- 1Institute of Biomaterials and Bioengineering, Faculty of Natural Science and Technology, Riga Technical University, Riga, Latvia
- 2Baltic Biomaterials Centre of Excellence, Headquarters at Riga Technical University, Riga, Latvia
Platelet-rich fibrin (PRF) is a protein matrix with growth factors and immune cells extracted from venous blood via centrifugation. Previous studies proved it a beneficial biomaterial for bone and soft tissue regeneration in dental surgeries. Researchers have combined PRF with a wide range of biomaterials for composite preparation as it is biocompatible and easily acquirable. The results of the studies are difficult to compare due to varied research methods and the fact that researchers focus more on the PRF preparation protocol and less on the interaction of PRF with the chosen material. Here, the literature from 2013 to 2024 is reviewed to help surgeons and researchers navigate the field of commonly used biomaterials in maxillofacial surgeries (calcium phosphate bone grafts, polymers, metal nanoparticles, and novel composites) and their combinations with PRF. The aim is to help the readers select a composite that suits their planned research or medical case. Overall, PRF combined with bone graft materials shows potential for enhancing bone regeneration both in vivo and in vitro. Still, results vary across studies, necessitating standardized protocols and extensive clinical trials. Overviewed methods showed that the biological and mechanical properties of the PRF and material composites can be altered depending on the PRF preparation and incorporation process.
1 Introduction
Platelet-rich fibrin (PRF) is an autologous biomaterial derived from venous blood via centrifugation without additives. This protein matrix contains over 1,500 bioactive molecules at up to 600 times the concentration of normal venous blood (Pavlovic et al., 2021; Peck et al., 2015). Since its first reports in 2001, PRF has gained significant interest in regenerative biomaterials for use in oral, maxillofacial, orthopedic, and gynecological surgeries (Dohan et al., 2006; Grecu et al., 2019; Wang et al., 2021). PRF is a second-generation platelet concentrate, succeeding platelet-rich plasma (PRP) (Dohan et al., 2006). Although PRP also has a high concentration of growth factors and potential healing properties, it requires an anticoagulant for preparation (Le et al., 2018). This leads to faster platelet activation and growth factor release, with 95% of growth factors being released shortly after contact with the anticoagulant (Miron et al., 2017).
Different PRF types vary based on preparation protocols, particularly centrifugation speed and time thus resulting in different platelet concentrations. The main types are leukocyte and platelet-rich fibrin (L-PRF), Injectable-PRF (I-PRF), and Advanced-PRF (A-PRF) (Dohan et al., 2006; Miron et al., 2017; Dohan et al., 2014; Ghanaati et al., 2014; Fujioka-Kobayashi et al., 2017). The first PRF preparation method, sometimes called Choukroun PRF or L-PRF (further in text L-PRF), requires 10 mL of a blood sample, that is centrifuged in glass-coated plastic tubes without any anticoagulant for 10 min at 400 × g (g stands for gravitational force) (Dohan et al., 2006). Future modifications to this protocol allowed the development of A-PRF by reducing centrifugation speed and increasing the centrifugation time to 14 min. This method increased the number of immune cells and platelets in the fibrin matrix compared to L-PRF (Ghanaati et al., 2014). A-PRF+ is similar to A-PRF, except the protocol suggests using only 200 × g for 8 min and proves to be even richer in growth factors than A-PRF (Fujioka-Kobayashi et al., 2017). I-PRF is in liquid form compared to other PRF types. The original I-PRF protocol reported in 2015 consists of horizontal centrifugation of 3,300 rpm for 2 min (Almeida Barros Mourão et al., 2015), but 2 years later in a publication by Miron, it was described using 700 rpm (60 × g) for 3 min (Miron et al., 2017). Although different PRF types have progressed over time to improve the biological and mechanical properties of the previous generations, the most used remains L-PRF (Barbosa et al., 2023).
Although PRF is biologically active, it lacks the necessary mechanical properties for soft and hard tissue renewal (Isobe et al., 2017). Studies indicate that Young’s modulus of L-PRF ranges from 187.6 ± 82.73 kPa in membrane form to 30.2 ± 16.7 kPa or lower in clot form (Lara et al., 2023; Haghparast-Kenarsari et al., 2024) In contrast, biomechanical studies on human cadavers show Young’s modulus of oral soft tissue ranges from 8 to 37 MPa, depending on the intraoral tissue site (Choi et al., 2020). To enhance PRF’s mechanical properties, it can be combined with synthetic and natural polymers, calcium phosphates, metals, and various composites. The choice of material to be combined with PRF should depend on the mechanical properties as well as biocompatibility, porosity and other requirements according to the targeted tissue repair.
Calcium phosphate (CaP) materials are widely used for bone tissue substitutes due to their high biocompatibility, osteoconductivity, and resemblance to bone composition (Jeong et al., 2019). Natural polymers offer high bioactivity and low immune response but suffer from poor thermal stability and mechanical strength, making them challenging for shaping and degradation control. Synthetic polymers exhibit suitable mechanical properties for bone tissue regeneration but have poor cell adhesion as they lack appropriate surface-free energy and cannot bond with human tissue (Gao et al., 2017). To overcome these limitations, biomaterials are frequently combined or modified with bioactive substances like growth factors for which PRF can be used (Bjelić and Finšgar, 2021; Fernandez-Medina et al., 2023).
Due to PRFs gelatinous structure, it is moldable, allowing users to process it in various ways based on the desired composite outcome as illustrated in Figure 1. The most used PRF types with other biomaterials are those that clot during centrifugation (L-PRF and A-PRF). After pressing PRF into the membrane it can be combined with other membranes, placed in the middle of scaffold layers, or minced and mixed with sponges (Pandikanda et al., 2019; Zhang L. et al., 2019; Sebastian et al., 2022). For incorporation into bioinks or scaffold solutions, lyophilized clot PRF, supernatant from PRF clots, minced PRF clots, and decellularized PRF (dPRF) can be used (Sui et al., 2023; Song et al., 2018; Chi et al., 2019; Tarif et al., 2023) Nanoparticles can be added and incorporated into PRF clots by adding them to the whole blood before centrifugation or by injecting nanoparticle solution into PRF clots (Khorshidi et al., 2018; Ghaznavi et al., 2019; Zalama et al., 2021). PRF clot fibrin structure can also be mineralized by adding alkaline phosphatase (ALP) to whole blood before centrifuging and incubating the PRF membrane for 3 days in calcium glycerophosphate (Douglas et al., 2012; Gassling et al., 2013). A widely used material called “Sticky bone” consists of biomaterial granules mixed with a platelet concentrate to create a moldable biomaterial. Sticky bone is the most common PRF/graft material composite in maxillofacial surgery and can be prepared by adding minced PRF clot, I-PRF, or both, to bone substitute biomaterial granules or particles. (Ramamurthy et al., 2022; Ponte et al., 2021; van Orten et al., 2022; Park et al., 2023; Feng et al., 2022).
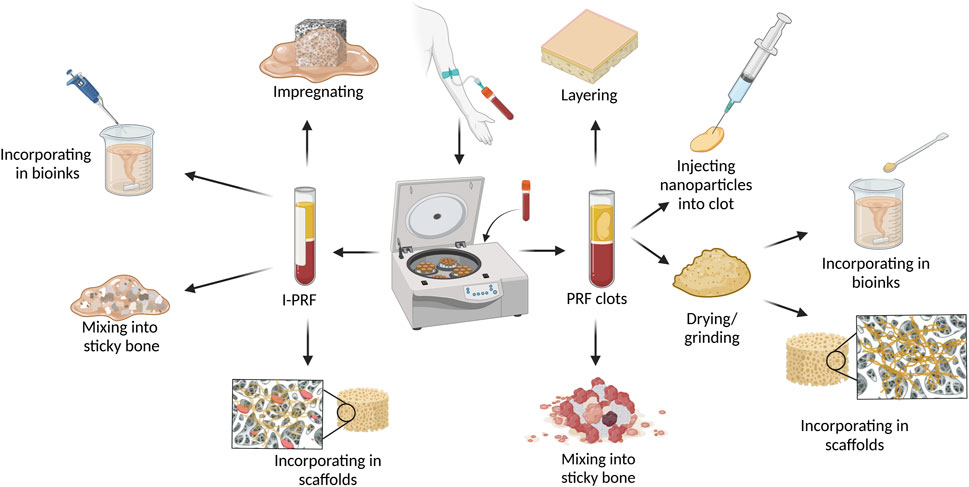
Figure 1. Methods and techniques to prepare PRF and biomaterial composites. Figure created with biorender.com.
Due to the I-PRF liquid nature, it can be used differently than PRF clot types. For scaffold preparation, I-PRF can be added straight to the 3D printing bioinks without any pre-processing like it would be needed for PRF clot types (Yi et al., 2022). Another advantage of I-PRF’s liquid form is its ability to impregnate porous materials like membranes and scaffolds (Patra et al., 2022).
Studies have explored the biological and mechanical properties of biomaterial and PRF combinations, but no review has summarized the effects of these composites to summarize their interactions (Al-Maawi et al., 2021; Blatt et al., 2020; Sampaio et al., 2023; Dambhare et al., 2019). This review aims to combine existing information about PRF usage in association with other biomaterials from in vitro, in vivo, and clinical studies in maxillofacial and oral surgery. This is done by summarizing articles from academic databased such as PubMed/MEDLINE, ScienceDirect, and Scopus in a time frame from 2013 to 2024, that involves the combinations of PRF with a biomaterial and is tested in vitro, in vivo, and in clinical studies. To limit the amount of included articles, studies in any way incorporating PRF within the material or vice versa are included. Studies using PRF and materials separately in the same defect are not included. This helps readers by summarizing information from open-source databases, providing easier navigation in the field of PRF and biomaterial composites. Thus, this article serves as a guide for selecting suitable composites for planned research or medical cases.
2 Inorganic materials
2.1 Calcium phosphate ceramics and bone grafts
Bone grafts can be autogenous, allogeneic, xenogeneic, or alloplastic. Autogenous grafts are taken from a patient’s rib or iliac crest allogeneic grafts are harvested from donors, while xenografts come from different species, typically pigs or bovines. Alloplastic grafts are synthetic and made from minerals similar to bone (Kumar et al., 2013). Calcium Phosphates (CaP) are minerals containing Ca2+ cations and inorganic phosphate anions, and are the main minerals in bone and tooth enamel, thus they are favored in regenerative surgery (Terzioğlu et al., 2018; Ma et al., 2023; Eliaz and Metoki, 2017). Commonly used CaP in clinical settings include hydroxyapatite (HA), tricalcium phosphate (TCP), and biphasic calcium phosphate (BCP) (Gao et al., 2017). Among the studies, different PRF and CaPs composites are the most frequently studied.
Multiple in vitro studies show that combining PRF with allogenic, alloplastic, and xenogenic bone substitute materials (BSM) enhances angiogenic, non-cytotoxic, and osteogenic properties (Blatt et al., 2021a; Blatt et al., 2021b; Kumar et al., 2021; Kumar et al., 2019) Table 1. I-PRF with these bone grafts reduces early platelet-derived growth factor (PDGF) release up to two-fold compared to A-PRF (Blatt et al., 2021a). L-PRF improves cell viability, proliferation, migration, and extracellular matrix formation on alloplastic and xenogenic BG, with higher PRF concentrations yielding better effects (Blatt et al., 2021b). L-PRF combined with dentin chips induces higher dentin sialophosphoprotein expression in primary human dental pulp stem cells (DPSCs) compared to L-PRF with nano-hydroxyapatite (nHA), although nHA + L-PRF induces higher DPSCs mineralization L-PRF with dentin chips (Girija and Kavitha, 2020). Kumar et al. found that mixing cut L-PRF membrane with biphasic calcium phosphate (BCP) inhibits the mitogen-activated protein kinase (MAPK) signaling pathway, reducing osteoclastic effects and osteoclast differentiation (Kumar et al., 2021; Kumar et al., 2019). Combining I-PRF with allogenic, alloplastic, and xenogenic BG results in up to a two-fold increase in new blood vessel formation within 24 h compared to native materials, attributed to elevated PDGF levels. This can be attributed to the authors' findings of elevated Platelet-Derived Growth Factor (PDGF) levels in these instances (Blatt et al., 2021a). Higher PDGF-D concentrations (100 ng/mL) enhance endothelial progenitor cell migration, adhesion, and tube formation (Zhang J. et al., 2019). Additionally, mixing nHA or dentin chips with L-PRF increases the radiopacity of the platelet concentrate, making it more visible in X-ray imaging. (Mahendran et al., 2019).
In vivo studies on animal models such as sheep, pigs, rats, rabbits, and dogs have shown that combining PRF with various calcium phosphate (CaP) bone grafts enhances osteoblast activity and accelerates new bone tissue formation, thereby reducing healing time (Abdullah, 2016; Yilmaz et al., 2014; Alkafarani and Baban, 2019; Pascawinata et al., 2023; Şimşek et al., 2016; Nacopoulos et al., 2014; Shevchenko and Rublenko, 2022; Acar et al., 2015; Bölükbaşı et al., 2013). For example, using L-PRF and BCP sticky bone to fill bone defects in sheep resulted in 42% defect coverage by new bone on day 20% and 54.9% on day 40, compared to 29.6% and 49.1% for BCP alone (Bölükbaşı et al., 2013). Similarly, Hwan Jung et al. found that L-PRF mixed with dentin powder improved implant stability and increased regenerated bone area and bone-to-implant contact after 8 weeks (Hwan Jung et al., 2020). Yuan et al. show that PRF in combination with deproteinized bovine bone mineral (DBBM) had higher osteoclast activity than just DBBM in a canine model (Yuan et al., 2021). In rabbits, L-PRF combined with autografts and xenografts promoted faster new bone formation after 8 weeks, though this effect was not observed with β-tricalcium phosphate (β-TCP). (Karayürek et al., 2019). Additionally, some studies show that PRF does not improve healing time when combined with graft materials (Park et al., 2023; Knapen et al., 2015; Lee et al., 2021; Kamal et al., 2017). Knappen et al. observed similar healing patterns in rabbit calvaria between L-PRF, bovine HA, and their combination at early time points of 1, 5, and 12 weeks (Knapen et al., 2015).
Clinical trials testing PRF combined with graft materials for bone defects have been conducted since 2010 (Inchingolo et al., 2010). Multiple studies have since shown that PRF with BSM accelerates dental implant stabilization and tissue healing after sinus lifts, ridge preservation, or bone augmentation have shown positive results in various in vivo studies and case reports (Ramamurthy et al., 2022; van Orten et al., 2022; Tiwari et al., 2020; Pichotano et al., 2018; Amam et al., 2023; hamuda et al., 2023; Simone et al., 2018; Massuda et al., 2023; Caramês et al., 2022; Alberto et al., 2020).
Residual bone grafts also tend to degrade quicker when BG is combined with PRF (Nizam et al., 2018). Pichotano showed that after L-PRF + DBBM usage in maxillary sinus augmentation, the residual bone graft material significantly reduced after 4 months in the test group (3.59% ± 4.22%) compared to the control group (13.75% ± 9.99%) with only DBBM in 8 months (Pichotano et al., 2019).
In clinical human studies and case reports, sticky bone is one of the most studied PRF and bone graft composites. Sticky bone is widely used for severe bone defects in maxillofacial surgery, which are summarized in Figure 2 (Elkholly et al., 2022; Deenadayalan et al., 2015; Hiremath et al., 2014; Lei et al., 2019; Pradeep et al., 2016; Shivashankar et al., 2013; Lorenz et al., 2018) Feng et al. described that using I-PRF with minced PRF clot for sticky bone preparation shortened solidification time, improved tensile resistance, and prolonged degradation time compared to sticky bone made with each PRF type separately. This preparation method provides a more moldable material for filling difficult bone defects (Feng et al., 2022).
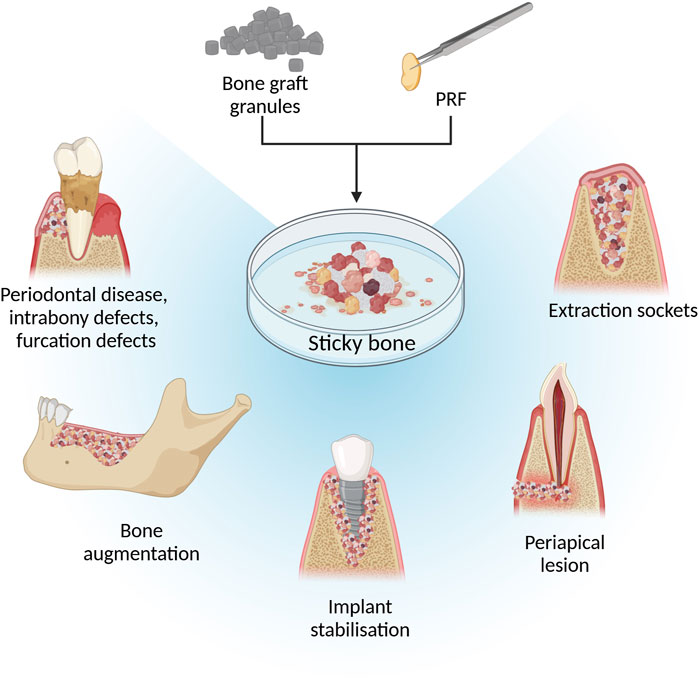
Figure 2. Illustration of using sticky bone in maxillofacial surgeries. Figure created with biorender.com.
Reports on the efficiency of PRF and biomaterial combinations for extraction socket wound healing are often contradictory. PRF improves healing when combined with β-TCP, but not with BCP. Ponte et al. found that sticky bone made with I-PRF and BCP induced slower new bone formation in 8 months compared to PRF clot or BCP alone, although earlier time points were not tested (Ponte et al., 2021). In contrast, β-TCP mixed with PRF clot improved bone density (620.0 ± 31.02) in 6 months compared to PRF (336.6 ± 66.65) or β-TCP (466.0 ± 38.24) alone and helped sustain alveolar ridge bone height and width (Mbarak et al., 2023). Similarly, β-TCP mixed with L-PRF is more efficient in socket wound healing than β-TCP mixed with platelet-rich plasma in parameters like alveolar bone width, height resorption, and bone density (abou shabana et al., 2023).
In 2019, Clark et al. (2018) noted that a combination of A-PRF and freeze-dried bone allograft (FDBA) for ridge preservation resulted in lower bone mineral density compared to FDBA alone. However, histology showed more vital bone volume with A-PRF and FDBA than with FDBA alone. For horizontal ridge defect treatment, better results are suggested when the sticky bone is made from a mineralized plasmatic matrix rather than L-PRF, showing increased bone surface area, osteopontin expression, and reduced collagen amount by bone maturation (Anwar and Hamid, 2022). Additionally, Maia et al. observed that covering a defect with collagen membranes can reduce the healing efficiency of L-PRF and BSM composites (Maia et al., 2019).
Literature on PRF and bone graft composites for intrabony defects have previously been summarized in multiple specific to this disease-focused meta-analyses and systematic reviews and thus will not be reviewed in this article (Pepelassi and Deligianni, 2022; Ye et al., 2023; Theodosaki et al., 2022). Shortly, 2022 systematic review by Theodosaki et al. (2022) noted that PRF added to inorganic bone grafts (BG) offers small improvements in healing size but faster healing time. Pepelassi and Deligianni (2022)Pepelassi et al. (2022) found that using L-PRF with osseous grafts reduces probing pocket depth and radiographic defect depth while improving clinical attachment levels in endosseous and class II furcation defects for non-smoking chronic periodontitis patients. However, a 2023 meta-analysis by Ye et al. (2023) showed insignificant differences in clinical outcomes between PRF + biomaterials and biomaterials alone. Couple of systematic reviews and meta-analyses focusing on PRF and bone graft adjunctive usage in sinus augmentation noted that this method has inconclusive results. Significant drawbacks in clinical studies include unstandardized PRF preparation protocols and short follow-up periods (Alotaibi et al., 2020; Liu et al., 2019).
2.2 Bioactive glass
Another synthetic bone substitute is bioactive glass, which promotes integration with living cells and facilitates the healing process by bonding both to soft and hard tissue by partly converting to hydroxyapatite (Bi et al., 2013; Wilson and Low, 1992). Bioactive glass is composed of minerals like SiO2, CaO, Na2O, and P2O5 (Hench et al., 2000). Available in various forms—particulate, powder, mesh, and cones—it can be molded to suit different needs and thus is used for bone reconstruction in maxillofacial surgery (Krishnan and Lakshmi, 2013; Han et al., 2020). However, bioactive glass and PRF combinations have been less studied than CaP material composites.
A few studies have tested PRF clots combined with bioactive glass for intrabony defects, showing positive effects (Agrawal et al., 2017; saravanan et al., 2019) (Table 2). Agrawal’s 2017 study mixed L-PRF with calcium phosphosilicate putty for filling intrabony defects, observing significantly better defect bone fill in the L-PRF + bioglass group compared to the bioglass group alone after 6 months. However, L-PRF + bioactive glass treatment had insignificant changes in pocket depth, clinical attachment level, and gingival recession compared to bioglass (Agrawal et al., 2017). Other studies also indicated that L-PRF + bioglass has similar efficiency to bioglass alone for treating intrabony defects or gingival recession (Vibhor et al., 2021). Vibhor et al. (2021) study found no statistical differences in treatment efficiency between bioglass and bioglass with L-PRF after three and 6 months postoperatively.
2.3 Metals
2.3.1 Zinc
Ionic zinc (Zn) has attracted attention due to its significant role as a micronutrient in physiological and biological systems, their cost-effectiveness, low toxicity, and usability in drug delivery and bioimaging (Su et al., 2019). These nanoparticles can be synthesized via “Green” methods that include extracting them from plants fungus, bacteria and algae (Kalpana et al., 2018). ZnONPs are used for cancer and antibacterial treatment due to the intracellular reactive oxygen species (ROS) generation (Jiang et al., 2018). Zinc also induces bone tissue formation, influences osteoblast proliferation, collagen synthesis, and ALP activity (Molenda and Kolmas, 2011). In maxillofacial surgery, ZnONPs are added to scaffolds to reduce bacterial biofilm production and enhance implant osteointegration (Pushpalatha et al., 2022).
Zalama et al. (2021); Zalama et al. (2022) studied the bone tissue regenerative effects of ZnONPs in size <100 nm by injecting them into L-PRF clots with insulin syringes. In two studies they treated New Zealand white rabbit critical ulnar defects with L-PRF/ZnONPs composite. In their 2021 study, radiographic examinations revealed similar healing scores and new tissue formation for both L-PRF and L-PRF/ZnONPs after 1 and 2 months (Zalama et al., 2021) However, their 2022 study provided a more detailed analysis, demonstrating that L-PRF/ZnONPs outperformed L-PRF alone at all postoperative time points (30, 60, and 90 days) in callus bridging scores, defect size reduction, and bone marrow canal formation. Specifically, L-PRF/ZnONPs promoted higher new bone tissue density at day 60 (1,498.95 ± 77.19 Hounsfield units), comparable to normal bone density (1,508.20 ± 144.52), whereas L-PRF and control groups failed to reach the required bone density even by day 90 (1,212.52 ± 79.18 and 1,284.53 ± 188.30, respectively) (Zalama et al., 2022). Although these studies did not compare L-PRF/ZnONPs to ZnONPs alone, they provided critical insight that combining PRF with ZnONPs significantly improves bone regeneration time and quality compared to PRF alone (Figure 3) (Table 3).
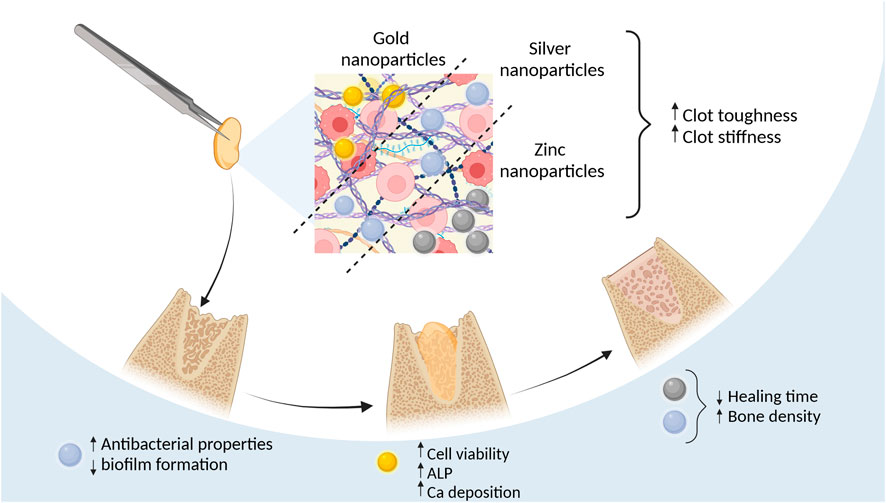
Figure 3. Effects of using metal nanoparticles incorporated into PRF Figure created with biorender.com.
2.3.2 Silver and gold nanoparticles
Silver nanoparticles (AgNP) are known for their antimicrobial, antifungal, and antioxidant properties (Burdus et al., 2018). Their antimicrobial effects are due to targeting cell membranes, generating reactive oxygen species (ROS), and inducing oxidative stress through silver ion release and particle size/form (Yan et al., 2018). However, these effects also occur in human cells, where AgNPs are seen as “non-self” by the immune system, triggering immune responses in a dose-dependent way (Dakal et al., 2016; Pauksch et al., 2014). Despite these challenges, AgNPs are beneficial for bone regeneration, as they promote early bone callus formation by attracting and promoting the proliferation of mesenchymal stem cells (Zhang et al., 2015).
Because of the antimicrobial activity of silver researchers have been interested in the AgNP and PRF composites (Table 4). The addition of AgNPs to LPRF membranes improved tensile strength 2-fold and stiffness 5-fold while the toughness of the L-PRF did not change (Khorshidi et al., 2018). L-PRF modified with AgNP in size <100 nm exhibits superior antimicrobial activity against Streptococcus, Klebsiella pneumoniae, and Candida species and inhibits biofilm formation (Khorshidi et al., 2018; Haddadi et al., 2018). Salih et al. (2018) demonstrated that the L-PRF/AgNPs combination significantly improved bone tissue regeneration speed and quality within 4 weeks compared to each material used separately. Notably, none of these studies reported the cytotoxic effects typically associated with AgNPs.
Similarly, gold nanoparticles (AuNPs) also have osteogenic and bactericidal effects, that are influenced by surface charge, reaction nature, and aggregation level (Basova et al., 2021; Zhang et al., 2021). The exact mechanism behind AuNPs’ antibacterial action remains unclear, with some attributing the effects to co-existing chemicals (Basova et al., 2021). The ability of AuNPs to induce osteogenic differentiation could be promoted by the ability to upregulate bone-related protein (Runx2, Col-1B, OPN, and ALP) expression and cell mineralization (Zhang et al., 2021). Concerns about AuNPs toxicity and long-term safety necessitate further in vivo studies to determine biodistribution and potential toxicity (Basova et al., 2021).
Indirect in vitro testing using conditioned medium from A-PRF+ (1,300 rpm, 8 min) enriched with 53 ± 2 nm AuNPs increased human mesenchymal stem cell (hMSCs) viability compared to only AuNPs that decreased viability in dose dose-dependent manner with the highest viability being at 0.005 mM and the lowest at 0.5 mM particle concentration. Osteogenic differentiation markers like the ALP in the supernatant from AuNPs/A-PRF+ were significantly higher than in the control and A-PRF+ groups. Alizarin Red staining revealed calcium deposition in human mesenchymal stem cells treated with AuNPs/A-PRF+ conditioned medium, indicating enhanced osteoconduction (Ghaznavi et al., 2019).
3 Polymers
3.1 Polycaprolactone
Polycaprolactone (PCL) is a semicrystalline, biodegradable polymer derived from petrochemical products (Bezwada et al., 1995). PCL is used for drug delivery and for tissue engineering (e.g., bone, blood vessel, cartilage) due to its non-toxicity, biocompatibility, and long degradation time of 2 to 3 years but it lacks hydrophilic functional groups thus inhibiting cellular growth (Engelberg and Kohn, 1991; Malikmammadov et al., 2018; Fu et al., 2012). Therefore, to enhance biocompatibility, PCL materials are often coated with growth factors or synthetic peptides (Zhang et al., 2009; Qin et al., 2022). In maxillofacial surgeries PCL is used for bone reconstructions in maxilla and mandibulae (Hwang et al., 2023; Naik et al., 2020).
Growth factors from PRF can enhance the biocompatibility of PCL biomaterials (Fernandez-Medina et al., 2023; Al-Maawi et al., 2021). Although hydrophobic, PCL scaffolds can physically bind growth factors on their surface, but the protein absorption quantity depends on material surface roughness and hydrophilicity which depends on the manufacturing process (Khampieng et al., 2018; Shen and Hu, 2021). Fernandez-Medina et al. demonstrated that leukocyte platelet-rich plasma (L-PRP) and I-PRF can induce different protein bindings on PCL surfaces. When immersed in I-PRF, PCL surfaces initially bind high molecular weight (>90 kDa) proteins, which gradually desorb and are replaced by middle-low (50–30 kDa) and low (<30 kDa) molecular weight proteins, such as IL-8, eotaxin, IP-10, and RANTES. Conversely, PCL surfaces coated with L-PRP show stable binding of middle-low and low molecular weight proteins like the γ- and β-chains of fibrinogen, which can induce pro-inflammatory processes (Fernandez-Medina et al., 2023; Luyendyk et al., 2019). PCL scaffolds coated with I-PRF have superior protein corona formation compared to those coated with pure platelet-rich plasma, L-PRP, or plasma, indicating better bioactivity. Notably, the presence of a CaP coating on the PCL surface did not affect the protein corona formation when I-PRF was applied (Fernandez-Medina et al., 2023). The method of PRF production plays a crucial role in the effectiveness of PCL-PRF composites. Al-Maawi et al. (2021) found that PCL meshes coated with I-PRF prepared using a low centrifugation method (44 × g) resulted in higher human primary osteoblast adherence after 7 days compared to a high-speed centrifugation method (710 × g). The low-speed PRF composite released twice as many growth factors over 3 and 7-day periods and promoted higher ALP expression from primary osteoblasts seeded on the PCL scaffold (Table 5).
The limited number of extensive in vivo studies on PCL and PRF composites limits the understanding of their overall effect. One study showed that even after the addition of L-PRF the surrounding bone tissue of rat calvaria did not adhere to these scaffolds and used connective tissue to attach to it. The same report stated that the addition of PRF on PCL has the same effect on new bone formation as native PCL. Their PRF preparation method involved a high-speed centrifugation method (1,670 × g), which could explain the reduced biocompatibility (Chen et al., 2021). Verma et al. (2014) showed that PCL with PRF promotes early bone healing in peri-implant defects. However, this study lacked a control group with native PCL or PCL with a different coating, making accurate interpretation of results difficult.
3.2 Collagen and gelatin
Collagen is the most abundant protein in the human body, providing a scaffold for cells and aiding in the transfer of internal and external forces (Meyer, 2019). There are over 27 types of collagens in vertebrates, with type I collagen being the most common in skin, bone, and tendon (Birk and Bruckner, 2005). Gelatin, a collagen derivative, is a biodegradable and biocompatible protein produced by hydrolyzing collagen’s triple helical structure into random coiled domains, resulting in a molecular structure similar to collagen (Shoulders and Raines, 2009; Davidenko et al., 2016). In maxillofacial surgery, collagen is used as a membrane for creating a barrier between soft and bone tissue, scaffolds for dental pulp regeneration and gelatin sponges are used as a space filler and a hemostatic absorbent (Shabat and Yousif, 2021; Debel et al., 2021; Sheikh et al., 2017).
Commercially available collagen membranes interact differently with I-PRF – for example, membranes with smaller pore sizes restrict the flow of PRF through its layers. This results in lower PRF absorption and shallower cell penetration in the material (Al-Maawi et al., 2019). However, collagen-based matrices with loosely arranged fibrils create a porous structure that allows PRF and its cells to be more easily absorbed (Udeabor et al., 2020). It is worth noting that no studies have described how this PRF absorbability affects cytokine release.
Studies show that when commercially available non-cross-linked equine-derived collagen hemostatic sponge is soaked with I-PRF it prolongs the cytokine release by 6 days, but induces proinflammatory cytokine release from PRF (Herrera-Vizcaíno et al., 2020). Compressing A-PRF+ with a collagen membrane, however, results in the highest growth factor release within 24 h (Blatt et al., 2020). Two studies by Blatt et al. (2020) have shown varying results with PRF and collagen combinations (Sebastian et al., 2022). In 2020, they found that pressed PRF (177 × g for 8 min) combined with three different porcine collagen membranes led to more new blood vessels and branching points than native materials (Blatt et al., 2020). In 2022, they reported that porcine- and bovine-derived membranes combined with PRF (177 × g for 8 min) had the same impact on new blood vessel formation as native membranes after 72 h (Sebastian et al., 2022). Hoda et al. (2021) showed that a three-collagen membrane incubated with A-PRF for 2 h increased gingival fibroblast adherence threefold and human osteosarcoma adhesion twofold. This effect was not seen with cell-free human dermal matrix or porcine-origin collagen matrix. A separate study by Park et al. (2018) showed that the addition of L-PRF to a porcine-derived collagen matrix significantly improves cell activity and mature endothelial cell migration by almost 8-fold.
In vivo studies and case reports show that I-PRF-soaked collagen matrices provide better results for gingival recession, extraction sockets, and sinus floor augmentation (Santamaria et al., 2023; Michels et al., 2023; Gülşen and Dereci, 2019) (Table 6). For gingival recession treatment, collagen membranes soaked with I-PRF achieved higher overall root coverage by 9.3% after 1 month, 15.6% after 3 months, and 13% after 6 months compared to native membranes (Patra et al., 2022). In another instance, Pandikanda et al. successfully treated oro-antral communication using minced L-PRF mixed with a collagen sponge, resulting in no complications and sustained vestibular depth (Pandikanda et al., 2019).
Compared to collagen membranes, there are fewer reports on gelatin in combination with PRF. Gelatin gels can effectively carry growth factors from PRF and prolong the growth factor release in the surrounding environment. (Suzuki et al., 2013; Mu et al., 2020). Gelatin nanoparticles (GNPs) are inherently fragile, with a compressive modulus of 9.2 ± 2.7 kPa and a tensile modulus of 14.1 ± 3.1 kPa. However, adding I-PRF to the hydrogel enhances its resistance to compressive (32.7 ± 4.6 kPa) and tensile forces (Elastic modulus 25.3 ± 4.1 kPa). These composite hydrogels exhibited self-healing and shear-thinning properties, making them suitable for injections before complete solidification. In a rabbit model for sinus augmentation, the GNP I-PRF composite led to significantly more new bone formation, better lamellar bone maturation, and improved new bone height and area compared to GNP gel alone over 8 weeks (Mu et al., 2020). Similarly, Yuan et al. (2021) observed that GNPs + I-PRF could be smoothly injected and maintained their form in water for 72 h even after shaking. The 20 w/v% GNPs in I-PRF hydrogel showed the highest toughness, with a yield stress of 33.2 kPa compared to 15 w/v% and 12 w/v% gels. This combination also resulted in higher bone density and blood vessel percentage area of the alveolar ridge in a canine model compared to DBBM and DBBM with PRF. Additionally, positive results were obtained when gelatin sponge pieces were combined with L-PRF for furcation defect treatment, showing improvements in horizontal and vertical clinical attachment and probing pocket depth. However, the study did not include a control group of only gelatin sponges without PRF, making it difficult to assess the specific impact of PRF (Ahuja et al., 2022).
3.3 Silk
In nature, silk is produced by certain lepidopteran larvae like silkworms, spiders, scorpions, and flies. Silk fibroin is made of repetitive protein sequences and composed of β-sheet structures (Bini et al., 2004). Silk biomaterials can be improved via amino acid side chain modifications or combining it with different biomaterials, thus allowing for broader applications. These types of materials are biocompatible and useful in wound healing and tissue engineering of bone, cartilage, tendon, and ligament (Vepari and Kaplan, 2007). Although silk is mostly used for sutures, some research groups are using silk proteins to produce scaffolds, hydrogels, and films for tissue repair (Holland et al., 2019).
Since 2013 only one study has investigated PRF combinations with silk biomaterials. This study done on patients showed similar results for implant stability quotients at 3 months between silk fibroin powder + cut PRF clot (type not specified) and PRF alone (57.0 ± 5.29 vs. 58.6 ± 4.95, respectively), but after 6 months the implant stability quotients for silk fibroin + PRF group (76.8 +/−3.65) was significantly higher than PRF (66.80 +/−5.79). Authors observed that bone density after 6 months was significantly higher with Silk fibroin + PRF (418.8 ± 181.3) compared to PRF (345.5 ± 179.5) (Ramy Salah et al., 2021). Although studies show that Silk fibroin with PRF has the potential to help in new bone regeneration studies comparing this composite to native silk fibroin are needed.
4 Composites
Composite materials retain the mechanical and biological properties of their components, making them widely used in tissue engineering. They are particularly useful in maxillofacial surgery due to their ability to be shaped for complex bone structures (Huang et al., 2024). An example is the use of 3D printed PCL/β-TCP composite scaffolds for facial bone reconstruction, combining PCL’s mechanical properties with β-TCP’s bone-mimicking characteristics (Jeong et al., 2022). This section focuses on studies where PRF is added to composite materials, analyzing three groups: composites with ceramic materials, polymer-polymer composites, and 3D-printed composite materials.
4.1 PRF with inorganic composite materials
Composite materials with inorganic compounds typically consist of polymers like collagen, gelatin, PLGA, and PCL, combined with an inorganic phase such as CaP or metals. This review summarizes 13 studies (Table 7), focusing on the combination of PRF with these materials. The most common method is mixing PRF with CaP to create sticky bone (Peker et al., 2016; Zhang Yue et al., 2023; Bastami et al., 2022), followed by coating synthesized scaffolds via impregnation (Zheng et al., 2015; Espitia-Quiroz et al., 2022) electrically binding to microspheres, or layering in multilayer scaffolds (Zhang L. et al., 2019; Alhasyimi et al., 2017; Alhasyimi et al., 2018; Zhang L. et al., 2023). The impact of PRF on the mechanical properties of these biomaterials is rarely documented. Tarif et al. reported that decellularized PRF coating on strontium-doped porous magnesium phosphate scaffolds did not affect the ultimate compressive strength (Tarif et al., 2023). Beiranvand et al. observed that I-PRF and hydroxyapatite (HA) coating enhanced the hydrophilicity of 3D-printed PCL scaffolds. They also found that platelet concentrate improved preosteoblast viability, doubling cell proliferation after 7 days, and increased RUNX2 gene expression on PCL/HA/PRF scaffolds after 14 days compared to PCL/HA scaffolds (Beiranvand et al., 2022). Similar improvements in cell viability were noted on nHA/PLGA scaffolds impregnated with PRF growth factors, L-PRF-coated HA scaffolds combined with collagen and PLGA copolymer, and L-PRF incorporated into a triple-layer scaffold consisting of an electrospun PCL/gelatin top layer and chitosan/poly (y-glutamic acid)/nHA hydrogel bottom layer (Zhang L. et al., 2019; Zhang L. et al., 2023).
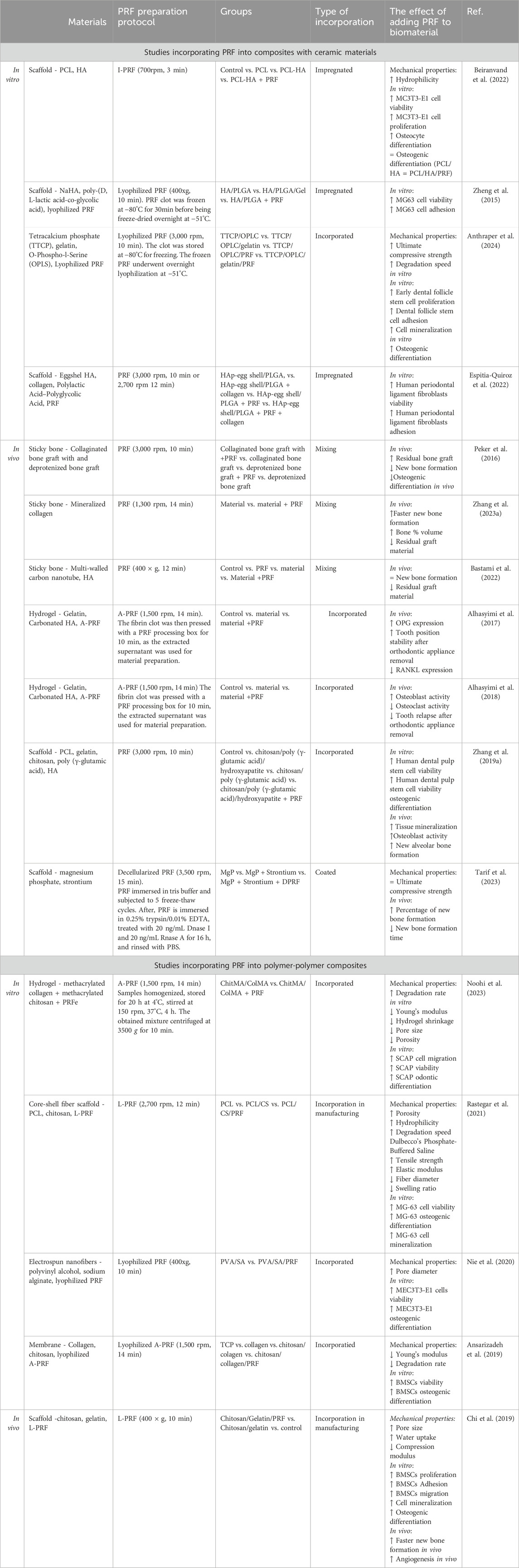
Table 7. Summarized results from studies incorporating PRF into composites with ceramic materials and composites with polymer materials.
Results from in vivo studies suggest similar results. Only two studies showed that the addition of PRF does not improve new bone formation - Peker et al. studied sticky bone made from collaginated bone graft and L-PRF and deproteinized bone graft with PRF for sinus floor augmentation. The authors saw insignificant differences between groups with and without PRF (Peker et al., 2016). Similarly, Bastami et al. (2022) found only slight, insignificant changes in bone defect healing in sheep using sticky bone made with multi-welled carbon nanotubes, HA, and minced PRF clots. In contrast, Alhasyimi et al. (2017); Alhasyimi et al. (2018) demonstrated that gelatin, carbonated HA, and A-PRF injectable hydrogel retain tooth position longer after orthodontic appliance removal by improving osteoblast activity and inhibiting osteoclast activity. Zhang L. et al. (2019); Zhang L. et al. (2023) triple-layer scaffold, consisting of an electrospun PCL/gelatin top layer and a chitosan/poly (y-glutamic acid)/nHA hydrogel bottom layer, showed enhanced healing in rat and New Zealand white rabbit models, with increased new bone tissue formation, higher OPN protein expression, and improved organization and collagen deposition in histological analyses. Additionally, in vivo studies observed quicker degradation of graft materials when PRF was used (Zhang Yue et al., 2023; Bastami et al., 2022). This could be due to the cell-mediated degradation of CaP materials and the PRF-induced promotion of cell migration, leading to faster biomaterial phagocytosis (Chi et al., 2019; Tajvar et al., 2023). Despite the lack of detailed information on the mechanical properties of these scaffolds, the overall results suggest that PRF serves as a beneficial growth factor source, enhancing the osteogenic effects of composite scaffolds.
4.2 PRF with polymer composite materials
For polymer-polymer composites, PRF has been incorporated during the fabrication process, similar to the methods shown in Figure 1. Analysis of the mechanical properties of these composites (Table 7) reveals varied effects. Out of five studies on different polymer-based compositions, three reported that adding PRF to biomaterials reduced mechanical durability (Chi et al., 2019; Noohi et al., 2023; Ansarizadeh et al., 2019). However, a PCL/chitosan core-shell fiber scaffold loaded with L-PRF showed increased tensile strength and elastic modulus (Rastegar et al., 2021). Incorporating PRF during scaffold fabrication often results in significant morphological changes. For example, adding decellularized L-PRF into gelatin and chitosan scaffolds, as well as L-PRF into electrospun nanofibers made from polyvinyl alcohol and sodium alginate, increased pore size (Chi et al., 2019; Nie et al., 2020), but L-PRF incorporation into PCL/chitosan core-shell fibers increased scaffold porosity (Rastegar et al., 2021). In contrast, adding PRF extract to methacrylated collagen (ColMa) and methacrylated gelatin (GelMa) hydrogel reduced both pore size and porosity (Noohi et al., 2023). Chi et al. (2019) observed that PRF improved the chitosan/gelatin scaffold water absorption, Noohi et al. (2023) found that PRF extracts reduced ColMa/GelMa hydrogel shrinkage, but Rastegar et al. (2021) noted that L-PRF decreased scaffold swelling ratio.
In vitro testing of all the polymer composites with PRF demonstrated positive results, boosted bone mesenchymal stem cell (BMSCs) proliferation, adhesion, and osteogenic differentiation were shown with chitosan/gelatin/L-PRF scaffolds and collagen/chitosan/lyophilized A-PRF membranes (Chi et al., 2019; Ansarizadeh et al., 2019). ColMa/GelMa/PRF enhanced odontic differentiation in stem cells from apical papilla (SCAP) (Noohi et al., 2023). Electrospun nanofibers from polyvinyl alcohol/sodium alginate and incorporated lyophilized L-PRF demonstrated better preosteoblast proliferation and osteogenic differentiation compared to nanofibers without L-PRF (Nie et al., 2020).
Although these biomaterials showed positive in vitro results, they lack extensive in vivo testing. Only Chi et al. (2019) tested their material in rat models, where micro-CT analysis showed greater bone volume and formation at 4- and 8 weeks post-implantation. Histological evaluation revealed vascularized bone tissue in groups treated with the chitosan/gelatin/L-PRF scaffold after 8 weeks.
These results show that even though PRF significantly improves biomaterial biocompatibility improving osteogenetic and angiogenetic properties, PRF impairs the mechanical properties of the materials.
4.3 3D printed PRF composite materials
3D bioprinting involves layer-by-layer deposition of biological materials to create structures mimicking living tissues or organs. This combines 3D printing with regenerative medicine to construct functional biological structures for medical applications (Mamo et al., 2023) Recently, 3D printing has been applied in bone tissue engineering and dentistry, allowing for precise fabrication of structures with biocompatible materials (Hadad et al., 2023; Haleem et al., 2020) It offers solutions for creating custom-designed scaffolds that mimic natural bone architecture and can improve the mechanical and biological properties of composite biomaterials (Tavoni et al., 2021).
Since 2018, several research groups have integrated PRF into bio-inks for 3D printing, demonstrating promising outcomes for both soft and hard tissue regeneration in animal studies (Table 8) (Sui et al., 2023; Song et al., 2018; Yi et al., 2022; Grandjean et al., 2024). Song et al. and Sui et al. have prepared 3D-printed scaffolds with PRF for mimicking bone tissue (Sui et al., 2023; Song et al., 2018). An alginate-gelatin and I-PRF scaffold designed by Yi et al. (2022) was aimed to help heal oral soft tissue. The enhanced biological properties of these scaffolds likely stem from PRF’s growth factors, which enhance cell adhesion through improved surface hydrophilicity (Bjelić and Finšgar, 2021). Second, Song et al. and Yi et al. noted that the printed scaffolds had rougher surfaces which could be due to the incorporated fibrin (Khampieng et al., 2018). However, incorporating PRF into 3D inks presents challenges. Yi et al. (2022) found that adding fresh I-PRF to alginate/gelatin ink decreased viscosity and reduced scaffold compressive strength, whereas Sui et al. (2023) using lyophilized L-PRF in L-PRF/chitosan/hydroxyapatite bio-ink observed increased viscosity. The viscosity of the bioink is not influenced only by the PRF but also the rest of the components. Crosslinking between PRF and the materials can start even before the printing process, requiring careful optimization through physical-chemical experiments before printing (Yi et al., 2022; Grandjean et al., 2024). The addition of lyophilized L-PRF did not improve the scaffold’s mechanical properties and with the increase of L-PRF concentration in the scaffold compressive modulus reduced (Sui et al., 2023). Song et al. (2018) also observed a similar reduction when incorporating PRF granules into BCP/PVA bio-ink. This means that the improvement of biological properties of 3D printed scaffolds by PRF comes with a cost of the materials’ mechanical properties, which limits the potential uses of the material.
5 Discussion
PRF enhances tissue regeneration across various biomaterials by promoting immunomodulatory protein corona formation, thereby facilitating cell attachment (Fernandez-Medina et al., 2023). The composition of protein corona on a material can impact the cell morphology and viability as well as the release profile of cytokines from the attached cells (Serpooshan et al., 2015). Studies demonstrate that PRF significantly improves cell adhesion (up to 13%) and proliferation, particularly on synthetic polymers (Chi et al., 2019; Al-Maawi et al., 2021; Hoda et al., 2021). Improved osteoblastic differentiation by higher ALP levels is observed in listed types of materials, like zinc, tricalcium phosphate, and xenogenic bone substitute materials (Blatt et al., 2021a; Molenda and Kolmas, 2011). Changes in osteoconduction are also observed as PRF composites improved Collagen I alpha-1 gene expression and calcium mineralization (Zhang L. et al., 2019; Song et al., 2018; Chi et al., 2019; van Orten et al., 2022; Blatt et al., 2021b; Rastegar et al., 2021; Zhang L. et al., 2023). Combining PRF with biomaterials prolongs cytokine release, supporting prolonged cellular activities crucial for tissue regeneration, including proliferation, migration, and differentiation (Sui et al., 2023; Song et al., 2018; Chi et al., 2019; Blatt et al., 2021a; Zheng et al., 2015; Le et al., 2023). This sustained release promotes persistent angiogenic responses, crucial for supporting blood vessel formation in damaged tissues and enhancing overall tissue regeneration success (Nurkesh et al., 2020; Ucuzian et al., 2010). The higher concentrations and prolonged release of growth factors from PRF compared to whole blood likely contribute to pronounced blood vessel formation and increased branching points when PRF is integrated with materials (Sebastian et al., 2022; Yi et al., 2022; Blatt et al., 2020; Nishimoto et al., 2015; Egle et al., 2021).
When looking at these composites in a bigger picture – in vivo and clinical studies show mostly positive results. In clinical studies, the most tested materials are CaP, bioglass, silk, collagen, and xenogenic bone grafts. Research of composite materials with PRF is limited to animal in vivo studies. The most coherent observations from these studies are that the PRF reduces the necessary time for new bone tissue formation (Zhang L. et al., 2019; Yilmaz et al., 2014; Tiwari et al., 2020; Zhang L. et al., 2023; Baghele et al., 2023; Abd-Elkawi et al., 2023). This acceleration is particularly evident during early healing stages, and control groups without PRF tend to achieve similar tissue formation levels in later stages (Song et al., 2018; Tarif et al., 2023; Abdullah, 2016; Alkafarani and Baban, 2019; Zhang Yue et al., 2023). Similarly, the addition of PRF reduces healing time after dental implant insertion and improves its stability (Hwan Jung et al., 2020; Pichotano et al., 2018; Pichotano et al., 2019; Ramy Salah et al., 2021; Angelo et al., 2015; Tabrizi et al., 2018; Potres et al., 2016; Mohamed Abdel-Aziz et al., 2023). These findings are crucial as they potentially alleviate healthcare burdens by minimizing patient recovery periods and reducing the duration of healthcare facility stays (Sen, 2021).
In vivo experiments have shown that biomaterials degrade more rapidly when combined with PRF, particularly noted in studies involving CaPs and their composites (Song et al., 2018; Nizam et al., 2018; Zhang Yue et al., 2023; Bastami et al., 2022). The reason for the observed effect of PRF on biomaterial degradation is still unknown and worth studying in future research. Several mechanisms may contribute to this phenomenon, including hydrolytic deposition, cell-mediated degradation, and loss of scaffold integrity due to mechanical stresses (Tajvar et al., 2023). One plausible mechanism involves leukocytes present in PRF, which can generate reactive oxygen species (ROS) such as hydrogen peroxide (H2O2), nitric oxide (NO), and superoxide (O2−). These ROS can degrade biomaterials by initiating hydrogen atom separation from polymer chains and initiating propagation reactions (Tajvar et al., 2023). Additionally, PRF contains matrix metalloproteinases (MMPs) that are involved in tissue remodeling and can contribute to collagen and its derivative degradation (Tajvar et al., 2023; Eren et al., 2016; Stamenkovic, 2003). In bioceramic degradation, a big role is played by osteoclasts that absorb CaPs like bone minerals (Tajvar et al., 2023). Unfortunately, PRF effects on osteoclastogenesis are inconclusive. Multiple studies show that PRF inhibits osteoclast activity and differentiation (Kumar et al., 2021; Kumar et al., 2019; Kargarpour et al., 2020), while others show that PRF mixing with biomaterials induces higher levels of proinflammatory cytokines (IL-6 and TNF-α) that can activate osteoclastogenesis (Park et al., 2023).
While many studies report positive outcomes from incorporating PRF into biomaterials, there remain inconclusive results, possibly due to variations in PRF protocol types. This article identifies specific PRF protocols used in included studies to explore how these choices affect tissue regeneration, though not all publications provided detailed PRF protocols. Adding to this issue, authors frequently deviate from established protocols. Commonly used PRF protocols include L-PRF (3,000 rpm or 400 g for 10 min), A-PRF (1,500 rpm for 14 min), and I-PRF (700 rpm for 3 min) (Dohan et al., 2006; Miron et al., 2017; Ghanaati et al., 2014). However, variations such as using 2,700 rpm for 3 min for I-PRF or 3,500 rpm for 15 min for clot-type PRF have been observed, complicating result analysis (Tarif et al., 2023; abou shabana et al., 2023; Fabbro et al., 2013). High centrifugation speeds (higher than 400 × g) for longer than 8 min reduce the leukocyte and platelet concentration for clot-type PRFs which could alter the healing properties (Miron et al., 2020). Moreover, differences in centrifuge equipment, including vibration frequencies, can impact cell populations within these clots (Dohan et al., 2018). Additionally, variations in platelet counts can occur both between individuals and within the same individual at different times of the day (Mazzocca et al., 2012). In conclusion, while combining PRF with bone graft materials shows promise for enhancing bone regeneration and healing, findings vary among studies. Standardized protocols and more extensive clinical trials are essential to fully understand and optimize these combinations. Researchers and clinicians should consider the concept of lower centrifugation speeds to maximize growth factor concentrations. The method of incorporating PRF into materials is also crucial; distributing PRF throughout the material prolongs its bioactive effects due to physical constraints imposed by scaffolds. Integration of PRF with scaffolds influences their physicochemical properties, necessitating thorough experimental studies to determine suitable mechanical and biological properties for specific procedures.
Author contributions
LI: Writing–original draft, Visualization. AD: Writing–review and editing, Supervision, Funding acquisition.
Funding
The author(s) declare that financial support was received for the research, authorship, and/or publication of this article. This research was funded by the European Union’s Horizon 2020 research and innovation program (grant number 857287) and the Latvian Council of Science research project (grant number lzp-2020/1-0054) “Development of antibacterial autologous fibrin matrices in maxillofacial surgery (MATRI-X)”. The European Union’s Recovery and Resilience Facility project Nr. 5.2.1.1.i.0/2/24/I/CFLA/003 grant agreement grant nr. 1023 “Investigation of the interaction of platelet-rich fibrin with biomaterials used in oral, facial and maxillofacial surgery”.
Conflict of interest
The authors declare that the research was conducted in the absence of any commercial or financial relationships that could be construed as a potential conflict of interest.
Publisher’s note
All claims expressed in this article are solely those of the authors and do not necessarily represent those of their affiliated organizations, or those of the publisher, the editors and the reviewers. Any product that may be evaluated in this article, or claim that may be made by its manufacturer, is not guaranteed or endorsed by the publisher.
References
Abd-Elkawi, M., Sharshar, A., Misk, T., Elgohary, I., and Gadallah, S. (2023). Effect of calcium carbonate nanoparticles, silver nanoparticles and advanced platelet-rich fibrin for enhancing bone healing in a rabbit model. Sci. Rep. 13, 15232. doi:10.1038/s41598-023-42292-x
Abdullah, W. A. (2016). Evaluation of bone regenerative capacity in rats claverial bone defect using platelet rich fibrin with and without beta tri calcium phosphate bone graft material. Saudi Dent. J. 28, 109–117. doi:10.1016/j.sdentj.2015.09.003
abou shabana, mohamed, mohaed, mohamed, and El, F. A. (2023). Effect of using tri-calcium phosphate combined with platelet rich fibrin versus platelet rich plasma in post extraction socket. Al-Azhar J. Dent. Sci. 26, 335–343. doi:10.21608/ajdsm.2021.76667.1201
Acar, A. H., Yolcu, Ü., Gül, M., Keleş, A., Erdem, N. F., and Altundag Kahraman, S. (2015). Micro-computed tomography and histomorphometric analysis of the effects of platelet-rich fibrin on bone regeneration in the rabbit calvarium. Arch. Oral Biol. 60, 606–614. doi:10.1016/j.archoralbio.2014.09.017
Agrawal, I., Chandran, S., and Priyadarshini, N. (2017). Comparative evaluation of the efficacy of platelet-rich fibrin and calcium phosphosilicate putty alone and in combination in the treatment of intrabony defects: a randomized clinical and radiographic study. Contemp. Clin. Dent. 8, 205–210. doi:10.4103/ccd.ccd_1147_16
Ahuja, A., Ahuja, V., Saha, A., Singhal, A., Priya, T., and Bhattacharjee, A. (2022). Effectiveness of gelatin sponge along with i-PRF in endodontically involved tooth with grade II furcation defects: a clinical and radiographic study. J. Contemp. Dent. Pract. 23, 1199–1202. doi:10.5005/jp-journals-10024-3449
Alberto, C., Valladão, A., Monteiro, M. F., and Joly, J. C. (2020). Guided bone regeneration in staged vertical and horizontal bone augmentation using platelet-rich fibrin associated with bone grafts: a retrospective clinical study. Int. J. Implant Dent. 6, 1–10. doi:10.1186/s40729-020-00266-y
Alhasyimi, A. A., Pudyani, P. P., Asmara, W., and Ana, I. D. (2018). Enhancement of post-orthodontic tooth stability by carbonated hydroxyapatite-incorporated advanced platelet-rich fibrin in rabbits. Orthod. Craniofac Res. 21, 112–118. doi:10.1111/ocr.12224
Alhasyimi, A. A., Pudyani, P. S., Asmara, W., and Ana, I. D. (2017). Locally inhibition of orthodontic relapse by injection of carbonated hydroxy apatite-Advanced platelet rich fibrin in a rabbit model. Key Eng. Mater 758 KEM, 255–263. Trans Tech Publications Ltd. doi:10.4028/www.scientific.net/KEM.758.255
Alkafarani, W., and Baban, L. (2019). Augmentation of surgically created bony defects using biphasic calcium phosphate with and without platelet rich fibrin: an experimental study in sheep. J. Univ. Duhok 22, 229–242. doi:10.26682/sjuod.2019.22.2.26
Al-Maawi, S., Dohle, E., Lim, J., Weigl, P., Teoh, S. H., Sader, R., et al. (2021). Biologization of pcl-mesh using platelet rich fibrin (prf) enhances its regenerative potential in vitro. Int. J. Mol. Sci. 22, 1–15. doi:10.3390/ijms22042159
Al-Maawi, S., Herrera-Vizcaíno, C., Orlowska, A., Willershausen, I., Sader, R., Miron, R. J., et al. (2019). Materials biologization of collagen-based biomaterials using liquid-platelet-rich fibrin: new insights into clinically applicable tissue engineering. Materials 12, 1–17. doi:10.3390/ma12233993
Almeida Barros Mourão, C. F. De, Valiense, H., Melo, E. R., Freitas Mourão, N. B. M., and Maia, M. D. C. (2015). Obtention of injectable platelets rich-fibrin (i-PRF) and its polymerization with bone graft: technical note. Rev. Col. Bras. Cir. 42, 421–423. doi:10.1590/0100-69912015006013
Alotaibi, N., Zulkiflee, A. B., Damsaz, M., Zumarán Castagnoli, C., Eshghpour, M., Hamidi Alamdari, D., et al. (2020). Evidence-based clinical efficacy of leukocyte and platelet-rich fibrin in maxillary sinus floor lift, graft and surgical augmentation procedures. Front. Surg. 7, 1–13. doi:10.3389/fsurg.2020.537138
Amam, M. A., Abdo, A., Alnour, A., Amam, A., and Jaafo, M. H. (2023). Comparison of calcium sulfate and tricalcium phosphate in bone grafting after sinus lifting for dental implantation: a randomized controlled trial. Dent. Med. Probl. 60, 239–246. doi:10.17219/dmp/151983
Angelo, T., Marcel, W., Andreas, K., and Izabela, S. (2015). Biomechanical stability of dental implants in augmented maxillary sites: results of a randomized clinical study with four different biomaterials and PRF and a biological view on guided bone regeneration. Biomed. Res. Int. 2015, 1–17. doi:10.1155/2015/850340
Ansarizadeh, M., Mashayekhan, S., and Saadatmand, M. (2019). Fabrication, modeling and optimization of lyophilized advanced platelet rich fibrin in combination with collagen-chitosan as a guided bone regeneration membrane. Int. J. Biol. Macromol. 125, 383–391. doi:10.1016/j.ijbiomac.2018.12.078
Anthraper, M. S. J., Chandramouli, A., Srinivasan, S., and Rangasamy, J. (2024). Lyophilized platelet rich fibrin and gelatin incorporated bioadhesive bone cement composite for repair of mandibular continuity defects. Int. J. Biol. Macromol. 258, 129086. doi:10.1016/j.ijbiomac.2023.129086
Anwar, S. K., and Hamid, H. M. A. (2022). Immuno-histopathologic evaluation of mineralized plasmatic matrix in the management of horizontal ridge defects in a canine model (a split-mouth comparative study). Odontology 110, 523–534. doi:10.1007/s10266-021-00684-3
Baghele, O. N., Thorat, M. S., and Malpani, P. S. (2023). Clinical and radiographic evaluation of platelet-rich fibrin and bone graft material (β-tricalcium phosphate + hydroxyapatite) in the treatment of intrabony defects of periodontitis patients: a randomized controlled trial. Quintessence Int. 54, 472–483. doi:10.3290/J.QI.B3920301
Barbosa, L., Lourenço, S., De Azevedo, E., Santos, J. V., Rodrigues, S., Rocha, N., et al. (2023). Biomaterials the effects of platelet-rich fibrin in the behavior of mineralizing cells related to bone tissue regeneration-A scoping review of in vitro evidence. Funct. Biomater. 14, 1–35. doi:10.3390/jfb14100503
Basova, T. V., Vikulova, E. S., Dorovskikh, S. I., Hassan, A., and Morozova, N. B. (2021) The use of noble metal coatings and nanoparticles for the modification of medical implant materials. Materials & Design 204, 109672. doi:10.1016/j.matdes.2021.109672
Bastami, F., Noori-Kooshki, M. H., Semyari, H., Tabrizi, R., Abrishamchian, A., Mashhadi-Abbas, F., et al. (2022). Multi-walled carbon nanotube/hydroxyapatite nanocomposite with leukocyte- and platelet-rich fibrin for bone regeneration in sheep model. Oral Maxillofac. Surg. 26, 63–72. doi:10.1007/s10006-020-00933-9
Beiranvand, S. Y., Nourani, H., and Mehrjerdi, H. K. (2022). Fabrication of platelet-rich fibrin-coated polycaprolactone/hydroxyapatite (PCL-HA/PRF) 3D printed scaffolds for bone tissue engineering. Iran. J. Vet. Med. 16. doi:10.22059/IJVM.2022.335899.1005219
Bezwada, R. S., Jamiolkowski, D. D., Lee, I.-Y., Agarwal, V., Persivale, J., Trenka-Benthin, S., et al. (1995). Monocryl@ suture, a new ultra-pliable absorbable monofilament suture. Biomaterials. 16(15):1141–8. doi:10.1016/0142-9612(95)93577-z
Bi, L., Rahaman, M. N., Day, D. E., Brown, Z., Samujh, C., Liu, X., et al. (2013). Effect of bioactive borate glass microstructure on bone regeneration, angiogenesis, and hydroxyapatite conversion in a rat calvarial defect model. Acta Biomater. 9, 8015–8026. doi:10.1016/j.actbio.2013.04.043
Bini, E., Knight, D. P., and Kaplan, D. L. (2004). Mapping domain structures in silks from insects and spiders related to protein assembly. J. Mol. Biol. 335, 27–40. doi:10.1016/j.jmb.2003.10.043
Birk, D. E., and Bruckner, P. (2005). Collagen suprastructures. Top. Curr. Chem. 247, 185–205. doi:10.1007/b103823
Bjelić, D., and Finšgar, M. (2021). The role of growth factors in bioactive coatings. Pharmaceutics 13, 1083. doi:10.3390/pharmaceutics13071083
Blatt, S., Burkhardt, V., Kämmerer, P. W., Pabst, A. M., Sagheb, K., Heller, M., et al. (2020). Biofunctionalization of porcine-derived collagen matrices with platelet rich fibrin: influence on angiogenesis in vitro and in vivo. Clin. Oral Investig. 24, 3425–3436. doi:10.1007/s00784-020-03213-8
Blatt, S., Thiem, D. G. E., Kyyak, S., Pabst, A., Al-Nawas, B., and Kämmerer, P. W. (2021b). Possible implications for improved osteogenesis? The combination of platelet-rich fibrin with different bone substitute materials. Front. Bioeng. Biotechnol. 9, 640053. doi:10.3389/fbioe.2021.640053
Blatt, S., Thiem, D. G. E., Pabst, A., Al-Nawas, B., and Kämmerer, P. W. (2021a). Does platelet-rich fibrin enhance the early angiogenetic potential of different bone substitute materials? An in vitro and in vivo analysis. Biomedicines 9, 61–16. doi:10.3390/biomedicines9010061
Bölükbaşı, N., Yeniyol, S., Soluk Tekkesin, M., and Altunatmaz, K. (2013). The use of platelet-rich fibrin in combination with biphasic calcium phosphate in the treatment of bone defects: a histologic and histomorphometric study. Curr. Ther. Res. 75, 15–21. doi:10.1016/j.curtheres.2013.05.002
Burdus, A.-C., Gherasim, O., Mihai Grumezescu, A., Mogoantă, iu, Ficai, A., and Andronescu, E. (2018). Biomedical applications of silver nanoparticles: an up-to-date overview. Nanomaterials 8, 1–25. doi:10.3390/nano8090681
Caramês, J. M. M., Vieira, F. A., Caramês, G. B., Pinto, A. C., Francisco, H. C. O., and Marques, D. N. da S. (2022). Guided bone regeneration in the edentulous atrophic maxilla using deproteinized bovine bone mineral (DBBM) combined with platelet-rich fibrin (PRF)—a prospective study. J. Clin. Med. 11, 894. doi:10.3390/jcm11030894
Chen, M. C., Chiu, H. C., Kuo, P. J., Chiang, C. Y., Fu, M. M., and Fu, E. (2021). Bone formation with functionalized 3D printed poly-ε-caprolactone scaffold with plasma-rich-fibrin implanted in critical-sized calvaria defect of rat. J. Dent. Sci. 16, 1214–1221. doi:10.1016/j.jds.2021.01.015
Chi, H., Song, X., Song, C., Zhao, W., Chen, G., Jiang, A., et al. (2019). Chitosan-gelatin scaffolds incorporating decellularized platelet-rich fibrin promote bone regeneration. ACS Biomater. Sci. Eng. 5, 5305–5315. doi:10.1021/acsbiomaterials.9b00788
Choi, J. J. E., Zwirner, J., Ramani, R. S., Ma, S., Hussaini, H. M., Waddell, J. N., et al. (2020). Mechanical properties of human oral mucosa tissues are site dependent: a combined biomechanical, histological and ultrastructural approach. Clin. Exp. Dent. Res. 6, 602–611. doi:10.1002/cre2.305
Clark, D., Rajendran, Y., Paydar, S., Ho, S., Cox, D., Ryder, M., et al. (2018). Advanced platelet-rich fibrin and freeze-dried bone allograft for ridge preservation: a randomized controlled clinical trial. J. Periodontol. 89, 379–387. doi:10.1002/JPER.17-0466
Dakal, T. C., Kumar, A., Majumdar, R. S., and Yadav, V. (2016). Mechanistic basis of antimicrobial actions of silver nanoparticles. Front. Microbiol. 7, 1831. doi:10.3389/fmicb.2016.01831
Dambhare, A., Laxmanrao Bhongade, M., Dhadse, P. V., Sehdev, B., Kumar Ganji, K., Thakare, K., et al. (2019). A randomized controlled clinical study of autologous platelet rich fibrin (PRF) in combination with HA and beta-TCP or HA and beta-TCP alone for treatment of furcation defects. J. Hard Tissue Biol. 28, 185–190. doi:10.2485/JHTB.28.185
Davidenko, N., Carlos, •, Schuster, F., Daniel, •, Bax, V., Farndale, R. W., et al. (2016). Evaluation of cell binding to collagen and gelatin: a study of the effect of 2D and 3D architecture and surface chemistry. J. Mater Sci. Mater Med. 27, 148. doi:10.1007/s10856-016-5763-9
Debel, M., Toma, S., Vandenberghe, B., Brecx, M. C., and Lasserre, J. F. (2021). Alveolar ridge dimensional changes after two socket sealing techniques. A pilot randomized clinical trial. Clin. Oral Investig. 25, 1235–1243. doi:10.1007/s00784-020-03428-9
Deenadayalan, E., Kumar, A., Kumar Tewari, R., Mishra, S. K., and Iftekhar, H. (2015). Management of large preiapical lesion with the combination of second generation platelet extract and hydroxyapatite bone graft: a report of three cases. J. Clin. Diagnostic Res. 9, 24–27. doi:10.7860/jcdr/2015/10885.5482
Dohan, D. M., Choukroun, J., Diss, A., Dohan, S. L., Dohan, A. J. J., Mouhyi, J., et al. (2006). Platelet-rich fibrin (PRF): a second-generation platelet concentrate. Part I: technological concepts and evolution. Oral Surg. Oral Med. Oral Pathol. Oral Radiol. Endod. 101, e37–e44. doi:10.1016/j.tripleo.2005.07.008
Dohan, E. D. M., Andia, I., Zumstein, M. A., Zhang, C.-Q., Pinto, N. R., and Bielecki, T. (2014). Classification of platelet concentrates (Platelet-Rich Plasma-PRP, Platelet-Rich Fibrin-PRF) for topical and infiltrative use in orthopedic and sports medicine: current consensus, clinical implications and perspectives. Ligaments Tendons J. 4, 3–9. doi:10.11138/MLTJ/2014.4.1.0013
Dohan, E. D. M., Pinto, N. R., Pereda, A., Jiménez, P., Corso, M. D., Kang, B. S., et al. (2018). The impact of the centrifuge characteristics and centrifugation protocols on the cells, growth factors, and fibrin architecture of a leukocyte- and platelet-rich fibrin (L-PRF) clot and membrane. Platelets 29, 171–184. doi:10.1080/09537104.2017.1293812
Douglas, T. E. L., Gassling, V., Declercq, H. A., Purcz, N., Pamula, E., Haugen, H. J., et al. (2012). Enzymatically induced mineralization of platelet-rich fibrin. J. Biomed. Mater Res. A 100 (A), 1335–1346. doi:10.1002/jbm.a.34073
Egle, K., Salma, I., and Dubnika, A. (2021). From blood to regenerative tissue: how autologous platelet-rich fibrin can be combined with other materials to ensure controlled drug and growth factor release. Int. J. Mol. Sci. 22, 11553. doi:10.3390/ijms222111553
Eliaz, N., and Metoki, N. (2017). Calcium phosphate bioceramics: a review of their history, structure, properties, coating technologies and biomedical applications. Materials 10, 334–104. doi:10.3390/ma10040334
Elkholly, A., Negm, M., Hassan, R., and Omar, N. (2022). Healing assessment of osseous defects after surgical removal of periapical lesions in the presence of hydroxyapatite, nanohydroxyapatite, and a combination of nanohydroxyapatite and platelet-rich fibrin: a clinical study. Open Access Maced. J. Med. Sci. 10, 406–414. doi:10.3889/oamjms.2022.10766
Engelberg, I., and Kohn, J. (1991). Physico-mechanical properties of degradable polymers used in medical applications: a comparative study. Biomaterials 12, 292–304. doi:10.1016/0142-9612(91)90037-b
Eren, G., Gürkan, A., Atmaca, H., Dönmez, A., and Atilla, G. (2016). Effect of centrifugation time on growth factor and MMP release of an experimental platelet-rich fibrin-type product. Platelets 27, 427–432. doi:10.3109/09537104.2015.1131253
Espitia-Quiroz, L. C., Fernández-Orjuela, A. L., Anaya-Sampayo, L. M., Acosta-Gómez, A. P., Gonzalo Sequeda-Castañeda, L., Janeth Gutiérrez-Prieto, S., et al. (2022). Viability and adhesion of periodontal ligament fibroblasts on a hydroxyapatite scaffold combined with collagen, polylactic acid-polyglycolic acid copolymer and platelet-rich fibrin: a preclinical pilot study. Dent. J. 10, 1–12. doi:10.3390/dj10090167
Fabbro, M. D., Bortolin, M., Taschieri, S., and Weinstein, R. L. (2013). Effect of autologous growth factors in maxillary sinus augmentation: a systematic review. Clin. Implant Dent. Relat. Res. 15, 205–216. doi:10.1111/j.1708-8208.2011.00343.x
Feng, M., Wang, Y., Wei, Y., Zhang, X., Xiao, L., Gong, Z., et al. (2022). Preparation, characterization and biological properties of a novel bone block composed of platelet rich fibrin and a deproteinized bovine bone mineral. Fundam. Res. 2, 321–328. doi:10.1016/j.fmre.2021.08.003
Fernandez-Medina, T., Vaquette, C., Gomez-Cerezo, M. N., and Ivanovski, S. (2023). Characterization of the protein corona of three chairside hemoderivatives on melt electrowritten polycaprolactone scaffolds. Int. J. Mol. Sci. 24, 1–21. doi:10.3390/ijms24076162
Fu, X., Sammons, R. L., Bertóti, I., Jenkins, M. J., and Dong, H. (2012). Active screen plasma surface modification of polycaprolactone to improve cell attachment. J. Biomed. Mater Res. B Appl. Biomater. 100 B, 314–320. doi:10.1002/jbm.b.31916
Fujioka-Kobayashi, M., Miron, R. J., Hernandez, M., Kandalam, U., Zhang, Y., and Choukroun, J. (2017). Optimized platelet-rich fibrin with the low-speed concept: growth factor release, biocompatibility, and cellular response. J. Periodontol. 88, 112–121. doi:10.1902/jop.2016.160443
Gao, C., Peng, S., Feng, P., and Shuai, C. (2017). Bone biomaterials and interactions with stem cells. Bone Res. 5, 17059. doi:10.1038/boneres.2017.59
Gassling, V., Douglas, T. E. L., Purcz, N., Schaubroeck, D., Balcaen, L., Bliznuk, V., et al. (2013). Magnesium-enhanced enzymatically mineralized platelet-rich fibrin for bone regeneration applications. Biomed. Mater. (Bristol) 8, 055001–055010. doi:10.1088/1748-6041/8/5/055001
Ghanaati, S., Booms, P., Orlowska, A., Kubesch, A., Lorenz, J., Rutkowski, J., et al. (2014). Advanced platelet-rich fibrin: a new concept for cell- Based tissue engineering by means of inflammatory cells. J. Oral Implant. 40, 679–689. doi:10.1563/aaid-joi-D-14-00138
Ghaznavi, D., Babaloo, A., Shirmohammadi, A., Zamani, A. R. N., Azizi, M., Rahbarghazi, R., et al. (2019). Advanced platelet-rich fibrin plus gold nanoparticles enhanced the osteogenic capacity of human mesenchymal stem cells. BMC Res. Notes 12, 721–726. doi:10.1186/s13104-019-4750-x
Girija, K., and Kavitha, M. (2020). Comparative evaluation of platelet-rich fibrin, platelet-rich fibrin + 50 wt% nanohydroxyapatite, platelet-rich fibrin + 50 wt% dentin chips on odontoblastic differentiation - an in vitro study-part 2. J. Conserv. Dent. 23, 354. doi:10.4103/JCD.JCD_3_20
Grandjean, T., Perumal, N., Manicam, C., Matthey, B., Wu, T., Thiem, D. G. E., et al. (2024). Towards optimized tissue regeneration: a new 3D printable bioink of alginate/cellulose hydrogel loaded with thrombocyte concentrate. Front. Bioeng. Biotechnol. 12, 1363380. doi:10.3389/fbioe.2024.1363380
Grecu, A. F., Reclaru, L., Ardelean, L. C., Nica, O., Ciucă, E. M., and Ciurea, M. E. (2019). Platelet-rich fibrin and its emerging therapeutic benefits for musculoskeletal injury treatmen. Med. (B Aires) 55, 1–12. doi:10.3390/medicina55050141
Gülşen, U., and Dereci, Ö. (2019). Evaluation of new bone formation in sinus floor augmentation with injectable platelet-rich fibrin-soaked collagen plug: a pilot study. Implant Dent. 28, 220–225. doi:10.1097/ID.0000000000000883
Hadad, H., Boos Lima, FBDJ, Shirinbak, I., Porto, T. S., Chen, J. E., and Guastaldi, F. P. S. (2023). The impact of 3D printing on oral and maxillofacial surgery. J. 3D Print Med. 7. doi:10.2217/3dp-2022-0025
Haddadi, P., Khorshidi, H., Raoofi, S., Nazhvani, A. D., and Badiee, P. (2018). Comparative evaluation of conventional and nanosilver-containing leucocyte and platelet-rich fibrin/biomaterial in the anti-biofilm formation of standard species of Candida and streptococcus. Jundishapur J. Microbiol. 11. doi:10.5812/jjm.68423
Haghparast-Kenarsari, T., Abdouss, M., Heidari Keshel, S., Heshmatipour, Z., Rahimi, A., and Biazar, E. (2024). Improving properties of platelet-rich fibrin scaffold with tannic acid for wound healing. Int. Wound J. 21, e14571. doi:10.1111/iwj.14571
Haleem, A., Javaid, M., Hasan Khan, R., and Suman, R. (2020). 3D printing applications in bone tissue engineering. J. Clin. Orthop. Trauma 11, S118–S124. doi:10.1016/j.jcot.2019.12.002
hamuda, H., Hosny, A., and wael, elmohandes (2023). Application of platelet -rich fibrin with β-tricalcium phosphate for reconstruction of bone in periapical defects. Al-Azhar J. Dent. Sci. 26, 67–74. doi:10.21608/ajdsm.2021.64598.1175
Han, B., Duan, B., Kargozar, S., Liao, L., Ai, F., Borosilicate, B. C., et al. (2020). Customized borosilicate bioglass scaffolds with excellent biodegradation and osteogenesis for mandible reconstruction. Front. Bioeng. Biotechnol. | WwwFrontiersinOrg 8, 610284. doi:10.3389/fbioe.2020.610284
Hench, L. L., Polak, J. M., Xynos, I. D., and Buttery, L. D. K. (2000). Bioactive materials to control cell cycle. Mat. Res. Innov. 3, 313–323. doi:10.1007/s100190000055
Herrera-Vizcaíno, C., Al-Maawi, and S., Sader, and R., Kirkpatrick, C. J., Choukroun and, J., and Ghanaati, S. (2020). Modification of collagen-based sponges can induce an upshift of the early inflammatory response and a chronic inflammatory reaction led by M1 macrophages: an in vivo study. Clin. Oral Investig. 24, 3485–3500. doi:10.1007/s00784-020-03219-2
Hiremath, H., Motiwala, T., Jain, P., and Kulkarni, S. (2014). Use of second-generation platelet concentrate (platelet-rich fibrin) and hydroxyapatite in the management of large periapical inflammatory lesion: a computed tomography scan analysis. Indian J. Dent. Res. 25, 517–520. doi:10.4103/0970-9290.142556
Hoda, R. B., Zahra Farhad, S., and Amini Sadeh, S. (2021). Evaluation and comparison number of gingival fibroblast and osteosarcoma cell (MG-63 cell line) adhesive to mocugraft, alloderm, and collagen membrane with or without advanced platelet-rich fibrin. Dent. Res. J. (Isfahan) 1. doi:10.4103/1735-3327.328750
Holland, C., Numata, K., Rnjak-Kovacina, J., and Seib, F. P. (2019). The biomedical use of silk: past, present, future. Adv. Healthc. Mater 8, e1800465. doi:10.1002/adhm.201800465
Huang, X., Lou, Y., Duan, Y., Liu, H., Tian, J., Shen, Y., et al. (2024). Biomaterial scaffolds in maxillofacial bone tissue engineering: a review of recent advances. Bioact. Mater 33, 129–156. doi:10.1016/j.bioactmat.2023.10.031
Hwang, B.-Y., Noh, K., and Lee, J.-W. (2023). Long-term follow-up of a novel surgical option combining fibula free flap and 3D-bioprinted, patient-specific polycaprolactone (PCL) implant for mandible reconstruction. Bioeng. (Basel). 10, 684. doi:10.3390/bioengineering10060684
Hwan Jung, M., Hun Lee, J., Wadhwa, P., Bo Jiang, H., Seok, J. H., and Seok, L. E. (2020). Bone regeneration in peri-implant defect using autogenous tooth biomaterial enriched with platelet-rich fibrin in animal model. Appl. Sci. 10, 1–11. doi:10.3390/app10061939
Inchingolo, F., Tatullo, M., Marrelli, M., Inchingolo, A. M., Scacco, S., Inchingolo, A. D., et al. (2010). Trial with Platelet-Rich Fibrin and Bio-Oss used as grafting materials in the treatment of the severe maxillar bone atrophy: clinical and radiological evaluations. Eur. Rev. Med. Pharmacol. Sci. 14, 1075–1084.
Isobe, K., Watanebe, T., Kawabata, H., Kitamura, Y., Okudera, T., Okudera, H., et al. (2017). Mechanical and degradation properties of advanced platelet-rich fibrin (A-PRF), concentrated growth factors (CGF), and platelet-poor plasma-derived fibrin (PPTF). Int. J. Implant Dent. 3, 17. doi:10.1186/s40729-017-0081-7
Jeong, J., Kim, J. H., Shim, J. H., Hwang, N. S., and Heo, C. Y. (2019). Bioactive calcium phosphate materials and applications in bone regeneration. Biomater. Res. 23, 4. doi:10.1186/s40824-018-0149-3
Jeong, W.-S., Kim, Y.-C., Min, J. C., Park, H. J., Lee, E. J., Shim, J. H., et al. (2022). Clinical application of 3D-printed patient-specific polycaprolactone/beta tricalcium phosphate scaffold for complex zygomatico-maxillary defects. Polym. (Basel). 14, 740. doi:10.3390/polym14040740
Jiang, J., Pi, J., Cai, J., and Fanizzi, F. P. (2018). The advancing of zinc oxide nanoparticles for biomedical applications. Bioinorg. Chem. Appl. 2018, 1–18. doi:10.1155/2018/1062562
Kalpana, V. N., Rajeswari, V. D., and Fanizzi, F. P. (2018). A review on green synthesis, biomedical applications, and toxicity studies of ZnO NPs. Bioinorg Chem Appl. 2018;3569758. doi:10.1155/2018/3569758
Kamal, A., Morsy, R. A., and K, E. (2017). Bone regeneration potential of beta tri-calcium phosphate and platelet rich fibrin in localized alveolar ridge defect with immediately placed dental implant. (an experimental study in dog). Oral Surg. Egypt. Dent. J. 63, 307–316. doi:10.21608/edj.2017.74744
Karayürek, F., Kadiroğlu, E. T., Nergiz, Y., Coşkun Akçay, N., Tunik, S., Ersöz, K. B., et al. (2019). Combining platelet rich fibrin with different bone graft materials: an experimental study on the histopathological and immunohistochemical aspects of bone healing. J. Cranio-Maxillofacial Surg. 47, 815–825. doi:10.1016/J.JCMS.2019.01.023
Kargarpour, Z., Nasirzade, J., Strauss, F. J., Di Summa, F., Hasannia, S., Müller, H. D., et al. (2020). Platelet-rich fibrin suppresses in vitro osteoclastogenesis. J. Periodontol. 91, 413–421. doi:10.1002/JPER.19-0109
Khampieng, T., Yamassatien, V., Ekabutr, P., Pavasant, P., and Supaphol, P. (2018). Protein adsorption and cell behaviors on polycaprolactone film: the effect of surface topography. Adv. Polym. Technol. 37, 2030–2042. doi:10.1002/adv.21861
Khorshidi, H., Haddadi, P., Raoofi, S., Badiee, P., and Nazhvani, A. D. (2018). Does adding silver nanoparticles to leukocyte-and platelet-rich fibrin improve its properties? Biomed. Res. Int. 27, 1–5. doi:10.1155/2018/8515829
Knapen, M., Gheldof, D., Drion, P., Layrolle, P., Rompen, E., and Lambert, F. (2015). Effect of leukocyte- and platelet-rich fibrin (L-PRF) on bone regeneration: a study in rabbits. Clin. Implant Dent. Relat. Res. 17, e143–e152. doi:10.1111/cid.12146
Krishnan, V., and Lakshmi, T. (2013). Bioglass: a novel biocompatible innovation. J. Adv. Pharm. Technol. Res. 4, 78–83. doi:10.4103/2231-4040.111523
Kumar, A., Mahendra, J., Mahendra, L., Abdulkarim, H. H., Sayed, M., Mugri, M. H., et al. (2021). Synergistic effect of biphasic calcium phosphate and platelet-rich fibrin attenuate markers for inflammation and osteoclast differentiation by suppressing nf-κb/mapk signaling pathway in chronic periodontitis. Molecules 26, 6578. doi:10.3390/molecules26216578
Kumar, A., Mahendra, J., Samuel, S., Govindraj, J., Loganathan, T., Vashum, Y., et al. (2019). Platelet-rich fibrin/biphasic calcium phosphate impairs osteoclast differentiation and promotes apoptosis by the intrinsic mitochondrial pathway in chronic periodontitis. J. Periodontol. 90, 61–71. doi:10.1002/JPER.17-0306
Kumar, P., Vinitha, B., and Fathima, G. (2013). Bone grafts in dentistry. J. Pharm. Bioallied Sci. 5, 125. doi:10.4103/0975-7406.113312
Lara, C., Bezmalinovic, A., García-Herrera, C., Ríos, S., Valenzuela, L. M., and Martínez, C. E. (2023). Leukocyte- and platelet-rich fibrin (L-PRF) obtained from smokers and nonsmokers shows a similar uniaxial tensile response in vitro. Biomedicines 11, 1–12. doi:10.3390/biomedicines11123286
Le, A. D. K., Enweze, L., DeBaun, M. R., and Dragoo, J. L. (2018). Current clinical recommendations for use of platelet-rich plasma. Curr. Rev. Musculoskelet. Med. 11, 624–634. doi:10.1007/s12178-018-9527-7
Le, T. T. V., Phan, N. T. H., and Tran, H. L. B. (2023). Alginate–gelatin hydrogel supplemented with platelet concentrates can be used as bioinks for scaffold printing. Asian Biomed. 17, 222–229. doi:10.2478/abm-2023-0063
Lee, Y.-K., Wadhwa, P., Cai, H., Jung, S.-U., Zhao, B. C., Rim, J.-S., et al. (2021). Micro-CT and histomorphometric study of bone regeneration effect with autogenous tooth biomaterial enriched with platelet-rich fibrin in an animal model. Scanning 2021, 1–7. doi:10.1155/2021/6656791
Lei, L., Yu, Y., Ke, T., Sun, W., and Chen, L. (2019). The application of three-dimensional printing model and platelet-rich fibrin technology in guided tissue regeneration surgery for severe bone defects. J. Oral Implant. 45, 35–43. doi:10.1563/aaid-joi-D-17-00231
Liu, R., Yan, M., Chen, S., Huang, W., Wu, D., and Chen, J. (2019). Effectiveness of platelet-rich fibrin as an adjunctive material to bone graft in maxillary sinus augmentation: a meta-analysis of randomized controlled trails. Biomed. Res. Int. 2019, 1–10. doi:10.1155/2019/7267062
Lorenz, J., Al-Maawi, S., Sader, R., and Ghanaati, S. (2018). Individualized titanium mesh combined with platelet-rich fibrin and deproteinized bovine bone: a new approach for challenging augmentation. J. Oral Implant. 44, 345–351. doi:10.1563/aaid-joi-D-18-00049
Luyendyk, J. P., Schoenecker, J. G., and Flick, M. J. (2019). The multifaceted role of fibrinogen in tissue injury and inflammation. Blood 133, 511, 520. doi:10.1182/blood-2018-07-818211
Ma, Q., Rubenis, K., Eysteinn, O., Onsson, S., Hildebrand, T., Standal, T., et al. (2023). Eggshell-derived amorphous calcium phosphate: synthesis, characterization and bio-functions as bone graft materials in novel 3D osteoblastic spheroids model. Smart Mater Med. 4, 522–537. doi:10.1016/j.smaim.2023.04.001
Mahendran, K., Kottuppallil, G., and Sekar, V. (2019). Comparative evaluation of radiopacity and cytotoxicity of platelet-rich fibrin, platelet-rich fibrin + 50wt% nano-hydroxyapatite, platelet-rich fibrin + 50wt% dentin chips: an in vitro study. J. Conserv. Dent. 22, 28–33. doi:10.4103/JCD.JCD_281_18
Maia, P. W., Teixeira, M. L., de Macedo, L. G. S., Aloise, A. C., Passos, C. A., Aragoneses, J. M., et al. (2019). Use of platelet-rich fibrin associated with xenograft in critical bone defects: histomorphometric study in rabbits. Symmetry (Basel) 11, 1293. doi:10.3390/sym11101293
Malikmammadov, E., Tanir, T. E., Kiziltay, A., Hasirci, V., and Hasirci, N. (2018). PCL and PCL-based materials in biomedical applications. J. Biomater. Sci. Polym. Ed. 29, 863–893. doi:10.1080/09205063.2017.1394711
Mamo, H. B., Adamiak, M., and Kunwar, A. (2023). 3D printed biomedical devices and their applications: a review on state-of-the-art technologies, existing challenges, and future perspectives. J. Mech. Behav. Biomed. Mat. 143, 105930. doi:10.1016/j.jmbbm.2023.105930
Massuda, C. K. M., Pereira, J. F. M., Armond, M. N. M., Violante de Souza, R., Rabelo, Í. de A., Carvalho, M. R., et al. (2023). Horizontal alveolar ridge augmentation in the mandibular posterior region using biphasic calcium phosphate and leukocyte- and platelet rich fibrin (L-PRF): a case report. Braz. J. Implant. Health Sci. 5, 78–95. doi:10.36557/2674-8169.2023v5n4p78-95
Mazzocca, A. D., McCarthy, M. B. R., Chowaniec, D. M., Cote, M. P., Romeo, A. A., Bradley, J. P., et al. (2012). Platelet-rich plasma differs according to preparation method and human variability. J. Bone Jt. Surg. 94, 308–316. doi:10.2106/JBJS.K.00430
Mbarak, W., Jouliq, A., Hassaneen, A. M., Abdullah, A. E.-M., and El-Ghafar, A. (2023). Evaluation of effect of beta-tricalcium phosphate with platelets rich fibrin on alveolar ridge post extraction. J. Pharm. Negat. Results 14, 209–215. doi:10.47750/pnr.2023.14.S01.23
Meyer, M. (2019). Processing of collagen based biomaterials and the resulting materials properties. Biomed. Eng. Online 18, 24. doi:10.1186/s12938-019-0647-0
Michels, R., Magrin, G. L., Cruz, A. C. C., Magini, R. S., and Benfatti, C. A. M. (2023). Functionalization of a volume-stable collagen matrix using liquid platelet-rich fibrin: a case report presenting a new approach for root coverage. Case Rep. Dent. 2023, 1–8. doi:10.1155/2023/3929269
Miron, R. J., Chai, J., Fujioka-Kobayashi, M., Sculean, A., and Zhang, Y. (2020). Evaluation of 24 protocols for the production of platelet-rich fibrin. BMC Oral Health 20, 310. doi:10.1186/s12903-020-01299-w
Miron, R. J., Fujioka-Kobayashi, M., Hernandez, M., Kandalam, U., Zhang, Y., Ghanaati, S., et al. (2017). Injectable platelet rich fibrin (i-PRF): opportunities in regenerative dentistry? Clin. Oral Investig. 21, 2619–2627. doi:10.1007/s00784-017-2063-9
Mohamed Abdel-Aziz, L., Abdallah, S. A., Mohammed bakr, N., Bahaa, S. M., Zainalabdeen, E. H., Alsharif, M., et al. (2023). Effectiveness of a polycaprolactone scaffold combined with platelet-rich fibrin as guided tissue regeneration materials for preserving an implant-supported overdenture. Saudi Dent. J. doi:10.1016/j.sdentj.2023.08.012
Molenda, M., and Kolmas, J. (2011). The role of zinc in bone tissue health and regeneration-a review. Biol. Trace Elem. Res. 201, 5640–5651. doi:10.1007/s12011-023-03631-1
Mu, Z., Chen, K., Yuan, S., Li, Y., Huang, Y., Wang, C., et al. (2020). Gelatin nanoparticle-injectable platelet-rich fibrin double network hydrogels with local adaptability and bioactivity for enhanced osteogenesis. Adv. Healthc. Mater 9, 1–16. doi:10.1002/adhm.201901469
Nacopoulos, C., Dontas, I., Lelovas, P., Galanos, A., Vesalas, A. M., Raptou, P., et al. (2014). Enhancement of bone regeneration with the combination of platelet-rich fibrin and synthetic graft. J. Craniofac Surg. 25, 2164–2168. doi:10.1097/SCS.0000000000001172
Naik, C., Srinath, N., Ranganath, M. K., Umashankar, D. N., and Gupta, H. (2020). Evaluation of polycaprolactone scaffold for guided bone regeneration in maxillary and mandibular defects: a clinical study. Natl. J. Maxillofac. Surg. 11, 207–212. doi:10.4103/njms.NJMS_35_20
Nie, J., Zhang, S., Wu, P., Liu, Y., and Su, Y. (2020). Electrospinning with lyophilized platelet-rich fibrin has the potential to enhance the proliferation and osteogenesis of mc3t3-E1 cells. Front. Bioeng. Biotechnol. 8, 595579. doi:10.3389/fbioe.2020.595579
Nishimoto, S., Fujita, K., Sotsuka, Y., Kinoshita, M., Fujiwara, T., Kawai, K., et al. (2015). Growth factor measurement and histological analysis in platelet rich fibrin: a pilot study. J. Maxillofac. Oral Surg. 14, 907–913. doi:10.1007/s12663-015-0768-3
Nizam, N., Eren, G., Akcalı, A., and Donos, N. (2018). Maxillary sinus augmentation with leukocyte and platelet-rich fibrin and deproteinized bovine bone mineral: a split-mouth histological and histomorphometric study. Clin. Oral Implants Res. 29, 67–75. doi:10.1111/clr.13044
Noohi, P., Abdekhodaie, M. J., Saadatmand, M., Nekoofar, M. H., H Dummer, P. M., and Mohammad Abdekhodaie, C. J. (2023). The development of a dental light curable PRFe-loaded hydrogel as a potential scaffold for pulp-dentine complex regeneration: an in vitro study. Int. Endod. J. 56, 447–464. doi:10.1111/iej.13882
Nurkesh, A., Jaguparov, A., Jimi, S., and Saparov, A. (2020). Recent advances in the controlled release of growth factors and cytokines for improving cutaneous wound healing. Front. Cell Dev. Biol. 8, 638. doi:10.3389/fcell.2020.00638
Pandikanda, R., Singh, R., Patil, V., Sharma, M., and Shankar, K. (2019). Flapless closure of oro-antral communication with PRF membrane and composite of PRF and collagen – a technical note. J. Stomatol. Oral Maxillofac. Surg. 120, 471–473. doi:10.1016/j.jormas.2018.12.012
Park, J. S., Pabst, A. M., Ackermann, M., Moergel, M., Jung, J., and Kasaj, A. (2018). Biofunctionalization of porcine-derived collagen matrix using enamel matrix derivative and platelet-rich fibrin: influence on mature endothelial cell characteristics in vitro. Clin. Oral Investig. 22, 909–917. doi:10.1007/s00784-017-2170-7
Park, J. Y., Hong, K. J., Ko, K. A., Cha, J. K., Gruber, R., and Lee, J. S. (2023). Platelet-rich fibrin combined with a particulate bone substitute versus guided bone regeneration in the damaged extraction socket: an in vivo study. J. Clin. Periodontol. 50, 358–367. doi:10.1111/jcpe.13742
Pascawinata, A., Revilla, G., Sahputra, R. E., and Arief, S. (2023). Alveolar bone preservation using a combination of nanocrystalline hydroxyapatite and injectable platelet-rich fibrin: a study in rats. Issues Mol. Biol. 45, 5967–5980. doi:10.3390/cimb45070377
Patra, L., Raj, C., Katti, N., Mohanty, D., Pradhan, S., Tabassum, S., et al. (2022). Comparative evaluation of effect of injectable platelet-rich fibrin with collagen membrane compared with collagen membrane alone for gingival recession coverage. World J. Exp. Med. 12, 68–91. doi:10.5493/wjem.v12.i4.68
Pauksch, L., Hartmann, S., Rohnke, M., Szalay, G., Alt, V., Schnettler, R., et al. (2014). Biocompatibility of silver nanoparticles and silver ions in primary human mesenchymal stem cells and osteoblasts. Acta Biomater. 10, 439–449. doi:10.1016/J.ACTBIO.2013.09.037
Pavlovic, V., Ciric, M., Jovanovic, V., Trandafilovic, M., and Stojanovic, P. (2021). Platelet-rich fibrin: basics of biological actions and protocol modifications. Open Med. 16, 446–454. doi:10.1515/med-2021-0259
Peck, M. T., Hiss, D., Stephen, L., Satti, A., and Majeed, A. (2015). Platelet- Rich Fibrin (PRF) - the effect of storage time on platelet concentration. South Afr. Dent. J. 70, 448–451.
Peker, E., Karaca, I., and Yildirim, B. (2016). Experimental evaluation of the effectiveness of demineralized bone matrix and collagenated heterologous bone grafts used alone or in combination with platelet-rich fibrin on bone healing in sinus floor augmentation. Int. J. Oral Maxillofac. Implants 31, e24–e31. doi:10.11607/jomi.4414
Pepelassi, E., and Deligianni, M. (2022). The adjunctive use of leucocyte- and platelet-rich fibrin inPeriodontal endosseous and furcation defects: a systematic review and meta-analysis. Materials 15, 1–27. doi:10.3390/ma15062088
Pichotano, E. C., de Molon, R. S., de Souza, R. V., Austin, R. S., Marcantonio, E., and Zandim-Barcelos, D. L. (2019). Evaluation of L-PRF combined with deproteinized bovine bone mineral for early implant placement after maxillary sinus augmentation: a randomized clinical trial. Clin. Implant Dent. Relat. Res. 21, 253–262. doi:10.1111/cid.12713
Pichotano, E. C., Scaf De Molon, R., Guilherme Freitas De Paula, L., Violante De Souza, R., Marcantonio, E., and Leal Zandim-Barcelos, D. (2018). Early placement of dental implants in maxillary sinus grafted with leukocyte and platelet-rich fibrin and deproteinized bovine bone mineral. J. Oral Implant. 3, 199–206. doi:10.1563/aaid-joi-D-17-00220
Ponte, J. S., Pérez-Guerrero, J. A., Aragão, F. A. A., Menezes, Y. A. T., Melo, M. M., and Castro-Silva, I. I. (2021). Histomorphometric evaluation of human extraction sockets treated with autologous fibrin, sticky bone or biphasic calcium phosphate. Acta Odontol. Latinoam. 34, 271–281. doi:10.54589/aol.34/3/271
Potres, Z., Deshpande, S., Klöeppel, H., Voss, K., and Klineberg, I. (2016). Assisted wound healing and vertical bone regeneration with simultaneous implant placement: a histologic pilot study. Int. J. Oral Maxillofac. Implants 31, 45–54. doi:10.11607/jomi.3951
Pradeep, K., Kudva, A., Narayanamoorthy, V., Cariappa, K., and Saraswathi, M. (2016). Platelet-rich fibrin combined with synthetic nanocrystalline hydroxy apatite granules in the management of radicular cyst. Niger. J. Clin. Pract. 19, 688–691. doi:10.4103/1119-3077.188711
Pushpalatha, C., Suresh, J., Gayathri, V. S., Sowmya, S. V., Augustine, D., Alamoudi, A., et al. (2022). Zinc oxide nanoparticles: a review on its applications in dentistry. Front. Bioeng. Biotechnol. 10, 917990. doi:10.3389/fbioe.2022.917990
Qin, X., Wu, Y., Liu, S., Yang, L., Yuan, H., Cai, S., et al. (2022). Surface modification of polycaprolactone scaffold with improved biocompatibility and controlled growth factor release for enhanced stem cell differentiation. Front. Bioeng. Biotechnol. 9, 802311. doi:10.3389/fbioe.2021.802311
Ramamurthy, P., Miller, K., Hamlet, S., Belinda Tony, J., Parthasarathy, H., Tadepalli, A., et al. (2022). CBCT evaluation of sticky bone in horizontal ridge augmentation with and without collagen membrane-A randomized parallel arm clinical CBCT evaluation of sticky bone in horizontal ridge augmentation with and without collagen membrane-A randomized parallel arm clinical trial. J. Funct. Biomater. 13, 1–10. doi:10.3390/jfb13040194
Ramy Salah, H., Taha, E. M., and Mohammed, M. U. (2021). Narrow Ridge expansion for implant placement using ultrasonic bone surgery and platelet rich fibrin with and without silk fibroin. Al-Azhar J. Dent. Sci. 24, 55–62. doi:10.21608/ajdsm.2020.26649.1049
Rastegar, A., Mahmoodi, M., Mirjalili, M., and Nasirizadeh, N. (2021). Platelet-rich fibrin-loaded PCL/chitosan core-shell fibers scaffold for enhanced osteogenic differentiation of mesenchymal stem cells. Carbohydr. Polym. 269, 118351. doi:10.1016/j.carbpol.2021.118351
Salih, S. I., Al-Falahi, N. H., Saliem, A. H., and Abedsalih, A. N. (2018). Effectiveness of platelet-rich fibrin matrix treated with silver nanoparticles in fracture healing in rabbit model. Vet. World 11, 944–952. doi:10.14202/vetworld.2018.944-952
Sampaio, N., Oliveira, M. N., Carvalho, O., Manzanares-Céspedes, M.-C., Silva, F., Henriques, B., et al. (2023). Porous zirconia blocks embedded with platelet rich fibrin for enhanced bone healing: mechanical and morphological assessment. Biomed. Mater. and Devices 1, 1–11. doi:10.1007/s44174-023-00076-x
Santamaria, M. P., Rossato, A., Ferreira Ferraz, L. F., Bonafé, A. C., Miguel, M. M. V., and Nunes, M. P. (2023). Collagen matrix biofunctionalized with injectable platelet-rich fibrin for the treatment of single gingival recession: a case report. Clin. Adv. Periodontics 13, 110–114. doi:10.1002/cap.10207
saravanan, D., Rethunam, S., Muthu, K., and Thangapandian, A. (2019). The combined effect of bioactive glass and platelet-rich fibrin in treating human periodontal intrabony defects – a clinicoradiographic study. Contemp. Clin. Dent. 10, 110–116. doi:10.4103/ccd.ccd_507_18
Sebastian, B., Saskia Vanessa, S., Andreas, P., Kämmerer, P. W., Keyvan, S., and Bilal, A.-N. (2022). Biofunctionalization of xenogeneic collagen membranes with autologous platelet concentrate—influence on rehydration protocol and angiogenesis. Biomedicines 10, 1–21. doi:10.3390/biomedicines10030706
Sen, C. K. (2021). Human wound and its burden: updated 2020 compendium of estimates. Adv. Wound Care (New Rochelle) 10, 281–292. doi:10.1089/wound.2021.0026
Serpooshan, V., Mahmoudi, M., Zhao, M., Wei, K., Sivanesan, S., Motamedchaboki, K., et al. (2015). Protein corona influences cell-biomaterial interactions in nanostructured tissue engineering scaffolds. Adv. Funct. Mater 25, 4379–4389. doi:10.1002/adfm.201500875
Shabat, M. A., and Yousif, B. S. (2021). Effect of the local application of bupivacaine in early pain control following impacted mandibular third molar surgery: a randomized controlled study. Dent. Med. Probl. 58, 483–488. doi:10.17219/dmp/133664
Sheikh, Z., Qureshi, J., Alshahrani, A. M., Nassar, H., Ikeda, Y., Glogauer, M., et al. (2017). Collagen based barrier membranes for periodontal guided bone regeneration applications. Odontology 105, 1–12. doi:10.1007/s10266-016-0267-0
Shen, H., and Hu, X. (2021). Growth factor loading on aliphatic polyester scaffolds. RSC Adv. 11, 6735–6747. doi:10.1039/d0ra10232f
Shevchenko, S., and Rublenko, M. (2022). The effect of osteosubstitution by platelet-rich autofibrin and hydroxyapatite ceramic with ß-tricalcium phosphate on biochemical parameters of blood in rabbits. Turk J. Vet. Anim. Sci. 46, 599–608. doi:10.55730/1300-0128.4231
Shivashankar, V., Johns, D. A., Vidyanath, S., and Sam, G. (2013). Combination of platelet rich fibrin, hydroxyapatite and PRF membrane in the management of large inflammatory periapical lesion. J. Conservative Dent. 16, 261–264. doi:10.4103/0972-0707.111329
Shoulders, M. D., and Raines, R. T. (2009). Collagen Structure And Stability. Annu. Rev. Biochem. 78, 929–958. doi:10.1146/annurev.biochem.77.032207.120833
Simone, C., Castro, A. B., Temmerman, A., Van Dessel, J., Pinto, N., Jacobs, R., et al. (2018). Leucocyte- and platelet-rich fibrin block for bone augmentation procedure: a proof-of-concept study. J. Clin. Periodontol. 45, 624–634. doi:10.1111/jcpe.12877
Şimşek, S., Özeç, İ., Kürkçü, M., and Benlidayı, E. (2016). Histomorphometric evaluation of bone formation in peri-implant defects treated with different regeneration techniques: an experimental study in a rabbit model. J. Oral Maxillofac. Surg. 74, 1757–1764. doi:10.1016/j.joms.2016.05.026
Song, Y., Lin, K., He, S., Wang, C., Zhang, S., Li, D., et al. (2018). Nano-biphasic calcium phosphate/polyvinyl alcohol composites with enhanced bioactivity for bone repair via low-temperature three-dimensional printing and loading with platelet-rich fibrin. Int. J. Nanomedicine 13, 505–523. doi:10.2147/IJN.S152105
Stamenkovic, I. (2003). Extracellular matrix remodelling: the role of matrix metalloproteinases. J. Pathology 200, 448–464. doi:10.1002/path.1400
Su, Y., Cockerill, I., Wang, Y., Qin, Y.-X., Chang, L., Zheng, Y., et al. (2019). Zinc-based biomaterials for regeneration and therapy. Trends Biotechnol. 37, 428–441. doi:10.1016/j.tibtech.2018.10.009
Sui, X., Zhang, H., Yao, J., Yang, L., Zhang, X., Li, L., et al. (2023). 3D printing of ‘green’ thermo-sensitive chitosan-hydroxyapatite bone scaffold based on lyophilized platelet-rich fibrin. Biomed. Mater. 18, 1–12. doi:10.1088/1748-605X/acbad5
Suzuki, S., Morimoto, N., and Ikada, Y. (2013). Gelatin gel as a carrier of platelet-derived growth factors. J. Biomater. Appl. 28, 595–606. doi:10.1177/0885328212468183
Tabrizi, R., Arabion, H., and Karagah, T. (2018). Does platelet-rich fibrin increase the stability of implants in the posterior of the maxilla? A split-mouth randomized clinical trial. Int. J. Oral Maxillofac. Surg. 47, 672–675. doi:10.1016/j.ijom.2017.07.025
Tajvar, S., Hadjizadeh, A., and Samandari, S. S. (2023). Scaffold degradation in bone tissue engineering: an overview. Int. Biodeterior. Biodegrad. 180, 105599. doi:10.1016/j.ibiod.2023.105599
Tarif, C. M., Mandal, S., Chakraborty, B., Sarkar, K., Mukherjee, P., Roy, M., et al. (2023). In vitro and in vivo assessment of decellularized platelet-rich fibrin-loaded strontium doped porous magnesium phosphate scaffolds in bone regeneration. J. Mech. Behav. Biomed. Mater 138, 105587. doi:10.1016/j.jmbbm.2022.105587
Tavoni, M., Dapporto, M., Tampieri, A., and Sprio, S. (2021). Bioactive calcium phosphate-based composites for bone regeneration. J. Compos. Sci. 5, 227. doi:10.3390/JCS5090227
Terzioğlu, P., Öğüt, H., and Kalemtaş, A. (2018). Natural calcium phosphates from fish bones and their potential biomedical applications. Mater Sci. Eng. C Mater Biol. Appl. 91, 899–911. doi:10.1016/j.msec.2018.06.010
Theodosaki, A.-M., Filippou, S., Kazantzidis, G., and Doufexi, A.-E. (2022). Effectiveness of platelet rich fibrin alone or in combination with bone grafts in the treatment of infrabony defects: systematic review and metanalysis. Health Sci. Rev. 5, 100066. doi:10.1016/j.hsr.2022.100066
Tiwari, U., Chandra, R., Tripathi, S., Jain, J., Jaiswal, S., and Tiwari, R. (2020). Comparative analysis of platelet-rich fibrin, platelet-rich fibrin with hydroxyapatite and platelet-rich fibrin with alendronate in bone regeneration: a cone-beam computed tomography analysis. J. Conserv. Dent. 23, 348. doi:10.4103/JCD.JCD_228_20
Ucuzian, A. A., Gassman, A. A., East, A. T., and Greisler, H. P. (2010). Molecular mediators of angiogenesis. J. Burn Care Res. 31, 158–175. doi:10.1097/BCR.0b013e3181c7ed82
Udeabor, S. E., Herrera-Vizcaíno, C., Sader, R., Kirkpatrick, C. J., Al-Maawi, S., and Ghanaati, S. (2020). Characterization of the cellular reaction to a collagen-based matrix: an in vivo histological and histomorphometrical analysis. Materials 13, 2730. doi:10.3390/ma13122730
van Orten, A., Goetz, W., and Bilhan, H. (2022). Tooth-derived granules in combination with platelet-rich fibrin (“Sticky tooth”) in socket preservation: a histological evaluation. Dent. J. (Basel) 10, 29–15. doi:10.3390/dj10020029
Vepari, C., and Kaplan, D. L. (2007). Silk as a biomaterial. Prog. Polym. Sci. Oxf. 32, 991–1007. doi:10.1016/j.progpolymsci.2007.05.013
Verma, H., Dilip Kumar, R., T, P. N., and S, S. E. (2014). Qualitative and quantitative analysis of bone formation in the peri-implant defects grafted with polycaprolactone (pcl) alloplast enriched with platelet rich fibrin (PRF): a clinical and radiological study. Int. J. Dent. Med. Res. 1.
Vibhor, H., Choudhary, A., Mishra, R., Kabbur, C. T., Ashima, T., and Pathak, P. K. (2021). Clinical and radiographic analysis of novabone putty with platelet-rich fibrin in the treatment of periodontal intrabony defects: a randomized control trial. Contemp. Clin. Dent. 12, 150–156. doi:10.4103/ccd.ccd_101_20
Wang, Z., Yang, M., Mao, L., Wang, X., Wang, S., Cui, G., et al. (2021). Efficacy and safety of autologous platelet-rich fibrin for the treatment of infertility with intrauterine adhesions. J. Obstetrics Gynaecol. Res. 47, 3883–3894. doi:10.1111/jog.14964
Wilson, J., and Low, S. 6 (1992). Bioactive ceramics for periodontal treatment: comparative studies in the patus monkey. J. Appl. Biomaterials 3, 123–129. doi:10.1002/jab.770030208
Yan, X., Bin He, ab, Lihong Liu, ab, Guangbo Qu, ab, Jianbo Shi, ab, Ligang, Hu ab, et al. (2018). Antibacterial mechanism of silver nanoparticles in Pseudomonas aeruginosa: proteomics approach. Metallomics 10, 557–564. doi:10.1039/c7mt00328e
Ye, L., Mubarak, M. A., Linhu, G., Fang, Y., Guo, X., Ge, Q., et al. (2023). Network meta-analysis of platelet-rich fibrin in periodontal intrabony defects. J. Oral Pathol. Med. 52, 206–215. doi:10.1111/jop.13409
Yi, K., Li, Q., Lian, X., Wang, Y., and Tang, Z. (2022). Utilizing 3D bioprinted platelet-rich fibrin-based materials to promote the regeneration of oral soft tissue. Regenarative Biomater. 9, rbac021–13. doi:10.1093/rb/rbac021
Yilmaz, D. I., Dogan, N. I., Ozkan, I. I. I. A., Sencimen, M. I., Eren Ora, lV. B., and Mutlu, I. V. (2014). Effect of platelet rich fibrin and beta tricalcium phosphate on bone healing. A histological study in pigs. Acta Cir. Bras. 29, 59–65. doi:10.1590/s0102-86502014000100009
Yuan, S., Li, Q., Chen, K., Mu, Z., Chen, T., Wang, H., et al. (2021). Ridge preservation applying a novel hydrogel for early angiogenesis and osteogenesis evaluation: an experimental study in canine. J. Biol. Eng. 15, 19. doi:10.1186/s13036-021-00271-8
Zalama, E., Karrouf, G., Rizk, A., Salama, B., and Samy, A. (2022). Does zinc oxide nanoparticles potentiate the regenerative effect of platelet-rich fibrin in healing of critical bone defect in rabbits? BMC Vet. Res. 18, 130. doi:10.1186/s12917-022-03231-6
Zalama, E., Samy, A., Rizk, A., Salama, B., and Karrouf, G. (2021). Comparative healing efficacy of platelet rich fibrin and zinc oxide nanoparticles on critical-sized ulnar bone defects in rabbits. J. Veterinary Healthc. 2, 29–37. doi:10.14302/issn.2575-1212.jvhc-21-3873
Zhang, H., Lin, C. Y., and Hollister, S. J. (2009). The interaction between bone marrow stromal cells and RGD-modified three-dimensional porous polycaprolactone scaffolds. Biomaterials 30, 4063–4069. doi:10.1016/j.biomaterials.2009.04.015
Zhang, J., Zhang, H., Chen, Y., Fu, J., Lei, Y., Sun, J., et al. (2019b). Platelet-derived growth factor D promotes the angiogenic capacity of endothelial progenitor cells. Mol. Med. Rep. 19, 125–132. doi:10.3892/mmr.2018.9692
Zhang, L., Dong, Y., Liu, Y., Liu, X., Wang, Z., Wan, J., et al. (2023b). Multifunctional hydrogel/platelet-rich fibrin/nanofibers scaffolds with cell barrier and osteogenesis for guided tissue regeneration/guided bone regeneration applications. Int. J. Biol. Macromol. 253, 126960. doi:10.1016/J.IJBIOMAC.2023.126960
Zhang, L., Dong, Y., Xue, Y., Shi, J., Zhang, X., Liu, Y., et al. (2019a). Multifunctional triple-layered composite scaffolds combining platelet-rich fibrin promote bone regeneration. ACS Biomater. Sci. Eng. 5, 6691–6702. doi:10.1021/acsbiomaterials.9b01022
Zhang, R., Lee, P., Lui, V. C. H., Chen, Y., Liu, X., Lok, C. N., et al. (2015). Silver nanoparticles promote osteogenesis of mesenchymal stem cells and improve bone fracture healing in osteogenesis mechanism mouse model. Nanomedicine 11, 1949–1959. doi:10.1016/J.NANO.2015.07.016
Zhang, Y., Cao, C., Jun, Li, Chi, L., Ketong, Mi, and Zhang, X. (2023a). Platelet-rich fibrin combined with new bone graft material for mandibular defect repair: a in vivo study on rabbits. Dent. Mater. J. 42, 241–247. doi:10.4012/dmj.2022-076
Zhang, Y., Wang, P., Wang, Y., Li, J., Qiao, D., Chen, R., et al. (2021). Gold nanoparticles promote the bone regeneration of periodontal ligament stem cell sheets through activation of autophagy. Int. J. Nanomedicine 16, 61–73. doi:10.2147/IJN.S282246
Keywords: platelet-rich fibrin, maxillofacial surgery, tissue regeneration, growth factors, bioengineering, 3D printing
Citation: Ieviņa L and Dubņika A (2024) Navigating the combinations of platelet-rich fibrin with biomaterials used in maxillofacial surgery. Front. Bioeng. Biotechnol. 12:1465019. doi: 10.3389/fbioe.2024.1465019
Received: 15 July 2024; Accepted: 24 September 2024;
Published: 07 October 2024.
Edited by:
Zuhair S. Natto, King Abdulaziz University, Saudi ArabiaReviewed by:
Phaedra Silva-Bermudez, National Institute of Rehabilitation Luis Guillermo Ibarra Ibarra, MexicoIoannis Vasilikos, University of Freiburg Medical Center, Germany
Arwa Badahdah, King Abdulaziz University, Saudi Arabia
Copyright © 2024 Ieviņa and Dubņika. This is an open-access article distributed under the terms of the Creative Commons Attribution License (CC BY). The use, distribution or reproduction in other forums is permitted, provided the original author(s) and the copyright owner(s) are credited and that the original publication in this journal is cited, in accordance with accepted academic practice. No use, distribution or reproduction is permitted which does not comply with these terms.
*Correspondence: Arita Dubņika, YXJpdGEuZHVibmlrYUBydHUubHY=