- 1Shaanxi Provincial Land Engineering Construction Group, Key Laboratory of Degraded and Unused Land Consolidation Engineering, Ministry of Natural Resources, Xi’an, China
- 2Shaanxi Engineering Research Center of Land Consolidation, Shaanxi Provincial Land Consolidation Engineering Technology Research Center, Xi’an, China
- 3Kweichow Moutai Winery (Group) Health Wine Co., LTD., Renhuai, China
- 4School of Environmental Science and Engineering, Suzhou University of Science and Technology, Suzhou, China
Reducing total nitrogen (TN) presents a significant challenge for numerous wastewater treatment facilities. In order to address this issue, the current study employed a biological aerated filter for the treatment of wastewater containing low nitrogen concentrations. Both lab-scale and pilot-scale biofilters were constructed to investigate the denitrification performance and maximum denitrification load. The findings indicated that the anaerobic denitrification process of established biofilm adhered to pseudo-first-order kinetics. The results of batch testing and continuous-flow experiments confirmed that the minimum hydraulic retention time (HRT) required for mature biofilm was determined to be 0.5 h. The optimal operating parameters were found to be as follows: influent NO3−-N concentration of 25 mg/L, HRT of 0.5 h, resulting in effluent TN levels below 1 mg/L. Under these conditions, the denitrifying load for the lab-scale I-BAF system was calculated to be 1.26 kg (TN)/(m3·d). Furthermore, it was observed that the maximum denitrifying load could reach 2.2 kg (TN)/(m3·d) when the influent NO3−-N concentration was increased to 50 mg/L while maintaining an HRT of 0.5 h. For the mature biofilter, the appropriate HRT ranged from 2 h to 0.5 h. Microbial diversity analysis revealed that the genus Enterobacter was dominant in all denitrification systems, followed by Comamonas and Rhodococcus. The operational parameters described in the paper could be recommended for a full-scale wastewater treatment facility.
1 Introduction
Currently, achieving low-pollutant effluent is a hot topic of study due to increasingly stringent discharge standards and water scarcity worldwide (Yaqoob et al., 2023). The primary challenge in this regard is to further reduce the concentration of total nitrogen (TN) (Omar et al., 2024). In China, the TN discharge concentration is limited to 15 mg/L (GB18918-2002) for wastewater treatment plants (WWTPs), while the European Union sets it at 10 mg/L. Furthermore, the World Health Organization (WHO) requires that nitrate concentration in drinking water should not exceed 10 mg/L (Su et al., 2020). TN in WWTP effluent is predominantly composed of nitrate, with the remaining nitrate originating from incomplete denitrification in mainstream wastewater treatment processes such as A2/O or oxidation ditches (Sheik et al., 2023). Currently, the TN concentration in secondary effluent from WWTPs in China ranges between 10 mg/L and 15 mg/L (Deng et al., 2021; Cui et al., 2023), and sometimes even reaches up to 25 mg/L (Liu et al., 2021). Such high nitrogen emissions can still cause eutrophication in many watersheds and pose a hazard to aquatic species. To address these issues, the Limited Denitrification Technology (LDT) has been proposed to further reduce the current TN concentration (Su et al., 2018).
The LDT method is primarily aimed at further reducing TN from low concentrations (15–25 mg/L) to ultra-low concentrations (<1 mg/L) in a stable manner. Applicable processes include short-term nitrification and denitrification, simultaneous nitrification-denitrification, aerobic denitrification, and anammox etc., (James and Vijayanandan, 2023). To achieve stable TN effluent standard, it may also be necessary to combine physical-chemical methods, such as sulfur autotrophic denitrification (Wang et al., 2023) and zero-valent iron denitrification processes (Zhou et al., 2024). These approaches can ensure ultra-low total nitrogen levels in the effluent while also promoting energy efficiency and reducing emissions. However, the key factor lies in whether the biological treatment stage can maintain a high TN removal efficiency. For example, if the biological treatment can reduce TN from 25 mg/L to 3 mg/L, then the sulfur autotrophic denitrification process can further reduce TN to around 1 mg/L.
Many studies assert that further reducing TN below 10 mg/L is an expensive process using mainstream technology (Chang and Wang, 2015), and some even suggest that achieving stable water quality with TN < 3 mg/L is impossible (Zhu et al., 2023). A large number of engineering applications indicate that traditional activated sludge processes can only ensure that the effluent TN remains stable at below 15 mg/L (Zhang et al., 2024). The biological filter process can achieve lower TN effluent concentration. However, commonly used media, such as volcanic rock and clay pellets, offer low biomass support, weak electron transfer capabilities, low microbial affinity, and are prone to clogging in practice (Yang et al., 2022). Additionally, the low effectiveness of inoculant strains also limits the ability of biological filters to further reduce TN effluent concentration (Simon et al., 2023). Therefore, the immobilized biological aerated filter (I-BAF) technology has been proposed to address these issues. In I-BAF, denitrifying microbes are immobilized on polyurethane foam (PUF) carriers, allowing the biofilm to develop a high-density biomass on the carriers and possess strong resistance to external stress (Gan et al., 2019). I-BAF can achieve ultra-low effluent nitrate concentrations under high loads and significantly reduce facility volume, demonstrating advantages in terms of economic efficiency and environmental friendliness. Several studies have aimed to elucidate the mechanisms and principles of I-BAF (Gan et al., 2020; Ye et al., 2005). However, there are few studies focused on ultra-low total nitrogen emissions and the maximum denitrifying load of biofilters, as observed in previous literature reviews.
In this study, both lab-scale and pilot-scale I-BAF systems were constructed to investigate the discharge of the lowest TN concentration using a mixed microbial consortium. Additionally, the microbial diversity characteristics of the biofilm under different operating conditions were investigated to elucidate the denitrifying performance of I-BAF. The operational parameters determined in this study could be applied to full-scale wastewater treatment facilities.
2 Materials and methods
2.1 Inoculation strain and assay methods
The inoculum strain used was a denitrifying microbial consortium (DMC), originally obtained from BIONETIX Co. (Canada) (Zhang et al., 2019). Synthetic wastewater was prepared based on the composition outlined in Table 1 (Gan et al., 2020). Prior to analysis, all samples were filtered through a 0.45 μm membrane. The TN concentration was defined as the sum of ammonia, nitrate, and nitrite (Cao et al., 2014).
The test methods employed were as follows: nitrate, nitrite, and ammonia levels were determined using a UV spectrophotometer (Hach, DR-5000) in accordance with Chinese Environmental Standards (HJ/T 346-2007, GB 7493-87, HJ 535-2009), respectively, COD was measured using the acidic potassium dichromate oxidation method (DRB-200, Hach Corporation, United States), SS was determined via the gravimetric method (GB 11901-89), pH values were monitored using a pH-201 m (Hanna Corporation, Italy), dissolved oxygen (DO) and temperature were monitored with a portable DO meter (JPB-607A). Degradation curves were fitted with linear kinetics and pseudo-1st-order kinetics to describe the mechanism of nitrogen removal (Deng and Wheatley, 2018). The fitting function of pseudo-1st-order model used
2.2 Nitrate nitrogen degradation curve for mature biofilm
Before the experiment, batch tests were conducted to investigate the dynamics of NO3−-N degradation using mature biofilm. The mature biofilm (see Supplementary Figure A1) was obtained from an I-BAF reactor that had been operational for 6 months. This biofilm exhibited efficient denitrification capability, which could determine the minimum HRT of the I-BAF system. The higher the denitrifying capability, the lower the HRT could be set. The mature biofilm, covered with denitrifying bacteria, was placed into three conical bottles (500 mL each) with a filling ratio of 95%. The composition used in this section is outlined in Table 1. The nitrate degradation curve was measured over time, as shown in Figure 1, indicating that nitrate degradation followed pseudo-1st-order kinetics with a half-life (T1/2) of 5 min. Nitrate nitrogen at a concentration of 25 mg/L was degraded to near 0 mg/L within 15–30 min, suggesting that the minimum HRT could be within this range when operating the continuous-flow I-BAF system.
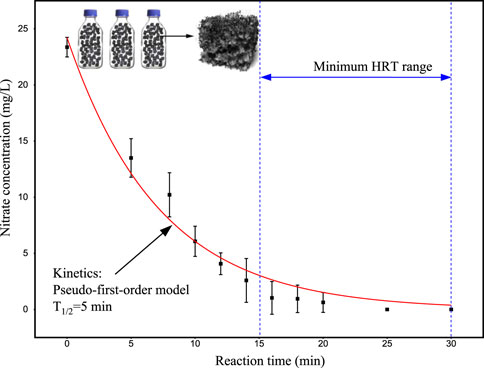
Figure 1. Nitrate removal kinetic of mature biofilm Experiment condition: 25°C, pH 7.5, initial NO3−-N = 25 mg/L, C/N/P = 100:20:1.
2.3 Lab-scale and pilot-scale I-BAF setup
As depicted in Supplementary Figure A2, lab-scale continuous-flow experiments were conducted in an organic glass cylindrical reactor (BAFL) with dimensions of 15 cm (inner diameter) by 75 cm (height), having a working volume of 13.2 L. The reactor was packed with polyurethane foam (PUF) carriers in the form of 3 cm × 3 cm × 3 cm cubes, possessing a specific surface area ranging from 80 to 120 m2/g and a wet density of 1 g/cm3, packed to a 95% filling ratio (Ye et al., 2005). Synthetic wastewater was continuously pumped from the bottom through the bioreactor using a peristaltic pump (BT100s, China). Nitrogen (N2) was introduced into the bottom of the reactor to regulate dissolved oxygen (DO) concentration and promote uniform water quality within the biofilm system. Four water sampling ports were positioned at 150 mm intervals along the height of the reactor, with the effluent port located at a height of 750 mm. Additionally, four biofilm-carrier sampling ports were situated on another side for microbial diversity analysis (Supplementary Figure A2).
As shown in Figures 6C, D, pilot-scale continuous-flow experiments were carried out in an organic glass cubic reactor (dimensions = 1 m × 1 m × 1 m) with the working volume of 500 L. PUFs carriers and commercial hollow ball (CHB) carriers packed the two reactors respectively at 95% filling ratio. The synthetic wastewater was continuously driven from the bottom into the bioreactors. Air was pumped into the bottom of the reactors to regulate DO concentration. Four water sampling ports (S1–S4) were set respectively every 120 mm height, and the effluent was discharged by tumbling bay. The bottom of the reactor was equipped with backwash system.
2.4 Experimental process and operating conditions
The composition and corresponding concentrations of the lab-scale experiment are listed in Table 1. A denitrifying microbial consortium (DMC) weighing 13.2 g was added to the BAFL and operated aerated with N2 for 48 h to allow the denitrifying bacteria to adapt to the environment (adaptation period). Following the 48-h adaptation period, the continuous-flow experiment proceeded to assess the denitrifying performance of the BAFL. The HRT was regulated to 6, 4, 2, 1, 0.5, and 0.25 h, as depicted in Supplementary Figure A3. Water samples were collected daily from the inlet and outlet to measure four indices (COD, nitrate, nitrite, ammonia). Furthermore, spatial denitrification performance (SDP) tests were conducted under HRTs of 6, 4, 2, 1, 0.5, and 0.25 h (Supplementary Figure A3). Subsequently, the limit denitrifying load (LDL) test was explored by increasing the influent nitrate concentration to 50 mg/L and 100 mg/L (Supplementary Figure A3). Water samples were collected daily from the inlet and outlet to measure COD, nitrate, nitrite, and ammonia concentrations.
For the pilot-scale experiment, 800 g of the inoculum strain was added into BAF1 and BAF2, respectively. The composition and corresponding concentrations are detailed in Table 1. The adaptation period and continuous-flow experiment were conducted as shown in Supplementary Figure A3, with air aeration. Water samples were collected daily from both the inlet and outlet. Additionally, four samples were taken at water sampling ports (S1–S4) every 2 days when the HRT was set to 1 h.
2.5 Microbial community analysis
Four biofilm samples were collected from biofilm carrier sampling ports (see Supplementary Figure A2) in BAFL during the stable operation phase (30th day, HRT = 0.5 h). Additionally, three biofilm samples were randomly collected from both BAF1 and BAF2 during the stable operation phase (64th day, HRT = 0.5 h). These samples, along with the inoculation strain, were collected and stored at 4°C for microbial community analysis (Liu et al., 2021).
3 Results and discussion
3.1 The start-up of biofilter
The spontaneous formation of the active layer in biological filters typically takes anywhere from a week to several months (Jing et al., 2012; Štembal et al., 2004; Bosander and Westlund, 2000). Generally, the duration of biofilter start-up is primarily determined by the inoculated microorganisms (Breda et al., 2019), the type of carrier (Smet et al., 1996), and the aeration patterns (Bacquet et al., 1991). Aeration patterns can be categorized into three forms: anaerobic, aerobic, and intermittent aeration. The aeration mode utilized in our lab-scale biofilter was an anaerobic pattern using nitrogen gas. As depicted in Figure 2A, nitrate decreased to zero within the initial 20 h (phase I), while nitrite reached its maximum concentration (72.4 mg/L) at this point. Such a phenomenon is commonly observed during the initial operation process of biological denitrifying filters (Gan et al., 2020). Initially, most of the nitrate is transformed into nitrite, which then gradually degrades into N2O and eventually into nitrogen gas (Das et al., 2021). In Figure 2A, the NO3−-N degradation rate constant (k1 = - 8) can be considered as the NO2−-N generation rate constant during phase I. This constant was faster than the nitrite degradation constant (k2 = −7.43), resulting in its accumulation during phase I (Zhang et al., 2020; Yue et al., 2009).
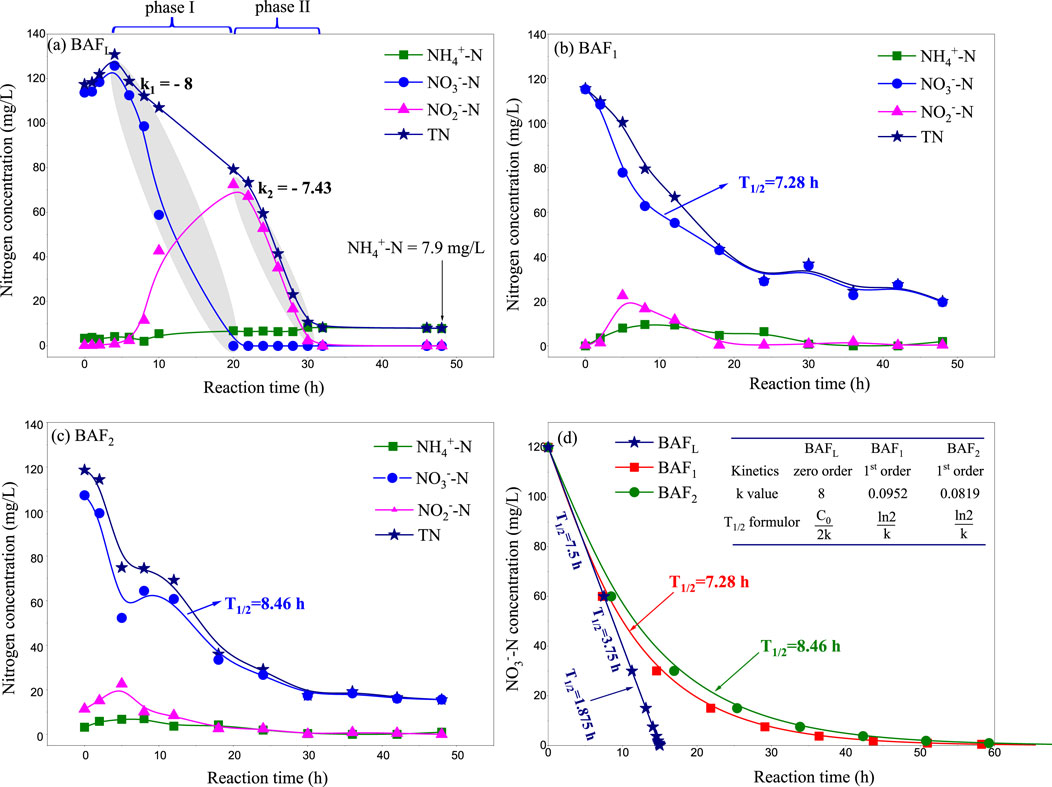
Figure 2. Composition change curve in adaptation stage of immobilized microbial filter (A) Lab-scale I-BAF, (B) Pilot-scale I-BAF loaded with PUFs carriers, (C) Pilot-scale I-BAF loaded with CHB carriers, (D) Simulated kinetic curve of nitrate degradation.
It is noteworthy that both the degradation of nitrate and nitrite conformed to linear dynamics (0-order kinetics) during the adaptation phase, a finding which is also consistent with Yao’s research (Yao et al., 2022). They suggested that TN removal follows zero-order kinetics in the presence of NH4+ during the initial nitrogen removal stage. Occasionally, ammonia accumulated gradually to 7.9 mg/L throughout the entire adaptation phase, as shown in Figure 2A.
The aeration mode of the pilot-scale biofilter was an aerobic pattern using air (Zupanèiè et al., 2008). As depicted in Figures 2B, C, nitrate degradation followed a pseudo-1st-order kinetics model, with half-life periods (T1/2) of BAF1 and BAF2 being 7.28 h and 8.46 h, respectively. This indicates that their denitrifying rates were significantly lower than that of BAFL. This suggests that the anaerobic aeration pattern is more conducive to the growth of denitrifying bacteria (Hong et al., 2019).
In Figure 2D, the half-life period (T1/2) of BAFL exhibited a linear relationship with the initial nitrate nitrogen concentration. At a concentration of 25 mg/L, the T1/2 of BAFL was 1.56 h, significantly larger than that of the mature biofilm (T1/2 = 5 min, as mentioned in Section 2.2). This indicates that the denitrifying capacity of the mature biofilm was 18.7 times higher than that of the initial biofilm.
3.2 Denitrification performance of lab-sacle I-BAF
The denitrification performance of a biofilter is mainly reflected in the effluent quality, organic load, and denitrifying load (Wu et al., 2021). In Figures 3A, B, concerning COD and TN, the continuous-flow test indicated that the lab-scale biofilter was not initially stable (at HRT = 6 h), suggesting that the denitrifying bacteria were still in the start-up stage (Jing et al., 2012). During this phase, both TN and COD levels in the effluent remained high, with COD exceeding the Chinese Discharge Standard of Pollutants for Municipal Wastewater Treatment Plants (GB 18918-2002, Level A < 50 mg/L).
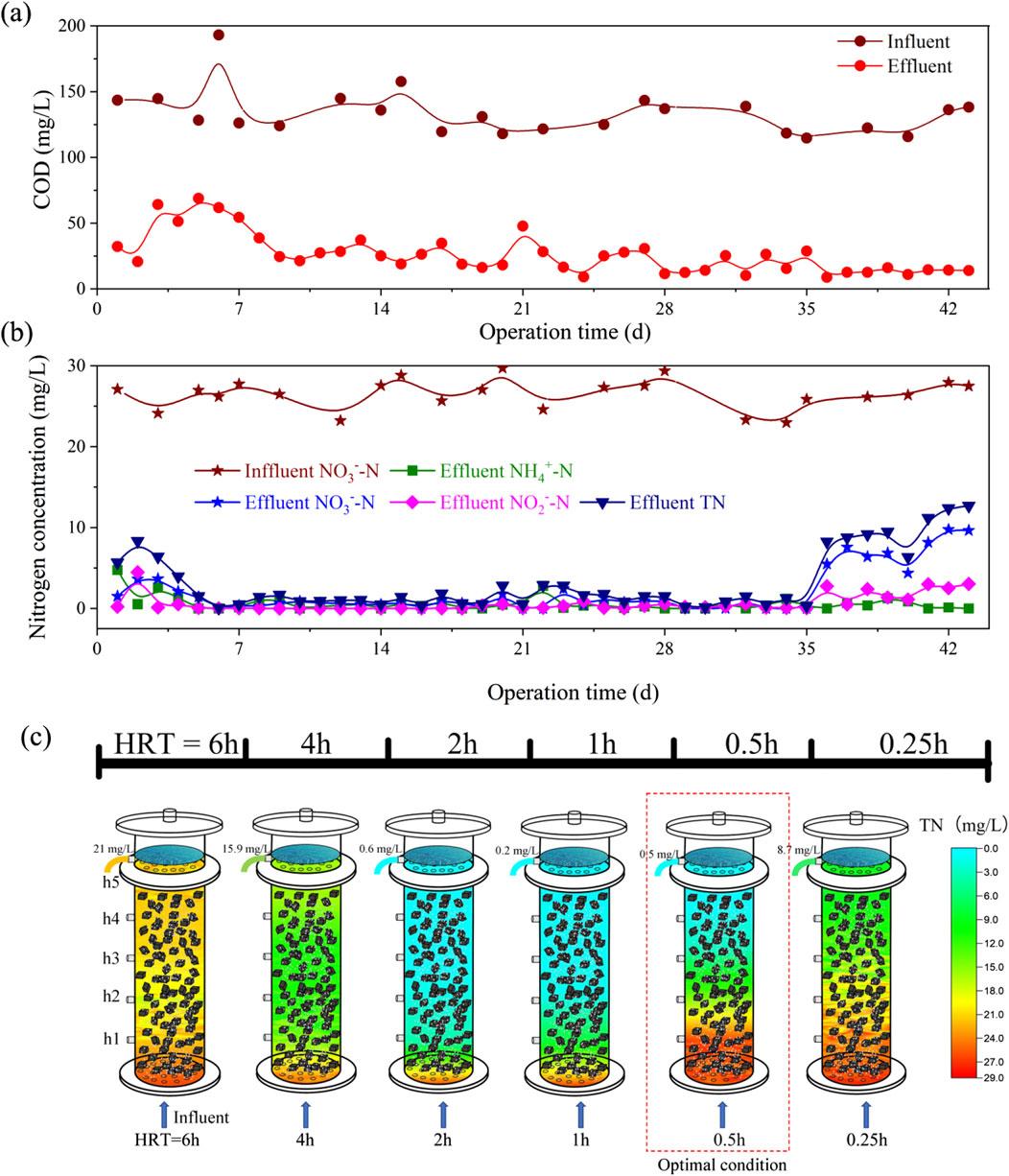
Figure 3. Nitrogen removal performance and TN spatial distribution of lab-scale I-BAF (A) COD removal performance, (B) Nitrogen removal performance, (C) Spatial distribution of TN with different HRT.
Figure 3B shows that TN levels ranged from 0.2 to 2.8 mg/L between the 7th and 35th day (corresponding to an HRT of 4 to 0.5 h), with an average of 1.2 mg/L. Concurrently, the effluent COD remained below 50 mg/L (as shown in Figure 3A). The water quality, with TN at 1.2 mg/L, was near the limit of biological nitrogen removal (Oleszkiewicz and Barnard, 2006). In China, TN values below 1 mg/L are even regulated as drinking water source standards (GB3838-2002). The achievement of ultra-low TN emission was attributed to the advantages of heterotrophic denitrification by immobilized microorganisms, wherein various dominant microbial populations could acclimate and rapidly propagate (Gan et al., 2020).
Continuing the operation of the I-BAF for over a month resulted in the complete formation of mature biofilm, as depicted in Supplementary Figure A1, which appeared in a colloidal state with a milky white appearance. The time from microbial inoculation to biofilm maturation typically takes about 2–3 weeks. The duration of biofilm maturation varies depending on factors such as inoculum type, media, nutrients, and temperature. Visually, mature biofilms display a significant amount of gel-like active microorganisms firmly attached to the carrier. Moreover, reactors with mature biofilms can quickly remove nitrogen compounds (25 mg/L to 1 mg/L) in a very short time (0.5 h).
Figure 3C illustrates the spatial distribution of TN in the mature biofilm I-BAF. In this depiction, cooler colors represent lower TN concentrations, while warmer colors indicate higher concentrations. The effluent TN concentrations were recorded as 21, 15.9, 0.6, 0.2, 0.5, and 8.7 mg/L at HRT of 6, 4, 2, 1, 0.5, and 0.25 h, respectively. Notably, the effluent quality achieved at HRT of 2 to 0.5 h met the target of TN < 1 mg/L. Particularly, an HRT of 0.5 h was deemed optimal as it achieved an effluent TN of 0.5 mg/L while maximizing the utilization of the biofilter. To the best of our knowledge, the minimum HRT achieved in biofilters for denitrification is 1.5 h (Cao et al., 2014). Thus, further reductions in HRT could lead to savings in building costs and area requirements. Conversely, some studies have set HRT to more than 6 h (Chen et al., 2019; Ryu and Lee, 2009), a practice deemed improper during the stable operation stage of mature biofilm based on our research findings.
The batch test conducted in Section 2.2 suggested that the minimum HRT might fall within the range of 0.5−0.25 h. However, the continuous-flow operation of the I-BAF (Figure 3) revealed that achieving an HRT of 0.25 h was challenging (with an average TN concentration of 9.8 mg/L) due to the hydraulic load exceeding the maximum capacity of BAFL. It is worth noting that a larger HRT was also not suitable for nitrogen removal (e.g., effluent TN concentration of 21 mg/L at HRT 6) due to the accumulation of ammonia at this point. Figure 4 illustrated the spatial distribution of three types of nitrogen. Ammonia remained at high concentrations (ranging from 10.6 to 21.5 mg/L) when HRT was between 4 and 6 h (Figure 4C), indicating that most nitrate was converted to ammonia nitrogen instead of nitrogen gas. Additionally, Figure 4D demonstrated that larger HRTs (4–6 h) also resulted in COD levels exceeding standards (GB 18918-2002).
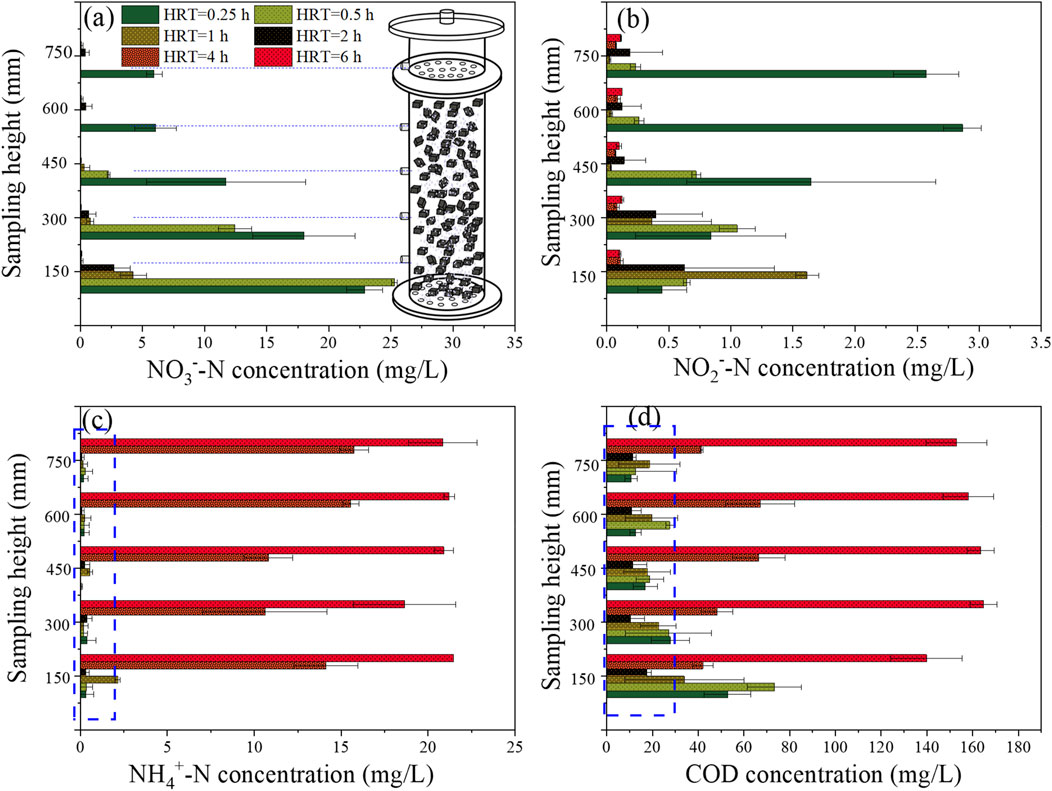
Figure 4. Spatial distribution of (A) nitrate, (B) nitrite, (C) ammonia and (D) COD under different HRT.
Figure 4A demonstrates that NO3−-N could be effectively removed under HRTs ranging from 6 to 0.5 h, with the exception of 0.25 h. The spatial distribution of nitrite is illustrated in Figure 4B. Notably, when the HRT was 0.25 h, the effluent nitrite concentration remained at 2.6 mg/L (at a height of 750 mm). Such level of nitrite concentration is still highly toxic to aquatic organisms (Lewis and Morris, 1986). Nitrite was also observed to be generated at other HRTs, such as 0.5–6 h, but it could be degraded to lower concentrations in the effluent. The results indicate that the biofilter undergoes a process of nitrite accumulation followed by degradation as the sampling height increases (Qiu and Ma, 2005).
3.3 Maximum denitrifying load in lab-scale I-BAF
The previous tests indicated that the optimal condition was an HRT of 0.5 h. This section aimed to explore the maximum denitrifying load using higher NO3−-N concentrations in the influent (50 mg/L and 100 mg/L). As shown in Figure 5A, increasing the influent NO3−-N concentration to 100 mg/L resulted in the deterioration of effluent quality, with NO3−-N reaching 54.6 mg/L. Figure 5B calculated the denitrifying load (Dload) at three stages, resulting in values of 1.26 kg (TN)/(m3·d), 2.2 kg (TN)/(m3·d), and 2.1 kg (TN)/(m3·d) respectively. The Dload peaked at 2.2 kg (TN)/(m3·d) when the influent nitrate concentration was 50 mg/L, with the effluent quality slightly higher (NO3−-N = 4.4 mg/L). However, the load remained unchanged as the influent nitrate concentration increased from 50 mg/L to 100 mg/L. Therefore, the I-BAF described in this article is suitable for influent nitrate concentrations ranging from 0 to 50 mg/L in wastewater treatment applications (HRT = 0.5 h).
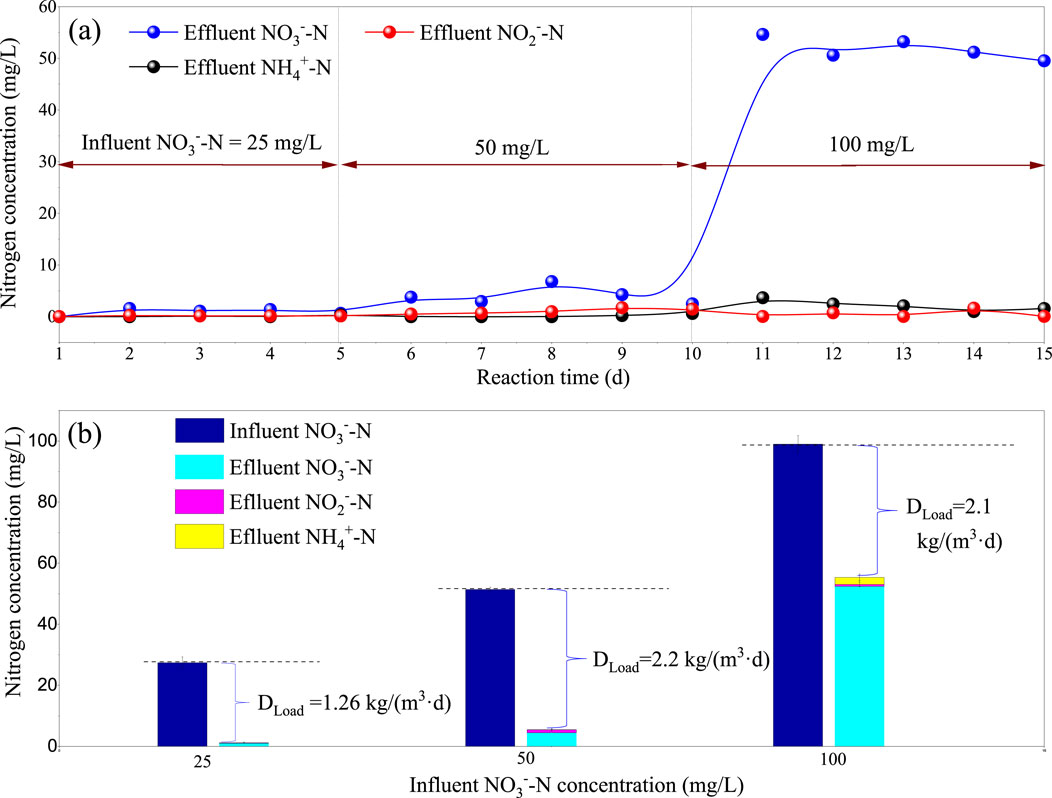
Figure 5. Effluent index (A) and denitrification load (B) Dload under influent nitrate nitrogen concentrations of 25, 50, and 100 mg/L.
When influent nitrate nitrogen increased from 50 to 100 mg/L, the filter was punctured, and Dload did not continue to rise, indicating that the maximum nitrogen removal load of the filter was 2.2 kg/(m3·d). Other conditions: HRT = 0.5 h, T = 25°C, pH = 7.5.
Table 2 presents the denitrifying load and water quality parameters from relevant studies. Wang et al. achieved a high denitrifying load of 0.84 kg (TN)/(m3·d) when treating effluent from the secondary sedimentation tank, although the effluent TN concentration was slightly higher at 10.3 mg/L (Wang et al., 2014). In contrast, Han’s research achieved a lower effluent TN concentration (5.75 mg/L), but their denitrifying load was just 0.04 kg (TN)/(m3·d) (Han et al., 2015). Few studies have examined the relationship between the lowest effluent TN and the maximum denitrifying load in actual biofilters. Table 2 demonstrates that both the denitrifying load and effluent quality in our research were superior to current technologies. In this study, we explored the limit parameters. By setting the HRT to 0.5 h and influent TN to 50 mg/L, we achieved the maximum denitrifying load of 2.2 kg (TN)/(m3·d). Furthermore, under an HRT of 0.5 h and influent TN of 25 mg/L, the I-BAF could treat up to 48 times its own volume of wastewater every day while maintaining an effluent TN concentration below 1 mg/L.
3.4 Denitrification performance and load analysis of pilot-sacle I-BAF
Figure 6 illustrates the nitrogen removal performance of the pilot-scale I-BAF (active volume = 0.5 m3). The continuous-flow experiment was divided into two phases: phase I (15 days) and phase II (49 days). Figures 6A, B indicate that nitrate levels during phase I remained high (10–15 mg/L), ultimately resulting in elevated TN concentrations. The primary reason for the low denitrifying efficiency was the fluctuation in dissolved oxygen (DO) levels in BAF1 and BAF2, ranging between 2.2 and 7.5 mg/L, which was not conducive to the survival of denitrifying bacteria (Gómez et al., 2002). These results suggest that ordinary air aeration alone could not achieve ultra-low nitrogen emissions (1 mg/L). To address this issue, intermittent aeration (30 min on, 30 min off) was adopted in phase II (Zhang et al., 2017). The results of phase II demonstrated that effluent nitrate quickly dropped to 1 mg/L, achieving the same water quality as observed in the lab-scale test (using N2 aeration). The 30-min aeration and 30-min no aeration pattern were determined based on the nitrate removal rate (Figure 1), which indicated that the mature biofilm could effectively remove nitrate within 30 min. Subsequent tests showed that TN levels remained relatively stable under different HRTs (12, 6, 4, 2, 1 h). However, at an HRT of 0.5 h, the nitrate concentration in the pilot-scale system increased slightly to a higher level (2.5 mg/L) compared to the lab-scale test (1.2 mg/L).
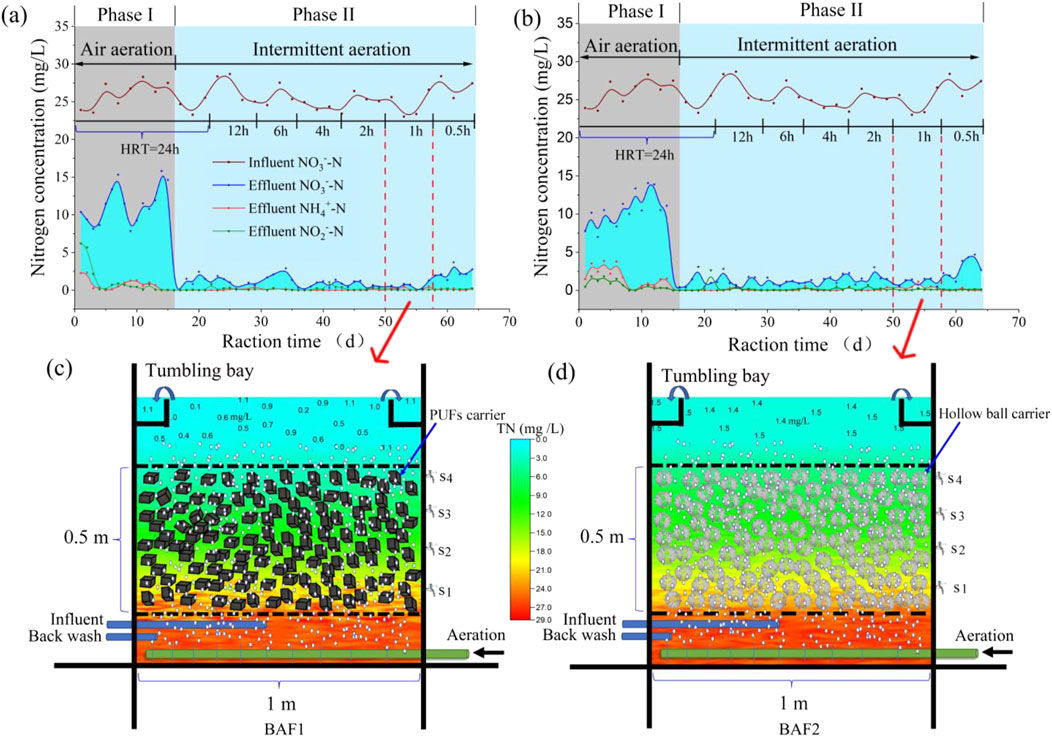
Figure 6. (A) Nitrogen removal performance of I-BAF packed with PUFs (A) and CHB (B). TN spatial distribution of PUFs I-BAF (C) and CHB I-BAF (D) when HRT was 1 h.
Figures 6C, D illustrates the spatial distribution profile of TN at an HRT of 1 h. It demonstrates that both BAF1 and BAF2 achieved ultra-low nitrate emissions. The effluent quality of BAF1 (1.1 mg/L) was superior to that of BAF2 (1.5 mg/L), indicating that the PUFs carrier performed better than the CHB carrier. Additionally, the SS in BAF1 (3.6 mg/L) was significantly lower than that in BAF2 (32.4 mg/L), with the latter exceeding Chinese standards (10 mg/L). Generally speaking, the I-BAF with PUFs was more conducive to biofilm culturing, resulting in clearer effluent water compared to the I-BAF with CHBs.
In summary, this study successfully achieved high-efficiency denitrification (TN near 1 mg/L) and obtained the maximum denitrifying load (denitrifying load = 1.26–2.2 kg (TN)/(m3·d)) in lab-scale experiments. Pilot-scale tests also achieved effluent TN concentrations of 1.1–1.5 mg/L at the minimum HRT of 1 h. The results from both lab-scale and pilot-scale tests could significantly reduce the facility construction costs of advanced wastewater treatment.
3.5 Microbial community analysis
Microbial community analysis is essential for understanding the composition and dynamics of microbial populations, which directly influence the efficiency of nitrogen removal processes. As highlighted by Du et al. (2016), such analyses offer valuable insights into the underlying principles governing nitrogen removal in biological systems. In this study, principal components analysis (PCA) was employed to categorize the samples into four distinct groups, as shown in Figure 7B. This classification reveals that samples originating from the same biological filter exhibit similar microbial community structures, indicating a degree of uniformity in microbial interactions and functional capabilities within individual systems. Furthermore, it is important to note that these microbial structures can evolve over time, particularly during the cultivation process in the integrated biological aerated filter (I-BAF). Variations in microbial composition may arise due to changes in operational conditions, nutrient availability, or external environmental factors.
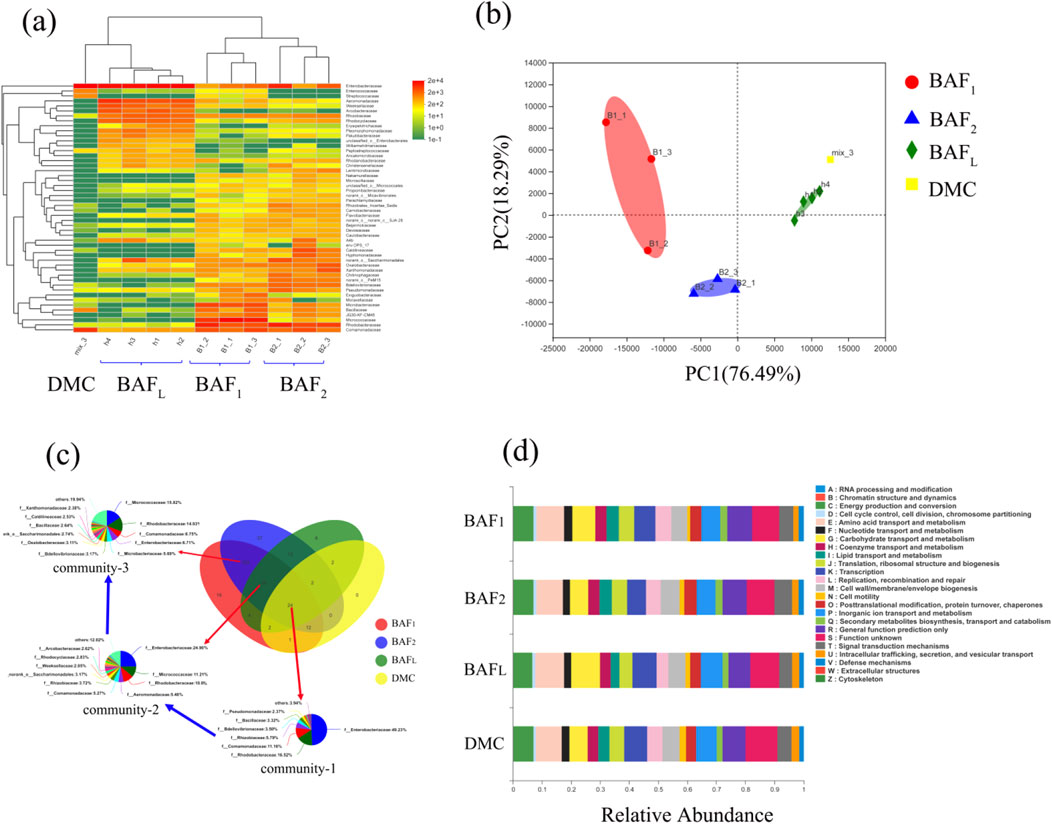
Figure 7. Microbial diversity analysis of community, (A) Community heatmap analysis on family level, (B) Principal components analysis on family level, (C) Species succession analysis, (D) COG function classification.
In Figure 7A, the analysis of the denitrifying microbial community (DMC) reveals that it primarily consists of three genera: Enterobacter, Comamonas, and Bacillus. This relatively simple composition indicates that the DMC used for inoculation contains relatively few types of denitrifying functional bacteria.
On the other hand, the biological aerated filter with high hydraulic loading (BAFL) displays a more complex microbial diversity, predominantly containing Enterobacter, Aeromonas, Comamonas, and Rhodococcus (The microbial species with the abundance greater than e^2 exceed 20 types). This diversity suggests that BAFL offers a more favorable environment for various microbial interactions, which can enhance the overall efficiency of nitrogen removal processes. Notably, BAF1 and BAF2 exhibited greater microbial similarity, primarily featuring Rhodococcus, Comamonas, Micrococcus, and Enterobacter, indicating that these systems may operate under similar conditions and support similar microbial populations.
The principal components analysis of BAFL reveals minimal differences in microbial diversity within the spatial distribution of the mature biofilm (Figure 7B), suggesting a stable and well-established community structure. Enterobacter consistently appeared across all four groups, a finding that aligns with previous studies indicating its role as a highly tolerant denitrifying bacterium (Ma et al., 2021). Furthermore, a comparison of microbial diversity shows that the mature biofilm, including BAFL, BAF1, and BAF2, contains a broader variety of microbial species than the DMC, as illustrated in Supplementary Figure A4. This observation highlights the significant proliferation of microorganisms during the biofilm culture process, which is crucial for optimizing bioreactor performance. The increased microbial diversity within the biofilm is likely to enhance the functional capabilities of the system, thereby improving nitrogen removal efficiency and overall treatment outcomes.
Figure 7C illustrates the presence of Enterobacter, Micrococcus, and Rhodococcus across all four groups, highlighting the capability of these three genera to perform both aerobic and anaerobic denitrification, as supported by various studies (Chen et al., 2012; Mpongwana et al., 2000; Tait, 1975). This versatility is crucial for optimizing nitrogen removal processes in varying environmental conditions. Notably, Rhodococcus was more identified in the biofilters (BAFL, BAF1, BAF2) instead of DMC, suggesting that this genus may have been introduced and established during the culture process, as indicated by Sun et al. (2021). This observation underscores the adaptive nature of microbial communities in response to specific operational conditions.
Despite the differences in microbial composition, the four groups displayed similar functional metabolic pathways based on COG pathway analysis. The primary functions identified were related to essential microbial processes, including carbohydrate transport and metabolism, energy production, and transcription, as depicted in Figure 7D. This functional consistency across diverse microbial communities indicates that, while the composition may vary, the fundamental metabolic capabilities necessary for effective denitrification remain intact. In conclusion, Enterobacter emerges as the dominant denitrifier in both anaerobic and aerobic processes, followed closely by Comamonas and Rhodococcus. This dominance suggests that enhancing the growth of these key microbial populations could significantly improve nitrogen removal efficiency in wastewater treatment systems. Additionally, the abundance of the aforementioned three types of denitrifying bacteria could serve as a criterion for assessing the denitrification capacity of future biological filters.
4 Conclusion
The lab-scale and pilot-scale I-BAF were constructed to investigate denitrification performance and maximum denitrifying load. During the adaptation phase, nitrite would accumulate within the initial 20 h. In the lab-scale I-BAF, the nitrate degradation process during the adaptation stage followed zero-order kinetics, while during the continuous-flow stage, it conformed to pseudo-first-order kinetics. The maximum denitrifying load was 2.2 kg (TN)/(m3·d) under conditions of influent NO3−-N = 50 mg/L and HRT = 0.5 h, achieving an effluent TN of 4.4 mg/L. The optimal combination was influent NO3−-N = 25 mg/L and HRT = 0.5 h, which could achieve a water quality of TN < 1 mg/L. Spatial distribution tests of mature biofilm revealed that the proper HRT ranged from 2 h to 0.5 h. Longer HRT led to ammonia accumulation, while much shorter HRT caused effluent NO2−-N levels to exceed 2.6 mg/L. Microbial diversity analysis showed that the genera Enterobacter were dominant in all denitrifying systems, followed by Comamonas and Rhodococcus, and the abundance of the aforementioned three types of denitrifying bacteria could serve as a criterion for assessing the denitrification capacity of future biological filters. This study combined batch tests and continuous-flow experiments to explore ultra-low TN emissions, providing support for further improvements in advanced wastewater treatment in future plants.
Data availability statement
The raw data supporting the conclusions of this article will be made available by the authors, without undue reservation.
Ethics statement
Ethical approval was not required for the study involving humans in accordance with the local legislation and institutional requirements. Written informed consent to participate in this study was not required from the participants or the participants’ legal guardians/next of kin in accordance with the national legislation and the institutional requirements.
Author contributions
JL: Conceptualization, Methodology, Writing–original draft. ZN: Software, Validation, Writing–review and editing. LL: Conceptualization, Methodology, Writing–review and editing. SZ: Software, Validation, Writing–review and editing.
Funding
The author(s) declare that financial support was received for the research, authorship, and/or publication of this article. This research was funded by the Qin Chuangyuan Introduced High-level Innovation and Entrepreneurship Talent Project (QCYRCXM-2022-299), Postdoctoral Program in Shaanxi Province (2023BSHGZZHQYXMZZ48), This study was supported by The technology Innovation Center for Land Engineering and Human Settlements, Shaanxi Land Engineering Construction Group Co. Ltd. and Xi’an Jiaotong University (Program No. 2021WHZ0093).
Conflict of interest
Authors JL, ZN were employed by Shaanxi Provincial Land Engineering Construction Group, Xi’an, China. Author LL was employed by Kweichow Moutai Winery (Group) Health Wine Co., LTD., Renhuai, China.
The authors declare that this study received funding from Shaanxi Land Engineering Construction Group Co. Ltd. The funder had the following involvement in the study: decision to submit it for publication.
The remaining author declares that the research was conducted in the absence of any commercial or financial relationships that could be construed as a potential conflict of interest.
Publisher’s note
All claims expressed in this article are solely those of the authors and do not necessarily represent those of their affiliated organizations, or those of the publisher, the editors and the reviewers. Any product that may be evaluated in this article, or claim that may be made by its manufacturer, is not guaranteed or endorsed by the publisher.
Supplementary material
The Supplementary Material for this article can be found online at: https://www.frontiersin.org/articles/10.3389/fbioe.2024.1463544/full#supplementary-material
References
Bacquet, G., Joret, J., Rogalla, F., and Bourbigot, M. M. (1991). Biofilm start-up and control in aerated biofilter. Environ. Technol. 12 (9), 747–756. doi:10.1080/09593339109385066
Bosander, J., and Westlund, A. D. (2000). Operation of full-scale fluidized bed for denitrification. Water Sci. Technol. 41 (9), 115–121. doi:10.2166/wst.2000.0184
Breda, I. L., Søborg, D. A., Ramsay, L., and Roslev, P. (2019). Manganese removal processes during start-up of inoculated and non-inoculated drinking water biofilters. Water Qual. Res. J. 54 (1), 47–56. doi:10.2166/wqrj.2018.016
Cao, X., Ai, N., and Meng, X. (2014). Simultaneous removal of di-(2-ethylhexyl) phthalate and nitrogen in a laboratory-scale pre-denitrification biofilter system. Bioresour. Technol. 156, 29–34. doi:10.1016/j.biortech.2013.12.128
Chang, H., and Wang, H. (2015). Advanced treatment technology for city tail water and its effects. Ecol. Environ. Sci. doi:10.16258/j.cnki.1674-5906.2015.03.013
Chen, H., Liu, Y., Xu, X., Sun, M., Jiang, M., Xue, G., et al. (2019). How does iron facilitate the aerated biofilter for tertiary simultaneous nutrient and refractory organics removal from real dyeing wastewater? Water Res. 148, 344–358. doi:10.1016/j.watres.2018.10.059
Chen, P., Ji, L., Li, Q. X., Wang, Y., Li, S., Ren, T., et al. (2012). Simultaneous heterotrophic nitrification and aerobic denitrification by bacterium Rhodococcus sp. CPZ24. Bioresour. Technol. 116, 266–270. doi:10.1016/j.biortech.2012.02.050
Cui, B., Zhang, C., Fu, L., Zhou, D., and Huo, M. (2023). Current status of municipal wastewater treatment plants in North-east China: implications for reforming and upgrading. Front. Environ. Sci. and Eng. 17 (6), 73. doi:10.1007/s11783-023-1673-5
Das, S., Devi, T., Goswami, M., Yenuganti, M., Bhardwaj, P., Ghosh, S., et al. (2021). Oxygen atom transfer promoted nitrate to nitric oxide transformation: a step-wise reduction of nitrate→ nitrite→ nitric oxide. Chem. Sci. 12 (31), 10605–10612. doi:10.1039/D1SC00803J
Deng, Y., and Wheatley, A. (2018). Mechanisms of phosphorus removal by recycled crushed concrete. Int. J. Environ. Res. Public Health. 15 (2), 357. doi:10.3390/ijerph15020357
Deng, Y. H., Wu, K., Yang, P. J., Zhao, Q. L., and Ye, Z. F. (2021). Development history and technical suggestions of urban sewage treatment plants in China. Industrial water and wastewater 52 (4), 1–5. doi:10.3969/j.issn.1009-2455.2021.04.001
Du, R., Cao, S., Li, B., Niu, M., Wang, S., and Peng, Y. (2016). Performance and microbial community analysis of a novel DEAMOX based on partial-denitrification and anammox treating ammonia and nitrate wastewaters. Water Res. 108, 46–56. doi:10.1016/j.watres.2016.10.051
Gan, Y., Ye, Z., Zhao, Q., Li, L., and Lu, X. (2020). Spatial denitrification performance and microbial community composition in an up-flow immobilized biofilter for nitrate micro-polluted water treatment. J. Clean. Prod. 258, 120913. doi:10.1016/j.jclepro.2020.120913
Gan, Y., Zhao, Q., and Ye, Z. (2019). Denitrification performance and microbial diversity of immobilized bacterial consortium treating nitrate micro-polluted water. Bioresour. Technol. 281, 351–358. doi:10.1016/j.biortech.2019.02.111
Gómez, M., Hontoria, E., and González-López, J. (2002). Effect of dissolved oxygen concentration on nitrate removal from groundwater using a denitrifying submerged filter. J. Hazard. Mater. 90 (3), 267–278. doi:10.1016/S0304-3894(01)00353-3
Han, J. H., Liu, Y., Zhu, H. J., Wei-Ping, L. I., and Jing, S. Y. (2015). Biofilm Formation and startup in denitrification biofilter. China Water Wastewater. doi:10.19853/j.zgjsps.1000-4602.2015.03.001
Hong, P., Wu, X., Shu, Y., Wang, C., Tian, C., Gong, S., et al. (2019). Denitrification characterization of dissolved oxygen microprofiles in lake surface sediment through analyzing abundance, expression, community composition and enzymatic activities of denitrifier functional genes. Amb. Expre 9, 129. doi:10.1186/s13568-019-0855-9
James, S. N., and Vijayanandan, A. (2023). Recent advances in simultaneous nitrification and denitrification for nitrogen and micropollutant removal: a review. Biodegradation 34 (2), 103–123. doi:10.1007/s10532-023-10015-8
Jing, Z. Q., Cao, Y. Y., Liu, S. W., and Liu, Y. (2012). Performance of double-layer biofilter packed with coal fly ash ceramic granules in treating highly polluted river water. Bioresour. Technol. 120, 212–217. doi:10.1016/j.biortech.2012.06.069
Lewis, W. M., and Morris, D. P. (1986). Toxicity of nitrite to fish: a review. Trans. Am. Fish. Soc. 115 (2), 183–195. doi:10.1577/1548-8659(1986)115<183:TONTF>2.0.CO,2
Li, X., Paul, E., Qiu, J., Roustan, M., and Mauviot, P. (2010). Rural sewage treatment by using combined process of multi-layer bio-filter and constructed Wetland. Am. Inst. Phys., 185–188. doi:10.1063/1.3529271
Liu, A., Nelson, M. J., Wang, X., Li, H., He, X., Zhao, Z., et al. (2021). Decentralized wastewater treatment in an urban setting: a pilot study of the circulating fluidized bed bioreactor treating septic tank effluent. Environ. Technol. 42 (12), 1911–1921. doi:10.1080/09593330.2019.1683614
Ma, T. F., Chen, Y. P., Yan, P., Fang, F., Feng, L., Mao, Z., et al. (2021). Adaptation mechanism of aerobic denitrifier Enterobacter cloacae strain HNR to short-term ZnO nanoparticle stresses. Environ. Res. 197, 111178. doi:10.1016/j.envres.2021.111178
Mpongwana, N., Mekuto, K. O., Mekuto, L., Akinpelu, E. A., Dyantyi, S., and Mpentshu, Y. (2000). Isolation of high-salinity-tolerant bacterial strains, Enterobacter sp., Serratia sp., Yersinia sp., for nitrification and aerobic denitrification under cyanogenic conditions. Water Sci. Technol. 73 (9), 2168–2175. doi:10.2166/wst.2016.070
Oleszkiewicz, J. A., and Barnard, J. L. (2006). Nutrient removal technology in north America and the European union: a review. Water Qual. Res. J. Can. 41 (4), 449–462. doi:10.2166/wqrj.2006.048
Omar, A., Almomani, F., Qiblawey, H., and Rasool, K. (2024). Advances in nitrogen-rich wastewater treatment: a comprehensive review of modern technologies. Sustainability 16 (5), 2112. doi:10.3390/su16052112
Qiu, L., and Ma, J. (2005). A novel approach to remove nitrogen in a biological aerobic filter (BAF). Environ. Technol. Lett. 26 (8), 923–930. doi:10.1080/09593332608618503
Ryu, H.-D., and Lee, S. (2009). Comparison of 4-stage biological aerated filter (BAF) with MLE process in nitrogen removal from low carbon-to-nitrogen wastewater. Environ. Eng. Sci. 26 (1), 163–170. doi:10.1089/ees.2007.0256
Sheik, A. G., Tejaswini, E. S. S., Seepana, M. M., and Ambati, S. R. (2023). Control of anaerobic-anoxic-aerobic (A2/O) processes in wastewater treatment: a detailed review. Environ. Technol. Rev. 12 (1), 420–440. doi:10.1080/21622515.2023.2220892
Simon, M., Joshi, H., and Hazra, M. (2023). Performance appraisal of microbial inoculants treating polluted surface water through bench-scale investigation. J. Chem. Technol. and Biotechnol. 98 (10), 2462–2476. doi:10.1002/jctb.7472
Smet, E., Chasaya, G., Van Langenhove, H., and Verstraete, W. (1996). The effect of inoculation and the type of carrier material used on the biofiltration of methyl sulphides. Appl. Microbiol. Biotechnol. 45 (1), 293–298. doi:10.1007/s002530050686
Štembal, T., Markić, M., Briški, F., and Sipos, L. (2004). Rapid start-up of biofilters for removal of ammonium, iron and manganese from ground water. J. Water Supply Res. Technol. 53 (7), 509–518. doi:10.2166/aqua.2004.0040
Su, C., Zhang, F., Cui, X., Cheng, Z., and Zheng, Z. (2020). Source characterization of nitrate in groundwater using hydrogeochemical and multivariate statistical analysis in the Muling-Xingkai Plain, Northeast China. Environ. Monit. Assess. 192 (7), 456. doi:10.1007/s10661-020-08347-6
Su, H., Ding, L., Wang, X., Pan, Y., Hu, H., Kan, L., et al. (2018). Biochar carrier application for nitrogen removal of domestic WWTPs in winter: challenges and opportunities. Appl. Microbiol. Biotechnol. 102, 9411–9418. doi:10.1007/s00253-018-9317-6
Sun, J., Su, C., Xie, Y., Huang, Z., Lin, X., Chen, M., et al. (2021). Rare earth mine wastewater treatment via modified constructed rapid infiltration system: nitrogen removal performance and microbial community. Process Saf. Environ. Prot. 150 (2), 223–232. doi:10.1016/j.psep.2021.04.008
Tait, G. H. (1975). The identification and biosynthesis of siderochromes formed by Micrococcus denitrificans. Biochem. J. 146 (1), 191–204. doi:10.1042/bj1460191
Wang, B., Mei, F., Xu-Ning, L. I., and Liu, H. (2014). Denitrifying biofilter for advanced nitrogen removal treatment of liquor wastewater. China Water Wastewater. doi:10.19853/j.zgjsps.1000-4602.2014.17.032
Wang, T., Li, X., Wang, H., Xue, G., Zhou, M., Ran, X., et al. (2023). Sulfur autotrophic denitrification as an efficient nitrogen removals method for wastewater treatment towards lower organic requirement: a review. Water Res. 245, 120569. doi:10.1016/j.watres.2023.120569
Wu, L., Wei, W., Xu, J., Chen, X., Liu, Y., Peng, L., et al. (2021). Denitrifying biofilm processes for wastewater treatment: developments and perspectives. Environ. Sci. Water Res. Technol. 7 (1), 40–67. doi:10.1039/D0EW00576B
Yang, C., Zhang, X., Tang, Y., Jiang, Y., Zhang, Y., Qin, Y., et al. (2022). Selection and optimization of the substrate in constructed wetland: a review. J. Water Process Eng. 49, 103140. doi:10.1016/j.jwpe.2022.103140
Yao, J., Mei, Y., Jiang, J., Xia, G., and Chen, J. (2022). Process optimization of electrochemical treatment of COD and total nitrogen containing wastewater. Int. J. Environ. Res. Public Health. 19 (2), 850. doi:10.3390/ijerph19020850
Yaqoob, A. A., Guerrero–Barajas, C., Ahmad, A., Ibrahim, M. N. M., and Alshammari, M. B. (2023). “Advanced technologies for wastewater treatment,” in Green chemistry for sustainable water purification, 179–202. doi:10.1002/9781119852322.ch8
Ye, Z., Wen, L., Borthwick, A., and Ni, J. (2005). Aerobic biological fluidized bed technology treatment of sulfur and high ammonia-nitrogen waste water in a petroleum refinery. J. Basic Sci. Eng. 13 (4), 345–357. doi:10.1360/biodiv.050058
Yue, Q., Han, S., Yue, M., Gao, B., Li, Q., Yu, H., et al. (2009). The performance of biological anaerobic filters packed with sludge-fly ash ceramic particles (SFCP) and commercial ceramic particles (CCP) during the restart period: effect of the C/N ratios and filter media. Bioresour. Technol. 100 (21), 5016–5020. doi:10.1016/j.biortech.2009.05.033
Zhang, F. Z., Peng, Y., Miao, L., Wang, Z., Wang, S., and Li, B. (2017). A novel simultaneous partial nitrification Anammox and denitrification (SNAD) with intermittent aeration for cost-effective nitrogen removal from mature landfill leachate. Chem. Eng. J. 313, 619–628. doi:10.1016/j.cej.2016.12.105
Zhang, M., Gao, J., Liu, Q., Fan, Y., and Wu, J. (2020). Nitrite accumulation and microbial behavior by seeding denitrifying phosphorus removal sludge for partial denitrification (PD): the effect of COD/NO3- ratio. Bioresour. Technol. 323 (4), 124524. doi:10.1016/j.biortech.2020.124524
Zhang, Y., Douglas, G. B., Kaksonen, A. H., Cui, L., and Ye, Z. (2019). Microbial reduction of nitrate in the presence of zero-valent iron. Sci. Total Environ. 646, 1195–1203. doi:10.1016/j.scitotenv.2018.07.112
Zhang, Y., Yin, Z., Liu, M., and Liu, C. (2024). Enhancing simultaneous nitrification and denitrification in a plant-scale integrated fixed-film activated sludge system: focusing on the cooperation between activated sludge and biofilm. Chem. Eng. J. 496, 154322. doi:10.1016/j.cej.2024.154322
Zhou, J. M., Sun, Y. J., Liao, Z. G., Ma, X., Feng, Z. T., Zhang, Q. Q., et al. (2024). Simultaneous removal of nitrate nitrogen and ammonium nitrogen by partial denitrification coupled with anammox synergy by zero-valent iron. J. Clean. Prod. 469, 143169. doi:10.1016/j.jclepro.2024.143169
Zhu, Z., Zhang, L., Li, X., Zhang, Q., Wang, S., and Peng, Y. (2023). Robust nitrogen removal from municipal wastewater by partial nitrification anammox at ultra-low dissolved oxygen in a pure biofilm system. Bioresour. Technol. 369, 128453. doi:10.1016/j.biortech.2022.128453
Keywords: immobilized biological aerated filter, total nitrogen removal, denitrification load, microbial community, advanced wastewater treatment
Citation: Li J, Niu Z, Li L and Zhou S (2024) Optimizing nitrogen removal in advanced wastewater treatment using biological aerated filters. Front. Bioeng. Biotechnol. 12:1463544. doi: 10.3389/fbioe.2024.1463544
Received: 12 July 2024; Accepted: 08 November 2024;
Published: 28 November 2024.
Edited by:
Helen Treichel, Federal University of the Southern Frontier, BrazilReviewed by:
Jadiane Paola Cavaler, Western Parana State University, BrazilAirton Kunz, EMBRAPA Swine and Poultry, Brazil
Copyright © 2024 Li, Niu, Li and Zhou. This is an open-access article distributed under the terms of the Creative Commons Attribution License (CC BY). The use, distribution or reproduction in other forums is permitted, provided the original author(s) and the copyright owner(s) are credited and that the original publication in this journal is cited, in accordance with accepted academic practice. No use, distribution or reproduction is permitted which does not comply with these terms.
*Correspondence: Lei Li, bGlsZWlwa3VAMTI2LmNvbQ==