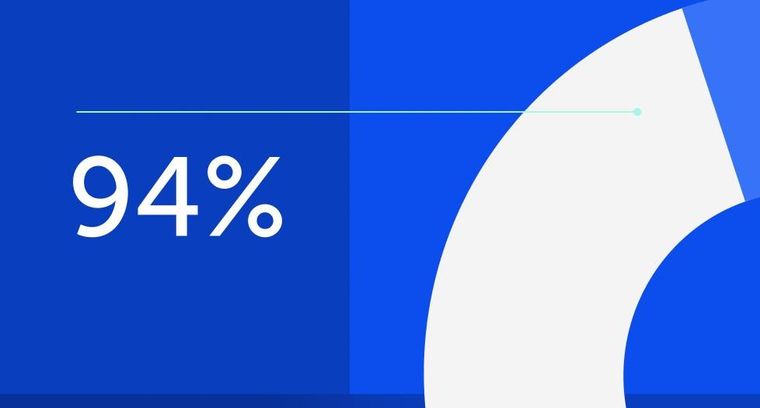
94% of researchers rate our articles as excellent or good
Learn more about the work of our research integrity team to safeguard the quality of each article we publish.
Find out more
ORIGINAL RESEARCH article
Front. Bioeng. Biotechnol., 24 March 2025
Sec. Bioprocess Engineering
Volume 13 - 2025 | https://doi.org/10.3389/fbioe.2025.1546801
Recent research has focused on issues related to contamination, nutrient availability, and strain selection, but there has been insufficient focus on harvesting research. This study employed an integrated continuous cultivation and harvesting strategy for a Spirulina microalgae biorefinery. The effects of nutrient-deficiency, harvesting ratio, and NaNO3 addition on biomass concentration and productivity and phycocyanin accumulation of Spirulina were investigated. The lowest biomass productivity of 0.015 g/L/day was observed in Spirulina cultivated in NaNO3 deficient medium. A harvesting ratio of 10% showed a consistent range of harvested dry biomass weight (0.20–0.22 g). Addition of 2.50 g/L NaNO3 resulted in a significant increase in C-phycocyanin (C-PC) and allophycocyanin (APC) concentration from 34.37 mg/g to 68.35 and 27.08 to 33.23 mg/g, respectively. Biomass productivity of 1-L and 10-L batch culture was found to be 0.23 g/L/d and 0.21 g/L/d, respectively. Both 1-L and 10-L batch cultures showed a significant increase in phycocyanin accumulation due to the addition of 2.50 g/L of NaNO3. These findings highlight the feasibility of continuous cultivation and optimized harvesting for scalable biomass and phycocyanin production, offering valuable insights for industrial biorefineries that seek to enhance microalgae-based bioactive compound extraction.
The global demand for the production of microalgae biomass as an alternative resource for applications such as biofuels, cosmetics, animal feed, carbon capture, pharmaceuticals, and food and nutritional supplements is currently expected to be at least 30,000 tons per year by 2030 (Amorim et al., 2021). Spirulina biomass accounts for 30% of the current 10,000 tons of total global algae biomass production (Lim et al., 2021). To realize this production target by 2030, researchers around the world have made many proposals to improve biomass productivity in the upstream processing of microalgae. Current industrial microalgae biomass production faces five challenges: contamination, cost, harvesting, nutrient availability, and strain selection (Venkata Subhash et al., 2022; Ummalyma et al., 2022).
Extensive research has explored alternative solutions to overcome issues of the selection, optimization, and formulation of cultivation medium in the microalgae biorefinery (Fábregas et al., 2000; Salunke et al., 2016). Dos Santos et al. (2016) reported that the addition of supplements (e.g., sugarcane vinasse) during microalgae cultivation increased microalgae biomass concentration by 23%, from 0.495 to 0.609 g/L, and yielded a higher protein content of 75%. Despite the selection and optimization of cultivation medium or addition of supplements to culture medium, the increase in biomass productivity is limited by different microalgae strains. Therefore, researchers have shifted their focuses by incorporating strategies involving genetic modification (Khoo et al., 2023). However, this is a relatively new field that requires much ongoing study and research before mutated species can be commercially utilized by industry (Fang et al., 2013). In addition, closed-system photobioreactors have reported that biomass productivity (12 g/m2/d) is higher than in open-pond systems (8 g/m2/d) due to the former’s closed environment and reduced contamination (Narala et al., 2016). However, there is a problem when scaling up the photobioreactor as biomass yield would decrease (Benner et al., 2022). Current research has focused on issues related to contamination, nutrient availability, and strain selection, but there has been insufficient research on harvesting.
Microalgae biomass harvesting methods such as coagulation and flocculation, centrifugation, electrical-based processes, and filtration have been studied (Singh and Patidar, 2018). Recent studies have focused extensively on optimizing these harvesting techniques to improve efficiency and reduce costs. However, researchers have also highlighted the advantages of continuous cultivation over batch and semi-batch strategies (Peter et al., 2022). In batch cultures, the cell composition may change over time as cells age and the bulk environment changes, resulting in harvested biomass of poor quality which may contain dead cells. However, continuous cultures enable efficient control of the growing environment, leading to tailor-made biomass composition at a constant and predetermined rate. Compared to batch mode (7.3 g/L/d), continuous cultivation systems offer higher productivity (42.6 g/L/d), 2.3 to 5 times higher, without accounting for the time required to clean and restart a batch culture (Fernandes et al., 2015; Gao et al., 2016). Despite the extensive research on harvesting methods and the benefits of continuous cultivation, there remains a critical gap in understanding the amount of biomass that should be harvested per unit time in a continuous system to optimize both the yield and sustainability of microalgae growth.
Therefore, our research here addresses the issue above by employing an integrated continuous cultivation and harvesting strategy for the biorefinery of Spirulina microalgae. This research aims to investigates the impact of different harvesting ratios (e.g., 10%, 20%, and 30%) on Spirulina cultivation. Additionally, it examines the effect of different concentrations of sodium nitrate (NaNO3) additions (e.g., 2.5 g/L, 3.5 g/L, and 4.5 g/L) on the phycocyanin (C-phycocyanin—CPC, and allo-phycocyanin—APC) accumulation in Spirulina. Phycocyanin has been studied for its various potential therapeutic properties, including antioxidant, anti-inflammatory, and immunomodulatory effects (ElFar et al., 2022). Based on findings, NaNO3 has been identified as the most important chemical for the growth of Spirulina. To improvise the harvesting strategy, instead of adding the entire fresh medium to replace the harvested biomass volume, we aimed to investigate whether the addition of just NaNO3 is sufficient to enhance the production of phycocyanin accumulation in the Spirulina microalgae, thereby reducing chemical usage in the system while optimizing phycocyanin production. Finally, the tested parameters of harvesting ratios and NaNO3 concentration were tested in different scales of 1-L and 10-L batch cultivation photobioreactor to evaluate and understand its effects on the biomass concentration, biomass productivity, harvested dry biomass, and phycocyanin accumulation. This research will provide insight on the impact of a continuous cultivation and harvesting strategy on biomass and phycocyanin production in a Spirulina microalgae biorefinery.
Spirulina platensis microalgae were collected from Biolina Sdn. Bhd., Malaysia. Cultures were maintained in Zarrouk medium (Dineshkumar et al., 2016) with some modifications—NaHCO3: 18 g/L; NaNO3: 2.5 g/L; MgSO4.7H2O: 0.2 g/L; CaCl2.2H2O: 0.04 g/L; K2HPO4: 0.5 g/L; NaCl: 1 g/L; K2SO4: 1 g/L; FeSO4.7H2O: 0.01 g/L; EDTA: 0.08 g/L; micronutrient: 1 mL/L. The micronutrient consisted of H3BO3: 2.86 g/L; MnCl2.4H2O: 1.81 g/L; ZnSO4.7H2O: 0.222 g/L; Na2MoO4.2H2O: 0.3 g/L; CuSO4.5H2O: 0.07 g/L; Co(NO3)2.6H2O: 0.04 g/L.
Spirulina microalgae were inoculated and pre-cultured in 250-mL optimized Zarrouk medium for 7 days. The pre-cultured Spirulina microalgae were then transferred to a 1-L batch cultivation. The experiments were carried out in a 1-L photobioreactor. These 1-L batch cultivations were placed on a magnetic stirrer to ensure homogeneous mixing. The batch culture was cultivated under photoautotrophic growth conditions. The batch culture experiments were carried out at an illumination of approximately 3000 lux using LED lights. The light intensity was measured using a lux meter (UT383, UNI-T). Aeration was supplied to the batch cultivation via an air compressor (ACO-308, HAILEA, China). The air flow rate was maintained at 400 mL/min using an airflow regulator (Dwyer, Malaysia) throughout the experiment.
A cylindrical closed-system photobioreactor with a 12 cm diameter and 150 cm height was fabricated by Donewell Resources Sdn. Bhd., Malaysia. The material used to fabricate the cultivation tank was acrylic plastic because of its transparency and chemical robustness (Huang et al., 2017). Transparency is crucial for Spirulina cultivation as it requires light for photosynthesis. Chemical robustness is important because of its chemical resistance to the many chemicals used to prepare the cultivation medium. The working volume of the cultivation tank was 10-L.
We prepared 1-L batch cultivation as the inoculum for 10-L batch cultivation. The batch culture was cultivated under photoautotrophic growth conditions. A nano-airstone (VN-132, Yek Fong Aquarium Accessories Sdn Bhd, Malaysia) was placed inside the 10-L cultivation tank to supply aeration via an air compressor for the Spirulina culture. The air flow rate was maintained at 20 L/min using an airflow regulator throughout the experiment. The 10-L batch culture experiments were carried out with an illumination of approximately 2000 lux with four LED light tubes installed around the cultivation tank. The intensity of light was measured with a lux meter. All scale-up cultures were supplemented with Zarrouk media.
Conventional methods typically harvest the entire batch at once. However, this study explored a gradual harvesting approach, starting with 10% of the biomass and progressively increasing the harvesting ratio (10%, 20%. 30%) until the batch culture could no longer maintain a consistent daily biomass production for three consecutive days. Each cultivation batch was cultured for 10 days before consecutive harvesting. The batch cultivation was replenished with a quantity of fresh culture medium equal to that harvested. For each batch cultivation, the absorbance of the culture was analyzed daily. The harvested fresh culture was centrifuged to remove the supernatant, and the resulting biomass was freeze-dried. After the optimum harvesting ratio was determined, it was tested in a large-scale 10-L Spirulina cultivation tank.
Instead of adding the culture medium, only NaNO3 was added since it is the primary source of nitrate in Zarrouk medium with a standard concentration of 2.5 g/L. Previous research has demonstrated increased biomass growth, phycocyanin, and allo-phycocyanin accumulation in nitrate fed-batch phototrophic cultivation of A. platensis FACHB-314 (Manirafasha et al., 2018). Therefore, it is reasonable to explore higher concentrations of 2.5–4.5 g/L to assess its impact on biomass production and phycocyanin accumulation.
The harvesting ratio was fixed at 10% based on previous optimized harvesting ratios conducted in 1-L Spirulina cultivation. The effect of concentration of NaNO3 (2.5, 3.5, and 4.5 g/L) on biomass concentration and phycocyanin accumulation were studied. Each batch of cultivation was cultured for 10 days before harvesting. The batch cultivation was replenished with fresh NaNO3 solution after harvesting. After the optimum NaNO3 addition was determined, it was tested in a large 10-L Spirulina cultivation tank. Table 1 shows an overview of the operating parameters investigated for the production of phycocyanin from Spirulina microalgae.
The cultivation progress was monitored by collecting biomass samples. The absorbance of the biomass concentration of the culture was measured with a UV–Vis spectrophotometer (UV-1800, Shimadzu, Japan) at a 688 nm wavelength (Chen et al., 2016). However, the UV–Vis spectrophotometer has a limitation of reading absorbance values up to 4, so the samples had to be diluted ten times and the average taken to ensure accurate readings. Fresh samples were used to determine the biomass concentration and productivity. Samples for phycocyanin extraction were stored at −20 ℃ until further analysis.
A sample of mature Spirulina medium was subjected to dilutions of 20%, 40%, 60%, 80%, and 100% with deionized water in 15-mL centrifuge tubes. The absorbance of each diluted sample was measured and recorded using a UV-Vis spectrophotometer at a specific wavelength of 688 nm. Subsequently, the samples were centrifuged to remove the supernatant and rinsed with deionized water to remove excess salt, and the resulting biomass was freeze-dried. The dry biomass weight was then measured and recorded. A standard calibration graph was generated by plotting the dry biomass weight against absorbance. The equation obtained from this graph was used to convert all measured absorbance data into dry biomass weight (g/L). The standard calibration graph allows us to estimate the dry biomass weight of Spirulina from the absorbance data. Finally, the remaining cultivated Spirulina biomass was poured into 50-mL centrifuge tubes and centrifuged at 6,500 rpm for 5 min (Koyande et al., 2019). The supernatant was then discarded, and the wet biomass was freeze-dried and stored at −20 ℃ for further analysis. The freeze-dried biomass was used for proximate analysis to determine the phycocyanin content.
The biomass productivity (Pb) and specific growth rate (µ) were calculated based on Equations 1 and 2 (Chew et al., 2018) as follows :
where N1 and N2 are biomass concentration (g/L) at time t1 and t2, respectively.
Two types of phycocyanin were analyzed: C-phycocyanin (CPC) and allo-phycocyanin (APC). Phosphate buffer was added to a measured quantity of freeze-dried Spirulina biomass in a 0.009% (m/v) biomass-to-solvent ratio. The use of phosphate buffer as the solvent was chosen in this study as it has been demonstrated to improve the structural stability of protein molecules during the extraction process, which may be subject to various forces that could lead to degradation or denaturation (G-Bioscience, 2019). The mixture was homogenized using a vortex mixer and then subjected to ultrasonic sonication at a frequency of 35 kHz for 5 min (Chew et al., 2019). This process disrupted the cell walls of the Spirulina cells and released the phycocyanin composition. The sonicated sample was then centrifuged at 6,500 rpm for 5 min, and the supernatant was collected for further analysis. The CPC and APC concentrations in the supernatant were quantified using a UV–Vis spectrophotometer at various wavelengths. Food grade phycocyanin was used as a standard to validate Equations 1 and 2 for quantifying phycocyanin concentrations.
CPC and APC concentrations were calculated based on Equations 3 and 4 (Bennett and Bogorad, 1973).
where OD615 and OD652 are the optical density wavelengths at 615 nm and 652 nm, respectively.
The purities of CPC and APC were calculated based on Equations 5 and 6 (Abalde et al., 1998).
where OD280, OD620, and OD652 are the optical density wavelengths at 280 nm, 625 nm, and 652 nm, respectively.
All cultures were performed in duplicate and average values were reported. The values were then expressed in terms of mean and standard deviation. Subsequently, the data were subjected to one-way analysis of variance (ANOVA) using Microsoft Excel software to assess any significant differences between the groups with a p-value of less than or equal to 0.05.
Spirulina cultivation in NaNO3-deficient medium has the lowest biomass productivity of 0.015 ± 0.002 g/L/day (Figure 1. The Spirulina cultivation in NaNO3-deficient medium did not survive the 10 days of cultivation. This is because NaNO3 is an essential nutrient for Spirulina cultivation as it is the nitrogen source in the cultivation medium. Nitrogen is important for the synthesis of proteins, nucleic acids, and chlorophyll content in microalgae (Wu and Miao, 2014). Nitrogen limitation will result in decreased photosynthesis, protein synthesis, and increase in lipid and carbohydrate synthesis (Li et al., 2012). Therefore, NaNO3-deficient medium shows the lowest biomass productivity. Moreover, phycocyanin is a type of pigment–protein complex. Since NaNO3 is responsible for protein synthesis, this study also investigated the effect of NaNO3 concentration on phycocyanin accumulation in Spirulina.
Figure 1. Effect of nutrient-deficient media on biomass productivity of Spirulina cultivation. [0] Zarrouk medium as control, [1] NaHCO3, [2] NaNO3, [3] NaCl, [4] K2SO4, [5] K2HPO4, [6] MgSO4.7H2O, [7, 9], FeSO4.7H2O and EDTA, [8] CaCl2.2H2O, and [10] micronutrient.
NaHCO3-deficient medium also exhibits a low biomass productivity of 0.143 ± 0.012 g/L/day. The Spirulina cultivation in NaHCO3-deficient medium survived the 10 days of cultivation. NaHCO3 is an essential nutrient for Spirulina cultivation because it contributes carbon to the cultivation medium. Only Spirulina is able to utilize carbonate or bicarbonate, supplied in the form of salts (Becker, 1994). The mechanism of NaHCO3 utilization as an inorganic carbon source for microalgae differs from CO2 uptake. The bicarbonate transporters are embedded in the chloroplast envelope and plasma membrane in microalgal cells. Bicarbonate ions (HCO3−) are converted to CO2 by carbonic anhydrase in the periplasmic space, which is then absorbed and utilized by microalgal cells (Mondal et al., 2017). Moreover, when NaHCO3 dissociates in the water, it produces hydroxyl ions in the medium that help sustain a high pH 9-10 throughout the cultivation condition (Michael et al., 2019). Research has reported that Chlorella vulgaris, under nutrient-sufficient condition with NaHCO3 as the carbon source, was able to reach 1.92 g/L maximum cell density—almost twice the biomass (1.04 g/L) cultivated under aeration alone (Abedini Najafabadi et al., 2015). Spirulina was able to grow in NaHCO3-deficient medium but resulted in low biomass productivity. NaHCO3 exhibits a dual role which acts as a carbon source and maintains a high pH condition suitable for Spirulina cultivation.
Beyond NaNO3 and NaHCO3, other nutrients such as K2HPO4 also play an important role in Spirulina growth. K2HPO4 serves as a phosphorus source, which is essential for cellular metabolism such as energy conversion, photosynthesis, and signal transduction (Nyabuto et al., 2015). Previous studies have shown that optimizing phosphate concentrations have significant effects on chlorophyll, metabolite, and carotenoid content (Abd El-Monem et al., 2021). Moreover, phosphorus deficiency has been reported to reduce photosynthetic efficiency and protein synthesis due to irregular nucleic acid synthesis, while shifting the cell’s focus to the synthesis of storage products, including carbohydrates and hydrocarbons (Dorry et al., 2024). Therefore, the balance of nutrients such as NaNO3, NaHCO3, and K2HPO4 is crucial not only for biomass productivity but also for the synthesis and purity of high-value compounds such as phycocyanin.
Overall, the deficiency of each nutrient has been shown to have lower biomass productivity than a control (0.632 ± 0.039 g/L/day) that contains the complete Zarrouk medium. NaNO3 and NaHCO3 have been identified as the most important nutrient sources for Spirulina cultivation. Although Zarrouk has been an established medium for cultivating cyanobacteria since 1966, not much information has been released regarding the formulation of Zarrouk medium. Therefore, the current finding is important in understanding the nutrients of Zarrouk medium on the biomass productivity of Spirulina. Further research would optimize the concentration of NaNO3, NaHCO3, and other nutrients to achieve a cost-effective cultivation medium for Spirulina. Statistical ANOVA shows that nutrient deficiency has a significant effect (p < 0.05) on the biomass productivity of Spirulina.
Figure 2a shows the biomass concentration of Spirulina cultivated for 10 days before being subjected to different harvesting ratios (e.g., 10%, 20%, and 30%). Figure 2b shows the dry biomass weights of Spirulina harvested from Days 10–13 consecutively at different harvesting ratios (10%, 20%, and 30%). Only the 10% harvesting ratio shows a more consistent harvested dry biomass weight, ranging from 0.2016 g to 0.2222 g. With the 20% and 30% harvesting ratios, the harvested dry biomass decreases from 0.4566 g to 0.3344 g and 0.5671 g to 0.3635 g, respectively. A lower harvesting ratio resulted in longer hydraulic retention time in which higher biomass density would be accumulated; thus, more nutrients would be assimilated, and the biomass concentration would be able to recover in a day. However, when the harvesting ratio increases, lesser biomass density is accumulated, and thus lesser nutrients would be assimilated, resulting in decreases of harvested dry biomass weights over the four consecutive days. Min et al. (2011) reported that 25% and 33% harvesting ratios showed better nutrient removal than half of its harvesting rate.
Figure 2. Effect of harvesting ratio (10%, 20%, and 30%) on Spirulina cultivation: (a) biomass concentration, (b) harvested dry Spirulina biomass weight, (c) C-phycocyanin concentration, (d) allo-phycocyanin concentration, (e) C-phycocyanin purity, and (f) allo-phycocyanin purity.
The current study investigated the harvesting ratio, but further research may investigate the harvesting frequency, such as harvesting intervals of 1 , 3 , and 5 days on Spirulina cultivation. Cai et al. (2013) reported that the biomass productivity of Nannochloropsis salina increased from 87.4 to 132.1 mg/L/d when the harvesting interval reduced from 3° to 1 day. This is because the long harvesting interval used to increase the number of larger clumps would raise the self-shading of cells and interfere with light absorption efficiency and the photosynthetic efficiency of the microalgae due to the reduced biomass productivity (Ishika et al., 2021). To conclude, a low harvesting ratio and frequent harvesting would be a good harvesting strategy to produce a high amount of biomass.
The respective reported CPC and APC concentrations ranged from 139.413 to 172.317 mg/g and 46.667 to 59.286 mg/g (Figures 2c, 2d). The reported CPC and APC purities ranged from 0.930 to 1.111 and 0.409 to 0.501, respectively. Statistical ANOVA analysis showed no significant effect (p > 0.05) on phycocyanin concentration or purity, while the effect of harvesting ratio has a significant effect (p < 0.05) on the harvested dry biomass weight of Spirulina. Overall, the findings provided valuable insights on the impact of harvesting ratio on harvested dry biomass and phycocyanin accumulation in Spirulina.
Figure 3a shows the biomass concentration of Spirulina cultivated for 10 days before being subject to a harvesting ratio of 10%. An equal volume of NaNO3 (2.5 g/L, 3.5 g/L, and 4.5 g/L) was added back and cultivated for another 3 days to study its effect on biomass concentration, harvested dry biomass weight, and phycocyanin accumulation. After adding NaNO3, all four-batch cultivations showed a similar trend, with the biomass concentration continuing to increase from Days 10 to 12 but decreasing on Day 13. Therefore, the batch cultivations were stopped and harvested. Figure 3b shows the harvested dry biomass weight increasing slightly. On the other hand, the addition of 2.5 g/L of NaNO3 showed a significant increase in C-PC and APC concentration from 34.368 to 68.346 mg/g and 27.075 to 33.232 mg/g, respectively (Figure 3c). The control also showed significant increase in C-PC and APC concentration from 43.594 to 66.008 mg/g and 25.623 to 30.224 mg/g, respectively. However, the addition of 3.5 g/L of NaNO3 showed a significant decrease in C-PC concentration from 30.750 to 6.035 mg/g. Moreover, the addition of 4.5 g/L NaNO3 showed a slight increase in C-PC and APC concentration from 26.638 to 28.613 mg/g and 26.637 to 28.903 mg/g, respectively.
Figure 3. Effect of NaNO3 addition (2.5 g/L, 3.5 g/L, and 4.5 g/L) on Spirulina cultivation: (a) biomass concentration, (b) harvested dry Spirulina biomass weight, (c) C-phycocyanin concentration, (d) allo-phycocyanin concentration (e) C-phycocyanin purity, and (f) allo-phycocyanin purity.
The current finding shows that both control and the addition of 2.5 g/L of NaNO3 increased phycocyanin accumulation. The control used Zarrouk medium to replace the 10% harvested Spirulina biomass. Instead of using the Zarrouk medium consisting of many chemicals, the current study shows that 2.5 g/L of NaNO3 only is capable of increasing phycocyanin accumulation. This is because phycocyanin is a type of pigment–protein complex, and NaNO3 is a nitrogen source that is responsible for the synthesis of proteins, nucleic acids, and chlorophyll in microalgae (Wu and Miao, 2014). Mousavi et al. (2022) found that medium supplemented with ammonium nitrogen sources have a slightly better growth rate than nitrogen sources (i.e., NaNO3), suggesting that ammonium plays a key role in supplying cells with the nitrogen source.
However, the concentration of 3.5 g/L NaNO3 onward showed no significant increase in phycocyanin accumulation. Sakawduan et al. (2019) reported a similar trend, with CPC concentration increasing from 25.5 to 44.59 mg/L/d when NaNO3 concentration increases from 1.5 to 3.5 g/L. Conversely, the CPC concentration decreases to 8.79 mg/L/d at 4.5 g/L of NaNO3. Vieira Costa et al. (2001) reported that when NaNO3 increases from 0.85 g/L to 2.55 g/L, the final biomass concentration increases from 1.559 g/L to 1.992 g/L, but the final biomass concentration decreases to 1.628 g/L when the NaNO3 is 4.25 g/L. It can be concluded that the tolerance level of NaNO3 concentration is approximately 3.5 g/L. Manirafasha et al. (2018) suggested that the nitrate concentration in culture should be kept between 1.2 and 1.6 g/L for high phycocyanin accumulation. It also worth noting that Sakawduan et al. (2019) and Mousavi et al. (2022) studied the effect of nitrogen sources during the active cultivation cycle whereas the current study investigated the effect of NaNO3 on the post-cultivation cycle. Further research is needed to explore alternative nitrogen sources (i.e., urea, ammonium nitrate, and ammonium sulfate) on the post-cultivation cycle and to optimize the conditions to achieve the maximum phycocyanin accumulation in Spirulina cultures.
The current finding has demonstrated that the addition of 2.5 g/L of NaNO3 after post-cultivation has the ability to enhance phycocyanin accumulation in Spirulina microalgae. This strategy offers a cost-effective solution for the microalgae industry by reducing the use of chemicals in achieving a continuous cultivation process. Statistical ANOVA analysis shows that there was no significant effect (p > 0.05) on phycocyanin purity, while the effect of harvesting ratio has significant effect (p < 0.05) on the harvested dry biomass weight of Spirulina.
Over the course of a 10-day cultivation period, the biomass concentration of Spirulina in 1-L and 10-L batch cultivations was determined to be 2.887 g/L and 2.431 g/L, respectively (Figure 4a. The biomass productivity of 1-L and 10-L batch cultivations was found to be 0.2284 g/L/d and 0.2122 g/L/d, respectively (Figure 4b). These findings suggest that the smaller scale 1-L system is more productive than the larger 10-L system, despite the latter having a larger volume for cultivation. When cultivating microalgae on a large scale, challenges arise, such as ensuring equal light distribution and mixing, providing sufficient gas exchange, and addressing the accumulation of soluble algal products in the culture medium (Jerney and Spilling, 2020).
Figure 4. Comparison of 1-L and 10-L Spirulina cultivation: (a) biomass concentration and (b) biomass productivity.
To address the scaling challenges in larger scale systems, several engineering solutions can be implemented to enhance biomass productivity. First, to ensure more even light distribution across the culture, the use of light-emitting diodes (LEDs) with optimized wavelengths, light reflectors, or diffusing layers can improve light penetration and uniformity (Mitchell et al., 2015). For better mixing and agitation, the optimization of impeller designs, incorporation of airlift pumps, or utilization of paddle-wheel mixers can help maintain culture homogeneity while minimizing shear stress (Kunjapur and Eldridge, 2010). Gas exchange can be improved by integrating spargers, air diffusers, or coiled tubing to facilitate efficient CO2 diffusion and oxygen removal (Janssen et al., 2003). Additionally, managing the accumulation of soluble algal products can be addressed through real-time monitoring and automated harvesting systems, which remove excess biomass or regulate nutrient levels (Lim et al., 2022). By incorporating these engineering solutions, the scaling challenges in larger systems can be mitigated, leading to improved productivity and efficiency in large-scale microalgae cultivation.
Figure 5a shows the biomass concentration of 1-L and 10-L Spirulina batch cultivations. The 1-L batch was cultivated for 10 days while the 10-L batch was cultivated for 11 days before being harvested for 3 consecutive days. Figure 5b demonstrates the results of 10% harvesting from the 10-L batch culture on Days 11, 12, 13, and 14 with a harvested dry biomass weight of 1.354 g, 1.088 g, 1.200 g, and 1.130 g, respectively. On Days 10, 11, 12, and 13, 10% of the 1-L batch culture was harvested with a harvested dry biomass weight of 0.2222 g, 0.2016 g, 0.2120, and 0.2200 g. 10% from 10-L is 1-L in volume and 10% of 1-L is 0.1-L in volume. Thus the results of the 1-L harvested dry biomass weight multiplied by 10 are higher than the harvested dry biomass weight from 10-L culture. This has also shown that the biomass productivity in 1-L batch culture is higher than the 10-L batch culture.
Figure 5. Comparison of 1-L and 10-L Spirulina batch culture with 10% harvesting ratio: (a) biomass concentration, (b) harvested dry Spirulina biomass weight, (c) C-phycocyanin concentration, (d) allo-phycocyanin concentration (e) C-phycocyanin purity, and (f) allo-phycocyanin purity.
Figures 5c and d shows a comparison of CPC and APC concentration between 1-L and 10-L Spirulina batch culture. In the 10-L batch culture, the CPC content range was between 166.9 and 238.4 mg/g and the APC content range between 58.43 and 77.74 mg/g. On the other hand, in the 1-L batch culture, the CPC content ranged between 139.4 and 162.9 mg/g, and APC content ranged between 46.67 and 55.32 mg/g. The 10-L batch culture had higher CPC and APC content than the 1-L batch culture. However, the purity of both CPC and APC had no significant effect over the 4 days of the 10% harvesting ratio.
Figure 6 compares the effect of 2.5 g/L of NaNO3 addition between 1-L and 10-L Spirulina batch cultures. Figures 6a and b show CPC and APC concentrations before and after the addition of 2.5 g/L of NaNO3. The initial concentrations of CPC and APC in the 10-L batch culture were 218.76 mg/g and 69.38 mg/g, respectively, which increased to 232.08 mg/g and 72.79 mg/g. Meanwhile, the initial concentrations of CPC and APC in the 1-L batch culture were 34.37 mg/g and 27.07 mg/g, respectively, which significantly increased to 68.35 mg/g and 33.23 mg/g. Both 1-L and 10-L batch cultures showed a significant increase in phycocyanin accumulation due to the addition of 2.5 g/L of NaNO3. Surprisingly, the 10-L batch culture had higher phycocyanin accumulation than the 1-L batch.
Figure 6. Comparison of 1-L and 10-L Spirulina cultivation with the addition of 2.5 g/L of NaNO3: (a) C-phycocyanin concentration, (b) allo-phycocyanin concentration (c) C-phycocyanin purity, and (d) allo-phycocyanin purity.
Although the addition of NaNO3 resulted an increase in both CPC and APC concentration and APC purity, it caused the purity of CPC to decrease in both 1-L and 10-L batch cultures (Figure 6c). This would depend on whether the company favored quantity or quality in its end product. The current finding has demonstrated that the addition of NaNO3 can enhance the accumulation of phycocyanin in Spirulina microalgae, concurrently minimizing chemical usage. However, this approach may not be a sustainable long-term solution since it relies on raw materials extracted from the earth. Further research could explore an alternative nitrogen source that favors an increase in both phycocyanin concentration and purity.
Table 2 shows the operating costs analysis of Spirulina cultivation for 1-L and 10-L. The operating cost consists of electricity, water, and chemicals. The cost of electricity is assumed to be the same, using four LED light units of 18 W power each and one air pump unit with a power of 30 W, for both ten 1-L Schott bottles and one 10-L cultivation tank. The cost of water was based on statistics provided by Air Selangor Sdn. Bhd., Malaysia (Air Selangor, 2022). Chemicals costs were based on lab-grade chemical prices (Table 3. The operating costs for both 1-L and 10-L batch cultures was $3.25. The largest expense was electricity at $2.05, followed by chemicals at $1.20. The biomass concentration of Spirulina microalgae was higher in the 1-L batch culture, with 2.887 g/L compared to 2.431 g/L in the 10-L batch culture. As a result, the cost of producing 1 kg of Spirulina biomass is $ 75.59 in the 1-L batch culture and $ 89.77 in the 10-L batch culture. These results suggest that producing Spirulina microalgae in smaller volumes may be cheaper, as it produces a higher yield of dry biomass per liter of culture medium. However, the limitation of this brief calculation has yet to consider the capital cost. The capital cost of ten 1-L vessels could be higher than fabricating a 10-L vessel. Overall, the current findings provide a brief insight into the economic feasibility of producing Spirulina biomass in 1-L and 10-L batch cultures. A more detailed economic feasibility of scaling-up Spirulina production for commercial scale should be assessed in future studies.
To improve cost efficiency in larger-scale operations, several cost-reduction strategies could be considered. One approach is integrating renewable energy solutions, such as solar-powered LED lighting combined with battery storage to reduce electricity costs associated with continuous lighting and aeration (Bahadur et al., 2013). Additionally, sourcing nutrients from food waste instead of lab-grade chemicals could significantly lower the cost of culture media while promoting sustainability (Ramandani et al., 2025). Engineering improvements, such as optimizing mixing, aeration, and light distribution in larger systems, could help bridge the productivity gap between small- and large-scale cultivation.
This study demonstrated a continuous cultivation and harvesting strategy for biomass and phycocyanin production in the biorefinery of Spirulina microalgae. The results show that a 10% harvesting ratio provided consistent weights of harvested dry biomass for three consecutive days. Furthermore, NaNO3 was found to be the most important nutrient for Spirulina cultivation, with the addition of 2.5 g/L significantly improving phycocyanin accumulation. The optimized parameters of a 10% harvesting ratio and 2.5 g/L of NaNO3 addition were tested in a 10-L batch culture, which showed decreased biomass productivity compared to the 1-L batch culture, resulting in higher production costs. However, future studies should assess the economic feasibility of scaling up Spirulina production for commercial use. Additionally, further research could optimize the concentration of NaNO3, NaHCO3, and other nutrients for a cost-effective cultivation medium, investigate harvesting frequency, and explore alternative nitrogen sources that increase phycocyanin concentration and purity.
The raw data supporting the conclusions of this article will be made available by the authors, without undue reservation.
HL: conceptualization, data curation, formal analysis, investigation, methodology, writing–original draft, and writing–review and editing. KK: conceptualization, project administration, resources, supervision, validation, and writing–review and editing. PS: conceptualization, funding acquisition, project administration, resources, supervision, validation, and writing–review and editing.
The authors declare that financial support was received for the research, authorship, and/or publication of this article. The authors gratefully acknowledge the financial support provided by Biolina Corporation Sdn. Bhd., Malaysia [NVEC0018].
The authors would also like to acknowledge the support of the recent algae initiative in gathering peers in algae research by launching an Algal Biotechnology Consortium (ABC), which is a platform for algae researchers to share, communicate, and outreach about their algae research with the intention of creating sustainable solutions and a greener future.
The authors declare that this study received funding from Biolina Corporation Sdn. Bhd. The funder provided Spirulina microalgae strains, financial support for the purchase of chemicals, and feedback/consultancy. The funder was involved in the study design, data collection and analysis, and interpretation of data but had no role in the writing of the manuscript or the decision to submit it for publication.
The authors declare that no generative AI was used in the creation of this manuscript.
All claims expressed in this article are solely those of the authors and do not necessarily represent those of their affiliated organizations, or those of the publisher, the editors and the reviewers. Any product that may be evaluated in this article, or claim that may be made by its manufacturer, is not guaranteed or endorsed by the publisher.
Abalde, J., Betancourt, L., Torres, E., Cid, A., and Barwell, C. (1998). Purification and characterization of phycocyanin from the marine cyanobacterium Synechococcus sp. IO9201. Plant Sci. 136, 109–120. doi:10.1016/s0168-9452(98)00113-7
Abd El-Monem, A. M., Gharieb, M. M., and Doman, K. M. (2021). Chemical constituents of zarrouk’s medium affect growth, pigments and metabolites productions of spirulina platensis Egypt. J. Bot. 61, 681–691. doi:10.21608/ejbo.2019.6052.1245
Abedini Najafabadi, H., Malekzadeh, M., Jalilian, F., Vossoughi, M., and Pazuki, G. (2015). Effect of various carbon sources on biomass and lipid production of Chlorella vulgaris during nutrient sufficient and nitrogen starvation conditions. Bioresour. Technol. 180, 311–317. doi:10.1016/j.biortech.2014.12.076
Air Selangor (2022). Water tariff information. Available online at: https://www2.airselangor.com/my-water-smart/water-tariff-information (Accessed March 15, 2023).
Amorim, M. L., Soares, J., Coimbra, J. S. D. R., Leite, M. D. O., Albino, L. F. T., and Martins, M. A. (2021). Microalgae proteins: production, separation, isolation, quantification, and application in food and feed. Crit. Rev. Food Sci. Nutr. 61, 1976–2002. doi:10.1080/10408398.2020.1768046
Bahadur, A., Zubair, M., and Khan, M. B. (2013). Design, construction and evaluation of solarized airlift tubular photobioreactor. J. Phys. Conf. Ser. 439, 012036. doi:10.1088/1742-6596/439/1/012036
Benner, P., Meier, L., Pfeffer, A., Krüger, K., Oropeza Vargas, J. E., and Weuster-Botz, D. (2022). Lab-scale photobioreactor systems: principles, applications, and scalability. Bioprocess Biosyst. Eng. 45, 791–813. doi:10.1007/s00449-022-02711-1
Bennett, A., and Bogorad, L. (1973). Complementary chromatic adaptation in A filamentous blue-green alga. J. Cell. Biol. 58, 419–435. doi:10.1083/jcb.58.2.419
Cai, T., Park, S. Y., Racharaks, R., and Li, Y. (2013). Cultivation of Nannochloropsis salina using anaerobic digestion effluent as a nutrient source for biofuel production. Appl. Energy 108, 486–492. doi:10.1016/j.apenergy.2013.03.056
Chen, C.-Y., Kao, P.-C., Tan, C. H., Show, P. L., Cheah, W. Y., Lee, W.-L., et al. (2016). Using an innovative pH-stat CO2 feeding strategy to enhance cell growth and C-phycocyanin production from Spirulina platensis. Biochem. Eng. J. 112, 78–85. doi:10.1016/j.bej.2016.04.009
Chew, K. W., Chia, S. R., Krishnamoorthy, R., Tao, Y., Chu, D.-T., and Show, P. L. (2019). Liquid biphasic flotation for the purification of C-phycocyanin from Spirulina platensis microalga. Bioresour. Technol. 288, 121519. doi:10.1016/j.biortech.2019.121519
Chew, K. W., Chia, S. R., Show, P. L., Ling, T. C., Arya, S. S., and Chang, J.-S. (2018). Food waste compost as an organic nutrient source for the cultivation of Chlorella vulgaris. Bioresour. Technol. 267, 356–362. doi:10.1016/j.biortech.2018.07.069
Dineshkumar, R., Narendran, R., and Sampathkumar, P. (2016). Cultivation of Spirulina platensis in different selective media. Indian J. Mar. Sci. 45, 1749–1754.
Dorry, A., Norastehnia, A., and Abedini, M. (2024). Effects of phosphorus removal and pH changes in the culture medium of spirulina sp. on the production rate of polyhydroxybutyrate. Curr. Appl. Sci. Technol., e0260240. doi:10.55003/cast.2024.260240
Dos Santos, R. R., Araújo, O. D. Q. F., De Medeiros, J. L., and Chaloub, R. M. (2016). Cultivation of Spirulina maxima in medium supplemented with sugarcane vinasse. Bioresour. Technol. 204, 38–48. doi:10.1016/j.biortech.2015.12.077
Elfar, O. A., Billa, N., Lim, H. R., Chew, K. W., Cheah, W. Y., Munawaroh, H. S. H., et al. (2022). Advances in delivery methods of Arthrospira platensis (spirulina) for enhanced therapeutic outcomes. Bioengineered 13, 14681–14718. doi:10.1080/21655979.2022.2100863
Fábregas, J., Domínguez, A., Regueiro, M., Maseda, A., and Otero, A. (2000). Optimization of culture medium for the continuous cultivation of the microalga Haematococcus pluvialis. Appl. Microbiol. Biotechnol. 53, 530–535. doi:10.1007/s002530051652
Fang, M., Jin, L., Zhang, C., Tan, Y., Jiang, P., Ge, N., et al. (2013). Rapid mutation of spirulina platensis by a new mutagenesis system of atmospheric and room temperature plasmas (ARTP) and generation of a mutant library with diverse phenotypes. PLoS one 8, e77046. doi:10.1371/journal.pone.0077046
Fernandes, B. D., Mota, A., Teixeira, J. A., and Vicente, A. A. (2015). Continuous cultivation of photosynthetic microorganisms: approaches, applications and future trends. Biotechnol. Adv. 33, 1228–1245. doi:10.1016/j.biotechadv.2015.03.004
Gao, F., Li, C., Yang, Z.-H., Zeng, G.-M., Feng, L.-J., Liu, J.-Z., et al. (2016). Continuous microalgae cultivation in aquaculture wastewater by a membrane photobioreactor for biomass production and nutrients removal. Ecol. Eng. 92, 55–61. doi:10.1016/j.ecoleng.2016.03.046
G-Bioscience (2019). Different types of extraction buffers and when to use them. Available online at: https://info.gbiosciences.com/blog/different-types-of-extraction-buffers-and-when-to-use-them#:∼:text=Here's%20how%20it%20helps%3A,other%20non%2Dsoluble%20cell%20components (Accessed November 11, 2022).
Huang, Q., Jiang, F., Wang, L., and Yang, C. (2017). Design of photobioreactors for mass cultivation of photosynthetic organisms. Engineering 3, 318–329. doi:10.1016/j.eng.2017.03.020
Ishika, T., Nwoba, E. G., Kwambai, C., and Moheimani, N. R. (2021). How harvesting frequency influence the biomass and lipid productivities of Nannochloropsis sp. Algal Res. 53, 102074. doi:10.1016/j.algal.2020.102074
Janssen, M., Tramper, J., Mur, L. R., and Wijffels, R. H. (2003). Enclosed outdoor photobioreactors: light regime, photosynthetic efficiency, scale-up, and future prospects. Biotechnol. Bioeng. 81, 193–210. doi:10.1002/bit.10468
Jerney, J., and Spilling, K. (2020). “Large scale cultivation of microalgae: open and closed systems,” in Biofuels from algae: methods and protocols. Editor K. Spilling (New York, NY: Springer New York).
Khoo, K. S., Ahmad, I., Chew, K. W., Iwamoto, K., Bhatnagar, A., and Show, P. L. (2023). Enhanced microalgal lipid production for biofuel using different strategies including genetic modification of microalgae: a review. Prog. Energy Combust. Sci. 96, 101071. doi:10.1016/j.pecs.2023.101071
Koyande, A. K., Chew, K. W., Lim, J.-W., Lee, S. Y., Lam, M. K., and Show, P.-L. (2019). Optimization of protein extraction from Chlorella Vulgaris via novel sugaring-out assisted liquid biphasic electric flotation system. Eng. Life Sci. 19, 968–977. doi:10.1002/elsc.201900068
Kunjapur, A. M., and Eldridge, R. B. (2010). Photobioreactor design for commercial biofuel production from microalgae. Ind. Eng. Chem. Res. 49, 3516–3526. doi:10.1021/ie901459u
Li, Y., Fei, X., and Deng, X. (2012). Novel molecular insights into nitrogen starvation-induced triacylglycerols accumulation revealed by differential gene expression analysis in green algae Micractinium pusillum. Biomass Bioenergy 42, 199–211. doi:10.1016/j.biombioe.2012.03.010
Lim, H. R., Khoo, K. S., Chew, K. W., Chang, C.-K., Munawaroh, H. S. H., Kumar, P. S., et al. (2021). Perspective of Spirulina culture with wastewater into a sustainable circular bioeconomy. Environ. Pollut. 284, 117492. doi:10.1016/j.envpol.2021.117492
Lim, H. R., Khoo, K. S., Chia, W. Y., Chew, K. W., Ho, S.-H., and Show, P. L. (2022). Smart microalgae farming with internet-of-things for sustainable agriculture. Biotechnol. Adv. 57, 107931. doi:10.1016/j.biotechadv.2022.107931
Manirafasha, E., Murwanashyaka, T., Ndikubwimana, T., Rashid Ahmed, N., Liu, J., Lu, Y., et al. (2018). Enhancement of cell growth and phycocyanin production in Arthrospira (Spirulina) platensis by metabolic stress and nitrate fed-batch. Bioresour. Technol. 255, 293–301. doi:10.1016/j.biortech.2017.12.068
Michael, A., Kyewalyanga, M. S., and Lugomela, C. V. (2019). Biomass and nutritive value of Spirulina (Arthrospira fusiformis) cultivated in a cost-effective medium. Ann. Microbiol. 69, 1387–1395. doi:10.1007/s13213-019-01520-4
Min, M., Wang, L., Li, Y., Mohr, M. J., Hu, B., Zhou, W., et al. (2011). Cultivating Chlorella sp. in a pilot-scale photobioreactor using centrate wastewater for microalgae biomass production and wastewater nutrient removal. Appl. Biochem. Biotechnol. 165, 123–137. doi:10.1007/s12010-011-9238-7
Mitchell, C. A., Dzakovich, M. P., Gomez, C., Lopez, R., Burr, J. F., Hernández, R., et al. (2015). Light-emitting diodes in horticulture. in Horticultural reviews. 1–87. doi:10.1002/9781119107781.ch01
Mondal, M., Ghosh, A., Oinam, G., Tiwari, O. N., Gayen, K., and Halder, G. N. (2017). Biochemical responses to bicarbonate supplementation on biomass and lipid productivity of Chlorella Sp. BTA9031 isolated from Coalmine area. Environ. Prog. Sustain. Energy 36, 1498–1506. doi:10.1002/ep.12594
Mousavi, M., Mehrzad, J., Najafi, M. F., Zhiani, R., and Shamsian, S. a. A. (2022). Nitrate and ammonia: two key nitrogen sources for biomass and phycocyanin production by Arthrospira (Spirulina) platensis. J. Appl. Phycol. 34, 2271–2281. doi:10.1007/s10811-021-02664-0
Narala, R. R., Garg, S., Sharma, K. K., Thomas-Hall, S. R., Deme, M., Li, Y., et al. (2016). Comparison of microalgae cultivation in photobioreactor, open raceway pond, and a two-stage hybrid system. Front. Energy Res. 4, 00029. doi:10.3389/fenrg.2016.00029
Nyabuto, D. K., Mariga, A. M., Kibue, G. W., Grace, W. K., Meilin, H., and Changhai, W. (2015). Growth performance and biochemical analysis of the genus spirulina under different physical and chemical environmental factors. Afr. J. Agric. Res. 10, 3614–3624. doi:10.5897/ajar2015.10210
Peter, A. P., Koyande, A. K., Chew, K. W., Ho, S.-H., Chen, W.-H., Chang, J.-S., et al. (2022). Continuous cultivation of microalgae in photobioreactors as a source of renewable energy: current status and future challenges. Renew. Sustain. Energy Rev. 154, 111852. doi:10.1016/j.rser.2021.111852
Ramandani, A. A., Sun, Y.-M., Lan, J. C.-W., Lim, J. W., Chang, J.-S., Srinuanpan, S., et al. (2025). Upcycling food waste as a low-cost cultivation medium for Chlorella sp. microalgae. J. Sci. Food Agric., 1–13. doi:10.1002/jsfa.13910
Sakawduan, K., Somkiat, J., Jaturapatr, V., Chanawat, N., and Kanjana, N. (2019). Kinetic models for phycocyanin production by fed batch cultivation of the spirulina platensis. GEOMATE J. 17, 187–194. doi:10.21660/2019.61.89205
Salunke, K., Magar, S., Joshi, R., and Wadikar, M. (2016). Comparative study on the growth of Spirulina platensis on different culture media. Biosci. Discov. 7, 90–92.
Singh, G., and Patidar, S. K. (2018). Microalgae harvesting techniques: a review. J. Environ. Manage. 217, 499–508. doi:10.1016/j.jenvman.2018.04.010
Ummalyma, S. B., Sirohi, R., Udayan, A., Yadav, P., Raj, A., Sim, S. J., et al. (2022). Sustainable microalgal biomass production in food industry wastewater for low-cost biorefinery products: a review. Phytochem. Rev. 22, 969–991. doi:10.1007/s11101-022-09814-3
Venkata Subhash, G., Rajvanshi, M., Raja Krishna Kumar, G., Shankar Sagaram, U., Prasad, V., Govindachary, S., et al. (2022). Challenges in microalgal biofuel production: a perspective on techno economic feasibility under biorefinery stratagem. Bioresour. Technol. 343, 126155. doi:10.1016/j.biortech.2021.126155
Vieira Costa, J. A., Cozza, K. L., Oliveira, L., and Magagnin, G. (2001). Different nitrogen sources and growth responses of Spirulina platensis in microenvironments. World J. Microbiol. Biotechnol. 17, 439–442. doi:10.1023/a:1011925022941
Keywords: harvesting ratio, Spirulina, phycocyanin, scale-up, harvesting strategy
Citation: Lim HR, Khoo KS and Show PL (2025) Impact of nutrient deficiency and harvesting strategy on biomass and phycocyanin production in Spirulina cultures. Front. Bioeng. Biotechnol. 13:1546801. doi: 10.3389/fbioe.2025.1546801
Received: 17 December 2024; Accepted: 21 February 2025;
Published: 24 March 2025.
Edited by:
Norio Nagao, Blue Scientific Shinkamitgoto Co., Ltd., JapanReviewed by:
Bikash Kumar, Indian Institute of Technology Indore, IndiaCopyright © 2025 Lim, Khoo and Show. This is an open-access article distributed under the terms of the Creative Commons Attribution License (CC BY). The use, distribution or reproduction in other forums is permitted, provided the original author(s) and the copyright owner(s) are credited and that the original publication in this journal is cited, in accordance with accepted academic practice. No use, distribution or reproduction is permitted which does not comply with these terms.
*Correspondence: Kuan Shiong Khoo, a3VhbnNoaW9uZy5raG9vQHNhdHVybi55enUuZWR1LnR3; Pau Loke Show, cGF1bG9rZS5zaG93QGt1LmFjLmFl
Disclaimer: All claims expressed in this article are solely those of the authors and do not necessarily represent those of their affiliated organizations, or those of the publisher, the editors and the reviewers. Any product that may be evaluated in this article or claim that may be made by its manufacturer is not guaranteed or endorsed by the publisher.
Research integrity at Frontiers
Learn more about the work of our research integrity team to safeguard the quality of each article we publish.