- 1College of Engineering, University of Tehran, Tehran, Iran
- 2Fertility and Infertility Research Center, Health Technology Institute, Kermanshah University of Medical Sciences, Kermanshah, Iran
- 3Department of Tissue Engineering, School of Medicine, Kermanshah University of Medical Sciences, Kermanshah, Iran
- 4Clinical Research Development Unit, Hajar Hospital, Shahrekord University of Medical Sciences, Shahrekord, Iran
- 5Medical Biology Research Center, Health Technology Institute, University of Medical Sciences, Kermanshah, Iran
- 6Cancer Research Center, Shahrekord University of Medical Sciences, Shahrekord, Iran
Lung diseases account for over four million premature deaths every year, and experts predict that this number will increase in the future. The top cause of death globally is diseases which include conditions like lung cancer asthma and COPD. Treating severe acute lung injury is a complex task because lungs struggle to heal themselves in the presence of swelling inflammation and scarring caused by damage, to the lung tissues. Though achieving lung regeneration, in controlled environments is still an ambition; ongoing studies are concentrating on notable progress, in the field of lung tissue engineering and methods for repairing lung damage. This review delves into methods, for regenerating lungs with a focus on exosome carry bioscaffolds and mesenchymal stem cells among others. It talks about how these new techniques can help repair lung tissue and improve lung function in cases of damage. Also noted is the significance of ex vivo lung perfusion (EVLP), for rejuvenating donor lungs and the healing properties of exosomes in supporting lung regeneration.
1 Introduction
Lung diseases account for over four million premature deaths annually, and their prevalence is expected to rise in the coming years. Chronic respiratory diseases, including lung cancer, asthma, and chronic obstructive pulmonary disease (COPD), together represent the third leading cause of death worldwide (Uthman, 2016). Repair of severe acute lung injury remains extremely challenging due to the lung’s limited ability to regenerate and the disordered environment formed by edema, inflammation, and fibrosis following lung disruption (Ma et al., 2018). Steady cell turnover helps the mature mammalian lung maintain homeostasis, and diverse cell populations are necessary for lung remodeling and renewal (Kotton and Morrisey, 2014). But following an injury, a specific subset of facultative lung progenitors is triggered to initiate remodeling, a process that repairs the damaged tissue. When this mechanism is disrupted, healing fibrosis develops, which results in scarring, aberrant lung regeneration, and compromised organ function (Beers and Morrisey, 2011; Wansleeben et al., 2013). For those with end-stage lung failure, lung transplantation (LTX) is still the only available treatment. Due to the limited number of tissue donors and viral contamination, an attempt has been made to construct the tissue using diverse cells and scaffolds, both natural and synthetic. This has resulted in the development of a new concept in science known as tissue engineering. As an academic field, tissue engineering has created a unique opportunity for the invention and refinement of therapeutic procedures for the treatment of congenital and acquired disorders, and its three basic pillars are scaffolds, growth factors, and cells. Scaffolds are a physical support and template for developing cells and tissues (Ashammakhi et al., 2022).
Bioengineered lung tissues are a new field of study that can help with this problem. While creating a fully functional bioengineered lung that might be transplanted is still difficult, new methods are being investigated to create ex vivo-engineered lung tissues that function and have the right gas exchange characteristics (Wagner et al., 2013). Despite advancements in organ preservation methods, particularly ex vivo lung perfusion (EVLP), new approaches are desperately needed to close the therapeutic gap in biological (acellular) lung scaffolds and increase the number of transplantable tissues available (Shakir et al., 2022). EVLP is a method that has revolutionized lung transplantation by improving the evaluation and use of donor lungs that might have been considered unfit, for transplant in the past. Before EVLP was introduced into practice numerous donor lungs with potential were rejected due to worries about their state leading to an organ utilization rate of 15–20 percent. Nevertheless, research indicates that approximately 40 percent of these declined lungs could be eligible for transplantation after undergoing further assessment, with EVLP. The importance of EVLP goes beyond evaluating lung viability as it plays a vital part, in revitalizing donor’s lungs that may be, on edge.
Increased Utilization: EVLP has greatly expanded the pool of useable donor lungs by offering a platform for further evaluation, enabling transplant centers to use organs previously thought to be unsuitable.
Decrease in Primary Graft Dysfunction (PGD): While PGD is still a risk, EVLP has been demonstrated to lessen its prevalence by enabling improved donor lung selection based on physiological data collected in real-time rather than only pre-transplant evaluations.
Identifying Biomarkers: EVLP offers the chance to find biomarkers that are indicative of post-transplant results, which will allow donor selection procedures to be further improved.
Therapeutic Interventions: According to recent research, pharmacological medicines or antibacterial therapies that are intended to enhance lung function before transplantation may be given during EVLP.
Exosomes are nanovesicles that carry bioactive molecules and play an important role in cell-to-cell communication (Record et al., 2014). They have important characteristics that make them superior to biological barriers and thus have a direct effect on various physiological and pathological processes (Rajput et al., 2022). Based on research, it has been determined that exosomes can be an effective mechanism in tissue regeneration, especially in lung tissue regeneration (Chen and Cai, 2023; Hong et al., 2019; Sen et al., 2023). Also, in research, exosomes that are derived from certain cells or tissues have been investigated as a cell-free approach for the treatment of lung diseases, which promotes tissue repair and regeneration by promoting interactions between different lung cell lineages and facilitating paracrine-mediated bioburden transport (Liu et al., 2019; Thomas et al., 2022; Zhang et al., 2019). For this reason, investigating the mechanisms of using exosomes as potential therapeutic agents for lung regenerative medicine is particularly important in tissue engineering and regenerative medicine (Hade et al., 2021). In recent years, the use of exosomes in the clinic has increased significantly (Figure 1).
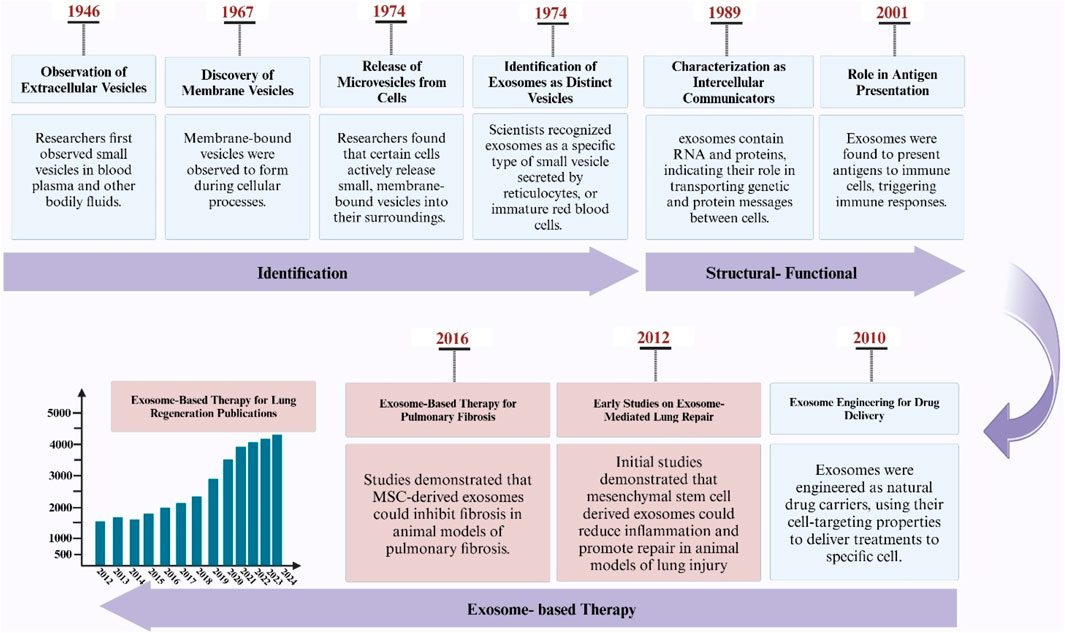
Figure 1. Illustration depicting the discovery timeline of exosomes, their application milestones in pulmonary research, and a PubMed-derived graph showing the increasing trend of exosome-related publications in lung studies.
2 Bio-scaffolds
2.1 Bioactive exosome-loaded scaffolds
Exosomes, also known as extracellular vesicles, play critical roles in a variety of biological processes, including cell proliferation, differentiation, and survival (Mantile et al., 2021). They originate from a variety of sources, including stem cells and immune cells, and are enclosed in a bilayer membrane to safeguard their genetic material and proteins before being delivered to target cells (Hong et al., 2019). Exosome-containing proteins include membrane transport proteins, tetraspanins, biogenesis-related proteins, and heat shock proteins (Vojtech et al., 2019). Scaffolds enriched with exosomes promise to be a potential strategy in regenerative medicine, particularly for lung tissue engineering (Antich-Rosselló et al., 2021). These scaffolds mix exosomes, which are extracellular vesicles necessary for intercellular communication, with biomaterial scaffolds to aid in tissue repair and regeneration (Re et al., 2021; Rahmati et al., 2023). The ability of exosome-loaded scaffolds to overcome the limitations of traditional cell therapies, like immune system rejection and decreased cell survival, is one of its key benefits (Gu et al., 2021).
Exosome-loaded bioactive scaffolds, which can be made into three-dimensional structures like bioactive glasses, offer a special cellular environment that can boost exosomes’ therapeutic potential and encourage tissue repair (Poongodi et al., 2021). The use of bioactive scaffolds can improve tissue repair and regeneration by promoting cell adhesion, proliferation, and differentiation (Pacelli et al., 2017) (Figure 2).
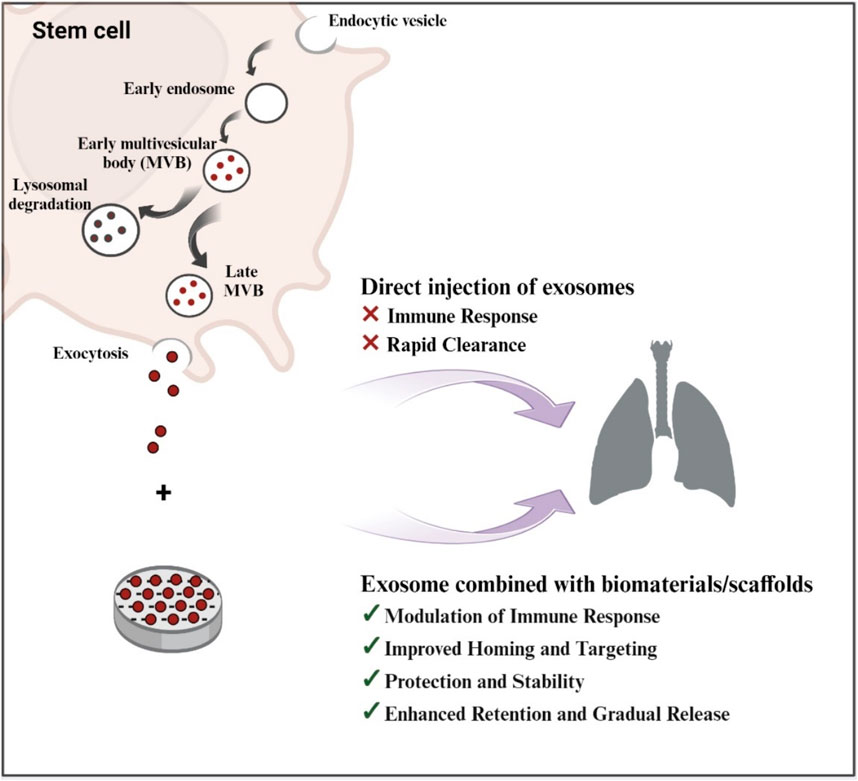
Figure 2. Schematic Illustration of exosome biogenesis and the benefits of exosome-loaded bio-scaffolds for lung regeneration (Created with BioRender.com).
To improve proangiogenic activity in bone healing, for instance, exosomes extracted from human bone marrow MSCs activated by dimethyloxaloylglycine were placed onto a porous hydroxyapatite scaffold (Vig and Fernandes, 2022). Similarly, exosomes extracted from human adipose-derived stem cells were combined with poly (lactic-co-glycolic acid) (PLGA)/polydopamine (pDA) structures to create a cell-free bone tissue engineering system (Torrecillas-Baena et al., 2023). Exosomes were released from the scaffold slowly and continuously, which promoted MSC migration and greatly enhanced bone repair (Swanson et al., 2020).
Recent developments have focused on the use of specific biomaterials and scaffolds to stimulate cells to increase exosome production and on the integration of exosomes into 3D scaffolds with different architectures for implantation into damaged tissue (Brennan et al., 2020). Researchers have also developed several methods to sustainably introduce exosomes into the environment after myocardial infarction (Bellin et al., 2019). For example, exosomes produced from cardiomyocyte-derived induced pluripotent stem cells and encapsulated in hydrogel patches were directly delivered into infarcted rats’ hearts (Cheng et al., 2021). Exosome patches increased ejection fraction, avoided cardiomyocytic hypertrophy, decreased ischemic injury, and improved heart repair (Ping et al., 2023). Bioactive scaffolds can enhance tissue repair and regeneration by stimulating cell adhesion, proliferation, and differentiation. Bioactive scaffolds can enhance tissue repair and regeneration by stimulating cell adhesion, proliferation, and differentiation (Stratton et al., 2016). Wang et al. discovered that stem cell transplants with a biomimetic scaffold promote lung recovery. To alter lung tissue, they used a scaffold loaded with exosomes from mesenchymal stem cells (MSC) (Wang et al., 2023). Exosomes were able to recruit and differentiate MSCs, resulting in the production of functional lung tissue (Wang et al., 2023).
Research by Yang and El Haj (2006) also highlighted the importance and value of designing and manufacturing scaffolds for cell and drug transfer, recognizing the need for biocompatible and biodegradable materials (Yang and El Haj, 2006). Huang et al. (2021) also discussed the practical importance of exosome-filled scaffolds in the tissue regeneration and repair process and emphasized the important role of exosomes in cell communication and tissue regeneration (Huang et al., 2021). Additionally, Goldberg investigated most nanostructured materials for biotechnology and drug delivery and suggested using them to create exosome-loaded structures (Goldberg et al., 2007).
2.1.1 Role of exosomes in donor lung regeneration
The therapeutic potential of exosomes in lung regeneration is supported by several studies demonstrating their ability to promote tissue repair, reduce inflammation, and enhance cellular function (Xie and Zeng, 2020). Research have shown that exosomes derived from lung spheroid cells (LSC-Exo) exhibit superior therapeutic benefits compared to those derived from mesenchymal stem cells (MSC-Exo) in models of pulmonary fibrosis (Dinh et al., 2020). Inhalation treatment with LSC-Exo significantly attenuated fibrosis induced by bleomycin and silica, restoring normal alveolar structure and decreasing the buildup of collagen and the growth of myofibroblasts appears to be a result of using exosomes in combating lung fibrosis. Moreover, exosomes have been shown to have inflammatory properties by influencing immune responses positively. In conclusion, Exosomes present an approach, to therapy with prospects, for improving the restoration of donor lungs. Their capability to enhance cell viability diminish inflammation and aid in tissue healing makes them crucial contributors to forthcoming approaches for lung ailments. Further exploration into the mechanisms and uses of exosomes will be crucial for creating treatments targeted at enhancing results for individuals, with impaired lung functionality.
Mesenchymal stem cell-derived exosomes can inhibit lung cancer cell proliferation and epithelial-mesenchymal transition (Wang et al., 2022). They could be achieved by delivering active cargoes such as miRNAs that directly target specific genes involved in tumor progression, such as MIER3 (Xiao-Ni et al., 2021). Exosomes also regulate processes such as cell movement, cell proliferation, cell phenotype, and cell growth (Xu et al., 2018). They have properties such as anti-angiogenic and anti-inflammatory, which significantly affect the tissue regeneration process (Olejarz et al., 2020). In addition, they can transport active miRNA and release anticancer drugs, which inhibit the growth of tumor cells and cause cell death (Fernandes Ribeiro et al., 2013).
One of the challenges associated with exosome-based bioscaffolds for lung tissue regeneration is the need to improve large-scale production of exosomes to overcome limitations including short cycle time and low targeting capacity (Lu et al., 2023). In addition, another important challenge is the stability and storage conditions of exosomes, which should be taken into account for their correct conversion into the clinical setting (Ana et al., 2021). It is important to closely monitor the biodistribution and pharmacokinetics of exosomes due to factors such as their size and diversity (Choi et al., 2021). Finally, engineering alpha-6 integrin-expressing exosomes to target lung epithelial cells the incorporation of tissue-specific components into exosomes can improve their targeting ability and thereby affect transmigration (Fedele et al., 2015).
Exosomes have important clinical applications, including the potential to provide important information in the early detection of lung cancer (Hu et al., 2020). They also have important properties that make them promising therapeutic agents for diseases such as lung cancer because MSCs as well as their exosomes offer advantages for regenerative medicine such as immunosuppressive and non-immunogenic effects (Reclusa et al., 2017). Exosome-loaded scaffolds have also been thoroughly investigated in research for lung tissue regeneration and repair (Su et al., 2021; Wang et al., 2024; Xie et al., 2021; Yan et al., 2020). Exosomes can also regulate the activity and proliferation of osteoclasts and make them suitable for the tissue regeneration process (Qin et al., 2016).
2.2 Cell-based scaffolds in lung regeneration
Cell-based scaffolds represent a new approach to tissue engineering that integrates cells into biodegradable and biocompatible scaffolds to mimic the extracellular matrix (Edgar et al., 2016). This mimicry provides structural support and biological, chemical, and mechanical signals that influence the development of new tissue (Barnes et al., 2017). Although cell-based scaffolds have shown promise in several applications, including lung regeneration, they also present challenges, such as the need for a reliable source of human-sized scaffolds and a definitive sterilization method (Tapias and Ott, 2014). Figure 3.
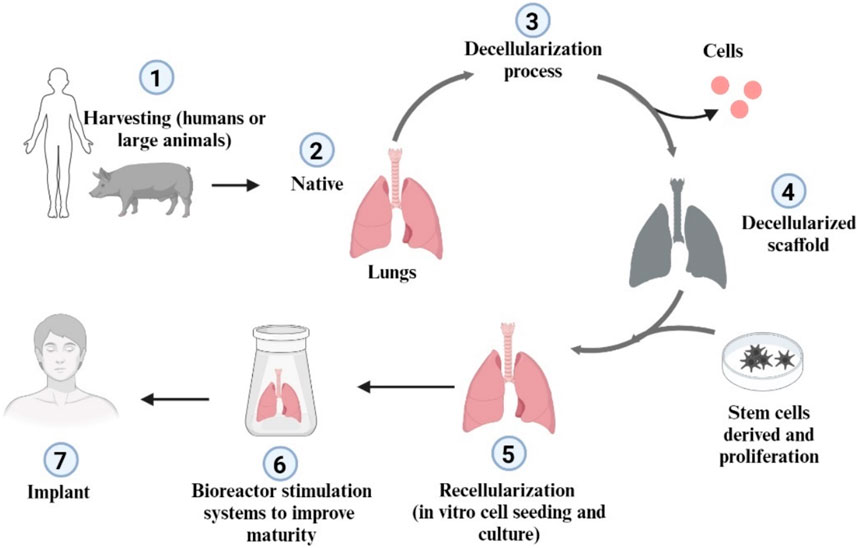
Figure 3. Schematic representation of lung decellularization and recellularization approach (Created with BioRender.com).
Furthermore, stem cell transplantation based on biomimetic scaffolds has been shown to promote lung regeneration (Willie et al., 2010). A three-dimensional artificial MSC implant based on a biomimetic scaffold was constructed to create a favorable regenerative environment for lung tissue (Wang et al., 2023). This approach could help overcome the problems associated with the shortage of organ donors and avoid the need for lung transplantation (Van Raemdonck et al., 2009). However, further research is needed to overcome the challenges associated with cell-based scaffolds, such as the need for a reliable source of human-sized scaffolds and a method for the final sterilization of the scaffolds (Griffin et al., 2018). Advances in artificial intelligence and stem cell transplantation based on biomimetic scaffolds open promising prospects for the future of lung regeneration (Zhang and Wang, 2019).
Recent advances in lung tissue engineering, including the development of smart biomaterials for scaffolds, and the use of on-chip organizational models to mimic the state of lung tissue, represent their important applications (Bennet et al., 2021; Kim et al., 2019). The development of smart biomaterials for scaffolds has also had a significant impact on the field of lung regenerative medicine (Aiman et al., 2022). These biomaterials actually activate certain tissue properties and can respond appropriately to changes in physiological conditions or external stimuli, in the same way that they have been used in various applications, including lung tissue repair and regeneration (Brovold et al., 2018). For example, Balestrini et al. (2015) looked at removing cells from pig pleural membranes to create a tissue engineering alternative to lung tissue repair (Balestrini et al., 2015). In another study by Kim et al. (2019), 3D artificial mesenchymal stem cell transplantation based on a biomimetic scaffold was used to create a positive regenerative niche for in situ lung regeneration from endogenous stem cells (Kim et al., 2019).
Lab-on-a-chip models are useful tools for simulating lung tissue conditions, especially in lung reconstructive medicine (Nichols et al., 2014). These models combine microfluidic systems and the cellular microenvironment to create constructs that mimic the structure and function of human organs and tissues while preserving dynamics (Li K. et al., 2019). Lung-on-a-chip models are especially used to summarize lung function at the level of organs in laboratory conditions, where this feature allows lung-on-a-chip models to simulate the special microenvironment of the lungs (Wang et al., 2021). Lung diseases such as cancer, lung infections, asthma, and pulmonary edema can be studied using lung-on-a-chip models (Jadhav et al., 2021). In addition, bionic lungs, which are self-assembled three-dimensional functional units, provide another application for mimicking lung tissue conditions, helping to study diseases and evaluate drug efficacy (Barreiro Carpio et al., 2021). Cell removal techniques were also used to manufacture bioprostheses (Do et al., 2022). The goal of this approach is to create patient-specific lungs for transplantation using cells derived from induced pluripotent stem cells (Wilkinson et al., 2017).
3 Hydrogel-based exosome delivery system
ECM of the lungs has been mimicked by artificial polymeric (Doryab et al., 2019). Scaffolds are crucial for tissue engineering (TE) development because they may be customized to imitate cellular microenvironments and provide structural and physicomechanical support, which can be used to moderate cellular physiology (Hussey et al., 2018). Three-dimensional macromolecular polymer networks called hydrogels offer a three-dimensional microenvironmenthat improves exosomes’ therapeutic potential (Frith et al., 2010). They have some viscosity and elasticity and can be created chemically or physically through the use of cross-linking agents (Lim et al., 2020). The use of hydrogels in biomedical applications have attracted a lot of attention because of their structural and mechanical similarities to numerous tissues (Maiz Fernández, 2021). A novel method of tissue engineering, especially for lung tissue regeneration, is represented by hydrogel-based exosome delivery devices (Xie et al., 2022). Exosomes are released by these systems in a regulated and controlled manner to maximize efficacy and reduce side effects. Exosomes are tiny extracellular vesicles that are loaded with certain lipids, proteins, and nucleic acids (Di Bella, 2022). They play a significant function in regulating intercellular communication and are exploited as transport vesicles for therapeutic applications (Cortes-Galvez et al., 2023). However, the stability and destiny of exosomes offer a substantial barrier to therapeutic uses, as they are degraded by the immune system immediately after injection in vivo (Ren et al., 2016). Therefore, the delivery method should be optimized to achieve high therapeutic efficacy and specificity, which means delivery of the desired exosomes to the target tissue (He et al., 2022). Biomaterials such as hydrogels allow exosomes to overcome poor tissue retention and provide a platform for controlled release to localize their activity (Pan et al., 2022).
3.1 Gelatin scaffold
Gelatin-based scaffolds have shown great promise in lung tissue engineering, as they mimic the natural ECM of lung tissue very well (Doryab and Schmid, 2022). These scaffolds, consisting of a gelatinous matrix, promote the growth and development of lung cells and therefore reflect the cellular microenvironment (Mahfouzi et al., 2021). This property makes gelatin-based scaffolds an ideal platform for research and the potential treatment of lung diseases (Bridge et al., 2018).
One way to improve the therapeutic potential of these scaffolds is to incorporate exosomes into the gelatinous matrix (Kang et al., 2023). Once implanted, these exosomes can be gradually released and provide cells with a continuous supply of nutrients and growth factors (Han et al., 2023). This method is successfully used to regenerate bone tissue, demonstrating the potential utility of gelatin-based scaffolds in tissue engineering beyond lung tissue (Guillén-Carvajal et al., 2023).
This gelatin scaffold exhibited desirable mechanical and degradation properties, flexibility, and cell adhesion (Stratton et al., 2016). Although gelatin-based scaffolds are very promising, other challenges remain, such as ensuring the long-term stability of the scaffolds, maintaining the integrity of the gelatin matrix, and optimizing the release rate of exosomes (Poongodi et al., 2021). Further research and development are needed to overcome these obstacles and maximize the potential of gelatin-based scaffolds for the treatment of lung diseases. In general, gelatin-based scaffolds, alone or in combination with other materials, are very promising for lung tissue development (Yuan et al., 2022). They have unique properties essential for optimal tissue function and have been shown to promote cell adhesion, proliferation and differentiation (Liu et al., 2022). Current research focuses on refining scaffold design and manufacturing and overcoming the challenges associated with integrating and controlling multiple nozzles in each cell (Giannitelli et al., 2014). Further studies are needed to fully understand the behavior of cells seeded on the scaffold and how they might integrate into the recipient nervous system. More supplementary information is also provided in Table 1 (Figure 4).
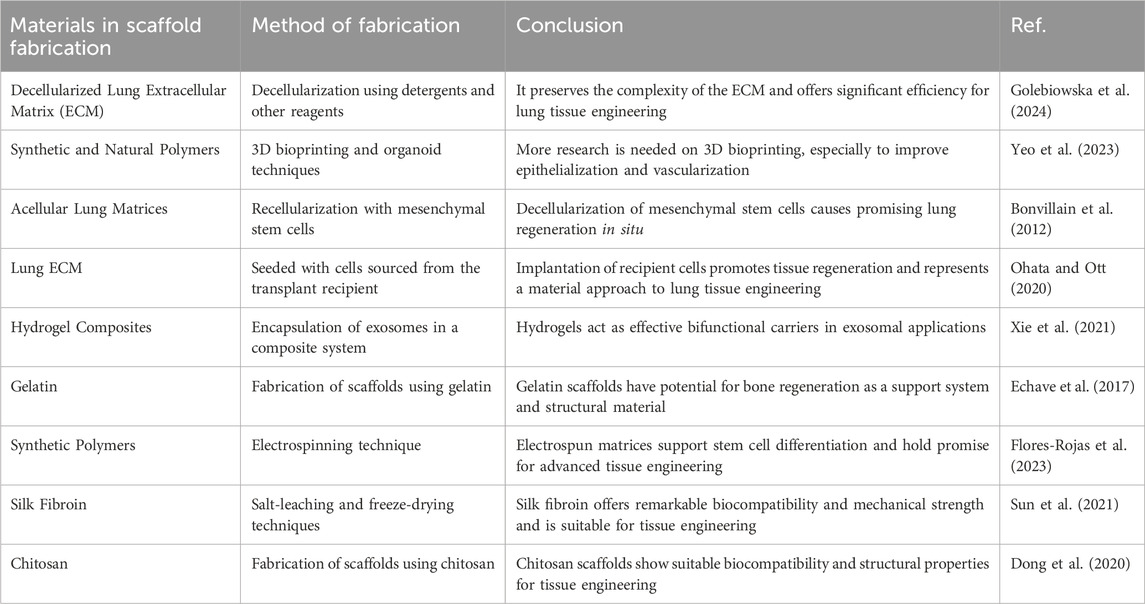
Table 1. Types of bio-scaffolds for regeneration of lung tissue: Materials, fabrication methods, and applications.
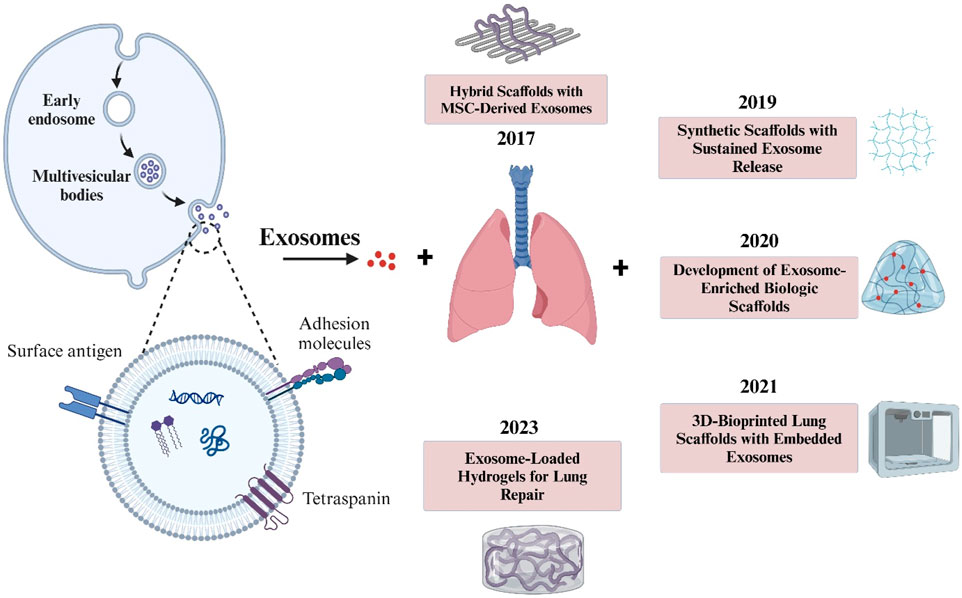
Figure 4. The illustration highlights key stages in exosome formation, from endocytosis to release, alongside notable advancements in scaffold-based lung therapies. This timeline underscores the evolving role of exosomes in tissue engineering for respiratory conditions.
4 Artificial lung scaffolds
Artificial lung structures are three-dimensional structures designed to mimic the architecture and function of natural lung tissue (Nichols et al., 2011). These structures are extremely important for tissue engineering and lung regeneration (Martins et al., 2007). They can be made from a variety of materials, including synthetic and natural polymers, and are designed to provide structural and physical support while mimicking the cellular microenvironment. One of the most essential aspects of these structures is their ability to renew the lung’s extracellular matrix (ECM) (Jiménez-Gastélum et al., 2019). The ECM is a complex network of proteins, lipids, and carbohydrates that provides structural support, controls cell development and differentiation, and facilitates cell-ECM interactions (Springer, 2019). Artificial lung scaffolds can create an appropriate environment for the growth and development of lung cells by utilizing the ECM function (Romero-López et al., 2017).
Despite the potential benefits of artificial lung structures, their development also poses challenges (Nichols et al., 2009). These include the identification of materials that mimic the ECM, provide physical support, and are biocompatible with the body (Qian et al., 2021). Other challenges include integrating cells into structures, maintaining the structural integrity of the structures, and preventing collapse (Turunen et al., 2018). The development of artificial lung scaffolds is hampered by their heterogeneity and potential xenogeneic problems, making it difficult to scale up this technique in a reproducible and regulated manner (Petrella and Spaggiari, 2018). However, artificial or synthetic lung scaffolds, which typically use synthetic and natural polymers, offer an alternative (Okamoto and John, 2013). One of the advantages of using synthetic materials for scaffold fabrication is the ability to tailor their biological and physical properties to achieve a desired scaffold (Turnbull et al., 2018). Despite advances in lung tissue engineering, limitations remain (Kim et al., 2015). These include the complex branched structure of the pulmonary airways and the challenge of poor biocompatibility and haemocompatibility, i.e., the ability to be compatible with living tissue and blood respectively (Bellet et al., 2021). Promising prototypes show that epithelialization and vascularization of grafts can be achieved using different methods (Singh et al., 2016). However, insufficient research has been conducted on 3D bioprinting and organoid techniques for parenchymal lung tissue (Louit et al., 2023). Figure 5.
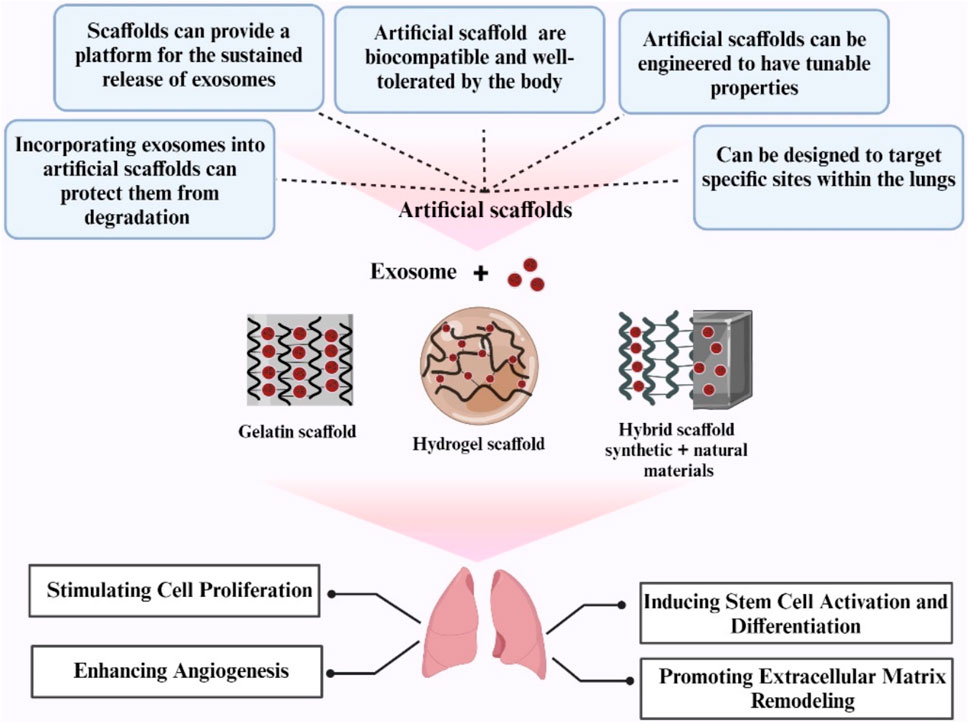
Figure 5. An overview of artificial scaffolds utilized in lung regeneration (Created with BioRender.com).
4.1 Hybrid scaffolds
In lung tissue engineering, significant progress has been made in scaffold manufacturing, with a focus on hybrid bioactive scaffolds (Edgar et al., 2016). These new scaffolds use a synthetic beta copolymer membrane with the ECM, creating a unique cellular microenvironment that supports tissue engineering in the lung (De Santis et al., 2018). Hybrid scaffolds are critical for tissue engineering as they provide essential properties such as strength, elasticity, nutrient transfer, and cellular remodeling, all of which are vital for optimal tissue functionality (Wasyłeczko et al., 2020). The use of hybrid materials in tissue engineering scaffolds in the lung is a growing area of research aimed at creating an optimal environment for tissue regeneration (Silva et al., 2010). The challenges and requirements for constructing such scaffolds are being resolved through interdisciplinary collaboration, and ongoing research focuses on perfecting scaffold design and manufacturing (Hollister, 2009). An important innovation in this area is the use of hybrid materials to build an optimized lung structure (Vallet-Regí et al., 2011). In this strategy, the favorable properties of two or more materials are combined to create a final scaffold that overcomes the limitations of each component (Pina et al., 2019). For example, the favorable properties of Acellular scaffolds for cell adhesion sites, organization, and differentiation signals combined with synthetic materials and advanced manufacturing techniques can lead to a desirable lung scaffold (Ratheesh et al., 2017).
Despite the promising potential of these hybrid scaffolds, it must be recognized that they represent an ambitious goal that requires further scientific research and development (Roseti et al., 2017). Ultimately, the goal is to create functional and biocompatible constructs for tissue regeneration, a challenge that must be addressed through in-depth research and experimentation (Leikeim, 2022). Furthermore, the integration of synthetic and natural materials into scaffolds not only promises to revolutionize the field of tissue engineering but also opens new avenues for the treatment of various lung diseases (Kim et al., 2015).
Lee et al. (2011) discovered that hybrid scaffolds that combine the mechanical strength of synthetic materials with the biological activity of natural materials have the potential to create an environment conducive to lung tissue growth and regeneration (Lee et al., 2011). Biodegradable polymers including poly (ε-caprolactone), polyethylene glycol, and poly (lactic-co-glycolic acid) are commonly used in hybrid scaffolds due to their mechanical stability and rapid decomposition. offers control (Lebourg et al., 2008). However, their natural fraction is frequently formed from extracellular matrix proteins and polysaccharides such as collagen, hyaluronic acid, and fibrin, all of which play critical roles in controlling cell activity and tissue integrity (Bindi et al., 2023). Electrospinning and 3D printing were among the advanced fabrication techniques used to fabricate these hybrid scaffolds with a specific microarchitecture and precise porosity (Abel et al., 2020). Although progress is being made in this area, more research is needed to address challenges such as long-term biocompatibility.
In general, over the past 2 decades, regenerative medicine has experienced significant technological advancements. Each milestone has introduced new methods that enhance tissue regeneration, scaffold design, and treatment efficiency. Table 2 outlines the chronological development of key technologies in this field, demonstrating how innovations have evolved to meet the growing demand for more effective and personalized therapeutic options.
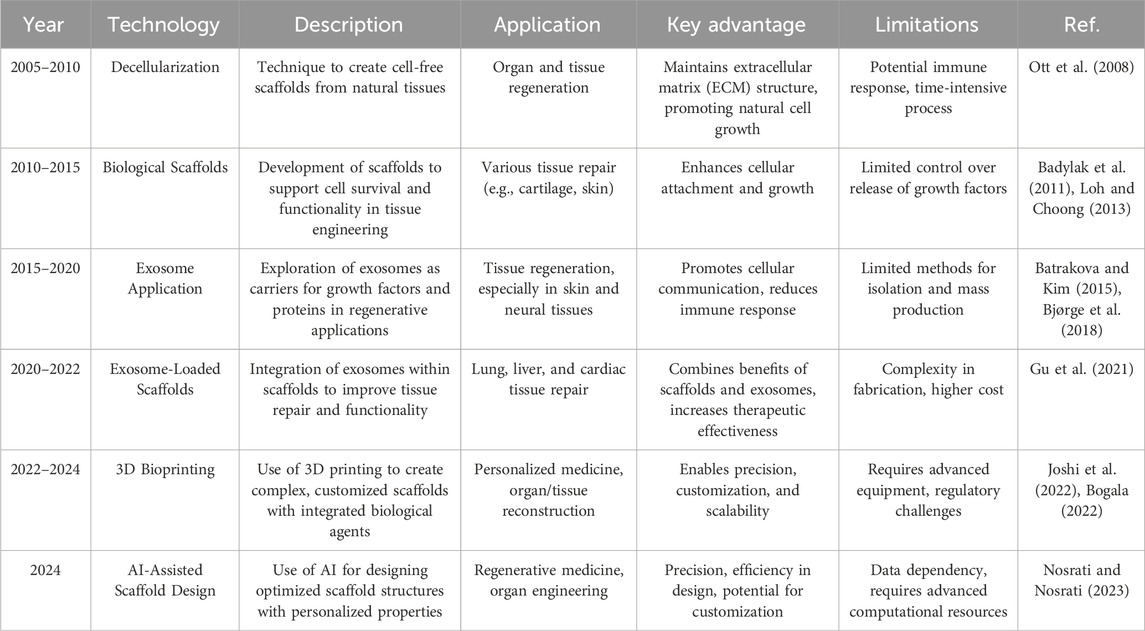
Table 2. Different strategies for exosome application in lung regeneration, and how methods have progressed and diversified.
5 Development of ex vivo transplantation
Transplantation of organs or tissues, outside the body, from a donor organism for evaluation and treatment before being transplanted into a recipient, is known as ex vivo transplantation. The process enables the assessment and improvement of organ viability and function before the actual transplant takes place to increase the likelihood of results.
In the field of lung regeneration, procedures like ex vivo transplantation are commonly used to evaluate donor lungs that may not meet transplant requirements by using methods such as EVLP (Ex Vivo Lung Perfusion). This approach enables professionals to assess the viability and function of these compromised lungs by perfusing them with a solution under controlled conditions outside the body before transplantation is considered further. During EVLP procedures donor lungs are maintained at a temperature. Perfused with a nutrient-rich solution, like the Steen solution, to simulate blood flow. This method aids in improving lung function and evaluating factors, like oxygen levels and flexibility along with the feasibility (Huang et al., 2023). Ex vivo transplant methods notably expand the supply of donor lungs by reconsidering organs that were previously rejected for transplantation suitability purposes. Moreover, they allow for interventions during the perfusion stage, like medication administration or tissue repairs which enhance the graft quality before it is implanted into a recipient. The use of ex vivo transplant techniques has demonstrated outcomes in enhancing transplant success rates and lowering issues linked to graft dysfunction (PGG). As studies advance in this area, Ex vivo methods might develop more by integrating cutting-edge treatments to improve organ retrieval and enhance patient outcomes post-transplantation.
A basic problem confronting the area of tissue engineering is the inability to create perfusable microvasculature networks capable of supporting tissue viability and withstanding physiological forces without leakage (Nichols et al., 2018). In small animal models, whole bioengineered lungs produced on acellular lung scaffolds were transplanted, but the lungs failed due to intravascular coagulation and endothelial barrier dysfunction, resulting in pulmonary edema (Song et al., 2011). There has been no strategy that has allowed for the long-term survival of bioengineered lungs following transplantation (Wang et al., 2023).
In vivo gas exchange is the main outcome of lung bioengineering research. Bioartificial lungs should be transplantable like cadaveric donor lungs, as the lung scaffolds maintain the vascular and airway architecture from the alveoli to the hilum. The viability of recellularized bioartificial lungs was first shown in a rat transplantation model (Ott et al., 2010; Petersen et al., 2010).
To prepare for human clinical trials, it is necessary to scale up from small animals to clinically relevant large animals. To achieve this goal, Zhou H and colleagues repopulated pig lung scaffolds with human basal airway stem cells and umbilical vascular endothelial cells, resulting in human-sized bioartificial lung grafts. Using the pulmonary trunk and left atrial appendage as anastomoses, respectively, the pulmonary artery and vein were heterotopically transplanted into these grafts. One hour after surgical implantation, the graft continued to function and tolerate normal pulmonary blood flow (Zhou et al., 2018).
5.1 Organoids in lung regeneration
The term “organoid,” meaning “resembling an organ,” was first used by Smith et al., in 1946 to describe an instance of cystic teratoma (Dutta et al., 2017). Organoids are three-dimensional, self-organizing are created by pluripotent or adult tissue stem cells. They provide an in vitro model for drug screening and replicate interactions between cells and niches in tissue formation, homeostasis, regeneration, and disease (Lu et al., 2021a). A unique organoid culture system has been developed due to the technological advancements in in vitro culture technology over the past decade. Organoid cultures have now been adapted to cells of many different epithelial lineages (Rosenbluth et al., 2020).
Lung organoids have been successfully generated using adult stem cells, human pluripotent stem cells (hPSCs), embryonic stem cells (ESCs), and induced pluripotent stem cells (iPSCs). However, recent improvements in lung organoid systems have focused on organoid findings, particularly those from distal airway stem/progenitor cells (Lu et al., 2021a).
Many human lung airways are lined with pseudostratified epithelium, which is composed of various cell types including airway basal cells, secretory cells, ciliated cells, tuft cells, and neuroendocrine cells. Basal cells are present throughout the airways of the human lungs, including the small bronchioles with a diameter of 1 mm. These basal cells constitute approximately 30% of the pseudostratified lung epithelium and are firmly attached to the basal lamina. During homeostasis and repair, they can self-renew and produce secretory and ciliated luminal cells (Lu et al., 2021b).
Lung organoids can originate from several types of cells: basal cells, alveolar type II cells, and airway secretory cells. The genes that are expressed characteristically in basal cells are Integrin α6, p63, cytokeratin 5 (Krt5), and nerve growth factor receptor (NGFR) (Lu et al., 2021b). The term “tracheosphere” refers to 3D organoids formed from trachea basal cells, and “bronchosphere” refers to organoids derived from bronchi or large airways (Barkauskas et al., 2017) According to Rock et al. (2009a) the first tracheosphere was formed in a week in Matrigel using Krt5-GFPpos murine tracheal basal cells, and it had a visible lumen. After 14 days, p63pos Krt5/Krt14pos basal cells formed the outer layer of a pseudostratified epithelium, whereas Krt8pos ductal secretory cells and ciliated cells formed the inner layer (Rock et al., 2009b) (Figure 6).
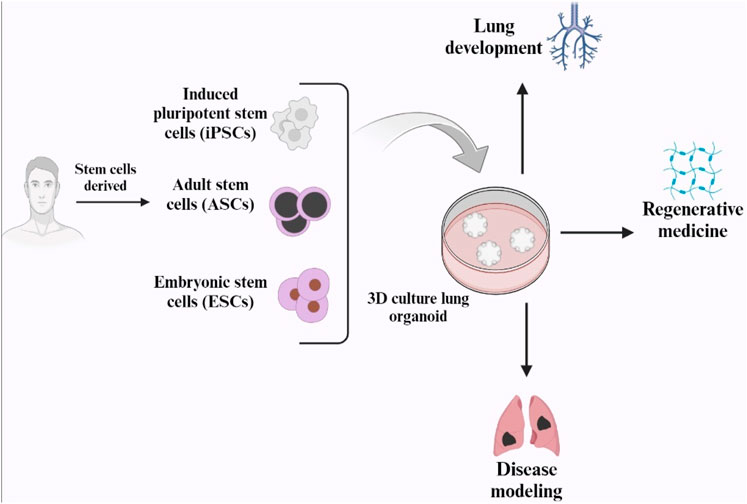
Figure 6. Schematic representation of human lung organoid and its applications (Created with BioRender.com).
6 Conclusions and future perspectives
The extracellular matrix of the lungs has various specialized cell types with a distinct architecture that must maintain compliance during respiration, making them difficult organs to engineer. On the other hand, limited production and isolation of exosomes remains a major problem that must be addressed to fully realize the potential of these exosome scaffolds for regenerative medicine (Hade et al., 2021). Furthermore, the short lifetime of exosomes in tissue after in vivo implantation remains a major challenge for clinical applications (Mastrolia et al., 2019). One approach to solving this issue is encapsulating exosomes in hydrogels which facilitate their ongoing diffusion into the damaged environment and thus improve their therapeutic effect (Chabria et al., 2021; Tracy et al., 2022). In conclusion, artificial lung constructs and hydrogel-based exosome delivery systems show promise for lung tissue engineering and regeneration (Deng et al., 2023). Types of bio-scaffolds for lung tissue regeneration are summarized in Table 1. The above-mentioned scaffold in this review has numerous applications in lung bioengineering and will lead to advancements in future transplants. However, further research and development are needed to overcome the challenges associated with their use, particularly in terms of material selection and also exosome stability and release (Li X. et al., 2019).
Development of a suitable framework sufficient to fully clarify the physiological and biochemical interactions is necessary before proceeding with the creation of transplantable tissues. Procedures for decellularization and recellularization must also be devised. It is also necessary to study the short- and long-term effects of these transplantable tissues in animal models prior to clinical trials (Taylor et al., 2014). Organoids represent a window of hope for lung regeneration. Lung organoids have proven to be an adaptable and useful tool for studying development, regeneration, homeostasis, and disease. Organoids are created by culturing different cells to represent chronic lung illnesses such as fibrosis and COPD. Future studies using newly separated cells from end-stage sick lungs will considerably increase our understanding of human lung illnesses, allowing for drug development and patient-specific treatment response monitoring.
Author contributions
MT: Conceptualization, Data curation, Writing–review and editing. LR: Data curation, Methodology, Writing–review and editing. ZH: Data curation, Methodology, Writing–review and editing. AM: Data curation, Investigation, Writing–review and editing. SR: Writing–original draft, Writing–review and editing.
Funding
The author(s) declare that no financial support was received for the research, authorship, and/or publication of this article.
Acknowledgments
The authors would like to thank the Cancer Research Center, Shahrekord University of Medical Sciences. This study was carried out under the approval code (IR.SKUMS.REC.1402.145) at Shahrekord University of Medical Sciences, Shahrekord, Iran.
Conflict of interest
The authors declare that the research was conducted in the absence of any commercial or financial relationships that could be construed as a potential conflict of interest.
Generative AI statement
The authors declare that no Generative AI was used in the creation of this manuscript.
Publisher’s note
All claims expressed in this article are solely those of the authors and do not necessarily represent those of their affiliated organizations, or those of the publisher, the editors and the reviewers. Any product that may be evaluated in this article, or claim that may be made by its manufacturer, is not guaranteed or endorsed by the publisher.
References
Abel, S. B., Ballarin, F. M., and Abraham, G. A. (2020). Combination of electrospinning with other techniques for the fabrication of 3D polymeric and composite nanofibrous scaffolds with improved cellular interactions. Nanotechnology 31 (17), 172002. doi:10.1088/1361-6528/ab6ab4
Aiman, A. R., Vigneswari, S., Amran, N. A., Murugaiyah, V., Amirul, A. A., and Ramakrishna, S. (2022). Advancing regenerative medicine through the development of scaffold, cell biology, biomaterials and strategies of smart material. Regen. Eng. Transl. Med. 8, 298–320. doi:10.1007/s40883-021-00227-w
Ana, I. D., Barlian, A., Hidajah, A. C., Wijaya, C. H., Notobroto, H. B., and Kencana Wungu, T. D. (2021). Challenges and strategy in treatment with exosomes for cell-free-based tissue engineering in dentistry. Future Sci. OA 7 (10), FSO751. doi:10.2144/fsoa-2021-0050
Antich-Rosselló, M., Forteza-Genestra, M. A., Monjo, M., and Ramis, J. M. (2021). Platelet-derived extracellular vesicles for regenerative medicine. Int. J. Mol. Sci. 22 (16), 8580. doi:10.3390/ijms22168580
Ashammakhi, N., GhavamiNejad, A., Tutar, R., Fricker, A., Roy, I., Chatzistavrou, X., et al. (2022). Highlights on advancing Frontiers in tissue engineering. Tissue Eng. Part B Rev. 28 (3), 633–664. doi:10.1089/ten.teb.2021.0012
Badylak, S. F., Taylor, D., and Uygun, K. (2011). Whole-organ tissue engineering: decellularization and recellularization of three-dimensional matrix scaffolds. Annu. Rev. Biomed. Eng. 13 (1), 27–53. doi:10.1146/annurev-bioeng-071910-124743
Balestrini, J. L., Gard, A. L., Liu, A., Leiby, K. L., Schwan, J., Kunkemoeller, B., et al. (2015). Production of decellularized porcine lung scaffolds for use in tissue engineering. Integr. Biol. 7 (12), 1598–1610. doi:10.1039/c5ib00063g
Barkauskas, C. E., Chung, M. I., Fioret, B., Gao, X., Katsura, H., and Hogan, B. L. M. (2017). Lung organoids: current uses and future promise. Development 144 (6), 986–997. doi:10.1242/dev.140103
Barnes, J. M., Przybyla, L., and Weaver, V. M. (2017). Tissue mechanics regulate brain development, homeostasis and disease. J. Cell Sci. 130 (1), 71–82. doi:10.1242/jcs.191742
Barreiro Carpio, M., Dabaghi, M., Ungureanu, J., Kolb, M. R., Hirota, J. A., and Moran-Mirabal, J. M. (2021). 3D bioprinting strategies, challenges, and opportunities to model the lung tissue microenvironment and its function. Front. Bioeng. Biotechnol. 9, 773511. doi:10.3389/fbioe.2021.773511
Batrakova, E. V., and Kim, M. S. (2015). Using exosomes, naturally-equipped nanocarriers, for drug delivery. J. Control. Release 219, 396–405. doi:10.1016/j.jconrel.2015.07.030
Beers, M. F., and Morrisey, E. E. (2011). The three R’s of lung health and disease: repair, remodeling, and regeneration. J. Clin. investigation 121 (6), 2065–2073. doi:10.1172/jci45961
Bellet, P., Gasparotto, M., Pressi, S., Fortunato, A., Scapin, G., Mba, M., et al. (2021). Graphene-based scaffolds for regenerative medicine. Nanomaterials 11 (2), 404. doi:10.3390/nano11020404
Bellin, G., Gardin, C., Ferroni, L., Chachques, J. C., Rogante, M., Mitrečić, D., et al. (2019). Exosome in cardiovascular diseases: a complex world full of hope. Cells 8 (2), 166. doi:10.3390/cells8020166
Bennet, T. J., Randhawa, A., Hua, J., and Cheung, K. C. (2021). Airway-on-a-chip: designs and applications for lung repair and disease. Cells 10 (7), 1602. doi:10.3390/cells10071602
Bindi, B., Perioli, A., Melo, P., Mattu, C., and Ferreira, A. M. (2023). Bioinspired collagen/hyaluronic acid/fibrin-based hydrogels for soft tissue engineering: design, synthesis, and in vitro characterization. J. Funct. Biomaterials 14 (10), 495. doi:10.3390/jfb14100495
Bjørge, I., Kim, S. Y., Mano, J. F., Kalionis, B., and Chrzanowski, W. (2018). Extracellular vesicles, exosomes and shedding vesicles in regenerative medicine–a new paradigm for tissue repair. Biomaterials Sci. 6 (1), 60–78. doi:10.1039/c7bm00479f
Bogala, M. R. (2022). Three-dimensional (3D) printing of hydroxyapatite-based scaffolds: a review. Bioprinting 28, e00244. doi:10.1016/j.bprint.2022.e00244
Bonvillain, R. W., Danchuk, S., Sullivan, D. E., Betancourt, A. M., Semon, J. A., Eagle, M. E., et al. (2012). A nonhuman primate model of lung regeneration: detergent-mediated decellularization and initial in vitro recellularization with mesenchymal stem cells. Tissue Eng. Part A 18 (23-24), 2437–2452. doi:10.1089/ten.tea.2011.0594
Brennan, M. Á., Layrolle, P., and Mooney, D. J. (2020). Biomaterials functionalized with MSC secreted extracellular vesicles and soluble factors for tissue regeneration. Adv. Funct. Mater. 30 (37), 1909125. doi:10.1002/adfm.201909125
Bridge, J. C., Amer, M., Morris, G. E., Martin, N. R. W., Player, D. J., Knox, A. J., et al. (2018). Electrospun gelatin-based scaffolds as a novel 3D platform to study the function of contractile smooth muscle cells in vitro. Biomed. Phys. and Eng. Express 4 (4), 045039. doi:10.1088/2057-1976/aace8f
Brovold, M., Almeida, J. I., Pla-Palacín, I., Sainz-Arnal, P., Sánchez-Romero, N., Rivas, J. J., et al. (2018). Naturally-derived biomaterials for tissue engineering applications. Adv. Exp. Med. Biol. 1077, 421–449. doi:10.1007/978-981-13-0947-2_23
Chabria, Y., Duffy, G., Lowery, A., and Dwyer, R. (2021). Hydrogels: 3D drug delivery systems for nanoparticles and extracellular vesicles. Biomedicines 9 (11), 1694. doi:10.3390/biomedicines9111694
Chen, Y.-X., and Cai, Q. (2023). Plant exosome-like nanovesicles and their role in the innovative delivery of RNA therapeutics. Biomedicines 11 (7), 1806. doi:10.3390/biomedicines11071806
Cheng, J., Chen, Z., Liu, C., Zhong, M., Wang, S., Sun, Y., et al. (2021). Bone mesenchymal stem cell-derived exosome-loaded injectable hydrogel for minimally invasive treatment of spinal cord injury. Nanomedicine 16 (18), 1567–1579. doi:10.2217/nnm-2021-0025
Choi, H., Choi, Y., Yim, H. Y., Mirzaaghasi, A., Yoo, J. K., and Choi, C. (2021). Biodistribution of exosomes and engineering strategies for targeted delivery of therapeutic exosomes. Tissue Eng. Regen. Med. 18 (4), 499–511. doi:10.1007/s13770-021-00361-0
Cortes-Galvez, D., Dangerfield, J. A., and Metzner, C. (2023). Extracellular vesicles and their membranes: exosomes vs. Virus-related particles. Membranes 13 (4), 397. doi:10.3390/membranes13040397
Deng, S., Cao, H., Cui, X., Fan, Y., Wang, Q., and Zhang, X. (2023). Optimization of exosome-based cell-free strategies to enhance endogenous cell functions in tissue regeneration. Acta Biomater. 171, 68–84. doi:10.1016/j.actbio.2023.09.023
De Santis, M. M., Bölükbas, D. A., Lindstedt, S., and Wagner, D. (2018). How to build a lung: latest advances and emerging themes in lung bioengineering. Eur. Respir. J. 52 (1), 1601355. doi:10.1183/13993003.01355-2016
Di Bella, M. A. (2022). Overview and update on extracellular vesicles: considerations on exosomes and their application in modern medicine. Biology 11 (6), 804. doi:10.3390/biology11060804
Dinh, P. U. C., Paudel, D., Brochu, H., Popowski, K. D., Gracieux, M. C., Cores, J., et al. (2020). Inhalation of lung spheroid cell secretome and exosomes promotes lung repair in pulmonary fibrosis. Nat. Commun. 11 (1), 1064. doi:10.1038/s41467-020-14344-7
Do, T., Synan, L., Ali, G., and Gappa-Fahlenkamp, H. (2022). 3D tissue-engineered lung models to study immune responses following viral infections of the small airways. Stem Cell Res. and Ther. 13 (1), 464. doi:10.1186/s13287-022-03134-1
Dong, X., Cheng, Q., Long, Y., Xu, C., Fang, H., Chen, Y., et al. (2020). A chitosan based scaffold with enhanced mechanical and biocompatible performance for biomedical applications. Polym. Degrad. Stab. 181, 109322. doi:10.1016/j.polymdegradstab.2020.109322
Doryab, A., and Schmid, O. (2022). Bioactive cell-derived ECM scaffold forms a unique cellular microenvironment for lung tissue engineering. Biomedicines 10 (8), 1791. doi:10.3390/biomedicines10081791
Doryab, A., Tas, S., Taskin, M. B., Yang, L., Hilgendorff, A., Groll, J., et al. (2019). Evolution of bioengineered lung models: recent advances and challenges in tissue mimicry for studying the role of mechanical forces in cell biology. Adv. Funct. Mater. 29 (39), 1903114. doi:10.1002/adfm.201903114
Dutta, D., Heo, I., and Clevers, H. (2017). Disease modeling in stem cell-derived 3D organoid systems. Trends Mol. Med. 23 (5), 393–410. doi:10.1016/j.molmed.2017.02.007
Echave, M. C., Sánchez, P., Pedraz, J., and Orive, G. (2017). Progress of gelatin-based 3D approaches for bone regeneration. J. Drug Deliv. Sci. Technol. 42, 63–74. doi:10.1016/j.jddst.2017.04.012
Edgar, L., McNamara, K., Wong, T., Tamburrini, R., Katari, R., and Orlando, G. (2016). Heterogeneity of scaffold biomaterials in tissue engineering. Materials 9 (5), 332. doi:10.3390/ma9050332
Fedele, C., Singh, A., Zerlanko, B. J., Iozzo, R. V., and Languino, L. R. (2015). The αvβ6 integrin is transferred intercellularly via exosomes. J. Biol. Chem. 290 (8), 4545–4551. doi:10.1074/jbc.c114.617662
Fernandes Ribeiro, M., Zhu, H., Millard, R. W., and Fan, G. C. (2013). Exosomes function in pro-and anti-angiogenesis. Curr. Angiogenes. 2 (1), 54–59. doi:10.2174/22115528113020020001
Flores-Rojas, G. G., Gómez-Lazaro, B., López-Saucedo, F., Vera-Graziano, R., Bucio, E., and Mendizábal, E. (2023). Electrospun scaffolds for tissue engineering: a review. Macromol 3 (3), 524–553. doi:10.3390/macromol3030031
Frith, J. E., Thomson, B., and Genever, P. G. (2010). Dynamic three-dimensional culture methods enhance mesenchymal stem cell properties and increase therapeutic potential. Tissue Eng. Part C. Methods 16 (4), 735–749. doi:10.1089/ten.tec.2009.0432
Giannitelli, S. M., Accoto, D., Trombetta, M., and Rainer, A. (2014). Current trends in the design of scaffolds for computer-aided tissue engineering. Acta biomater. 10 (2), 580–594. doi:10.1016/j.actbio.2013.10.024
Goldberg, M., Langer, R., and Jia, X. (2007). Nanostructured materials for applications in drug delivery and tissue engineering. J. Biomaterials Sci. Polym. Ed. 18 (3), 241–268. doi:10.1163/156856207779996931
Golebiowska, A. A., Intravaia, J. T., Sathe, V. M., Kumbar, S. G., and Nukavarapu, S. P. (2024). Decellularized extracellular matrix biomaterials for regenerative therapies: advances, challenges and clinical prospects. Bioact. Mater. 32, 98–123. doi:10.1016/j.bioactmat.2023.09.017
Griffin, M., Naderi, N., Kalaskar, D. M., Malins, E., Becer, R., Thornton, C. A., et al. (2018). Evaluation of sterilisation techniques for regenerative medicine scaffolds fabricated with polyurethane nonbiodegradable and bioabsorbable nanocomposite materials. Int. J. Biomaterials 2018, 1–14. doi:10.1155/2018/6565783
Gu, C., Feng, J., Waqas, A., Deng, Y., Zhang, Y., Chen, W., et al. (2021). Technological advances of 3D scaffold-based stem cell/exosome therapy in tissues and organs. Front. Cell Dev. Biol. 9, 709204. doi:10.3389/fcell.2021.709204
Guillén-Carvajal, K., Valdez-Salas, B., Beltrán-Partida, E., Salomón-Carlos, J., and Cheng, N. (2023). Chitosan, gelatin, and collagen hydrogels for bone regeneration. Polymers 15 (13), 2762. doi:10.3390/polym15132762
Hade, M. D., Suire, C. N., and Suo, Z. (2021). Mesenchymal stem cell-derived exosomes: applications in regenerative medicine. Cells 10 (8), 1959. doi:10.3390/cells10081959
Han, S., Yang, H., Ni, X., Deng, Y., Li, Z., Xing, X., et al. (2023). Programmed release of vascular endothelial growth factor and exosome from injectable chitosan nanofibrous microsphere-based PLGA-PEG-PLGA hydrogel for enhanced bone regeneration. Int. J. Biol. Macromol. 253, 126721. doi:10.1016/j.ijbiomac.2023.126721
He, J., Ren, W., Wang, W., Han, W., Jiang, L., Zhang, D., et al. (2022). Exosomal targeting and its potential clinical application. Drug Deliv. Transl. Res. 12 (10), 2385–2402. doi:10.1007/s13346-021-01087-1
Hollister, S. J. (2009). Scaffold engineering: a bridge to where? Biofabrication 1 (1), 012001. doi:10.1088/1758-5082/1/1/012001
Hong, P., Yang, H., Wu, Y., Li, K., and Tang, Z. (2019). The functions and clinical application potential of exosomes derived from adipose mesenchymal stem cells: a comprehensive review. Stem Cell Res. and Ther. 10, 242. doi:10.1186/s13287-019-1358-y
Hu, C., Meiners, S., Lukas, C., Stathopoulos, G. T., and Chen, J. (2020). Role of exosomal microRNAs in lung cancer biology and clinical applications. Cell Prolif. 53 (6), e12828. doi:10.1111/cpr.12828
Huang, J., Xiong, J., Yang, L., Zhang, J., Sun, S., and Liang, Y. (2021). Cell-free exosome-laden scaffolds for tissue repair. Nanoscale 13 (19), 8740–8750. doi:10.1039/d1nr01314a
Huang, L., Vellanki, R. N., Zhu, Z., Wouters, B. G., Keshavjee, S., and Liu, M. (2023). De novo design and development of a nutrient-rich perfusate for ex vivo lung perfusion with cell culture models. Int. J. Mol. Sci. 24 (17), 13117. doi:10.3390/ijms241713117
Hussey, G. S., Dziki, J. L., and Badylak, S. F. (2018). Extracellular matrix-based materials for regenerative medicine. Nat. Rev. Mater. 3 (7), 159–173. doi:10.1038/s41578-018-0023-x
Jadhav, S. P., Singh, H., Hussain, S., Gilhotra, R., Mishra, A., Prasher, P., et al. (2021). “Introduction to lung diseases,” in Targeting cellular Signalling pathways in lung diseases (Singapore: Springer), 1–25.
Jiménez-Gastélum, G. R., Aguilar-Medina, E. M., Soto-Sainz, E., Ramos-Payán, R., and Silva-Benítez, E. L. (2019). Antimicrobial properties of extracellular matrix scaffolds for tissue engineering. BioMed Res. Int. 2019, 1–7. doi:10.1155/2019/9641456
Joshi, A., Choudhury, S., Gugulothu, S. B., Visweswariah, S. S., and Chatterjee, K. (2022). Strategies to promote vascularization in 3D printed tissue scaffolds: trends and challenges. Biomacromolecules 23 (7), 2730–2751. doi:10.1021/acs.biomac.2c00423
Kang, Y., Xu, J., Meng, L., Su, Y., Fang, H., Liu, J., et al. (2023). 3D bioprinting of dECM/Gel/QCS/nHAp hybrid scaffolds laden with mesenchymal stem cell-derived exosomes to improve angiogenesis and osteogenesis. Biofabrication 15 (2), 024103. doi:10.1088/1758-5090/acb6b8
Kim, H., Bae, C., Kook, Y. M., Koh, W. G., Lee, K., and Park, M. H. (2019). Mesenchymal stem cell 3D encapsulation technologies for biomimetic microenvironment in tissue regeneration. Stem Cell Res. and Ther. 10, 51–14. doi:10.1186/s13287-018-1130-8
Kim, S. Y., Wong, A. H. M., Abou Neel, E. A., Chrzanowski, W., and Chan, H. K. (2015). The future perspectives of natural materials for pulmonary drug delivery and lung tissue engineering. Expert Opin. drug Deliv. 12 (6), 869–887. doi:10.1517/17425247.2015.993314
Kotton, D. N., and Morrisey, E. E. (2014). Lung regeneration: mechanisms, applications and emerging stem cell populations. Nat. Med. 20 (8), 822–832. doi:10.1038/nm.3642
Lebourg, M., Sabater Serra, R., Más Estellés, J., Hernández Sánchez, F., Gómez Ribelles, J. L., and Suay Antón, J. (2008). Biodegradable polycaprolactone scaffold with controlled porosity obtained by modified particle-leaching technique. J. Mater. Sci. Mater. Med. 19, 2047–2053. doi:10.1007/s10856-007-3282-4
Lee, H., Yeo, M., Ahn, S., Kang, D., Jang, C. H., Park, G. M., et al. (2011). Designed hybrid scaffolds consisting of polycaprolactone microstrands and electrospun collagen-nanofibers for bone tissue regeneration. J. Biomed. Mater. Res. Part B Appl. biomaterials 97 (2), 263–270. doi:10.1002/jbm.b.31809
Leikeim, A. (2022). Vascularization strategies for full-thickness skin equivalents to model melanoma progression.
Li, K., Yang, X., Xue, C., Zhao, L., Zhang, Y., and Gao, X. (2019a). Biomimetic human lung-on-a-chip for modeling disease investigation. Biomicrofluidics 13 (3), 031501. doi:10.1063/1.5100070
Li, X., Corbett, A. L., Taatizadeh, E., Tasnim, N., Little, J. P., Garnis, C., et al. (2019b). Challenges and opportunities in exosome research—perspectives from biology, engineering, and cancer therapy. Apl. Bioeng. 3 (1), 011503. doi:10.1063/1.5087122
Lim, K. S., Galarraga, J. H., Cui, X., Lindberg, G. C. J., Burdick, J. A., and Woodfield, T. B. F. (2020). Fundamentals and applications of photo-cross-linking in bioprinting. Chem. Rev. 120 (19), 10662–10694. doi:10.1021/acs.chemrev.9b00812
Liu, H., Chen, F., Zhang, Y., Wu, P., Yang, Z., Zhang, S., et al. (2022). Facile fabrication of biomimetic silicified gelatin scaffolds for angiogenesis and bone regeneration by a bioinspired polymer-induced liquid precursor. Mater. and Des. 222, 111070. doi:10.1016/j.matdes.2022.111070
Liu, S.-L., Sun, P., Li, Y., and Lu, Y. (2019). Exosomes as critical mediators of cell-to-cell communication in cancer pathogenesis and their potential clinical application. Transl. Cancer Res. 8 (1), 298–311. doi:10.21037/tcr.2019.01.03
Loh, Q. L., and Choong, C. (2013). Three-dimensional scaffolds for tissue engineering applications: role of porosity and pore size. Tissue Eng. Part B Rev. 19, 485–502. doi:10.1089/ten.teb.2012.0437
Louit, A., Galbraith, T., and Berthod, F. (2023). In vitro 3D modeling of neurodegenerative diseases. Bioengineering 10 (1), 93. doi:10.3390/bioengineering10010093
Lu, T., Cao, Y., Zhao, P., Shen, S., and Xi, Y. (2021a). Organoid: a powerful tool to study lung regeneration and disease. Cell Regen. 10 (1), 21–10. doi:10.1186/s13619-021-00082-8
Lu, T., Cao, Y., Zhao, P., Shen, S., and Xi, Y. (2021b). Organoid: a powerful tool to study lung regeneration and disease. Cell Regen. 10 (1), 21. doi:10.1186/s13619-021-00082-8
Lu, Y., Mai, Z., Cui, L., and Zhao, X. (2023). Engineering exosomes and biomaterial-assisted exosomes as therapeutic carriers for bone regeneration. Stem Cell Res. and Ther. 14 (1), 55. doi:10.1186/s13287-023-03275-x
Ma, Q., Ma, Y., Dai, X., Ren, T., Fu, Y., Liu, W., et al. (2018). Regeneration of functional alveoli by adult human SOX9+ airway basal cell transplantation. Protein and Cell 9 (3), 267–282. doi:10.1007/s13238-018-0506-y
Mahfouzi, S. H., Tali, S. H. S., and Amoabediny, G. (2021). 3D bioprinting for lung and tracheal tissue engineering: criteria, advances, challenges, and future directions. Bioprinting 21, e00124. doi:10.1016/j.bprint.2020.e00124
Maiz Fernández, S. (2021). Development of in situ forming, polysaccharide-based, self-healable and printable hydrogels por soft actuators and biomedical applications.
Mantile, F., Franco, P., Stoppelli, M. P., and Liguori, G. L. (2021). “Biological role and clinical relevance of extracellular vesicles as key mediators of cell communication in cancer,” in Advances in biomembranes and lipid self-assembly (Elsevier), 37–117.
Martins, A., Araújo, J. V., Reis, R. L., and Neves, N. M. (2007). Electrospun nanostructured scaffolds for tissue engineering applications. Nanomedicine (Lond) 2, 929–942. doi:10.2217/17435889.2.6.929
Mastrolia, I., Foppiani, E. M., Murgia, A., Candini, O., Samarelli, A. V., Grisendi, G., et al. (2019). Challenges in clinical development of mesenchymal stromal/stem cells: concise review. Stem cells Transl. Med. 8 (11), 1135–1148. doi:10.1002/sctm.19-0044
Nichols, J. E., La Francesca, S., Niles, J. A., Vega, S. P., Argueta, L. B., Frank, L., et al. (2018). Production and transplantation of bioengineered lung into a large-animal model. Sci. Transl. Med. 10 (452), eaao3926. doi:10.1126/scitranslmed.aao3926
Nichols, J. E., Niles, J. A., and Cortiella, J. (2009). Design and development of tissue engineered lung: progress and challenges. Organogenesis 5 (2), 57–61. doi:10.4161/org.5.2.8564
Nichols, J. E., Niles, J. A., and Cortiella, J. (2011). “Engineering complex synthetic organs,” in Tissue engineering in regenerative medicine (Springer), 297–313.
Nichols, J. E., Niles, J. A., Vega, S. P., Argueta, L. B., Eastaway, A., and Cortiella, J. (2014). Modeling the lung: design and development of tissue engineered macro-and micro-physiologic lung models for research use. Exp. Biol. Med. 239 (9), 1135–1169. doi:10.1177/1535370214536679
Nosrati, H., and Nosrati, M. (2023). Artificial intelligence in regenerative medicine: applications and implications. Biomimetics 8 (5), 442. doi:10.3390/biomimetics8050442
Ohata, K., and Ott, H. C. (2020). Human-scale lung regeneration based on decellularized matrix scaffolds as a biologic platform. Surg. today 50 (7), 633–643. doi:10.1007/s00595-020-02000-y
Okamoto, M., and John, B. (2013). Synthetic biopolymer nanocomposites for tissue engineering scaffolds. Prog. Polym. Sci. 38 (10-11), 1487–1503. doi:10.1016/j.progpolymsci.2013.06.001
Olejarz, W., Kubiak-Tomaszewska, G., Chrzanowska, A., and Lorenc, T. (2020). Exosomes in angiogenesis and anti-angiogenic therapy in cancers. Int. J. Mol. Sci. 21 (16), 5840. doi:10.3390/ijms21165840
Ott, H. C., Clippinger, B., Conrad, C., Schuetz, C., Pomerantseva, I., Ikonomou, L., et al. (2010). Regeneration and orthotopic transplantation of a bioartificial lung. Nat. Med. 16 (8), 927–933. doi:10.1038/nm.2193
Ott, H. C., Matthiesen, T. S., Goh, S. K., Black, L. D., Kren, S. M., Netoff, T. I., et al. (2008). Perfusion-decellularized matrix: using nature's platform to engineer a bioartificial heart. Nat. Med. 14 (2), 213–221. doi:10.1038/nm1684
Pacelli, S., Basu, S., Whitlow, J., Chakravarti, A., Acosta, F., Varshney, A., et al. (2017). Strategies to develop endogenous stem cell-recruiting bioactive materials for tissue repair and regeneration. Adv. drug Deliv. Rev. 120, 50–70. doi:10.1016/j.addr.2017.07.011
Pan, Z., Sun, W., Chen, Y., Tang, H., Lin, W., Chen, J., et al. (2022). Extracellular vesicles in tissue engineering: biology and engineered strategy. Adv. Healthc. Mater. 11 (21), 2201384. doi:10.1002/adhm.202201384
Petersen, T. H., Calle, E. A., Zhao, L., Lee, E. J., Gui, L., Raredon, M. B., et al. (2010). Tissue-engineered lungs for in vivo implantation. Science 329 (5991), 538–541. doi:10.1126/science.1189345
Petrella, F., and Spaggiari, L. (2018). Artificial lung. J. Thorac. Dis. 10 (Suppl. 20), S2329–S2332. doi:10.21037/jtd.2017.12.89
Pina, S., Ribeiro, V. P., Marques, C. F., Maia, F. R., Silva, T. H., Reis, R. L., et al. (2019). Scaffolding strategies for tissue engineering and regenerative medicine applications. Materials 12 (11), 1824. doi:10.3390/ma12111824
Ping, P., Guan, S., Ning, C., Yang, T., Zhao, Y., Zhang, P., et al. (2023). Fabrication of blended nanofibrous cardiac patch transplanted with TGF-β3 and human umbilical cord MSCs-derived exosomes for potential cardiac regeneration after acute myocardial infarction. Nanomedicine Nanotechnol. Biol. Med. 54, 102708. doi:10.1016/j.nano.2023.102708
Poongodi, R., Chen, Y. L., Yang, T. H., Huang, Y. H., Yang, K. D., Lin, H. C., et al. (2021). Bio-scaffolds as cell or exosome carriers for nerve injury repair. Int. J. Mol. Sci. 22 (24), 13347. doi:10.3390/ijms222413347
Qian, Y., Di, S., Wang, L., and Li, Z. (2021). Recent advances in the synthesis and applications of graphene–polypeptide nanocomposites. J. Mater. Chem. B 9 (33), 6521–6535. doi:10.1039/d1tb00779c
Qin, Y., Sun, R., Wu, C., Wang, L., and Zhang, C. (2016). Exosome: a novel approach to stimulate bone regeneration through regulation of osteogenesis and angiogenesis. Int. J. Mol. Sci. 17 (5), 712. doi:10.3390/ijms17050712
Rahmati, S., Khazaei, M., Nadi, A., Alizadeh, M., and Rezakhani, L. (2023). Exosome-loaded scaffolds for regenerative medicine in hard tissues. Tissue Cell 82, 102102. doi:10.1016/j.tice.2023.102102
Rajput, A., Varshney, A., Bajaj, R., and Pokharkar, V. (2022). Exosomes as new generation vehicles for drug delivery: biomedical applications and future perspectives. Molecules 27 (21), 7289. doi:10.3390/molecules27217289
Ratheesh, G., Venugopal, J. R., Chinappan, A., Ezhilarasu, H., Sadiq, A., and Ramakrishna, S. (2017). 3D fabrication of polymeric scaffolds for regenerative therapy. ACS Biomaterials Sci. and Eng. 3 (7), 1175–1194. doi:10.1021/acsbiomaterials.6b00370
Re, F., Gabusi, E., Manferdini, C., Russo, D., and Lisignoli, G. (2021). Bone regeneration improves with mesenchymal stem cell derived extracellular vesicles (EVs) combined with scaffolds: a systematic review. Biology 10 (7), 579. doi:10.3390/biology10070579
Reclusa, P., Taverna, S., Pucci, M., Durendez, E., Calabuig, S., Manca, P., et al. (2017). Exosomes as diagnostic and predictive biomarkers in lung cancer. J. Thorac. Dis. 9 (Suppl. 13), S1373–S1382. doi:10.21037/jtd.2017.10.67
Record, M., Carayon, K., Poirot, M., and Silvente-Poirot, S. (2014). Exosomes as new vesicular lipid transporters involved in cell–cell communication and various pathophysiologies. Biochimica Biophysica Acta (BBA)-Molecular Cell Biol. Lipids 1841 (1), 108–120. doi:10.1016/j.bbalip.2013.10.004
Ren, J., He, W., Zheng, L., and Duan, H. (2016). From structures to functions: insights into exosomes as promising drug delivery vehicles. Biomaterials Sci. 4 (6), 910–921. doi:10.1039/c5bm00583c
Rock, J. R., Onaitis, M. W., Rawlins, E. L., Lu, Y., Clark, C. P., Xue, Y., et al. (2009a). Basal cells as stem cells of the mouse trachea and human airway epithelium. Proc. Natl. Acad. Sci. U. S. A. 106 (31), 12771–12775. doi:10.1073/pnas.0906850106
Rock, J. R., Onaitis, M. W., Rawlins, E. L., Lu, Y., Clark, C. P., Xue, Y., et al. (2009b). Basal cells as stem cells of the mouse trachea and human airway epithelium. Proc. Natl. Acad. Sci. 106 (31), 12771–12775. doi:10.1073/pnas.0906850106
Romero-López, M., Trinh, A. L., Sobrino, A., Hatch, M. M., Keating, M. T., Fimbres, C., et al. (2017). Recapitulating the human tumor microenvironment: colon tumor-derived extracellular matrix promotes angiogenesis and tumor cell growth. Biomaterials 116, 118–129. doi:10.1016/j.biomaterials.2016.11.034
Rosenbluth, J. M., Schackmann, R. C. J., Gray, G. K., Selfors, L. M., Li, C. M. C., Boedicker, M., et al. (2020). Organoid cultures from normal and cancer-prone human breast tissues preserve complex epithelial lineages. Nat. Commun. 11 (1), 1711. doi:10.1038/s41467-020-15548-7
Roseti, L., Parisi, V., Petretta, M., Cavallo, C., Desando, G., Bartolotti, I., et al. (2017). Scaffolds for bone tissue engineering: state of the art and new perspectives. Mater. Sci. Eng. C 78, 1246–1262. doi:10.1016/j.msec.2017.05.017
Sen, S., Xavier, J., Kumar, N., Ahmad, M. Z., and Ranjan, O. P. (2023). Exosomes as natural nanocarrier-based drug delivery system: recent insights and future perspectives. 3 Biotech. 13 (3), 101. doi:10.1007/s13205-023-03521-2
Shakir, S., Hackett, T. L., and Mostaço-Guidolin, L. B. (2022). Bioengineering lungs: an overview of current methods, requirements, and challenges for constructing scaffolds. Front. Bioeng. Biotechnol. 10, 1011800. doi:10.3389/fbioe.2022.1011800
Silva, N. A., Salgado, A. J., Sousa, R. A., Oliveira, J. T., Pedro, A. J., Leite-Almeida, H., et al. (2010). Development and characterization of a novel hybrid tissue engineering–based scaffold for spinal cord injury repair. Tissue Eng. Part A 16 (1), 45–54. doi:10.1089/ten.tea.2008.0559
Singh, D., Singh, D., and Han, S. S. (2016). 3D printing of scaffold for cells delivery: advances in skin tissue engineering. Polymers 8 (1), 19. doi:10.3390/polym8010019
Song, J. J., Kim, S. S., Liu, Z., Madsen, J. C., Mathisen, D. J., Vacanti, J. P., et al. (2011). Enhanced in vivo function of bioartificial lungs in rats. Ann. Thorac. Surg. 92 (3), 998–1006. doi:10.1016/j.athoracsur.2011.05.018
Springer, N. L. (2019). The role of obesity-associated extracellular matrix remodeling in macrophage activation and potential implications to breast cancer.
Stratton, S., Shelke, N. B., Hoshino, K., Rudraiah, S., and Kumbar, S. G. (2016). Bioactive polymeric scaffolds for tissue engineering. Bioact. Mater. 1 (2), 93–108. doi:10.1016/j.bioactmat.2016.11.001
Su, N., Hao, Y., Wang, F., Hou, W., Chen, H., and Luo, Y. (2021). Mesenchymal stromal exosome–functionalized scaffolds induce innate and adaptive immunomodulatory responses toward tissue repair. Sci. Adv. 7 (20), eabf7207. doi:10.1126/sciadv.abf7207
Sun, W., Gregory, D. A., Tomeh, M. A., and Zhao, X. (2021). Silk fibroin as a functional biomaterial for tissue engineering. Int. J. Mol. Sci. 22 (3), 1499. doi:10.3390/ijms22031499
Swanson, W. B., Zhang, Z., Xiu, K., Gong, T., Eberle, M., Wang, Z., et al. (2020). Scaffolds with controlled release of pro-mineralization exosomes to promote craniofacial bone healing without cell transplantation. Acta biomater. 118, 215–232. doi:10.1016/j.actbio.2020.09.052
Tapias, L. F., and Ott, H. C. (2014). Decellularized scaffolds as a platform for bioengineered organs. Curr. Opin. organ Transplant. 19 (2), 145–152. doi:10.1097/mot.0000000000000051
Taylor, D. A., Caplan, A. L., and Macchiarini, P. (2014). Ethics of bioengineering organs and tissues. Expert Opin. Biol. Ther. 14 (7), 879–882. doi:10.1517/14712598.2014.915308
Thomas, S. C., Kim, J. W., Pauletti, G. M., Hassett, D. J., and Kotagiri, N. (2022). Exosomes: biological pharmaceutical nanovectors for theranostics. Front. Bioeng. Biotechnol. 9, 808614. doi:10.3389/fbioe.2021.808614
Torrecillas-Baena, B., Pulido-Escribano, V., Dorado, G., Gálvez-Moreno, M. Á., Camacho-Cardenosa, M., and Casado-Díaz, A. (2023). Clinical potential of mesenchymal stem cell-derived exosomes in bone regeneration. J. Clin. Med. 12 (13), 4385. doi:10.3390/jcm12134385
Tracy, E. P., Stielberg, V., Rowe, G., Benson, D., Nunes, S. S., Hoying, J. B., et al. (2022). State of the field: cellular and exosomal therapeutic approaches in vascular regeneration. Am. J. Physiology-Heart Circulatory Physiology 322 (4), H647–H680. doi:10.1152/ajpheart.00674.2021
Turnbull, G., Clarke, J., Picard, F., Riches, P., Jia, L., Han, F., et al. (2018). 3D bioactive composite scaffolds for bone tissue engineering. Bioact. Mater. 3 (3), 278–314. doi:10.1016/j.bioactmat.2017.10.001
Turunen, S., Kaisto, S., Skovorodkin, I., Mironov, V., Kalpio, T., Vainio, S., et al. (2018). 3D bioprinting of the kidney—hype or hope? AIMS Cell Tissue Eng. 2, 119–162. doi:10.3934/celltissue.2018.3.119
Uthman, O. A. (2016). Global, regional, and national life expectancy, all-cause mortality, and cause-specific mortality for 249 causes of death, 1980-2015: a systematic analysis for the Global Burden of Disease Study 2015. Lancet 388 (10053), 1459–1544. doi:10.1016/S0140-6736(16)31012-1
Vallet-Regí, M., Colilla, M., and González, B. (2011). Medical applications of organic–inorganic hybrid materials within the field of silica-based bioceramics. Chem. Soc. Rev. 40 (2), 596–607. doi:10.1039/c0cs00025f
Van Raemdonck, D., Neyrinck, A., Verleden, G. M., Dupont, L., Coosemans, W., Decaluwe, H., et al. (2009). Lung donor selection and management. Proc. Am. Thorac. Soc. 6 (1), 28–38. doi:10.1513/pats.200808-098go
Vig, S., and Fernandes, M. H. (2022). Bone cell exosomes and emerging strategies in bone engineering. Biomedicines 10 (4), 767. doi:10.3390/biomedicines10040767
Vojtech, L., Zhang, M., Davé, V., Levy, C., Hughes, S. M., Wang, R., et al. (2019). Extracellular vesicles in human semen modulate antigen-presenting cell function and decrease downstream antiviral T cell responses. PLoS One 14 (10), e0223901. doi:10.1371/journal.pone.0223901
Wagner, D. E., Bonvillain, R. W., Jensen, T., Girard, E. D., Bunnell, B. A., Finck, C. M., et al. (2013). Can stem cells be used to generate new lungs? ex vivo lung bioengineering with decellularized whole lung scaffolds. Respirology 18 (6), 895–911. doi:10.1111/resp.12102
Wang, D., Cong, Y., Deng, Q., Han, X., Zhang, S., Zhao, L., et al. (2021). Physiological and disease models of respiratory system based on organ-on-a-chip technology. Micromachines 12 (9), 1106. doi:10.3390/mi12091106
Wang, L., Feng, M., Zhao, Y., Chen, B., Zhao, Y., and Dai, J. (2023). Biomimetic scaffold-based stem cell transplantation promotes lung regeneration. Bioeng. and Transl. Med. 8, e10535. doi:10.1002/btm2.10535
Wang, L., Ouyang, M., Xing, S., Zhao, S., Liu, S., Sun, L., et al. (2022). Mesenchymal stem cells and their derived exosomes promote malignant phenotype of polyploid non-small-cell lung cancer cells through AMPK signaling pathway. Anal. Cell. Pathol. 2022, 1–15. doi:10.1155/2022/8708202
Wang, T., Zhou, Y., Zhang, W., Xue, Y., Xiao, Z., Zhou, Y., et al. (2024). Exosomes and exosome composite scaffolds in periodontal tissue engineering. Front. Bioeng. Biotechnol. 11, 1287714. doi:10.3389/fbioe.2023.1287714
Wansleeben, C., Barkauskas, C. E., Rock, J. R., and Hogan, B. L. M. (2013). Stem cells of the adult lung: their development and role in homeostasis, regeneration, and disease. Wiley Interdiscip. Rev. Dev. Biol. 2 (1), 131–148. doi:10.1002/wdev.58
Wasyłeczko, M., Sikorska, W., and Chwojnowski, A. (2020). Review of synthetic and hybrid scaffolds in cartilage tissue engineering. Membranes 10 (11), 348. doi:10.3390/membranes10110348
Wilkinson, D. C., Alva-Ornelas, J. A., Sucre, J. M., Vijayaraj, P., Durra, A., Richardson, W., et al. (2017). Development of a three-dimensional bioengineering technology to generate lung tissue for personalized disease modeling. Stem cells Transl. Med. 6 (2), 622–633. doi:10.5966/sctm.2016-0192
Willie, B. M., Petersen, A., Schmidt-Bleek, K., Cipitria, A., Mehta, M., Strube, P., et al. (2010). Designing biomimetic scaffolds for bone regeneration: why aim for a copy of mature tissue properties if nature uses a different approach? Soft Matter 6 (20), 4976–4987. doi:10.1039/c0sm00262c
Xiao-Ni, L. I. U., Zhang, C. B., Lin, H., Tang, X. Y., Zhou, R., Wen, H. L., et al. (2021). microRNA-204 shuttled by mesenchymal stem cell-derived exosomes inhibits the migration and invasion of non-small-cell lung cancer cells via the KLF7/AKT/HIF-1α axis. Neoplasma 68 (4), 719–731. doi:10.4149/neo_2021_201208n1328
Xie, L., and Zeng, Y. (2020). Therapeutic potential of exosomes in pulmonary fibrosis. Front. Pharmacol. 11, 590972. doi:10.3389/fphar.2020.590972
Xie, M., Wu, D., Li, G., Yang, J., and Zhang, Y. S. (2021). Exosomes targeted towards applications in regenerative medicine. Nano Sel. 2 (5), 880–908. doi:10.1002/nano.202000251
Xie, Y., Guan, Q., Guo, J., Chen, Y., Yin, Y., and Han, X. (2022). Hydrogels for exosome delivery in biomedical applications. Gels 8 (6), 328. doi:10.3390/gels8060328
Xu, J., Liao, K., and Zhou, W. (2018). Exosomes regulate the transformation of cancer cells in cancer stem cell homeostasis. Stem cells Int. 2018, 1–16. doi:10.1155/2018/4837370
Yan, H.-C., Yu, T. T., Li, J., Qiao, Y. Q., Wang, L. C., Zhang, T., et al. (2020). The delivery of extracellular vesicles loaded in biomaterial scaffolds for bone regeneration. Front. Bioeng. Biotechnol. 8, 1015. doi:10.3389/fbioe.2020.01015
Yang, Y., and El Haj, A. J. (2006). Biodegradable scaffolds–delivery systems for cell therapies. Expert Opin. Biol. Ther. 6 (5), 485–498. doi:10.1517/14712598.6.5.485
Yeo, M., Sarkar, A., Singh, Y. P., Derman, I. D., Datta, P., and Ozbolat, I. T. (2023). Synergistic coupling between 3D bioprinting and vascularization strategies. Biofabrication 16 (1), 012003. doi:10.1088/1758-5090/ad0b3f
Yuan, Z., Ren, Y., Shafiq, M., Chen, Y., Tang, H., Li, B., et al. (2022). Converging 3D printing and electrospinning: effect of poly (L-lactide)/gelatin based short nanofibers aerogels on tracheal regeneration. Macromol. Biosci. 22 (1), 2100342. doi:10.1002/mabi.202100342
Zhang, D., and Wang, Y. (2019). Functional protein-based bioinspired nanomaterials: from coupled proteins, synthetic approaches, nanostructures to applications. Int. J. Mol. Sci. 20 (12), 3054. doi:10.3390/ijms20123054
Zhang, Y., Liu, Y., Liu, H., and Tang, W. H. (2019). Exosomes: biogenesis, biologic function and clinical potential. Cell and Biosci. 9, 19–18. doi:10.1186/s13578-019-0282-2
Keywords: lung regeneration, tissue engineering, exosome-loaded scaffolds, organoids, decelullarization
Citation: Torkashvand M, Rezakhani L, Habibi Z, Mikaeili A and Rahmati S (2024) Innovative approaches in lung tissue engineering: the role of exosome-loaded bioscaffolds in regenerative medicine. Front. Bioeng. Biotechnol. 12:1502155. doi: 10.3389/fbioe.2024.1502155
Received: 26 September 2024; Accepted: 04 December 2024;
Published: 20 December 2024.
Edited by:
Jangho Kim, Chonnam National University, Republic of KoreaReviewed by:
Mahboubeh Nabavinia, The Research Institute at Nationwide Children’s Hospital, United StatesJinho Kim, Stevens Institute of Technology, United States
Copyright © 2024 Torkashvand, Rezakhani, Habibi, Mikaeili and Rahmati. This is an open-access article distributed under the terms of the Creative Commons Attribution License (CC BY). The use, distribution or reproduction in other forums is permitted, provided the original author(s) and the copyright owner(s) are credited and that the original publication in this journal is cited, in accordance with accepted academic practice. No use, distribution or reproduction is permitted which does not comply with these terms.
*Correspondence: Shima Rahmati, c2ltYXJhaG1hdGkxOTg3QGdtYWlsLmNvbQ==
†These authors share first authorship