- 1Department of Pathology and Laboratory Medicine, University of Rochester School of Medicine and Dentistry, Rochester, NY, United States
- 2Department of Orthopedics and Physical Performance, Center for Musculoskeletal Research, University of Rochester Medical Center, Rochester, NY, United States
Tendon injuries disrupt successful transmission of force between muscle and bone, resulting in reduced mobility, increased pain, and significantly reduced quality of life for affected patients. There are currently no targeted treatments to improve tendon healing beyond conservative methods such as rest and physical therapy. Tissue engineering approaches hold great promise for designing instructive biomaterials that could improve tendon healing or for generating replacement graft tissue. More recently, engineered microphysiological systems to model tendon injuries have been used to identify therapeutic targets. Despite these advances, current tissue engineering efforts that aim to regenerate, replace, or model injured tendons have largely failed due in large part to a lack of understanding of how the mechanical environment of the tendon influences tissue homeostasis and how altered mechanical loading can promote or prevent disease progression. This review article draws inspiration from what is known about tendon loading from in vivo animal models and identifies key metrics that can be used to benchmark success in tissue engineering applications. Finally, we highlight important challenges and opportunities for the field of tendon tissue engineering that should be taken into consideration in designing engineered platforms to understand or improve tendon healing.
Introduction
Tendons are dense, connective tissues that connect muscle to bone to facilitate movement within the musculoskeletal system. Though frequently injured, either through acute injury resulting from trauma or through degenerative processes (i.e., tendinopathies), current treatments are largely conservative and do not adequately address the underlying cellular dysfunction that is at the heart of the failed healing response (Irby et al., 2020). Tissue engineering offers an attractive alternative to traditional treatment modalities that could help recover, improve, and maintain tendon function (Lim et al., 2019; Ruiz-Alonso et al., 2021; Ning et al., 2023). The overall goal of tissue engineering is to regenerate, replace, or model pathological tendon tissues. The typical tissue engineering approach has been to combine various types of cells, biomaterials, bioactive molecules, and mechanical loading to try and generate tendon tissue; however, current efforts have largely failed to successfully recreate the complex tendon architecture due to an incomplete understanding of the required mechanical strain, appropriate cellular behavior and composition, spatial complexity, and tendon-specific considerations needed to model healthy or diseased tendons. More recently, tissue engineering constructs have also been used to model tissue dysfunction in the form of microphysiological systems (MPS) or “organ-on-a chip” models. These models strive to be physiologically relevant, often combining multiple compartments seeded with different interacting cell types to model either healthy tissue or pathological processes (Wang et al., 2020; Awad et al., 2023). However, largely absent from current tendon MPS is the role of mechanical strain in promoting or preventing tendon dysfunction. Though there is still much to be discovered and clarified regarding the specific cell populations, mechanisms, and structure/function relationships guiding tendon function, in vivo animal models can offer important insight into how altered mechanical loading affects tendon cell behavior, and how this translates to a tissue-level response. The goal of this review is to summarize the findings of in vivo animal models of tendon under- and over-loading and to identify key structural, histological, mechanical, and material outcomes that can be used to guide and inform the design of engineered tendon constructs and MPS. Importantly, we also highlight how a lack of understanding of key biological features of tendons impedes tissue engineering progress and identify crucial areas for future study that will enhance our ability to design accurate tendon tissue mimetics.
Proper tendon function is dependent on maintenance of a complex extracellular matrix
Critical to tendon function is the ability to withstand and recover from extreme loads, a quality that is imparted to the tissue due to the highly aligned extracellular matrix (ECM) which makes up the body of the tendon. Collagen type I is the primary constituent of the tendon ECM and comprises ∼60%–85% of the tendon dry weight (Screen et al., 2015). In addition to the collagen matrix, proteoglycans and glycosaminoglycans (GAGs) provide structural support and, by increasing water content, provide resistance to compressive force (Thorpe et al., 2013). Beyond the collagen matrix, tendons of larger mammals including humans possess a hierarchical structure wherein individual fascicles (bundles of collagen fibrils) are surrounded by other types of ECM, including the interfascicular matrix (IFM) (Patel et al., 2021) and the poorly defined endotenon (Kannus, 2000), which is surrounded by a thin, loose connective tissue called the epitenon (Nichols et al., 2023). The endotenon and epitenon are contiguous structures that house the vasculature and nerves that supply the tissue with nutrients and innervation. This complex matrix and structure allows tendons to transmit forces, dissipate energy, and prevent mechanical failure without compromising structural integrity (Franchi et al., 2007). Tendons, both healthy and injured, are frequently assessed by measuring the biomechanical properties of the tissue, which gives information on both the structural properties (e.g., stiffness, maximum load) and material quality (e.g., peak stress, elastic modulus) of the tendon. While outside the scope of this review, we point readers towards several excellent papers on the mechanical properties of tendons and means of assessment (Lomas et al., 2015; Lake et al., 2023). Cross-sectional area (CSA) is also frequently used as a metric of tendon health; however, whether in CSA are reflective of natural adaptive processes or the development of tendinopathy is unclear and likely both context- and time-dependent. Importantly, the composition of the ECM, and therefore the mechanical properties, of the tendon is dependent on the particular tendon type. For example, human Achilles tendons have increased sulphated GAG and water content compared to the anterior tibialis tendon (Birch, 2007) and lower water content is associated with a stiffer tendon (Birch, 2007), which suggests that the properties of any tendon must be considered in the proper anatomical context.
In healthy tendons, the primary resident tendon cell is a specialized type of fibroblast called the tenocyte, or tendon fibroblast. Tenocytes are interspersed sparsely throughout the tendon ECM, and appear as thin, spindle-shaped, cells oriented along the axis of tension in linear arrays. Tenocytes are thought to be the main cellular players that maintain tendon homeostasis. In response to appropriate (e.g., physiological) mechanical load, tenocytes facilitate matrix turnover and repair by upregulating the production of growth factors (e.g., transforming growth factor-β, fibroblast growth factor-2, etc.), collagen synthesis enzymes, and matrix metalloproteinases (MMPs) which ultimately preserves the integrity of the tendon ECM (Birch, 2007; Franchi et al., 2007; Subramanian et al., 2018). Precisely how tenocytes sense and respond to mechanical stimulation, or how this cellular behavior results in tissue-level changes to the tendon, is unknown. Similar to the ECM composition, the cellular density (Birch, 2007) and specific tenocyte populations present vary between tendons (Kendal et al., 2020), reinforcing the idea that different tendons must be considered as distinct entities. In addition to tenocytes, recent studies have reported the presence of innate (e.g., macrophages) and adaptive (e.g., T cells) immune cells (Kendal et al., 2020; Garcia-Melchor et al., 2021; Muscat et al., 2022; Bautista et al., 2023) within healthy tendons, though their role in tendon homeostasis, or how these populations might vary tendon to tendon is unclear. Interestingly, recent work has suggested that resident immune cells have mechanosensing capabilities in other tissues, and that changes in the local mechanical environment can activate immune cells to an inflammatory phenotype (Meli et al., 2020; Zhang et al., 2020). Whether resident immune cells play a similar role in the tendon response to loading is unknown but warrants further investigation.
Improper mechanical stimulation is a primary driver of tendinopathy
Tendinopathy is a broad term that describes a slow, degenerative process that compromises the integrity of the organized tendon ECM, leading to reduced function, limited mobility, and pain (Steinmann et al., 2020; Millar et al., 2021; Chatterjee et al., 2022). Tendinopathy is complex and multifactorial as age, sex, activity level, and obesity are all known risk factors that can increase the risk of developing tendinopathies (Millar et al., 2021); however, improper mechanical loading is believed to be the overarching primary driver of tendinopathy. Tendinopathies due to overload or overuse are thought to be a failed healing response, where supraphysiological (overload) or repetitive injurious loads (overuse) cause damage to the ECM that tenocytes are unable to repair. This leads to further damage and degeneration due to the inability of the compromised tendon to withstand the forces it experiences (Millar et al., 2021). Conversely, tendon underloading can prevent proper stimulation of matrix turnover by tenocytes which weakens the organized matrix of the tendon, leading to a similar degenerative cycle as overload injuries (Herod and Veres, 2018). As such, tendinopathic tendons frequently exhibit altered mechanical and material properties compared to healthy tendons (Arya and Kulig, 2010).
In addition to structural changes, tendinopathic tendons are generally characterized histologically by disorganization of the collagen matrix, increased staining for proteoglycans/GAGs, increased cellularity, and in some cases, increases in the presence of sensory nerve fibers and microvasculature within the body of the tendon (Fredberg and Stengaard-Pedersen, 2008), though as we detail below, the specifics of these tendinopathic changes are both tendon- and context-dependent. Tenocytes present in tendinopathic tendons can exhibit changes in cellular morphology that include loss of the linear cellular alignment and cell rounding (Fredberg and Stengaard-Pedersen, 2008). Tendinopathic tenocytes also exhibit greater transcriptional diversity compared to uninjured tenocytes (Kendal et al., 2020; Millar et al., 2021; Mimpen et al., 2024a), and increased expression of cell activation markers including alpha smooth muscle actin (a myofibroblast marker) (Kendal et al., 2020). Historically, the role of inflammation in human tendinopathy has been debated as late-stage tendinopathic tendons (when most samples are collected) frequently do not exhibit the presence of immune cells (Jomaa et al., 2020). However, other work has shown a role for inflammation in tendon degeneration (Millar et al., 2017). Tendinopathic human Achilles tendon biopsies exhibited an inflammatory signature marked by increased numbers of CD14+ and CD68+ myeloid cells and upregulation of pro-inflammatory NF-kβ and interferon pathways compared to healthy tendons (Dakin et al., 2018), which suggests that chronic inflammation may be involved in tendinopathic progression. This is supported by a rat model of collagenase-induced Achilles tendinopathy, which also showed evidence of non-resolving inflammation and accumulation of CD163+ macrophages (Marsolais et al., 2001). In addition to macrophages, T cells have been observed in tendinopathic samples from human rotator cuff, and coculture experiments demonstrated that tenocytes and T cells can stimulate each other to a pro-inflammatory phenotype (Garcia-Melchor et al., 2021). Whether these immune cells arise from resident cells or are recruited from the blood is unknown. Combined, these data suggest that both immune cells and tenocytes can promote an inflammatory environment that may lead to the development of tendinopathy, however the specific mechanisms by which this occurs remain to be determined.
It is clear that proper mechanical stimulation is required for tendon health, as too much or too little can lead to tissue degeneration. Therefore, in order for tissue engineering to create new tendon tissue (proper loading) or to model tendinopathy (underloading or overuse), it is crucial to identify multiscale features that can be used to assess failure or success of engineered constructs. Below, we outline what is known about both underloading and overuse injuries in human tendons, describe animal models that aim to replicate the human conditions, and leverage these findings to establish cellular, molecular, and structural hallmarks that can be used to guide future tendon tissue engineering efforts (Figure 1).
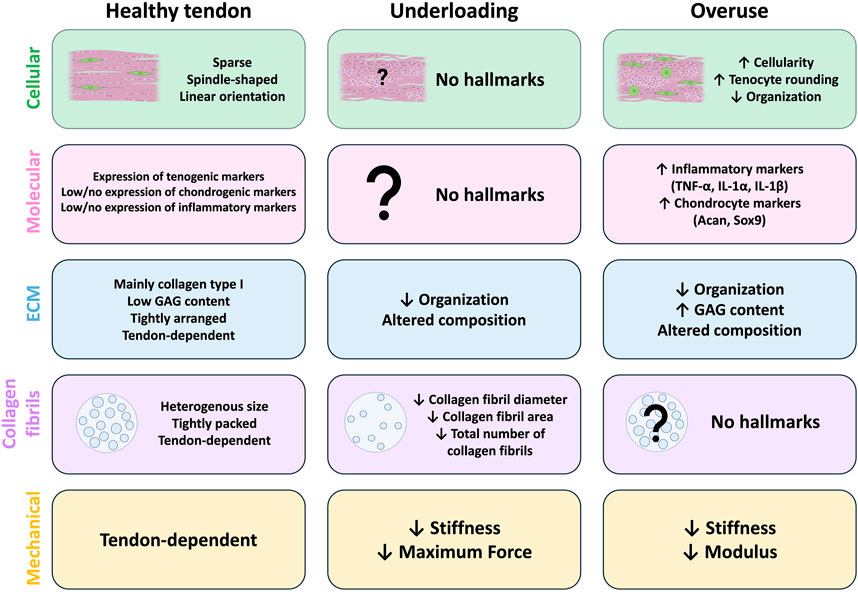
Figure 1. Hallmarks of tendon underloading and overuse compared to healthy tendons. Common cellular (green), molecular (pink), extracellular matrix (ECM; blue), collagen fibril (purple), and mechanical (yellow) hallmarks identified in animal models of healthy tendons (left) and those with underload (middle) or overuse-induced (right) tendon degeneration. In healthy tendons (left), tenocytes are sparse and arranged in linear arrays along the axis of tension. Healthy tendons typically have high expression of tenogenic markers and low/no expression of chondrogenic or inflammatory markers. The exact composition of the ECM is tendon-dependent, but the ECM of healthy tendons is highly organized, composed primarily of collagen type I with low glycosaminoglycan (GAG) content. Collagen fibrils are heterogenous in size and tightly packed, however the size composition is tendon-dependent. Mechanical and material properties of healthy tendons are also tendon-dependent. No studies report any cellular or molecular hallmarks of tendon underloading (middle). Underloading generally leads to altered ECM composition and decreased organization compared to healthy tendons. The overall number of collagen fibrils is reduced and the individual fibrils themselves are smaller, and underloaded tendons exhibit reduced stiffness and max force compared to healthy tendons. (Right) Models of tendon overuse demonstrate cellular hallmarks such as increased cellularity, tenocyte rounding, and decreased cellular organization. Molecular hallmarks include increased expression of inflammatory (TNF-α, IL-1 α, IL-1 β) and chondrocyte (Acan, Sox9) markers compared to healthy tendons. The ECM composition of overused is altered, with increased GAG content and decreased organization. Collagen fibril density and size alterations from overloading models are not reported. Overuse tendons exhibit decreased stiffness and elastic modulus compared to healthy tendons.
Tendon underloading in humans
Tendon underload is defined as the disruption of mechanical stimuli that causes a loss of load transmission to resident tendon cell populations. In humans, tendon underuse can occur during bedrest (Kubo et al., 2004; Reeves et al., 2005) or can be modeled by unilateral limb suspension via casting (Christensen et al., 2008; Couppe et al., 2012) or suspension with crutches in healthy individuals (de Boer et al., 2007a; de Boer et al., 2007b). In all cases, underloading appears to result in impaired mechanical and material properties without large structural changes. Achilles tendons of bed-rested volunteers had reductions in stiffness (58%) and elastic modulus (57%) after 90 days compared to pre-bed rest measurements with no changes in CSA (Reeves et al., 2005). Most human underuse studies see no changes in CSA but this could potentially be attributed to short periods of disuse (<90 days) (Kubo et al., 2004; Reeves et al., 2005; de Boer et al., 2007a; de Boer et al., 2007b; Christensen et al., 2008). With chronic disuse (at least 1.5 years), as seen in paralyzed patients from a previous spinal cord injury, the patellar tendon exhibited significant reductions in CSA (17%), stiffness (77%), and elastic modulus (59%) compared to able-bodied controls (Maganaris et al., 2006). Taken together, these human underuse studies suggest that impairments in mechanical and material properties occur early within the first few months of immobilization. Longer lengths of underloading are necessary to induce large structural changes in the tendon (i.e., changes in CSA).
While decreases in stiffness and elastic modulus with tendon disuse are consistent in human models of underloading, animal models are sparse and inconsistent. In this next section, we describe efforts to establish animal models of tendon disuse that model human equivalents (Figure 2). Specifics regarding the models and outcomes of all studies outlined below are provided in Table 1.
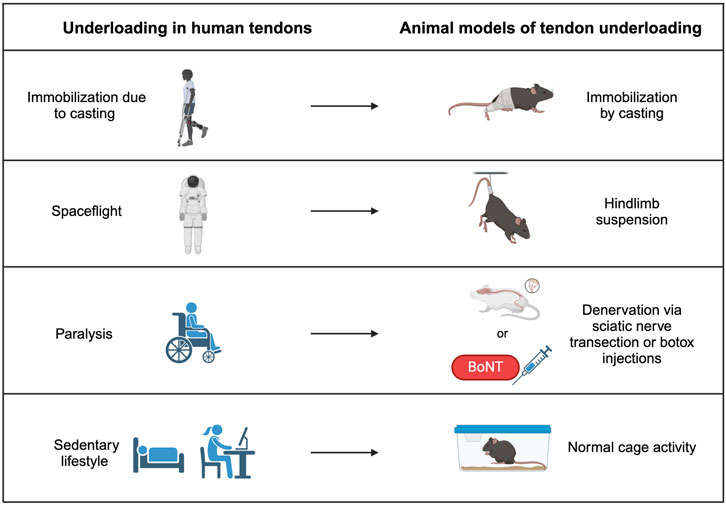
Figure 2. Tendon underloading in humans and corresponding animal models. Immobilization from a cast due to another injury causes underloading in tendon and can be modeled by casting the hindlimbs of animals. Loss of gravity observed in spaceflight can cause underloading in tendons of astronauts and is modeled by hindlimb suspension in animal models. Paralysis from a previous injury causes underloading in human tendons and can be replicated by either transecting the sciatic nerve or injecting Botulism toxin (Botox), which both prevent muscle contraction and therefore reduce load on the tendon. Sedentary lifestyles promote underloading in human tendons and may be modeled in mice by normal cage activity.
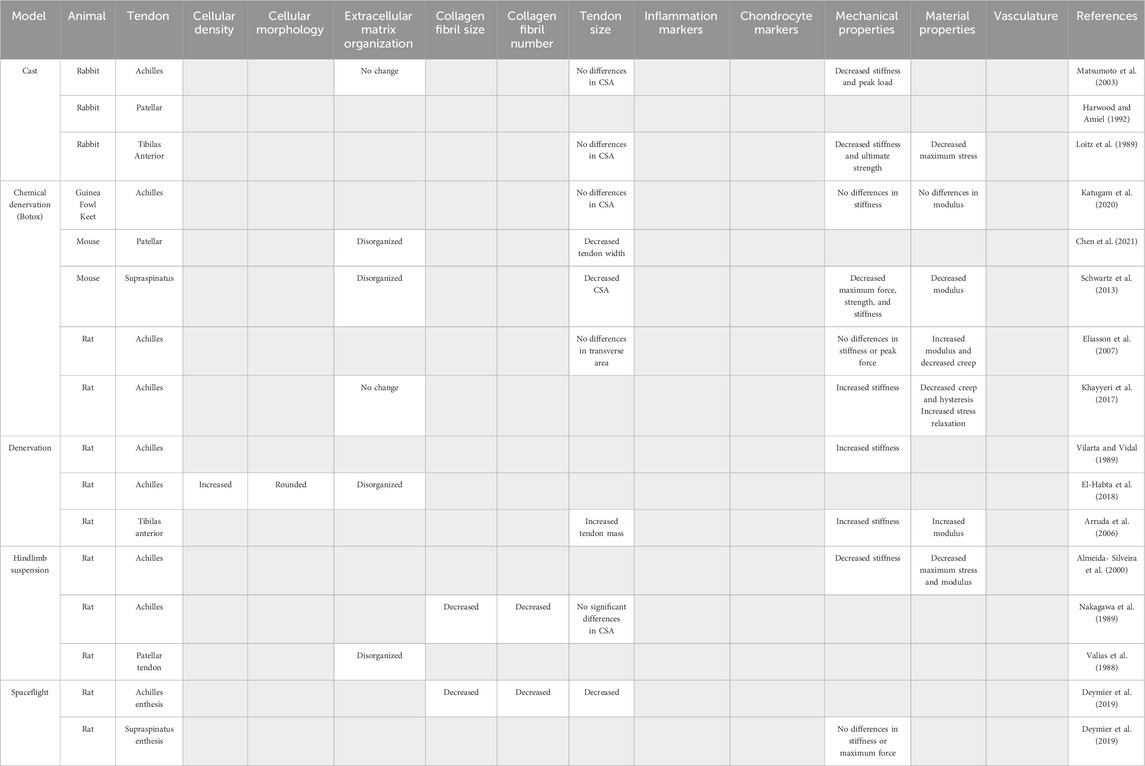
Table 1. Models of tendon underloading and their reported outcomes used to identify common hallmarks of tendon underloading. Gray boxes indicate outcome was not reported.
In vivo animal models of tendon underloading
Immobilization
To replicate tendon underloading due to casting in humans, animal models of limb casting are frequently used. In a rabbit model in which a fiberglass cast was used to prevent mechanical loading of the Achilles tendon, 4 weeks of immobilization resulted in significantly reduced stiffness (64%) and peak load (14%) with no changes to the CSA or collagen alignment (Matsumoto et al., 2003). Similarly, studies of patellar (Harwood and Amiel, 1992) and tibialis anterior tendon (Loitz et al., 1989) immobilization by casting report decreases in stiffness and ultimate strength, while changes in CSA or collagen organization were not examined.
Hindlimb suspension
Hindlimb suspension is a popular model to reduce tendon loading in animal models and is frequently used to mimic physiological responses due to lack of mechanical loading seen during spaceflight (Morey, 1979; Morey-Holton and Globus, 1998). While 20 days of hindlimb suspension is sufficient to enact structural changes in bone (Morey, 1979), hindlimb suspension models in tendon require longer durations (three to 5 weeks) (Vailas et al., 1988; Nakagawa et al., 1989; Almeida-Silveira et al., 2000). In the Achilles tendon, collagen fiber diameter decreased by 23.1% after 5 weeks of hindlimb suspension in Wistar rats compared to controls (Nakagawa et al., 1989). No changes in Achilles CSA were detected after 3 weeks of hindlimb suspensions in rats; however, both stiffness (41.5%) and maximum stress (37.4%) were significantly decreased compared to control tendons (Almeida-Silveira et al., 2000).
Denervation
To model tendon paralysis due to nerve injury in human patients, denervation via transection of the sciatic nerve in animals, which leads to limb tendon unloading, is a common model. Denervation of the sciatic nerve resulted in a 291% increase in the stiffness of rat tibias anterior tendons (Arruda et al., 2006). Disorganization of newly deposited collagen, hypercellularity, and rounded cell morphology suggest unilateral tendon unloading via sciatic nerve transection was sufficient to induce pathologic changes in rat Achilles tendons after 2 weeks (El-Habta et al., 2018).
Chemical denervation using Botulism toxin type A (Botox) injections is minimally invasive and has become a popular choice to immobilize lower extremities in rodents. Botox is a non-lethal neuromuscular blocking agent that targets motor nerve terminals in muscle with high specificity by blocking acetylcholine release, preventing action potentials and therefore muscle contraction (Brin, 1997). Studies using Botox as a model of disuse have established that muscle contraction is necessary to maintain bone mass and strength (Chappard et al., 2001; Warner et al., 2006; Grimston et al., 2007; Bach-Gansmo et al., 2016; Brent et al., 2020); however, unloading by Botox injections has yielded various results in both anatomically distinct tendons and different animals models, suggesting both a species and tendon-specific response to Botox unloading. In the rat Achilles tendon, Botox unloading lead to a 45% increase in elastic modulus and a 19% decrease in hysteresis (Eliasson et al., 2007; Khayyeri et al., 2017) with no change in CSA (Khayyeri et al., 2017) or tendon length (Eliasson et al., 2007). Other studies have reported both increased (Khayyeri et al., 2017) and no change (Eliasson et al., 2007) in rat Achilles tendon stiffness with Botox unloading. In contrast, Botox unloading of the mouse patellar tendon resulted in significantly reduced tendon width (Chen et al., 2021). The enthesis of the supraspinatus tendon demonstrated a 25% decrease in CSA and a 80% decrease in yield stress following Botox unloading (Schwartz et al., 2013). Botox unloading in avian models such as guinea fowl keets demonstrated no changes in CSA, stiffness, elastic modulus and hysteresis (Katugam et al., 2020).
Hallmarks of tendon underloading
Despite the relatively limited number of studies, it is clear that inadequate mechanical stimulation leads to altered tendon biomechanical properties; however, the magnitude and specific alterations appear to be tendon-dependent. Most short-term studies reported no changes in CSA, suggesting that long-term or chronic disuse is necessary for large-scale structural changes. Instead, reductions in collagen fiber area and diameter (Nakagawa et al., 1989) could explain mechanical and material deficits (Loitz et al., 1989; Almeida-Silveira et al., 2000; Matsumoto et al., 2003; Schwartz et al., 2013) seen with in vivo animal models of unloading. Absent from the unloading animal studies are models that replicate bedrest, or a sedentary lifestyle; however, investigators should consider that normal cage activity as seen in lab mice may be a model of unloading, given that wild mice typically run long distances (Meijer and Robbers, 2014) and when given voluntary access to running wheels, laboratory mice will run up to 20 km per day (Manzanares et al., 2018). Additionally, there are clear differences in how different tendons respond to various types of unloading. As such, it is not possible to identify a “one-size-fits-all” strain rate that will result in tendon unloading. Investigations aiming to create healthy tendon mimetics should therefore focus on identifying homeostatic levels of mechanical strain within a given tissue engineering or MPS system that will result in a collagen fibril distribution, cellular organization, and matrix composition that matches the specific tendon under investigation as success criteria for tissue engineered constructs. As there were no consistent molecular markers reported with tendon underloading, additional work is needed to identify specific molecular markers of aberrant cellular activity that could inform the development of engineered constructs that attempt to model underload-induced tendinopathy.
Tendon overuse injuries in humans
Overuse-induced tendinopathy commonly occurs in professional athletes or in people whose professions require repetitive movements over long periods of time, and is most frequent in the Achilles, elbow, rotator cuff, and patellar tendons (Herod and Veres, 2018). In contrast to human tendon underloading studies, there are few studies that examine the effects of overuse on tendon morphology, ECM, or cell behavior which may be attributed to several issues including the fact that there is likely a “threshold” response that varies from person to person, which makes clinically defining “overuse” difficult and the fact overuse injuries can take a long time to develop, which makes linking activity to clinical outcomes challenging. Nonetheless, elite athletes provide the best evidence of the effects of overuse on tendon function. The patellar tendons of volleyball players diagnosed with patellar tendinopathy (i.e., “jumper’s knee”) exhibited increased CSA (19%), decreased stiffness (32%), and decreased elastic modulus (29%) compared to their non-dominant healthy patellar tendon (Helland et al., 2013). Pain is a common symptom of overuse tendinopathy (Swank et al., 2022; Kakade, 2023) and is associated with increased innervation within tendinopathic patellar tendon of athletes from various sports (Lian et al., 2006) suggesting that increased sensory nerve ingrowth may play role in symptomatic pain felt in overuse-induced tendinopathies; however the cause of this nerve ingrowth is unknown.
In addition to the challenges mentioned above, the lack of clinical presentation and samples gathered during early phases of overuse tendinopathy also hinders the field’s ability to understand the complex etiology and pathological progression in human patients. In the next section, we identify common outcomes from in vivo animal models of tendon overuse that mimic human activities (Figure 3). These models can give provide insight into the early pathological changes that occur with tendon overuse and highlight important considerations for tissue engineering applications. The animal models, specific tendons, and outcomes of all studies outlined below are provided in Table 2.
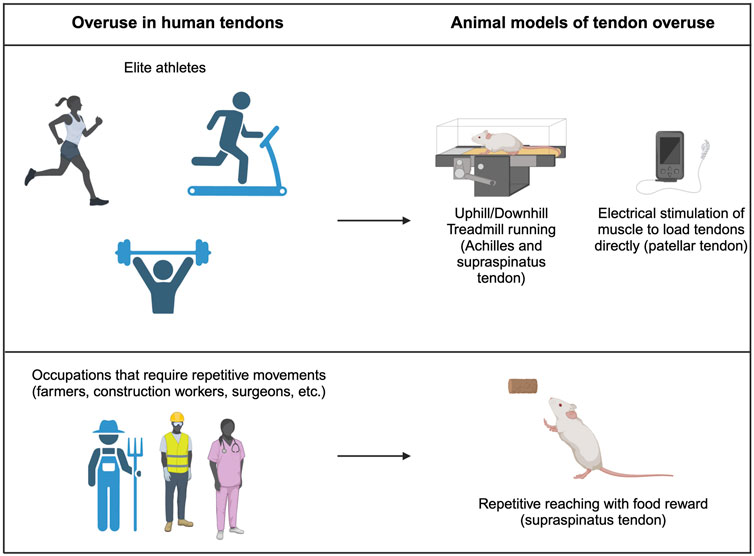
Figure 3. Tendon overuse in humans and corresponding animal models. Tendon overuse injuries in humans are best exemplified by elite athletes. Corresponding animals models use uphill and downhill treadmill running to cause overuse of the supraspinatus and achilles tendons. Additionally, direct electrical stimulation can be used to directly load patellar tendons. Physically demanding jobs that require repetitive movements such as farmers, construction workers and chefs frequently have report overuse tendon injuries which are best modeled by repetitive reach rodent models.
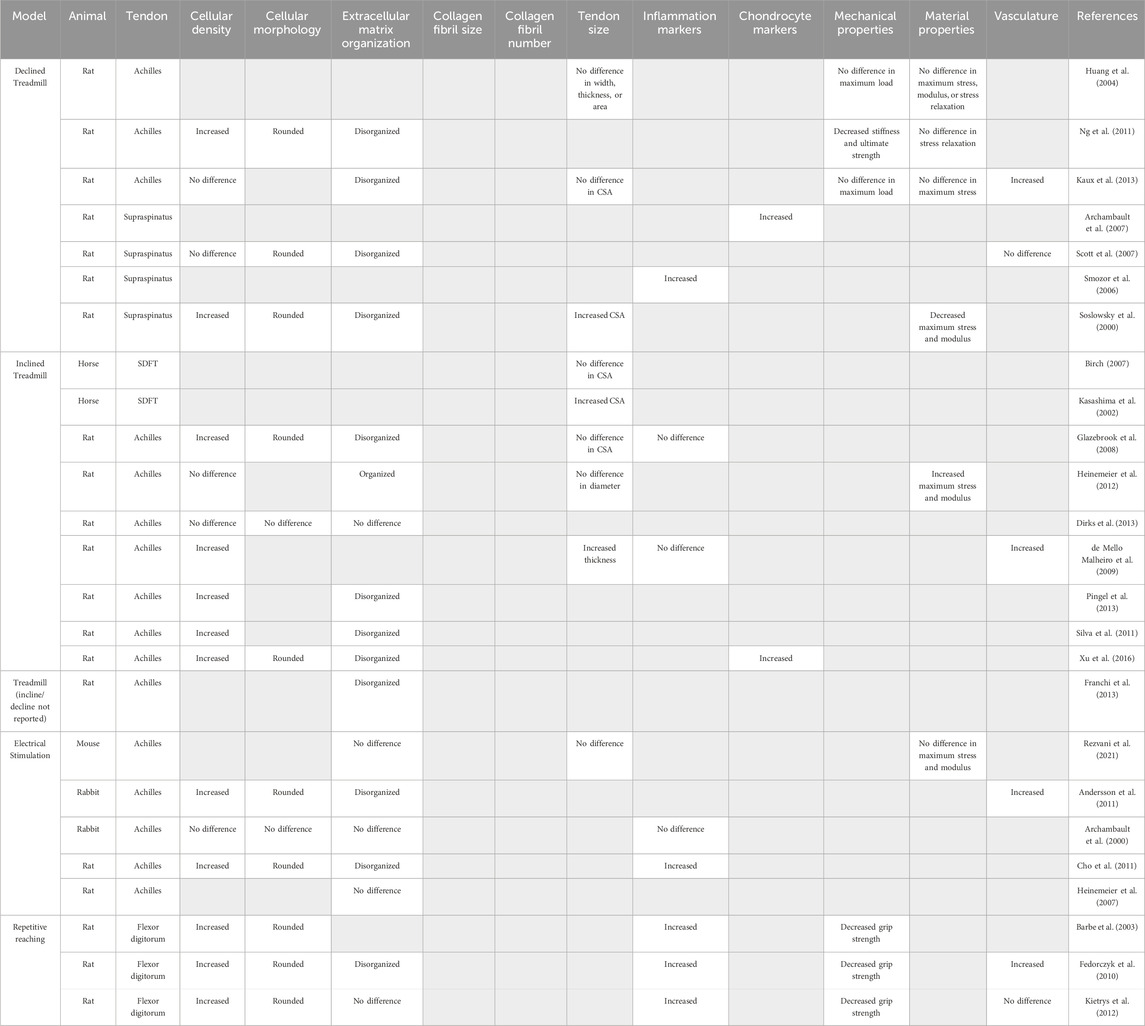
Table 2. Models of tendon overuse and their reported outcomes used to identify hallmarks of tendon overuse. Gray boxes indicate outcome was not reported.
In vivo animal models of tendon overuse
Forced treadmill running
Forced treadmill running is frequently used to mimic overuse-induced tendinopathy seen in human runners, particularly of the Achilles tendon. In rodents, treadmill overuse models report no changes in the CSA of the Achilles tendon with uphill (Glazebrook et al., 2008; Kaux et al., 2013) or downhill running (Huang et al., 2004; Kaux et al., 2013), suggesting no large structural changes as a result of overuse. In rat Achilles tendons, 12 weeks of treadmill running on a 10° incline increased both the elastic modulus (41%) and failure stress (35%) with no changes in collagen fiber alignment or evidence of tearing (Heinemeier et al., 2012), suggesting that uphill running may actually provide positive adaptions rather than cause tissue damage. Indeed, 9 weeks of 15° inclined treadmill running resulted in no changes in cellular density or collagen fiber dispersion in rat Achilles tendons (Dirks et al., 2013). In contrast, other uphill rat running studies examining the Achilles tendon report evidence of tendinopathy indicated by hypercellularity and rounded nuclei (De Mello Malheiro et al., 2009; Silva et al., 2011; Pingel et al., 2013; Xu et al., 2016), increased proteoglycan content (Silva et al., 2011), disorganized collagen matrix (Franchi et al., 2013; Pingel et al., 2013; Xu et al., 2016), microtears (Silva et al., 2011) and elevated expression of chondrocyte markers including SRY-box transcription factor 9 (Sox9), aggrecan (Acan), and bone morphogenic protein-2 (Bmp2) at both the gene and protein level (Xu et al., 2016). Similar to uphill running, one study reported that downhill treadmill running for 1 h/day, 7 days/week for 8 weeks on a 20° decline decreased both stiffness (58%) and max load (53%) which, coupled with rounded nuclei and increased disorganization of the collagen matrix, indicating the development of overuse tendinopathy in rat Achilles tendon (Ng et al., 2011). However, another study employing downhill treadmill training (17 m/min, 10° decline, 1 h/day 5 days/week) reported no changes in mechanical properties or evidence of tendon degeneration in rat Achilles tendons after 16 weeks (Huang et al., 2004), supporting the notion that an ill-defined “threshold” of overuse may be required to trigger downhill induced tendinopathy. Interestingly, treadmill running in horses increased the superficial digital flexor tendon CSA (Kasashima et al., 2002), suggesting a species-specific effect of overuse on tendon hypertrophy. Downhill running employs more eccentric loading and poses greater risk to tendon injury than concentric exercises like uphill running (Friden and Lieber, 1992) and may serve as a better model of over-load induced tendinopathy, though this requires further investigation and refinement.
In addition to the Achilles tendon, many investigators have examined the effects of downhill treadmill running on the supraspinatus tendon. While this model does not recreate human supraspinatus tendon use or loading as humans do not bear weight on their shoulders while running, similar degenerative changes are seen in the supraspinatus tendon of animal after treadmill running as are observed in human cases of supraspinatus tendinopathy (Seitz et al., 2011). Eight weeks of treadmill running at a 10° decline induced a pathological response marked by increased cellularity and cell rounding, disrupted collagen organization, and decreased maximum stress (43%) and elastic modulus (49%) in the rat rotator cuff (Soslowsky et al., 2000), compared to cage activity controls. Other studies reported increased collagen fibril disorganization in Sprague-Dawley rats after 12 weeks (1 km/h, 11° decline, 1 h/day, up to 16 weeks) (Scott et al., 2007), elevated expression of the inflammatory marker gene nitric oxide synthase (Sprague-Dawley rats, 1 km/h, 10° decline, 1 h/day, 5 days/week, 4 weeks) (Szomor et al., 2006) and increased expression of the chondrocyte markers Sox9 and Acan (Sprague-Dawley rats, 1 km/h, 10° decline, 1 h/day, 5 days/week, 4 weeks) (Archambault et al., 2007), which was interpreted as overload-induced fibrocartilage differentiation of tenocytes. Combined, these studies suggest that downhill treadmill running in rodents is sufficient to drive the development of overuse tendinopathy of the supraspinatus tendon.
Direct loading
Direct tendon loading by electric stimulation of attached muscles is a common method used to mechanically stimulate tendons which allows the operator fine control over the frequency and magnitude of the load (Archambault et al., 2000; Heinemeier et al., 2007; Andersson et al., 2011; Cho et al., 2011; Rezvani et al., 2021). Direct stimulation of muscle contraction resulted in increased disorganization of the collagen matrix and increased cellularity in the Achilles tendons of both rats and rabbits (Andersson et al., 2011; Cho et al., 2011). Interestingly, stimulation of isometric, eccentric, and concentric muscle contractions produce different gene expression responses in the rat Achilles tendon. Specifically, eccentric contractions increased mRNA expression of collagen-promoting growth factors including connective tissue growth factor (Ctgf), whereas eccentric and concentric contractions increased mRNA expression of type 3 collagen (Col3a1) indicating different types of muscle loading promote different patterns of gene expression (Heinemeier et al., 2007). However, no changes in collagen organization, CSA, maximum stress, or elastic modulus were seen when comparing eccentric vs. concentric contractions in a mouse Achilles tendon (Rezvani et al., 2021), which suggests that changes in gene expression may not result in large-scale structural changes to the tendon.
Repetitive reaching
To more accurately represent repetitive movement in occupational settings that can lead to overuse tendinopathies, a rat model using voluntary exertions in a repetitive forepaw and wrist-intensive task was developed in which the rats have to reach through a narrow tube to retrieve food pellets (Barr et al., 2000; Barr and Barbe, 2002). Both high repetition, high force and low repetition, negligible force repetitive reaching studies report increased expression of inflammatory markers (e.g., interleukin-Iβ and -1α, cyclooxygenase-2), increased numbers of resident macrophages, in rat flexor tendons compared to contralateral controls (Barr et al., 2000; Barbe et al., 2003; Fedorczyk et al., 2010). Combined, these studies point towards a substantial inflammatory component during repetitive overuse tendinopathy.
Hallmarks of tendon overuse
In vivo animal models of tendon overuse consistently report increases in cell density, which may occur due to increased immune cell recruitment to the tendon given the elevated inflammatory cytokine expression reported in some studies and lack of evidence for tenocyte proliferation in vivo in response to increased inflammation; however, it is important to note that the specific cause of hypercellularity noted in these models is still unknown. As such, deciphering the composition of increased cellular density will be imperative to accurately design and evaluate engineered tendon constructs. Additionally, tenocytes in overloaded tendons are typically rounder and enlarged compared to healthy tenocytes, and coupled with elevated cartilage markers, this suggests the potential that tenocytes become more chondrocyte-like with overuse. In terms of ECM composition and integrity, collagen tearing and disruptions to the organized matrix are commonly reported among overused tendons. Increases in CSA are thought to be a hallmark of an overused tendon but treadmill running models have been unable to replicate this, and other models (direct loading, repetitive reaching, etc.) do not report changes in CSA even in the presence of other tendinopathic hallmarks. Interpreting changes in CSA of engineered constructs should therefore be done in concert with the evaluation of other tendinopathic markers (e.g., changes in cellularity, inflammatory markers, etc.) to delineate whether an increase in CSA is a result of hypertrophy (growth), inflammation (swelling), or fibrotic processes. Moreover, it is likely that the tendon response to load is dynamic over time such that alterations that appear pathological in the beginning may lead to long-term physiological adaptation. For example, it is well known that skeletal muscle adaptation to mechanical load involves an initial inflammatory response that is required to initiate muscle growth (Gomez-Cabrera et al., 2016). How this process is mediated in tendon is unknown, therefore longitudinal studies are needed to determine whether measured inflammatory responses in tissue engineered tendon constructs are indicative of adaptive remodeling or pathological progression.
While attempts to generate healthy tissue engineered replacement tissue should avoid the tendinopathic hallmarks described above, MPS aiming to model overuse tendinopathy should verify that the platform recapitulates pathological changes seen in in vivo studies including ECM disorganization, hypercellularity, tenocyte rounding, and impaired functional properties compared to healthy tendons. Tendon mimetics should also consider alterations in the expression of chondrogenic (Aggrecan, Sox9, etc.) or inflammatory (TNFα, IL-1a, IL-1b, etc.) markers that could signify a tendinopathic response. Similar to underloading, there is a clear difference in how different tendons respond to overuse. It is critical for tissue engineering efforts to take these tendon-specific responses into consideration and to design and evaluate constructs with specific tendons as the comparator.
Discussion
In this review, we have described in vivo models of tendon underloading and overuse and identified hallmarks of tendon pathology that tissue engineering platforms can use to model against in the context of the development of engineered replacement tissues to replace damaged tissue or augment tendon healing, or to incorporate in the context of MPS that seek to mimic specific types of degenerative tendinopathy. As described above, tendinopathy can result from both under and overuse, and while both alterations in mechanical strain led to similar outcomes, the specific mechanisms and hallmarks of each type of tendon degeneration are different. For example, overuse tendon injuries are frequently associated with inflammation, whereas the current models of underloading do not point towards a role for inflammation in underload-induced degeneration. This is a crucial point to consider for both attempts to engineer replacement tissues and for the design of MPS models: in the case of tissue engineered tendon, distinct markers of underuse (smaller collagen fibril area and diameter) or overuse (chondrocyte and inflammatory markers) can be used to fine-tune mechanical stimulation to promote healthy cellular behavior vs. damage whereas MPS should attempt to model specific types of tendinopathy (overuse or underload) in order to have more definable design benchmarks and interpretable outcome measures.
Notably absent from both the current review and the literature at large is a thorough understanding of the cellular and molecular mechanisms that maintain tendon homeostasis in response to physiological load. It is well established that appropriate loading is required to maintain homeostasis (Bohm et al., 2015), and as we have highlighted throughout this review, too much or too little mechanical stimulation can lead to degeneration, making it crucial to identify the “correct” amount of loading to maintain the health of engineered constructs. However, the precise cellular mechanisms that allow tenocytes to translate physiological load into a tissue wide adaptation response is unknown. This lack of information makes evaluating the effectiveness of various loading regimes in the context of tissue engineering extremely difficult, as defining the fine line between “good” load and “bad” load is currently not possible. This is further complicated by the fact that there are clearly tendon-specific and species-specific responses to loading. As such, there is no “universal” threshold for proper mechanical stimulation needed to maintain tendon homeostasis, or that can be prescribed to accurately mimic in vivo tendon behavior in tissue engineered constructs. In the absence of such information, tissue engineering attempts to generate new tendon tissue should aim to recapitulate the native ECM composition, cellular composition and organization, and mechanical properties of specific tendons by using the native tendon as the “gold standard” comparison to evaluate success. For example, tissue engineering studies frequently report an increase in the expression of tenogenic markers within a given system as a “good” outcome; however, these comparisons are typically made in reference to either earlier timepoints in the study or to biomaterials of different composition and not to a specific tendon. As increased expression of tendon markers, including the tenogenic transcription factor scleraxis, can be homeostatic or pathological depending on the context (Kendal et al., 2020; Morita et al., 2021), it is critical for tissue engineers to consider the context and to strive to recreate gene and/or protein expression patterns that are rooted in native tendon tissue rather than changes within an engineered system. Similarly, the effects of loading and tendinopathic progression must be considered in a tendon-specific manner for tissue engineering or MPS efforts that aim to model tendon degeneration. Rather than attempting to broadly mimic tendon degeneration, investigators should focus on a specific tendon (e.g., Achilles or supraspinatus) and type of load-induced pathology (e.g., underload or overuse) in order to have specific, definable points of comparison.
An additional aspect to consider for tissue engineering efforts to generate whole tendon tissues is that there is enormous spatial variation within a tendon at both the cellular and structural scale. For example, the distal portion of the Achilles tendon of long distance runners is significantly larger compared to non-runner controls (Magnusson and Kjaer, 2003), which suggests distinct loading requirements and subsequent responses to loading. Indeed, the distal, midsubstance, and proximal portions of the mouse Achilles tendon display region-specific transcriptomic signatures and mechanical properties, emphasizing the need to consider spatial specific adaptation responses to loading (Disser et al., 2022). Recent single-cell RNA sequencing of tendon cells has also revealed vast heterogeneity among tenocytes, identifying multiple subpopulations during homeostasis and healing both within a given tendon and between different types of tendons (De Micheli et al., 2020; Kendal et al., 2020; Ackerman et al., 2022; Nichols et al., 2023; Mimpen et al., 2024b). Considering that different anatomical tendons experience different types of loads (tensile, compressive, and shear stresses), combined with both spatial and cellular heterogeneity within the tendon, tissue engineering efforts that aim to mimic overuse or underuse tendinopathy should consider how this mechanical, cellular, and matrix heterogeneity may influence the onset of degenerative changes.
Limitations of in vivo animal models of tendon loading
Not all animal models of tendon loading were able to consistently recapitulate the same degenerative changes seen in human cases, some of which can be attributed to inherent limitations of animal models. Many animal models (e.g., forced treadmill running and hindlimb suspension) cause the animals to perform unnatural or difficult tasks, which leads to a systematic stress response that likely influences the tendon mechanoresponse. Degenerative changes seen in these models could therefore be the result of elevated systemic inflammation in concert with loading, rather than solely load-induced.
While animal models provide an attractive framework to manipulate loading induced tendinopathies in a controlled and reproducible manner, many lab animals are quadrupeds and therefore do not experience the same magnitude or type of load as humans (Hast et al., 2014). Furthermore, there are known molecular differences between animals and humans. For instance, rodents do not possess a homologue of the MMP1 human gene (Foley and Kuliopulos, 2014), which complicates our understanding of how MMP1 contributes to ECM remodeling in in vivo models of loading. There are also important differences in the anatomy and mechanical properties of animal tendons compared to human tendons, which are discussed in-depth in a recent review (Burgio et al., 2022). For example, the rat possesses the most similar anatomical structure of the rotator cuff to humans and therefore may serve as a better point of comparison than other rodent models. Another important difference is in the hierarchical structure of tendons. While the tendons of small mammals like mice are relatively simple, consisting of a collagen matrix surrounded by the epitenon, larger mammals have a more complex hierarchical structure that includes the IFM. If and how these other compartments of the tendon may be involved in the response to tendon loading therefore cannot be ascertained using mouse models.
Furthermore, many in vivo animal studies of tendon loading report limited outcome measures, typically focusing on changes in mechanical properties or largely superficial characterizations of cellular, molecular, and ECM changes. As discussed above, this makes interpretation of results difficult, as there is no one singular hallmark of tendon health or degeneration. For example, unloading via Botox increased the elastic modulus of rat Achilles tendons (Khayyeri et al., 2017) which authors interpreted as a degenerative response due to an association with disorganized collagen matrix. In contrast, increased modulus of the Achilles tendon from inclined treadmill trained rats was considered nonpathological; however, no other metrics were reported so this interpretation cannot be fully evaluated (Heinemeier et al., 2012). In order to clarify outcomes and understand the effects of load on tendon health from in vivo animal models as well as in tissue engineering efforts, multiple outcomes must be examined.
Perspectives and future directions
While tissue engineering approaches hold great promise to improve tendon health and healing, there is still substantial work to do to best leverage these techniques and technologies. The vast heterogeneity and disparate outcomes noted in the animal models of tendon loading highlighted in this review speak to an urgent need to better understand specific types of load-induced tendinopathy in anatomically-distinct tendons, as well as the cellular and molecular mechanisms responsible for driving different degenerative tendon pathologies. The past few years have seen an enormous effort dedicated to characterizing the cellular heterogeneity within different tendons using both genetic reporter mice and new technologies including single-cell RNA-sequencing and spatial transcriptomics, in both healthy and diseased tendons across multiple species (Garcia-Melchor et al., 2021; Ackerman et al., 2022; Korcari et al., 2023; Nichols et al., 2023). Tissue engineers can leverage these transcriptomic markers and signatures to identify cell populations that should be present in engineered constructs or mimetics and to ensure that engineered systems promote the desired cellular behavior. Additionally, while most tissue engineering efforts use relatively simple constructs to represent tendon, most frequently with cells embedded in simple hydrogels or biomaterials, tendon is not a simple or homogenous tissue. As mentioned above, there is great spatial heterogeneity not only in the types of cells present but also in the ECM and mechanical loads experienced along the length of a given tendon. Recently, several investigators have characterized these properties in native tendon, which can be directly translated into tissue engineered constructs. For example, a study characterized the transcriptomic and mechanical properties along the length of the rat Achilles tendon, and gave defined CSA, stress, and elastic moduli for three defined regions (proximal, middle, and distal) (Disser et al., 2022). While more work is needed to perform similar characterization in other tendons, and across species, tissue engineering efforts can utilize these metrics to ensure engineered constructs more faithfully recapitulate the properties of native tendons.
Finally, while most tissue engineering efforts to date have focused on generating the collagen matrix of tendons, few have attempted to model the hierarchical structure of native tendons. The components and properties of other compartments within the tendon have been poorly defined historically which has precluded their inclusion in tissue engineered constructs. Recent work to define both the IFM (Thorpe et al., 2016) and the epitenon (Nichols et al., 2023) at both the cellular and molecular level has provided a wealth of markers and metrics that tissue engineers could incorporate into engineered tendon constructs. Because it is difficult to study or image these structures in vivo, it is still unknown how different tendon compartments interact, which represents an excellent opportunity for tissue engineers to create systems in which these relationships can be interrogated. Generation of more complex engineered constructs will not only enhance our understanding of fundamental tendon biology but will also increase the physiological relevance and clinical translatability of tissue engineered tendon.
Conclusion
Despite the significant healthcare burden tendon injuries pose, there are no clinically approved tissue engineering constructs to replace or regenerate degenerative tendons. Current approaches have largely failed to mimic native tendons, in part due to the lack of benchmarks to evaluate the effects of mechanical loading. In this review, we have defined cellular, molecular, structural, and mechanical hallmarks of tendon underloading and overuse from various in vivo animal models of loading as a guide to help inform tissue engineering efforts. Moreover, we urge tissue engineers to consider the tendon-specific requirements for mechanical loading, as well as the cellular, mechanical, and structural complexity of individual tendons. Focusing on the precise cellular and molecular mechanisms in which tendons translate mechanical stimulation to a physiological, rather than pathological, tissue-wide adaptation response will ultimately improve the design of engineered constructs and hopefully help expedite clinical translation.
Author contributions
SM: Writing–original draft, Writing–review and editing. AN: Funding acquisition, Conceptualization, Writing–review and editing.
Funding
The author(s) declare that financial support was received for the research, authorship, and/or publication of this article. This work was supported in part by NIH/NIAMS K99/R00 AR080757 (to AECN).
Conflict of interest
The authors declare that the research was conducted in the absence of any commercial or financial relationships that could be construed as a potential conflict of interest.
Publisher’s note
All claims expressed in this article are solely those of the authors and do not necessarily represent those of their affiliated organizations, or those of the publisher, the editors and the reviewers. Any product that may be evaluated in this article, or claim that may be made by its manufacturer, is not guaranteed or endorsed by the publisher.
References
Ackerman, J. E., Best, K. T., Muscat, S. N., Pritchett, E. M., Nichols, A. E. C., Wu, C. L., et al. (2022). Defining the spatial-molecular map of fibrotic tendon healing and the drivers of Scleraxis-lineage cell fate and function. Cell. Rep. 41 (8), 111706. doi:10.1016/j.celrep.2022.111706
Almeida-Silveira, M. I., Daniel, L., Pérot, C., and Goubel, F. (2000). Changes in stiffness induced by hindlimb suspension in rat Achilles tendon. J. Appl. Phys. 81, 252–257. doi:10.1007/s004210050039
Andersson, G., Forsgren, S., Scott, A., Gaida, J. E., Stjernfeldt, J. E., Lorentzon, R., et al. (2011). Tenocyte hypercellularity and vascular proliferation in a rabbit model of tendinopathy: contralateral effects suggest the involvement of central neuronal mechanisms. Br. J. Sports Med. 45 (5), 399–406. doi:10.1136/bjsm.2009.068122
Archambault, J. M., Hart, D. A., and Herzog, W. (2000). Response of rabbit achilles tendon to chronic repetitive loading. Connect. Tissue Res. 42 (1), 13–23. doi:10.3109/03008200109014245
Archambault, J. M., Jelinsky, S. A., Lake, S. P., Hill, A. A., Glaser, D. L., and Soslowsky, L. J. (2007). Rat supraspinatus tendon expresses cartilage markers with overuse. J. Orthop. Res. 25 (5), 617–624. doi:10.1002/jor.20347
Arruda, E. M., Calve, S., Dennis, R. G., Mundy, K., and Baar, K. (2006). Regional variation of tibialis anterior tendon mechanics is lost following denervation. J. Appl. Physiol. 101 (4), 1113–1117. doi:10.1152/japplphysiol.00612.2005
Arya, S., and Kulig, K. (2010). Tendinopathy alters mechanical and material properties of the Achilles tendon. J. Appl. Physiol. 108 (3), 670–675. doi:10.1152/japplphysiol.00259.2009
Awad, H., Ajalik, R., Alenchery, R., Linares, I., Wright, T., Miller, B., et al. (2023). Human tendon-on-a-chip for modeling vascular inflammatory fibrosis. Res. Sq. doi:10.21203/rs.3.rs-3722255/v1
Bach-Gansmo, F. L., Wittig, N. K., Bruel, A., Thomsen, J. S., and Birkedal, H. (2016). Immobilization and long-term recovery results in large changes in bone structure and strength but no corresponding alterations of osteocyte lacunar properties. Bone 91, 139–147. doi:10.1016/j.bone.2016.07.005
Barbe, M. F., Barr, A. E., Gorzelany, I., Amin, M., Gaughan, J. P., and Safadi, F. F. (2003). Chronic repetitive reaching and grasping results in decreased motor performance and widespread tissue responses in a rat model of MSD. J. Orthop. Res. 21 (1), 167–176. doi:10.1016/S0736-0266(02)00086-4
Barr, A. E., and Barbe, M. F. (2002). Pathophysiological tissue changes associated with repetitive movement: a review of the evidence. Phys. Ther. 82 (2), 173–187. doi:10.1093/ptj/82.2.173
Barr, A. E., Safadi, F. F., Garvin, R. P., Popoff, S. N., and Barbe, M. F. (2000). Evidence of progressive tissue pathophysiology and motor behavior degradation in a rat model of work related musculoskeletal disease. Proc. Hum. Factors Ergon. Soc. Annu. Meet. 44 (30), 5-584–5-587. doi:10.1177/154193120004403051
Bautista, C. A., Srikumar, A., Tichy, E. D., Qian, G., Jiang, X., Qin, L., et al. (2023). CD206+ tendon resident macrophages and their potential crosstalk with fibroblasts and the ECM during tendon growth and maturation. Front. Physiol. 14, 1122348. doi:10.3389/fphys.2023.1122348
Birch, H. L. (2007). Tendon matrix composition and turnover in relation to functional requirements. Int. J. Exp. Pathol. 88 (4), 241–248. doi:10.1111/j.1365-2613.2007.00552.x
Bohm, S., Mersmann, F., and Arampatzis, A. (2015). Human tendon adaptation in response to mechanical loading: a systematic review and meta-analysis of exercise intervention studies on healthy adults. Sports Med. Open 1 (1), 7. doi:10.1186/s40798-015-0009-9
Brent, M. B., Lodberg, A., Thomsen, J. S., and Bruel, A. (2020). Rodent model of disuse-induced bone loss by hind limb injection with botulinum toxin A. MethodsX 7, 101079. doi:10.1016/j.mex.2020.101079
Brin, M. F. (1997). Botulinum toxin: chemistry, pharmacology, toxicity, and immunology. Muscle and Nerve 20 (S6), 146–168. doi:10.1002/(sici)1097-4598(1997)6+<146::aid-mus10>3.0.co;2-4
Burgio, V., Civera, M., Rodriguez Reinoso, M., Pizzolante, E., Prezioso, S., Bertuglia, A., et al. (2022). Mechanical properties of animal tendons: a review and comparative study for the identification of the most suitable human tendon surrogates. Processes 10 (3), 485. doi:10.3390/pr10030485
Chappard, D., Chennebault, A., Moreau, M., Legrand, E., Audran, M., and Basle, M. F. (2001). Texture analysis of X-ray radiographs is a more reliable descriptor of bone loss than mineral content in a rat model of localized disuse induced by the Clostridium botulinum toxin. Bone 28(1), 72–79. doi:10.1016/S8756-3282(00)00438-5
Chatterjee, M., Muljadi, P. M., and Andarawis-Puri, N. (2022). The role of the tendon ECM in mechanotransduction: disruption and repair following overuse. Connect. Tissue Res. 63 (1), 28–42. doi:10.1080/03008207.2021.1925663
Chen, P., Chen, Z., Mitchell, C., Gao, J., Chen, L., Wang, A., et al. (2021). Intramuscular injection of Botox causes tendon atrophy by induction of senescence of tendon-derived stem cells. Stem Cell. Res. Ther. 12 (1), 38. doi:10.1186/s13287-020-02084-w
Cho, N. S., Hwang, J. H., Lee, Y. T., and Chae, S. W. (2011). Tendinosis-like histologic and molecular changes of the Achilles tendon to repetitive stress: a pilot study in rats. Clin. Orthop. Relat. Res. 469 (11), 3172–3180. doi:10.1007/s11999-011-2000-1
Christensen, B., Dyrberg, E., Aagaard, P., Kjaer, M., and Langberg, H. (2008). Short-term immobilization and recovery affect skeletal muscle but not collagen tissue turnover in humans. J. Appl. Physiol. 105 (6), 1845–1851. doi:10.1152/japplphysiol.90445.2008
Couppé, C., Suetta, C., Kongsgaard, M., Justesen, L., Hvid, L. G., Aagaard, P., et al. (2012). The effects of immobilization on the mechanical properties of the patellar tendon in younger and older men. Clin. Biomech. 27 (9), 949–954. doi:10.1016/j.clinbiomech.2012.06.003
Dakin, S. G., Newton, J., Martinez, F. O., Hedley, R., Gwilym, S., Jones, N., et al. (2018). Chronic inflammation is a feature of Achilles tendinopathy and rupture. Br. J. Sports Med. 52 (6), 359–367. doi:10.1136/bjsports-2017-098161
de Boer, M. D., Maganaris, C. N., Seynnes, O. R., Rennie, M. J., and Narici, M. V. (2007a). Time course of muscular, neural and tendinous adaptations to 23 day unilateral lower-limb suspension in young men. J. Physiol. 583 (Pt 3), 1079–1091. doi:10.1113/jphysiol.2007.135392
de Boer, M. D., Selby, A., Atherton, P., Smith, K., Seynnes, O. R., Maganaris, C. N., et al. (2007b). The temporal responses of protein synthesis, gene expression and cell signalling in human quadriceps muscle and patellar tendon to disuse. J. Physiol. 585 (Pt 1), 241–251. doi:10.1113/jphysiol.2007.142828
De Mello Malheiro, O. C., Giacomini, C. T., Justulin, L. A., Delella, F. K., Dal-Pai-Silva, M., and Felisbino, S. L. (2009). Calcaneal tendon regions exhibit different MMP-2 activation after vertical jumping and treadmill running. Anat. Rec. Hob. 292 (10), 1656–1662. doi:10.1002/ar.20953
De Micheli, A. J., Swanson, J. B., Disser, N. P., Martinez, L. M., Walker, N. R., Oliver, D. J., et al. (2020). Single-cell transcriptomic analysis identifies extensive heterogeneity in the cellular composition of mouse Achilles tendons. Am. J. Physiol. Cell. Physiol. 319 (5), C885–c894. doi:10.1152/ajpcell.00372.2020
Deymier, A. C., Schwartz, A. G., Cai, Z., Daulton, T. L., Pasteris, J. D., Genin, G. M., et al. (2019). The multiscale structural and mechanical effects of mouse supraspinatus muscle unloading on the mature enthesis. Acta Biomater 83, 302–313. doi:10.1016/j.actbio.2018.10.024
Dirks, R. C., Richard, J. S., Fearon, A., Scott, A., Koch, L., Britton, S., et al. (2013). Uphill treadmill running does not induce histopathological changes in the rat Achilles tendon. BMC Musculoskelet. Disord. 14 (90), 90. doi:10.1186/1471-2474-14-90
Disser, N. P., Piacentini, A. N., De Micheli, A. J., Schonk, M. M., Yao, V. J. H., Deng, X. H., et al. (2022). Achilles tendons display region-specific transcriptomic signatures associated with distinct mechanical properties. Am. J. Sports Med. 50 (14), 3866–3874. doi:10.1177/03635465221128589
El-Habta, R., Chen, J., Pingel, J., and Backman, L. J. (2018). Tendinosis-like changes in denervated rat Achilles tendon. BMC Musculoskelet. Disord. 19 (1), 426. doi:10.1186/s12891-018-2353-7
Eliasson, P., Fahlgren, A., Pasternak, B., and Aspenberg, P. (2007). Unloaded rat Achilles tendons continue to grow, but lose viscoelasticity. J. Appl. Physiol. 103 (2), 459–463. doi:10.1152/japplphysiol.01333.2006
Fedorczyk, J. M., Barr, A. E., Rani, S., Gao, H. G., Amin, M., Amin, S., et al. (2010). Exposure-dependent increases in IL-1β, substance P, CTGF, and tendinosis in flexor digitorum tendons with upper extremity repetitive strain injury. J. Orthop. Res. 28 (3), 298–307. doi:10.1002/jor.20984
Foley, C. J., and Kuliopulos, A. (2014). Mouse matrix metalloprotease-1a (Mmp1a) gives new insight into MMP function. J. Cell. Physiol. 229 (12), 1875–1880. doi:10.1002/jcp.24650
Franchi, M., Trire, A., Quaranta, M., Orsini, E., and Ottani, V. (2007). Collagen structure of tendon relates to function. ScientificWorldJournal 7, 404–420. doi:10.1100/tsw.2007.92
Franchi, M., Torricelli, P., Giavaresi, G., and Fini, M. (2013). Role of moderate exercising on Achilles tendon collagen crimping patterns and proteoglycans. Connect. Tissue Res. 54 (4-5), 267–274. doi:10.3109/03008207.2013.807808
Fredberg, U., and Stengaard-Pedersen, K. (2008). Chronic tendinopathy tissue pathology, pain mechanisms, and etiology with a special focus on inflammation. Scand. J. Med. Sci. Sports 18 (1), 3–15. doi:10.1111/j.1600-0838.2007.00746.x
Fridén, J., and Lieber, R. (1992). Structural and mechanical basis of exercise-induced muscle injury. Med. Sci. Sports Exerc 24 (2), 521–530. doi:10.1249/00005768-199205000-00005
Garcia-Melchor, E., Cafaro, G., MacDonald, L., Crowe, L. A. N., Sood, S., McLean, M., et al. (2021). Novel self-amplificatory loop between T cells and tenocytes as a driver of chronicity in tendon disease. Ann. Rheum. Dis. 80, 1075–1085. doi:10.1136/annrheumdis-2020-219335
Glazebrook, M. A., Wright, J. R., Langman, M., Stanish, W. D., and Lee, J. M. (2008). Histological analysis of achilles tendons in an overuse rat model. J. Orthop. Res. 26 (6), 840–846. doi:10.1002/jor.20546
Gomez-Cabrera, M. C., Vina, J., and Ji, L. L. (2016). Role of redox signaling and inflammation in skeletal muscle adaptations to training. Antioxidants (Basel) 5 (4), 48. doi:10.3390/antiox5040048
Grimston, S. K., Silva, M. J., and Civitelli, R. (2007). Bone loss after temporarily induced muscle paralysis by Botox is not fully recovered after 12 weeks. Ann. N. Y. Acad. Sci. 1116, 444–460. doi:10.1196/annals.1402.009
Harwood, F. L., and Amiel, D. (1992). Differential metabolic responses of periarticular ligaments and tendon to joint immobilization ligaments and tendon to joint immobilization. J. Appl. Physiol. 72(5), 1687–1691. doi:10.1152/jappl.1992.72.5.1687
Hast, M. W., Zuskov, A., and Soslowsky, L. J. (2014). The role of animal models in tendon research. Bone Jt. Res. 3, 193–202. doi:10.1302/2046-3758.36.2000281
Heinemeier, K. M., Olesen, J. L., Haddad, F., Langberg, H., Kjaer, M., Baldwin, K. M., et al. (2007). Expression of collagen and related growth factors in rat tendon and skeletal muscle in response to specific contraction types. J. Physiol. 582 (Pt 3), 1303–1316. doi:10.1113/jphysiol.2007.127639
Heinemeier, K. M., Skovgaard, D., Bayer, M. L., Qvortrup, K., Kjaer, A., Kjaer, M., et al. (2012). Uphill running improves rat Achilles tendon tissue mechanical properties and alters gene expression without inducing pathological changes. J. Appl. Physiol. 113 (5), 827–836. doi:10.1152/japplphysiol.00401.2012
Helland, C., Bojsen-Moller, J., Raastad, T., Seynnes, O. R., Moltubakk, M. M., Jakobsen, V., et al. (2013). Mechanical properties of the patellar tendon in elite volleyball players with and without patellar tendinopathy. Br. J. Sports Med. 47 (13), 862–868. doi:10.1136/bjsports-2013-092275
Herod, T. W., and Veres, S. P. (2018). Development of overuse tendinopathy: a new descriptive model for the initiation of tendon damage during cyclic loading. J. Orthop. Res. 36 (1), 467–476. doi:10.1002/jor.23629
Huang, T. F., Perry, S., and Soslowsky, L. (2004). The effect of overuse activity on achilles tendon in an animal model: a biomechanical study. Ann. Biomed. Eng. 32 (3), 336–341. doi:10.1023/b:abme.0000017537.26426.76
Irby, A., Gutierrez, J., Chamberlin, C., Thomas, S. J., and Rosen, A. B. (2020). Clinical management of tendinopathy: a systematic review of systematic reviews evaluating the effectiveness of tendinopathy treatments. Scand. J. Med. Sci. Sports 30 (10), 1810–1826. doi:10.1111/sms.13734
Jomaa, G., Kwan, C. K., Fu, S. C., Ling, S. K., Chan, K. M., Yung, P. S., et al. (2020). A systematic review of inflammatory cells and markers in human tendinopathy. BMC Musculoskelet. Disord. 21 (1), 78. doi:10.1186/s12891-020-3094-y
Kannus, P. (2000). Structure of the tendon connective tissue. Scand. J. Med. Sci. Sports 10 (6), 312–320. doi:10.1034/j.1600-0838.2000.010006312.x
Kakade, A. (2023). Prevalence of Achilles Tendinopathy in Building Construction Workers. CHCMJ 12 (02), 99–102. doi:10.24321/2278.2044.202335
Kasashima, Y., Smith, R. K., Birch, H. L., Takahashi, T., Kusano, K., and Goodship, A. E. (2002). Exercise-induced tendon hypertrophy: cross-sectional area changes during growth are influenced by exercise. Equine Vet. J. Suppl. 34 (34), 264–268. doi:10.1111/j.2042-3306.2002.tb05430.x
Katugam, K., Cox, S. M., Salzano, M. Q., De Boef, A., Hast, M. W., Neuberger, T., et al. (2020). Altering the mechanical load environment during growth does not affect adult achilles tendon properties in an avian bipedal model. Front. Bioeng. Biotechnol. 8, 994. doi:10.3389/fbioe.2020.00994
Kaux, J. F., Drion, P., Libertiaux, V., Colige, A., Hoffmann, A., Nusgens, B., et al. (2013). Eccentric training improves tendon biomechanical properties: a rat model. J. Orthop. Res. 31 (1), 119–124. doi:10.1002/jor.22202
Kendal, A. R., Layton, T., Al-Mossawi, H., Appleton, L., Dakin, S., Brown, R., et al. (2020). Multi-omic single cell analysis resolves novel stromal cell populations in healthy and diseased human tendon. Sci. Rep. 10 (1), 13939. doi:10.1038/s41598-020-70786-5
Khayyeri, H., Blomgran, P., Hammerman, M., Turunen, M. J., Lowgren, A., Guizar-Sicairos, M., et al. (2017). Achilles tendon compositional and structural properties are altered after unloading by botox. Sci. Rep. 7 (1), 13067. doi:10.1038/s41598-017-13107-7
Korcari, A., Nichols, A. E. C., Buckley, M. R., and Loiselle, A. E. (2023). Scleraxis-lineage cells are required for tendon homeostasis and their depletion induces an accelerated extracellular matrix aging phenotype. Elife 12, e84194. doi:10.7554/eLife.84194
Kietrys, D. M., Barr-Gillespie, A. E., Amin, M., Wade, C. K., Popoff, S. N., and Barbe, M. F. (2012). Aging contributes to inflammation in upper extremity tendons and declines in forelimb agility in a rat model of upper extremity overuse. PLoS One 7 (10), e46954. doi:10.1371/journal.pone.0046954
Kubo, K., Akima, H., Ushiyama, J., Tabata, I., Fukuoka, H., Kanehisa, H., et al. (2004). Effects of 20 days of bed rest on the viscoelastic properties of tendon structures in lower limb muscles. Br. J. Sports Med. 38 (3), 324–330. doi:10.1136/bjsm.2003.005595
Lake, S. P., Snedeker, J. G., Wang, V. M., Awad, H., Screen, H. R. C., and Thomopoulos, S. (2023). Guidelines for ex vivo mechanical testing of tendon. J. Orthop. Res. 41 (10), 2105–2113. doi:10.1002/jor.25647
Lian, O., Dahl, J., Ackermann, P. W., Frihagen, F., Engebretsen, L., and Bahr, R. (2006). Pronociceptive and antinociceptive neuromediators in patellar tendinopathy. Am. J. Sports Med. 34 (11), 1801–1808. doi:10.1177/0363546506289169
Lim, W. L., Liau, L. L., Ng, M. H., Chowdhury, S. R., and Law, J. X. (2019). Current progress in tendon and ligament tissue engineering. Tissue Eng. Regen. Med. 16 (6), 549–571. doi:10.1007/s13770-019-00196-w
Loitz, B. J., Zernicke, R. F., Vailas, A. C., Kody, M. H., and Meals, R. A. (1989). Effects of short-term immobilization versus continuous passive motion on the biomechanical and biochemical properties of the rabbit tendon. Clin. Orthop. Relat. Res. 244, 265–271. doi:10.1097/00003086-198907000-00029
Lomas, A. J., Ryan, C. N., Sorushanova, A., Shologu, N., Sideri, A. I., Tsioli, V., et al. (2015). The past, present and future in scaffold-based tendon treatments. Adv. Drug Deliv. Rev. 84, 257–277. doi:10.1016/j.addr.2014.11.022
Maganaris, C. N., Reeves, N. D., Rittweger, J., Sargeant, A. J., Jones, D. A., Gerrits, K., et al. (2006). Adaptive response of human tendon to paralysis. Muscle Nerve 33 (1), 85–92. doi:10.1002/mus.20441
Magnusson, S. P., and Kjaer, M. (2003). Region-specific differences in Achilles tendon cross-sectional area in runners and non-runners. Eur. J. Appl. Physiol. 90 (5-6), 549–553. doi:10.1007/s00421-003-0865-8
Manzanares, G., Brito-da-Silva, G., and Gandra, P. G. (2018). Voluntary wheel running: patterns and physiological effects in mice. Braz J. Med. Biol. Res. 52 (1), e7830. doi:10.1590/1414-431X20187830
Marsolais, D., Cote, C. H., and Frenette, J. (2001). Neutrophils and macrophages accumulate sequentially following Achilles tendon injury. J. Orthop. Res. 19 (6), 1203–1209. doi:10.1016/S0736-0266(01)00031-6
Matsumoto, F., Trudel, G., Uhthoff, H. K., and Backman, D. S. (2003). Mechanical effects of immobilization on the Achilles' tendon. Arch. Phys. Med. Rehabil. 84 (5), 662–667. doi:10.1016/s0003-9993(02)04834-7
Meijer, J. H., and Robbers, Y. (2014). Wheel running in the wild. Proc. Biol. Sci. 281 (1786), 20140210. doi:10.1098/rspb.2014.0210
Meli, V. S., Atcha, H., Veerasubramanian, P. K., Nagalla, R. R., Luu, T. U., Chen, E. Y., et al. (2020). YAP-mediated mechanotransduction tunes the macrophage inflammatory response. Sci. Adv. 6(49), eabb8471. doi:10.1126/sciadv.abb8471
Millar, N. L., Murrell, G. A., and McInnes, I. B. (2017). Inflammatory mechanisms in tendinopathy - towards translation. Nat. Rev. Rheumatol. 13 (2), 110–122. doi:10.1038/nrrheum.2016.213
Millar, N. L., Silbernagel, K. G., Thorborg, K., Kirwan, P. D., Galatz, L. M., Abrams, G. D., et al. (2021). Tendinopathy. Nat. Rev. Dis. Prim. 7 (1), 1. doi:10.1038/s41572-020-00234-1
Mimpen, J. Y., Baldwin, M. J., Paul, C., Ramos-Mucci, L., Kurjan, A., Cohen, C. J., et al. (2024a). Exploring cellular changes in ruptured human quadriceps tendons at single-cell resolution. bioRxiv. doi:10.1101/2024.09.06.611599
Mimpen, J. Y., Ramos-Mucci, L., Paul, C., Kurjan, A., Hulley, P. A., Ikwuanusi, C. T., et al. (2024b). Single nucleus and spatial transcriptomic profiling of healthy human hamstring tendon. FASEB J. 38 (10), e23629. doi:10.1096/fj.202300601RRR
Morey, E. (1979). Spaceflight and bone turnover: correlation with a new rat model of weightlessness. BioScience 29(3), 168–172. doi:10.2307/1307797
Morey-Holton, E. R., and Globus, R. K. (1998). Hindlimb unloading of growing rats: a model for predicting skeletal changes during space flight. Bone 22 (5), 83S–88S. doi:10.1016/S8756-3282(98)00019-2
Morita, W., Snelling, S. J. B., Wheway, K., Watkins, B., Appleton, L., Murphy, R. J., et al. (2021). Comparison of cellular responses to TGF-β1 and BMP-2 between healthy and torn tendons. Am. J. Sports Med. 49 (7), 1892–1903. doi:10.1177/03635465211011158
Muscat, S., Nichols, A. E. C., Gira, E., and Loiselle, A. E. (2022). CCR2 is expressed by tendon resident macrophage and T cells, while CCR2 deficiency impairs tendon healing via blunted involvement of tendon-resident and circulating monocytes/macrophages. FASEB J. 36 (11), e22607. doi:10.1096/fj.202201162R
Nakagawa, Y., Totsuka, M., Sato, T., Fukuda, Y., and Hirota, K. (1989). Effect of disuse on the ultrastructure of the achilles tendon in rats. Eur. J. Appl. Physiol. 59, 239–242. doi:10.1007/BF02386194
Ng, G. Y., Chung, P. Y., Wang, J. S., and Cheung, R. T. (2011). Enforced bipedal downhill running induces Achilles tendinosis in rats. Connect. Tissue Res. 52 (6), 466–471. doi:10.3109/03008207.2011.562334
Nichols, A. E. C., Wagner, N. W., Ketonis, C., and Loiselle, A. E. (2023). Epitenon-derived cells comprise a distinct progenitor population that contributes to both tendon fibrosis and regeneration following acute injury. bioRxiv, 2023, 526242. doi:10.1101/2023.01.30.526242
Ning, C., Li, P., Gao, C., Fu, L., Liao, Z., Tian, G., et al. (2023). Recent advances in tendon tissue engineering strategy. Front. Bioeng. Biotechnol. 11, 1115312. doi:10.3389/fbioe.2023.1115312
Patel, D., Zamboulis, D. E., Spiesz, E. M., Birch, H. L., Clegg, P. D., Thorpe, C. T., et al. (2021). Structure-function specialisation of the interfascicular matrix in the human achilles tendon. Acta Biomater. 131, 381–390. doi:10.1016/j.actbio.2021.07.019
Pingel, J., Wienecke, J., Kongsgaard, M., Behzad, H., Abraham, T., Langberg, H., et al. (2013). Increased mast cell numbers in a calcaneal tendon overuse model. Scand. J. Med. Sci. Sports 23 (6), e353–e360. doi:10.1111/sms.12089
Reeves, N. D., Maganaris, C. N., Ferretti, G., and Narici, M. V. (2005). Influence of 90-day simulated microgravity on human tendon mechanical properties and the effect of resistive countermeasures. J. Appl. Physiol. 98 (6), 2278–2286. doi:10.1152/japplphysiol.01266.2004
Rezvani, S. N., Nichols, A. E. C., Grange, R. W., Dahlgren, L. A., Brolinson, P. G., and Wang, V. M. (2021). A novel murine muscle loading model to investigate Achilles musculotendinous adaptation. J. Appl. Physiol. 130 (4), 1043–1051. doi:10.1152/japplphysiol.00638.2020
Ruiz-Alonso, S., Lafuente-Merchan, M., Ciriza, J., Saenz-Del-Burgo, L., and Pedraz, J. L. (2021). Tendon tissue engineering: cells, growth factors, scaffolds and production techniques. J. Control Release 333, 448–486. doi:10.1016/j.jconrel.2021.03.040
Schwartz, A. G., Lipner, J. H., Pasteris, J. D., Genin, G. M., and Thomopoulos, S. (2013). Muscle loading is necessary for the formation of a functional tendon enthesis. Bone 55 (1), 44–51. doi:10.1016/j.bone.2013.03.010
Scott, A., Cook, J. L., Hart, D. A., Walker, D. C., Duronio, V., and Khan, K. (2007). Tenocyte responses to mechanical loading in vivo: a role for local insulin-like growth factor 1 signaling in early tendinosis in rats. Arthritis Rheum. 56 (3), 871–881. doi:10.1002/art.22426
Screen, H. R. C., Berk, D. E., Kadler, K. E., Ramirez, F., and Young, M. F. (2015). Tendon functional extracellular matrix. J. Orthop. Res. 33 (6), 793–799. doi:10.1002/jor.22818
Seitz, A. L., McClure, P. W., Finucane, S., Boardman, N. D., and Michener, L. A. (2011). Mechanisms of rotator cuff tendinopathy: intrinsic, extrinsic, or both? Clin. Biomech. (Bristol, Avon) 26 (1), 1–12. doi:10.1016/j.clinbiomech.2010.08.001
Silva, R. D., Glazebrook, M. A., Campos, V. C., and Vasconcelos, A. C. (2011). Achilles tendinosis - a morphometrical study in a rat model. Int. J. Clin. Exp. Pathol. 4 (7), 683–691.
Soslowsky, L. J., Thomopoulos, S., Tun, S., Flanagan, C. L., Keefer, C. C., Mastaw, J., et al. (2000). Neer Award 1999: overuse activity injures the supraspinatus tendon in an animal model: a histologic and biomechanical study. J. Shoulder Elb. Surg. 9 (2), 79–84. doi:10.1067/mse.2000.101962
Steinmann, S., Pfeifer, C. G., Brochhausen, C., and Docheva, D. (2020). Spectrum of tendon pathologies: triggers, trails and end-state. Int. J. Mol. Sci. 21 (3), 844. doi:10.3390/ijms21030844
Subramanian, A., Kanzaki, L. F., Galloway, J. L., and Schilling, T. F. (2018). Mechanical force regulates tendon extracellular matrix organization and tenocyte morphogenesis through TGFbeta signaling. Elife 7, e38069. doi:10.7554/eLife.38069
Swank, K. R., Furness, J. E., Baker, E., Gehrke, C. K., and Rohde, R. (2022). A Survey of Musculoskeletal Disorders in the Orthopaedic Surgeon: Identifying Injuries, Exacerbating Workplace Factors, and Treatment Patterns in the Orthopaedic Community. J. Am. Acad. Orthop. Surg. Glob. Res. Rev. 6 (5). doi:10.5435/JAAOSGlobal-D-20-00244
Szomor, Z. L., Appleyard, R. C., and Murrell, G. A. (2006). Overexpression of nitric oxide synthases in tendon overuse. J. Orthop. Res. 24 (1), 80–86. doi:10.1002/jor.20009
Thorpe, C. T., Birch, H. L., Clegg, P. D., and Screen, H. R. (2013). The role of the non-collagenous matrix in tendon function. Int. J. Exp. Pathol. 94 (4), 248–259. doi:10.1111/iep.12027
Thorpe, C. T., Peffers, M. J., Simpson, D., Halliwell, E., Screen, H. R., and Clegg, P. D. (2016). Anatomical heterogeneity of tendon: fascicular and interfascicular tendon compartments have distinct proteomic composition. Sci. Rep. 6, 20455. doi:10.1038/srep20455
Vailas, A., Deluna, D., Lewis, L., Curwin, S., Roland, R., and Alford, E. (1988). Adaptation of bone and tendon to prolonged hindlimb suspension in rats. J. Appl. Physiol. 65(1), 373–376. doi:10.1152/jappl.1988.65.1.373
Vilarta, R., and Vidal Bde, C. (1989). Anisotropic and biomechanical properties of tendons modified by exercise and denervation: aggregation and macromolecular order in collagen bundles. Matrix 9 (1), 55–61. doi:10.1016/s0934-8832(89)80019-8
Wang, K., Man, K., Liu, J., Liu, Y., Chen, Q., Zhou, Y., et al. (2020). Microphysiological systems: design, fabrication, and applications. ACS Biomater. Sci. Eng. 6 (6), 3231–3257. doi:10.1021/acsbiomaterials.9b01667
Warner, S. E., Sanford, D. A., Becker, B. A., Bain, S. D., Srinivasan, S., and Gross, T. S. (2006). Botox induced muscle paralysis rapidly degrades bone. Bone 38 (2), 257–264. doi:10.1016/j.bone.2005.08.009
Xu, S. Y., Li, S. F., and Ni, G. X. (2016). Strenuous treadmill running induces a chondrocyte phenotype in rat achilles tendons. Med. Sci. Monit. 22, 3705–3712. doi:10.12659/MSM.897726
Keywords: tendon, loading, tissue engineering, adaptation, disuse, underloading, overuse
Citation: Muscat S and Nichols AEC (2024) Leveraging in vivo animal models of tendon loading to inform tissue engineering approaches. Front. Bioeng. Biotechnol. 12:1449372. doi: 10.3389/fbioe.2024.1449372
Received: 14 June 2024; Accepted: 24 September 2024;
Published: 07 October 2024.
Edited by:
Florien Jenner, University of Veterinary Medicine Vienna, AustriaReviewed by:
Mohammad El Khatib, University of Teramo, ItalyYoung Hoon Son, Emory University, United States
Copyright © 2024 Muscat and Nichols. This is an open-access article distributed under the terms of the Creative Commons Attribution License (CC BY). The use, distribution or reproduction in other forums is permitted, provided the original author(s) and the copyright owner(s) are credited and that the original publication in this journal is cited, in accordance with accepted academic practice. No use, distribution or reproduction is permitted which does not comply with these terms.
*Correspondence: Anne E. C. Nichols, YW5uZV9uaWNob2xzQHVybWMucm9jaGVzdGVyLmVkdQ==