qinglongshan Laboratory Animal Center. Fresh O-type red blood cells from Wuxi Central blood bank. Yeast extract (LP0021), glucose, and starch were obtained from Oxoid (UK). Dimethyl sulfoxide (DMSO) and agar were purchased from Sangon Biotech (China). Cell Counting Kit-8, crystal violet, and hydrogen peroxide (H2O2) were acquired from Aladdin Chemistry (Shanghai, China). Propidium iodide (PI) and Reactive Oxygen Species Assay Kit were sourced from Beyotime Biotechnology (Shanghai, China), while SYTO (Panda et al., 2018) green fluorescent nucleic acid stain was obtained from Thermo Fisher Scientific (Waltham, MA).
Calculations were performed using the CP2K 2024.1 program. In the geometry optimization, Basis Set Superposition Error (BSSE) correction, and single-point calculations, the PBE-D3(BJ) functional was employed, incorporating Grimme’s dispersion correction (D3) and the Becke-Johnson (BJ) damping factor. The DZVP-MOLOPT-SR-GTH basis set and the TZVP-MOLOPT-SR-GTH basis set were used for optimization and single-point calculations, respectively. Optimization continued until atomic forces were less than 0.02 eV/Å, with the self-consistent electronic energy convergence criterion set at 10−6 eV. Multiwfn 3.8 Dev was used for computational analysis, and visualization was performed with VESTA and Visual Molecular Dynamics (VMD) 1.9.3.
S. aureus and E. coli were cultured in LB medium, composed of 10 g of tryptone, 5 g of yeast extract, 10 g of sodium chloride, and 15–20 g of agar powder for solid medium. Agar plates and liquid cultures were incubated at 37°C, and frozen stocks of strains were stored at −80°C in LB containing 30% (v/v) glycerol.
A colony of S. aureus or E. coli was randomly selected from LB agar plates, inoculated into 5 mL of LB culture, and incubated at 37°C for 12–18 h. After 24 h, the required amount of S. aureus or E. coli bacterial solution was transferred into fresh medium at a 1:100 ratio and cultured at 37°C for 4–6 h. When the OD600 reached 0.5, 100 μL of bacterial inoculum was mixed with H2O (900 μL) as the control, while the experimental groups included L-His-Fe3O4, L-His-Fe3O4 + H2O2, and H2O2. After incubation at 37°C for a specified time, bacterial viability was assessed by plating bacteria with appropriate dilution and calculating the colony-forming units per mL (CFU/mL). The fluorescent probe DCFH-DA was used to assess lipid peroxidation levels in the bacteria.
To facilitate observation of S. aureus biofilm changes, biofilms were cultured on a specialized cell climbing plate in a 24-well format, following previous S. aureus biofilm culture methods. Tryptone soy broth (TSB) was used as the medium. The procedure is as follows.
(1) A sterile cell pad was placed at the bottom of a well in a 24-well plate, and 2.8 mL TSB culture solution was added and incubated for 30 min before discarding the TSB.
(2) S. aureus solution was diluted with TSB to an OD600 of 0.5 at a 1:100 ratio, with 2.5 mL added per well, and incubated at 37°C for 24 h. To maintain a humid environment conducive to biofilm growth, saline was added to the remaining empty wells.
(3) Once the biofilm matured, the specified treatment substance was added. The culture solution and non-adherent bacteria were gently removed, and each well was washed twice with 2.5 mL PBS. The treatment was then applied for 3 h.
(4) For laser confocal observation, bacterial cells within the biofilm were stained with SYTO9/PI using a double-labeled fluorescence method and observed under a confocal laser microscope (SYTO9 = 0.5 µM; PI = 1 µM).
8-aniline-1-naphthalenesulfonic acid (ANS) has a non-polar benzene ring, which binds to the membrane lipid at the lipid-water junction. The fluorescence intensity of ANS is related to the fluidity of the polar region of the membrane lipid. Membrane permeability was assessed using the ANS uptake method. A solution of 20 µM ANS and S. aureus (107 CFU/mL) was prepared in 0.9% NaCl and incubated in darkness for 30 min. After centrifugation at 5000 g for 5 min, samples were washed and re-suspended in 0.9% NaCl. Fluorescence emission was measured at 450–600 nm with excitation at 380 nm. 3, 3′- Dipropylthiadicarbocyanine Iodide [diSC3(5)], a (C3) short alkyl tail carbonyl cyanine dyes, this kind of cationic dye can be used to detect and measure caused by membrane modification reagent across membrane potential or structural changes. Depolarization measurements were performed using diSC3(5) uptake with similar processing steps [diSC3(5), 4 μM; 622 nm excitation, 670 nm emission].
For each group, 0.5 mL of bacterial suspension (1.0 × 109 CFU/mL) was added to a 1.5 mL aseptic centrifuge tube along with 0.5 mL of ultra-pure water, H2O2, or L-His-Fe3O4 + H2O2. Each group’s bacterial solution was incubated at 37°C for 3 h. After incubation, samples were centrifuged and washed twice with ultra-pure water. The bacterial precipitate was then mixed with 2.5% glutaraldehyde solution and fixed at 4°C for 24 h. Bacterial morphology was observed and photographed using TEM and SEM.
S. aureus solution with OD600 = 0.5 was diluted with biofilm culture medium (TSB) at a ratio of 1:100, with 100 µL added per well. Cultures were incubated at 37°C with 5% CO2 for 24 h.
A medium containing the appropriate concentration of the test compound was added, and the cell culture plate was incubated for the specified time. Rinsing to remove floating bacteria: Methanol was used to rinse off floating bacteria, followed by a 15-min fixation with methanol, samples were then air-dried before staining.
1 mL of 0.1% crystal violet solution was added to each well and stained for 15 min. Excess stain was rinsed off with distilled water. Dissolution of Crystal Violet: 33% glacial acetic acid was added to dissolve the crystal violet.
HaCaT cells were inoculated into 96-well plates at a density of 5 × 103 cells per well and cultured in a serum-containing medium for 24 h (37°C, 5% CO2). Culture medium containing varying concentrations of L-His-Fe3O4 (0, 100, 200, 400, 500 μg/mL) was then applied for 24 and 48 h. The medium was discarded, cells were washed once with PBS, and then cultured with CCK-8 for 3–4 h. After incubation, the 96-well plates were centrifuged (1,000 rpm for 5 min), 100 μL of supernatant was pipetted, and absorbance was measured at 490 nm.
Fresh O-type red blood cells from Wuxi Central blood bank (Ethics Committee of Jiangsu Institute of Parasitic Diseases: JIPD-2022-005). An appropriate amount of human red blood cells was obtained and diluted fivefold with PBS buffer to prepare a red blood cell suspension. A 20 μL aliquot of the diluted suspension was mixed with different concentrations of L-His-Fe3O4. Triton X-100 and PBS buffer were used as positive and negative controls, respectively. All samples were incubated at 37°C for 2 h, then centrifuged at 1,000 rpm for 5 min. Photos were taken, and the supernatant was transferred to a 96-well plate for absorbance measurement at 540 nm.
Mouse wounds were treated externally with L-His-Fe3O4 once daily for seven consecutive days. After this period, histopathological sections of major organs (heart, liver, spleen, lung and kidney) were prepared for HE staining and photographed under the bright-field mode of a fluorescence microscope.
Male Balb/c mice (20–25 g, 6–7 weeks of age) were randomly divided into four groups (n = 8), consisting of (1) control, (2) vancomycin (2 mg/mL, 20 μL), (3) hydrogen peroxide, and (4) L-His-Fe3O4 + H2O2 (2 mg/mL, 20 μL). After shaving, mice were anesthetized, and a circular wound approximately 0.8 cm in diameter was created on the back using surgical scissors. MRSA (1.0 × 108 CFU/mL) was then applied. After 24 h, an animal model of wound infection was established. Treatments were administered daily for the first 7 days following infection. On the 7th day, three mice from each group were randomly selected, wound tissue was collected, ground, diluted, and plated to observe S. aureus counts by plate counting. Photos of wounds were taken on days 1, 3, 5, 7, 9 and 11 to document changes and measure wound size. Mice were euthanized, and organs and wound tissues were collected for hematoxylin-eosin (H&E) staining and Masson analysis.
L-His-Fe3O4 was synthesized by stirring at room temperature for 1 min. Briefly, ferrous chloride and L-His solutions were mixed, followed by the addition of 28% ammonium hydroxide. After stirring at room temperature for 1 min and drying at 60°C, L-His-Fe3O4 was obtained (Figure 1a). The yield of L-His-Fe3O4 is 36.48%, the morphology of L-His-Fe3O4 was observed by transmission electron microscopy (TEM). As shown in Figure 1b, TEM images demonstrated spherical particles of L-His-Fe3O4 with a diameter of approximately 20 nm, primarily composed of Fe and O elements. The selected area electron diffraction (SAED) pattern (Figure 1c) of L-His-Fe3O4 confirms the apparent diffraction rings, which correspond to the (440) (511) (400), and (311) crystal planes. To confirm the efficiency of the synthesis method, X-ray diffraction (XRD) analysis was used to monitor the crystal phase at different reaction times. As shown in Figure 1d, the diffraction peaks of the product at 1 min aligned well with those of Fe3O4 (PDF#19–0,629). After 3, 6, and 12 h of reaction, the crystal phase remained unchanged, indicating the high efficiency of the synthesis method. The particle size distribution of L-His-Fe3O4 ranged from 200 to 800 nm (Figure 1e), and the zeta potential varied with changes in pH (Figure 1f). The generation of oxygen vacancies (OVs) in L-His-Fe3O4 was measured by electron paramagnetic resonance (EPR). The g = 2.003 and the peak with magnetic field at around 3,500 revealed that L-His-Fe3O4 possessed at ypical signal of Ovs (Figures 1g, h).
Figure 1. Synthesis and characterization of L-His-Fe3O4. (a) The preparation process of L-His-Fe3O4. (b) TEM image and the corresponding element mapping image. (c) HAADF-STEM image. (d) XRD spectrums. (e) Size distribution. (f) Zeta potentials at different pH conditions. (g, h). The EPR spectra of L-His-Fe3O4.
To assess the peroxidase -like activity of L-His-Fe3O4, the absorption intensity of the L-His-Fe3O4 nanozyme-catalyzed TMB colorimetric reaction at 652 nm was measured (Figure 2a). The absorbance intensity of oxidized TMB (ox-TMB) increased over time. Furthermore, compared with Fe3O4, L-His-Fe3O4 exhibited higher POD-like activity. The generation of ∙OH radicals was monitored using electron spin resonance (ESR) spectroscopy, and the results (Figure 2b) showed a significant increase in ∙OH signal intensity, indicating that L-His-Fe3O4 could effectively catalyze the formation of ∙OH from H2O2.
Figure 2. Enzyme activity characterization and theoretical calculation of L-His-Fe3O4. (a) Reaction-time curves for the TMB colorimetric reaction catalyzed by Fe3O4 and L-His-Fe3O4. (b) ESR spectra of hydroxyl radicals generated by H2O2, Fe3O4, and L-His-Fe3O4 + H2O2. (c) Electrostatic potential analysis of Fe3O4 and L-His-Fe3O4. (d) Density of states diagram of L-His-Fe3O4. (e) Energy changes during the reaction process of L-His-Fe3O4. (f) Reaction pathway diagram of L-His-Fe3O4.
To investigate L-His’s role in the catalytic reduction of H2O2 from an electronic structure perspective, we conducted electrostatic potential analysis, density of states analysis, and differential charge density mapping before and after L-His modification of the surface. Electrostatic potential analysis on the Fe3O4 (311) surface was performed before and after L-His adsorption (Figure 2c). Red regions indicate positive electrostatic potential, while blue regions indicate negative electrostatic potential. These results demonstrate that the modification increased the polarity of the Fe3O4 surface, facilitating the reaction between Fe3O4 and H2O2 during TMB oxidation.
Density of states plots for the slab model of the Fe3O4 (311) surface before and after L-His adsorption is shown in Figures 2d, e. The results indicate that L-His modification led to a more continuous distribution of states near the Fermi level in Fe3O4, enhancing electron transfer during the catalytic process, reducing the energy barrier, and promoting the reaction (Supplementary Figure S1). Differential charge density maps for H2O2 adsorption on the Fe3O4 (311) surface before and after L-His modification is presented in Supplementary Figure S2. The modification increased the interaction between the Fe3O4 surface and H2O2, with differential charge density results also indicating enhanced charge exchange between the modified Fe3O4 (311) surface and H2O2. According to Coulomb’s law, this intensifies the interaction between the two.
To evaluate the impact of modification on Fe3O4’s catalytic process in the oxidation of TMB by H2O2, we calculated the energy changes throughout the reaction, as shown in Figures 2e, f. Steps one to six correspond to the stages in the reaction pathway diagram, with “*” denoting the adsorption state on the catalyst surface. Oxidized TMB (oxTMB) refers to TMB in its oxidized form after hydrogen loss (Figure 2f) (Equations 1-6).
The calculations revealed that: (1) Compared to the unmodified Fe3O4 surface, the binding energy of L-His-modified Fe3O4 with H2O2 is more negative, indicating a stronger interaction between the modified Fe3O4 surface and H2O2, which facilitates H2O2 activation during catalysis; (2) The energy barrier during the reaction process, occurring in the reduction of H2O2 to ?OH by TMB, was significantly lowered from 5.7058 eV to 1.2987 eV with L-His modification, greatly enhancing the reaction progress.
Nanozymes with peroxidase (POD)-like enzyme activity can catalyze H2O2 to produce ∙OH, a ROS that induces bacterial cell death. The combination of nanozyme and H2O2 represents the primary mechanism of the antibacterial effect of nanozymes. To achieve a satisfactory antibacterial effect, we selected L-His-Fe3O4, which has high POD-like activity, for further investigation of its antibacterial performance in the presence of H2O2. To mitigate the toxicity of high H2O2 concentrations, we used a concentration much lower than the clinically effective range (0.5%–3%, wt%). The experimental results are shown in Figure 3B Following L-His modification, the antibacterial effect of Fe3O4 was significantly enhanced, with efficacy increasing proportionally with concentration (Figure 3a). Results in Figure 3b demonstrate that treating S. aureus with H2O2 or L-His-Fe3O4 (100 μg/mL) alone reduced bacterial counts by approximately 0.5 lg (CFU/mL) compared to the control. However, combining L-His-Fe3O4 (100 μg/mL) with H2O2 (L-His-Fe3O4/H2O2) reduced bacterial counts by 3.04 lg (CFU/mL). Additionally, the morphology of S. aureus after L-His-Fe3O4 treatment showed noticeable damage (Supplementary Figures S3, S4). Similar effects were observed in the elimination of other Gram-negative bacteria, such as E. coli (Figure 3c). Post L-His-Fe3O4 treatment, laser confocal microscopy revealed a significant increase in the proportion of dead cells within the S. aureus biofilm (Figure 3d), corresponding fluorescence intensity analysis of Figure 3d (Supplementary Figure S5). Along with a marked reduction in crystal violet staining and biofilm dry weight compared with the control (Figures 3e, f). The lipid oxidation fluorescent ratio-probe DCFH-DA indicated increased lipid ROS when S. aureus was treated with L-His-Fe3O4 (Figure 3g). ANS as a fluorescent probe can be used to detect membrane permeability. Fluorescence monitoring of ANS dye in S. aureus cells before and after L-His-Fe3O4 treatment revealed a significant increase in membrane permeability (Figure 3h). Using the membrane potential-sensitive probe DiSC3, we observed that L-His-Fe3O4 depolarized the S. aureus cell membrane, resulting in enhanced fluorescence (Figure 3i), indicating a reduction in membrane potential as DiSC3(5) was released into the solution upon membrane depolarization. This suggested that L-His-Fe3O4 interacted with the bacterial cell membrane, disrupting its integrity and leading to oxidative stress and cell death.
Figure 3. Test of antibacterial property and bactericidal mechanism of L-His-Fe3O4. (a) Measurement of S. aureus viability with varying concentrations of L-His-Fe3O4 and Fe3O4. b-c. Viability of S. aureus (b) and E. coli (c) treated with H2O2 + L-His-Fe3O4. (d) Impact of L-His-Fe3O4 on S. aureus biofilm viability (Scale bar: 20 µm). (e) Crystal violet staining of S. aureus biofilm treated with L-His-Fe3O4. (f) Dry weight of S. aureus biofilm treated with L-His-Fe3O4. (g) ROS levels in S. aureus treated with L-His-Fe3O4. (h) Outer membrane permeabilization by L-His-Fe3O4. (i) Cytoplasmic membrane depolarization by L-His-Fe3O4. The data are presented as the means ± SD. *p < 0.05, **p < 0.01, ***p < 0.001, ****p < 0.0001.
Good biocompatibility is essential for the in vivo and in vitro applications of L-His-Fe3O4. The hemolysis rate is a key indicator of material biocompatibility; thus, human red blood cells were used in a hemolysis test. As shown in Figures 4a, b, even at the highest concentrations of Fe3O4 and L-His-Fe3O4, the hemolysis rate remained below 1%. The viability of HaCaT cells incubated with various concentrations of L-His-Fe3O4 was assessed using a CCK-8 assay, revealing that cell viability remained above 95% across all concentrations (Figure 4c). To further evaluate the in vivo safety of L-His-Fe3O4, mice were treated with L-His-Fe3O4 for 7 days. After treatment, several major organs (heart, liver, spleen, lungs, kidneys) were collected, fixed in 4% neutral formaldehyde, and subjected to histopathological analysis with H&E staining. No lesions were detected in any of the groups, underscoring the biosafety of L-His-Fe3O4 (Figure 4d).
Figure 4. Biosafety assessment of L-His-Fe3O4. In vitro and in vivo toxicity evaluation of L-His-Fe3O4. (a) Hemolysis rate following treatment with Fe3O4 at varying concentrations. (b) Hemolysis rate following treatment with L-His-Fe3O4 at varying concentrations. (c) Cytotoxicity assessment results for L-His-Fe3O4. (d) Tissue sections of major organs treated with PBS and L-His-Fe3O4 (H&E staining; scale bar: 500 µm).
To further investigate the antibacterial efficacy of L-His-Fe3O4 and its potential to promote wound healing, we established a skin wound model of MRSA-infected mice with reference to the experimental method of Liu (Liu et al., 2023). Throughout treatment, wound changes were documented with photographs. As shown in Figure 5a, all experimental groups exhibited gradual scab formation and wound contraction. On day 7, the control and Vancomycin groups displayed severe purulent symptoms, whereas the H2O2 and L-His-Fe3O4 + H2O2 groups showed no purulence and began to scab. By day 9, scabs started forming in the control and Vancomycin groups, while those in the H2O2 and L-His-Fe3O4 + H2O2 groups began to peel off. On day 11, the wounds in the L-His-Fe3O4 + H2O2 group were significantly smaller than those in the other groups. To evaluate wound healing, H&E and Masson staining were performed on the wound tissues. As shown in Figure 5b, after 11 days of treatment, the control, Vancomycin, and H2O2 groups displayed incomplete epidermal layers, while the treatment group showed uniformly distributed collagen fibers. Additionally, we monitored the weight changes in each group during treatment (Figure 5c), finding no significant weight differences among the groups. On day 7, three mice from each group were selected, and the wound tissues were ground and subjected to plate counting; the results are shown in Figure 5d. The L-His-Fe3O4 + H2O2 treatment group exhibited significantly stronger antibacterial effects than the other groups, confirming that the treatment of infected wounds in mice with L-His-Fe3O4 + H2O2 is effective.
Figure 5. Application evaluation of bacterial infection in skin wounds. (a) Wound area of mice treated with PBS, Vancomycin, H2O2 and L-His-Fe3O4 over time. (b) H&E (top) and Masson staining (bottom) of infected tissues following different treatments. (c) Changes in body weight of mice over time with various treatments (n = 5) (d) Residual bacterial counts in wound tissue after different treatments. The data are presented as the means ± SD. *p < 0.05, **p < 0.01.
With the increasing of antibiotic resistance in the world, the search for new antimicrobial agents has become a hot spot in today’s research (Dance, 2024). As a kind of antibacterial agent with high efficiency, selectivity and biocompatibility, nanozymes have received extensive attention (Labrag et al., 2023; Chakraborty et al., 2022). Among them, Fe3O4 nano-enzyme has become the focus of research because of its excellent performance and wide application prospect. In 2007, Gao et al. (2007) first identified magnetic iron oxide nanoparticles (Fe3O4 nanoparticles) with significant enzyme-like activity. Studies showed that Fe3O4 had a pH-dependent peroxide-like activity and catalase activity. In particular, Fe3O4 effectively catalyzes hydrogen peroxide to produce hydroxyl radicals in acidic conditions, which is antibacterial and kills tumor cells (Gao et al., 2016; Shi et al., 2018). Although Fe3O4 nanoenzymes have broad application prospects in biomedicine, there are still some challenges. Firstly, the synthesis method of Fe3O4 nanozymes need to be further optimized to improve their yield and stability. This study provides a convenient and rapid synthesis method for Fe3O4 at room temperature, supports large-scale production, and has important application prospects in the field of antibacterial.
The commonly used methods for preparing Fe3O4 include chemical coprecipitation, thermal decomposition, sol-gel, mechanical synthesis and oil phase coprecipitation (Yang et al., 2011). However, the existing preparation methods still have some defects: the preparation process is complicated, multiple parameters need to be controlled, and the preparation cycle is long (Wang et al., 2024). For example, hydrothermal synthesis of Fe3O4 often requires a high temperature of more than 200°C in a closed high-pressure reactor for 12 h (Gao et al., 2007), which requires high equipment and complex operation, which may cause certain pressure and safety hazards to the environment. We have synthesized L-His-Fe3O4 at room temperature by an improved method, L-His-Fe3O4 was quickly synthesized by stirring at room temperature for 1 min. TEM images show that L-His- Fe3O4 is a spherical particle with a diameter of about 20 nm, mainly composed of iron and oxygen elements (Figure 1). This method is simple to operate, the prepared iron oxide nanoparticles are of high quality and the end modification is flexible.
As a commonly used high reactive oxygen species, H2O2 has been widely used to prevent and control the infection of various pathogenic microorganisms. However, high doses of H2O2 can cause unnecessary damage to normal tissue, delaying healing. Fe3O4 nanozymes have peroxisase-like activity, which can promote the transformation of H2O2 to OH, and have great potential in tumor catalytic therapy and antimicrobial resistance (Zarandona et al., 2023; Anghel et al., 2014). Fe3O4 nanozymes with enzyme properties can effectively improve the antibacterial performance of H2O2, while avoiding the side effects of high concentration of H2O2, and become a promising antibacterial agent (Uwaya et al., 2020). We report a novel strategy for the rapid synthesis of Fe3O4 nanozymes by adding L-His. We demonstrated that L-His- Fe3O4 can be used to kill resistant bacteria such as S. aureus and E. coli, destroy biofilm (Figure 3). Animal experiments demonstrated the potential of L-His- Fe3O4 as a multifunctional material for wound infections (Figure 5).
In summary, this study demonstrates the synthesis of L-His-Fe3O4 using an ultrafast, low-cost method at room temperature. The addition of L-His significantly enhanced POD-like activity, endowing L-His-Fe3O4 with excellent antibacterial efficacy against S. aureus and E. coli. Following L-His modification, the antibacterial rate against S. aureus increased from 72.72% to 99.92%. The antibacterial effect relied on the POD-like activity of L-His-Fe3O4, which generated ∙OH to damage bacterial cell membranes. Cytotoxicity assessments via CCK-8 and red blood cell hemolysis tests confirmed high biosafety. In vivo experiments demonstrated a substantial therapeutic effect of L-His-Fe3O4 on bacteria-infected wounds. The synthesis method preserves the integrity of amino acids and can be applied to modify various functional groups, suggesting it as a potentially universal approach. This study offers a convenient and rapid synthesis method for Fe3O4, supporting large-scale production and presenting significant promise for future applications in the antibacterial field.
The original contributions presented in the study are included in the article/Supplementary Material, further inquiries can be directed to the corresponding authors.
Ethical approval was not required for the studies on humans in accordance with the local legislation and institutional requirements because only commercially available established cell lines were used. The animal study was approved by Life Science Ethics Review Committee, Zhengzhou University: GZR 2022-1328. The study was conducted in accordance with the local legislation and institutional requirements.
YY: Funding acquisition, Investigation, Methodology, Project administration, Writing–original draft. YL: Investigation, Methodology, Writing–original draft. ZS: Investigation, Methodology, Writing–original draft. HW: Investigation, Methodology, Writing–original draft. LM: Investigation, Methodology, Writing–original draft. XG: Investigation, Methodology, Writing–original draft. BJ: Project administration, Writing–review and editing. LF: Data curation, Funding acquisition, Writing–original draft.
The author(s) declare that financial support was received for the research and/or publication of this article. This work has been supported by the Top Talent Support Program for young and middle-aged people of Wuxi Health Committee (HB2023115); The National Natural Science Foundation of China (NO. 82302562). In addition, the authors gratefully acknowledge the financial support from the National Natural Science Foundation of China (32,401,186, 32,201,162), the 70th general grant of China Postdoctoral Science Foundation (2021M702947), the 74th general grant of China Postdoctoral Science Foundation (2023M743208). The animal study was approved by Life Science Ethics Review Committee, Zhengzhou University: GZR 2022-1328.
The authors declare that the research was conducted in the absence of any commercial or financial relationships that could be construed as a potential conflict of interest.
The author(s) declare that no Generative AI was used in the creation of this manuscript.
All claims expressed in this article are solely those of the authors and do not necessarily represent those of their affiliated organizations, or those of the publisher, the editors and the reviewers. Any product that may be evaluated in this article, or claim that may be made by its manufacturer, is not guaranteed or endorsed by the publisher.
The Supplementary Material for this article can be found online at: https://www.frontiersin.org/articles/10.3389/fbioe.2025.1548025/full#supplementary-material
Alim-Marvasti, A., Bi, W., Mahroo, O. A., Barbur, J. L., and Plant, G. T. (2016). Transient smartphone blindness. N. Engl. J. Med. 374, 2502–2504. doi:10.1056/NEJMc1514294
Anghel, A. G., Grumezescu, A., Chirea, M., Grumezescu, V., Socol, G., Iordache, F., et al. (2014). MAPLE fabricated Fe3O4@Cinnamomum verum antimicrobial surfaces for improved gastrostomy tubes. Molecules 19, 8981–8994. doi:10.3390/molecules19078981
Brown, E. D., and Wright, G. D. (2016). Antibacterial drug discovery in the resistance era. Nature 529, 336–343. doi:10.1038/nature17042
Brussow, H. (2024). The antibiotic resistance crisis and the development of new antibiotics. Microb. Biotechnol. 17, e14510. doi:10.1111/1751-7915.14510
Burki, T. K. (2018). Superbugs: an arms race against bacteria. Lancet Respir. Med. 6, 668. doi:10.1016/S2213-2600(18)30271-6
Chakraborty, N., Gandhi, S., Verma, R., and Roy, I. (2022). Emerging prospects of nanozymes for antibacterial and anticancer applications. Biomedicines 10, 1378. doi:10.3390/biomedicines10061378
Dance, A. (2024). Five ways science is tackling the antibiotic resistance crisis. Nature 632, 494–496. doi:10.1038/d41586-024-02601-4
Dong, H., Du, W., Dong, J., Che, R., Kong, F., Cheng, W., et al. (2022). Depletable peroxidase-like activity of Fe(3)O(4) nanozymes accompanied with separate migration of electrons and iron ions. Nat. Commun. 13, 5365. doi:10.1038/s41467-022-33098-y
Du, Y., Ke, Z., Zhang, J., and Feng, G. (2022). Dual-signal output paper sensor based on coordinative self-assembly biomimetic nanozyme for point-of-care detection of biomarker. Biosens. Bioelectron. 216, 114656. doi:10.1016/j.bios.2022.114656
Fan, X., Yang, F., Nie, C., Ma, L., Cheng, C., and Haag, R. (2021). Biocatalytic nanomaterials: a new pathway for bacterial disinfection. Adv. Mater 33, e2100637. doi:10.1002/adma.202100637
Gao, L., Liu, Y., Kim, D., Li, Y., Hwang, G., Naha, P. C., et al. (2016). Nanocatalysts promote Streptococcus mutans biofilm matrix degradation and enhance bacterial killing to suppress dental caries in vivo. Biomaterials 101, 272–284. doi:10.1016/j.biomaterials.2016.05.051
Gao, L., Wei, H., Dong, S., and Yan, X. (2024). Nanozymes. Adv. Mater 36, e2305249. doi:10.1002/adma.202305249
Gao, L., Zhuang, J., Nie, L., Zhang, J., Zhang, Y., Gu, N., et al. (2007). Intrinsic peroxidase-like activity of ferromagnetic nanoparticles. Nat. Nanotechnol. 2, 577–583. doi:10.1038/nnano.2007.260
Gupta, A., Mumtaz, S., Li, C. H., Hussain, I., and Rotello, V. M. (2019). Combatting antibiotic-resistant bacteria using nanomaterials. Chem. Soc. Rev. 48, 415–427. doi:10.1039/c7cs00748e
He, W., Kim, H. K., Wamer, W. G., Melka, D., Callahan, J. H., and Yin, J. J. (2014). Photogenerated charge carriers and reactive oxygen species in ZnO/Au hybrid nanostructures with enhanced photocatalytic and antibacterial activity. J. Am. Chem. Soc. 136, 750–757. doi:10.1021/ja410800y
Jiang, D., Ni, D., Rosenkrans, Z. T., Huang, P., Yan, X., and Cai, W. (2019). Nanozyme: new horizons for responsive biomedical applications. Chem. Soc. Rev. 48, 3683–3704. doi:10.1039/c8cs00718g
Labrag, J., Abbadi, M., Hnini, M., Bekkali, C. E., Bouziani, A., Robert, D., et al. (2023). Antibiotic photocatalysis and antimicrobial activity of low-cost multifunctional Fe(3)O(4)@HAp nanocomposites. J. Environ. Health Sci. Eng. 21, 429–440. doi:10.1007/s40201-023-00869-8
Lee, A., Xie, Y. L., Barry, C. E., and Chen, R. Y. (2020). Current and future treatments for tuberculosis. BMJ 368, m216. doi:10.1136/bmj.m216
Li, X., Bai, H., Yang, Y., Yoon, J., Wang, S., and Zhang, X. (2019). Supramolecular antibacterial materials for combatting antibiotic resistance. Adv. Mater 31, e1805092. doi:10.1002/adma.201805092
Liu, Q., Liu, X., He, X., Wang, D., Zheng, C., Jin, L., et al. (2024). Iron-single-atom nanozyme with NIR enhanced catalytic activities for facilitating MRSA-infected wound therapy. Adv. Sci. (Weinh) 11, e2308684. doi:10.1002/advs.202308684
Liu, Q., Wan, K., Shang, Y., Wang, Z. G., Zhang, Y., Dai, L., et al. (2021). Cofactor-free oxidase-mimetic nanomaterials from self-assembled histidine-rich peptides. Nat. Mater 20, 395–402. doi:10.1038/s41563-020-00856-6
Liu, Y., Wang, X., Fan, X., Ge, M., Fang, L., Yuan, Y., et al. (2023). Coordination-precipitation synthesis of metal sulfide with phase transformation enhanced reactivity against antibiotic-resistant bacteria. Adv. Funct. Mater. 33. doi:10.1002/adfm.202212655
Makabenta, J. M. V., Nabawy, A., Li, C. H., Schmidt-Malan, S., Patel, R., and Rotello, V. M. (2021). Nanomaterial-based therapeutics for antibiotic-resistant bacterial infections. Nat. Rev. Microbiol. 19, 23–36. doi:10.1038/s41579-020-0420-1
Panda, S., Rout, T. K., Prusty, A. D., Ajayan, P. M., and Nayak, S. (2018). Electron transfer directed antibacterial properties of graphene oxide on metals. Adv. Mater 30. doi:10.1002/adma.201702149
Pogue, J. M., Kaye, K. S., Cohen, D. A., and Marchaim, D. (2015). Appropriate antimicrobial therapy in the era of multidrug-resistant human pathogens. Clin. Microbiol. Infect. 21, 302–312. doi:10.1016/j.cmi.2014.12.025
Seaberg, J., Clegg, J. R., Bhattacharya, R., and Mukherjee, P. (2023). Self-therapeutic nanomaterials: applications in biology and medicine. Mater TodayKidlingt. 62, 190–224. doi:10.1016/j.mattod.2022.11.007
Sheng, J., Wu, Y., Ding, H., Feng, K., Shen, Y., Zhang, Y., et al. (2024). Multienzyme-like nanozymes: regulation, rational design, and application. Adv. Mater 36, e2211210. doi:10.1002/adma.202211210
Shi, S., Wu, S., Shen, Y., Zhang, S., Xiao, Y., He, X., et al. (2018). Iron oxide nanozyme suppresses intracellular Salmonella Enteritidis growth and alleviates infection in vivo. Theranostics 8, 6149–6162. doi:10.7150/thno.29303
Song, N., Yu, Y., Zhang, Y., Wang, Z., Guo, Z., Zhang, J., et al. (2024). Bioinspired hierarchical self-assembled nanozyme for efficient antibacterial treatment. Adv. Mater 36, e2210455. doi:10.1002/adma.202210455
Theuretzbacher, U., Outterson, K., Engel, A., and Karlen, A. (2020). The global preclinical antibacterial pipeline. Nat. Rev. Microbiol. 18, 275–285. doi:10.1038/s41579-019-0288-0
Trujillo-Alonso, V., Pratt, E. C., Zong, H., Lara-Martinez, A., Kaittanis, C., Rabie, M. O., et al. (2019). FDA-approved ferumoxytol displays anti-leukaemia efficacy against cells with low ferroportin levels. Nat. Nanotechnol. 14, 616–622. doi:10.1038/s41565-019-0406-1
Uwaya, G. E., Fayemi, O. E., Sherif, E. M., Junaedi, H., and Ebenso, E. E. (2020). Synthesis, electrochemical studies, and antimicrobial properties of Fe(3)O(4) nanoparticles from callistemon viminalis plant extracts. Mater. (Basel) 13, 4894. doi:10.3390/ma13214894
Wang, X., Liu, T., Chen, M., Liang, Q., Jiang, J., Chen, L., et al. (2024). An erythrocyte-templated iron single-atom nanozyme for wound healing. Adv. Sci. (Weinh) 11, e2307844. doi:10.1002/advs.202307844
Wang, Y., Sun, S., Zhang, Z., and Shi, D. (2018). Nanomaterials for cancer precision medicine. Adv. Mater 30, e1705660. doi:10.1002/adma.201705660
Wei, F., Cui, X., Wang, Z., Dong, C., Li, J., and Han, X. (2021). Recoverable peroxidase-like Fe(3)O(4)@MoS(2)-Ag nanozyme with enhanced antibacterial ability. Chem. Eng. J. 408, 127240. doi:10.1016/j.cej.2020.127240
Xie, L., Wu, H., Li, Y., Shi, L., and Liu, Y. (2024). Recent development of nanozymes for combating bacterial drug resistance: a review. Adv. Healthc. Mater, e2402659. doi:10.1002/adhm.202402659
Yang, C., Wu, J., and Hou, Y. (2011). Fe3O4 nanostructures: synthesis, growth mechanism, properties and applications. Chem. Commun. (Camb) 47, 5130–5141. doi:10.1039/c0cc05862a
Yang, M., He, H., Du, J., Peng, H., Ke, G., and Zhou, Y. (2019). Insight into the kinetic influence of oxygen vacancies on the WO(3) photoanodes for solar water oxidation. J. Phys. Chem. Lett. 10, 6159–6165. doi:10.1021/acs.jpclett.9b02365
Zarandona, I., Correia, D. M., Moreira, J., Costa, C. M., Lanceros-Mendez, S., Guerrero, P., et al. (2023). Magnetically responsive chitosan-pectin films incorporating Fe(3)O(4) nanoparticles with enhanced antimicrobial activity. Int. J. Biol. Macromol. 227, 1070–1077. doi:10.1016/j.ijbiomac.2022.11.286
Keywords: nanozyme, antibacterial, l-histidine, Fe3O4, biofilm
Citation: Yuan Y, Liu Y, Shen Z, Wu H, Meng L, Guo X, Jiang B and Fang L (2025) Ultrafast synthesis of L-His-Fe3O4 nanozymes with enhanced peroxidase-like activity for effective antibacterial applications. Front. Bioeng. Biotechnol. 13:1548025. doi: 10.3389/fbioe.2025.1548025
Received: 19 December 2024; Accepted: 20 March 2025;
Published: 28 March 2025.
Edited by:
Yunjiao Zhang, South China University of Technology, ChinaReviewed by:
Liqian Gao, Sun Yat-sen University, ChinaCopyright © 2025 Yuan, Liu, Shen, Wu, Meng, Guo, Jiang and Fang. This is an open-access article distributed under the terms of the Creative Commons Attribution License (CC BY). The use, distribution or reproduction in other forums is permitted, provided the original author(s) and the copyright owner(s) are credited and that the original publication in this journal is cited, in accordance with accepted academic practice. No use, distribution or reproduction is permitted which does not comply with these terms.
*Correspondence: Bing Jiang, amlhbmdiaW5nQHp6dS5lZHUuY24=; Ling Fang, ZmFuX2dsaW5fZ0AxMjYuY29t
†These authors share first authorship
Disclaimer: All claims expressed in this article are solely those of the authors and do not necessarily represent those of their affiliated organizations, or those of the publisher, the editors and the reviewers. Any product that may be evaluated in this article or claim that may be made by its manufacturer is not guaranteed or endorsed by the publisher.
Research integrity at Frontiers
Learn more about the work of our research integrity team to safeguard the quality of each article we publish.