- 1Department of Chemical, Pharmaceutical and Agricultural Sciences, University of Ferrara, Ferrara, Italy
- 2Department of Environmental and Prevention Sciences, University of Ferrara, Ferrara, Italy
- 3Faculty of Earth Sciences, Dep. Ciencia de La Tierra, Universidad Estatal Amazónica, Puyo, Ecuador
- 4Department of Life Sciences and Biotechnology, University of Ferrara, Ferrara, Italy
Introduction: Lactic acid (LA) production from fossil resources is unsustainable owing to their depletion and environmental concerns. Thus, this study aimed to optimize the production of LA by Lactobacillus casei in a cultured medium containing fruit wastes (FWs) from agro-industries and second cheese whey (SCW) from dairy production, supplemented with maize steep liquor (MSL, 10% v/v) as the nitrogen source.
Methods: The FWs were selected based on seasonal availability [early summer (early ripening peach), full summer (melon), late summer (pear), and early autumn (apple)] and SCW as annual waste. Small-scale preliminary tests as well as controlled fermenter experiments were performed to demonstrate the potential of using various food wastes as substrates for LA fermentation, except for apple pomace.
Results and discussion: A 5-cycle repeated batch fermentation was conducted to optimize waste utilization and production, resulting in a total of 180.56 g/L of LA with a volumetric productivity of 0.88 g/L∙h. Subsequently, mechanical filtration and enzymatic hydrolysis were attempted. The total amount of LA produced in the 5-cycle repeated batch process was 397.1 g/L over 288 h, achieving a volumetric productivity of 1.32 g/L∙h. These findings suggest a promising biorefinery process for low-cost LA production from agri-food wastes.
1 Introduction
Lactic acid (LA) is a well-established and one of the most widely recognized organic acids (Thygesen et al., 2021). Its actual worldwide production of approximately 1.5 million metric tons is anticipated to witness a compounded annual growth rate (CAGR) of 8.2% by 2030 (Ojo and de Smidt, 2023). The global market for LA is rapidly expanding, fueled by food applications as a preservative (Aguirre-Garcia et al., 2024), production of polylactic acid (PLA) as a biodegradable and compostable thermoplastic (Ahmad et al., 2024), and pharmaceutical and personal care applications owing to its antibacterial and detergent properties (Ruiz-Ruiz et al., 2017). Because LA has both carboxylic and hydroxyl functional groups, it can also be considered as a platform and an intermediate for transformation into several different useful and valuable chemicals (Gao et al., 2011).
LA is one of the large-scale compounds for which the biotechnological production has almost completely prevailed through the petrochemical route, with about 90% of the current production achieved by microbial fermentation (Macedo et al., 2020). The fermentative production of LA has been extensively studied for years using a broad range of microorganisms and different types of substrates to optimize yield and productivity (Tian et al., 2021).
The most well-known wild-type LA producers are lactic acid bacteria (LAB), which are non-spore-forming, Gram positive, non-aerobic or aerotolerant, acid tolerant, and strictly fermentative organisms (Fidan et al., 2022). Among the LAB, Lactobacillus is the genus of greatest commercial interest as it is homofermentative and produces LA primarily through the Embden–Meyerhoff–Parnas (EMP) pathway by converting one molecule of glucose into two molecules of LA (Singhvi et al., 2018). Recombinant strains of Escherichia coli, Bacillus coagulans, Corynebacterium glutamicum, Bacillus licheniformis, and metabolically engineered yeasts have also been evaluated for LA production (Awasthi et al., 2018).
Although industrial-scale biotechnological LA production has been long established, there is room for further process improvements (Abedin et al., 2023). The main obstacle to the use of LAB is their complex nutrient requirement and mesophilia, which lead to increased costs and contamination risks, respectively (Abedi and Hashemi, 2020). Regarding carbon substrates, several agro-industrial low- or no-value wastes, such as molasses, juices wastes, and starchy biomass dairy wastes, have been traditionally fermented into LA (Alexandri et al., 2019; Sakr et al., 2021). More recently, agricultural and forestry residues have also been proposed as carbon sources (Ajala et al., 2020; Yankov, 2022). However, the high costs of the raw materials and fermentation–separation processes as well as selection of highly efficient LA producing microorganisms have severely limited such applications (Ren et al., 2022).
Considerable endeavors have been dedicated to developing fermentation strategies, such as consolidated bioprocesses (CBPs), simultaneous saccharification and fermentation (SSF), and simultaneous saccharification and co-fermentation (SSCF), as promising alternatives (Mazzoli, 2021). To this end, two main concepts have been implemented, i.e., development of synthetic microbial consortia based on co-cultures (Sun et al., 2021) and genetically engineered microorganisms (Levit et al., 2022). In contrast to pure cultures, microbial consortia have been demonstrated to be less vulnerable to environmental disturbances and contamination while exhibiting higher conversion efficiencies (Sun et al., 2019). Nevertheless, the development of reliable methods for co-cultures, growth dynamics, monitoring, and control is still challenging owing to the complex interactions among the microbial population (Mittermeier et al., 2023). Metabolic engineering aims to develop single strains with efficient product formation, yet substantial efforts are needed for major genetic and metabolic redesigning of microorganisms (Hossain et al., 2023).
The second bottleneck for LA production is the overall process cost (Marchesan et al., 2021) from feedstock treatments and sterilization, which are necessary to avoid contamination unless when using thermophilic strains (Garita-Cambronero et al., 2021), to downstream LA production and purification through fermentation. Relative to fermentation, carbon sources are a challenge in terms of cost efficiency as they account for up to 70% of the overall costs (Rawoof et al., 2021). Although refined sugars can theoretically be used as feedstock, with unavoidably lower purification costs as the main advantage, their large-scale industrial use is not economically feasible (Costa et al., 2021). Therefore, wastes and byproducts are preferred because they are inexpensive, abundant, and renewable. Moreover, their use contributes to efficient waste management and reduced environmental pollution in the wider perspective of a circular economy (Walzberg et al., 2021). The downstream processes from the fermentation broth are similarly pivotal to the overall economic sustainability and can account for about 50% of the operational costs (Kumar et al., 2020).
Another limitation to the widespread valorization of byproducts and wastes is related to logistic issues, namely collection and transportation (Caldeira et al., 2020). In fact, one of the intrinsic causes for concern is the environmental impact of transportation over long distances from the waste production to processing site (Read et al., 2020). At the moment, the sidestream residues are suitable raw materials only for small- and medium-scale upgrading or in situ valorization in food processing facilities (Vigneshwar et al., 2022). It has been recently estimated that about 30% of global food production is discarded and lost, with 13% lost during the production supply chain and about 17% lost at the consumer level (Ortiz-Sanchez et al., 2023).
Fruit processing industries generate large amounts of wastes, i.e., inedible parts of fruits, damaged or rotten fruit, peel, seeds, pomace, pulp, rinds, and empty fruit bunches, which may account for 20%–80% of the entire processed fruit (Kandemir et al., 2022; Suri et al., 2022). While these are already being employed as animal feed, a major proportion is now destined to be composted or used for biogas production despite more valuable forms of valorization (Ganesh et al., 2022). Some studies have already been published on LA fermentation from fruit wastes (FWs), like using mango peels (Jawad et al., 2013), orange peels (Bustamante et al., 2019), ficus indica wastes (Derabli et al., 2022), tropical fruit byproducts (Ngouénam et al., 2021), and date pulp wastes (Ahmad et al., 2024), as substrates. Whey is the principal residue from the dairy industry (Tsermoula et al., 2021) and consists of cheese whey, which is the byproduct of cheese manufacturing, and second cheese whey (SCW), which is the liquid remaining after further processing of whey cheese that represents more than 90% of the original whey (Pires et al., 2021). Owing to their high organic load and water content, both components can cause severe environmental concerns if not appropriately treated (Poništ et al., 2021). Although several studies have been conducted on cheese whey valorization and a wide range of cheese-whey-derived products are available in the market, SCW has been insufficiently investigated as a substrate for LA fermentation (Sommella et al., 2016). Cheese whey has been earlier proposed as substrate for LA fermentation (Sayed et al., 2020; Zotta et al., 2020; Catone et al., 2021), in addition to biohydrogen (Ordoñez-Araque et al., 2022; Ribeiro et al., 2022) and biofuel production (Osorio-González et al., 2022; Kumar et al., 2023). The use of SCW as a substrate has been attempted for bioethanol (Sansonetti et al., 2009) and biodiesel (Carota et al., 2017) productions; further, it has been used as a substrate for lactobionic acid fermentation using Pseudomonas taetrolens (De Giorgi et al., 2018), PHA polymers (Bosco et al., 2021), as an additive in the production of artisanal beers (Pasta et al., 2023), and as a substrate for LAB fermentation for use as probiotics and starters (Secchi et al., 2012). The only example of ovine SCW bioconversion to LA was reported by Secchi et al. (2012), whereas bovine SCW has been used as a substrate to produce LA by Costa et al. (2021) and Dedenaro et al. (2016).
The aim of the present work was to establish LA fermentation by Lactobacillus casei using a culture medium based on FWs and SCW as the carbon substrates with maize steep liquor (MSL) as the nitrogen source. MSL is a byproduct of the wet grinding process of maize and is commonly used as a cheap source of nitrogen, amino acids, vitamins, and minerals in fermentation processes (Zhou et al., 2022; Wahjudi et al., 2023). Based on seasonal availability, potential subsequent FW combinations were also investigated. Thus, five main groups were designated in this study, namely, annual (SCW), early summer (early ripening peach), full summer (melon), late summer (pear), and early autumn (apple), to promote full exploitation of the available wastes. Emilia Romagna (Northeast Italy) was identified as the representative region for the present case study given its agri-food production and processing industries.
2 Materials and methods
2.1 Microorganism and inoculum
The homofermentative L. casei (DSMZ 20011) was purchased from the Leibniz Institute DSMZ GmbH (Braunschweig, Germany) and used in this study. The master cell bank was maintained at −20°C in a standard semisynthetic De Man, Rogosa and Sharpe (MRS) medium (1 mL) mixed with glycerol (0.5 mL) as a cryoprotective agent. The standard MRS medium (Fluka Analytical) contained 20 g/L of glucose, 10 g/L of bacteriological peptone, 8 g/L of meat extract, 4 g/L of yeast extract, 5 g/L of CH3COONa·3H2O, 2 g/L of K2HPO4, 2 g/L of ammonium citrate tribasic, 0.2 g/L of MgSO4·7H2O, and 0.05 g/L of MnSO4·4H2O. The working cell bank was preserved at 4°C in MRS-agar slants for 6 months and used for the seed cultures. All chemicals were purchased from Merck KGaA (Darmstadt, Germany) unless otherwise stated. For the inoculum preparation, L. casei was cultured at 30°C and pH = 6.3 for 24 h in sterile MRS medium.
2.2 Substrates: SCW and fruit pomace
Fruit pomace (apple, pear, melon, and peach) samples were provided by Conserve Italia (San Lazzaro, Bologna, Italy) based on seasonal availability: early ripening peach (group early summer), melon (group full summer), pear (group late summer), and apple (early autumn); the fruits were harvested and processed in June, July, August–September, and October, respectively. Single raw materials were mixed using a food blender to obtain a thick puree before rough filtration to eliminate the coarser fibrous components as well as skin residues to avoid clogging of the sample pipeline in the fermenter. SCW and MSL were supplied by Granarolo Spa (Bologna, Italy) and Cargill Spa (Calstelmassa, Rovigo, Italy), respectively. MSL supplement was used as an alternative to yeast extract or peptone as the nitrogen source. All materials were stored in plastic tanks at −18°C for laboratory analyses and use as fermentation substrates.
2.3 Apple pomace filtration and hydrolysis
Apple pomace was subjected to two alternative pretreatments: filtration and enzymatic hydrolysis. Filtration was performed with a mechanical filter with a 5-mm-sieve via gravity at 4°C; approximately 300 mL of apple filtrate was recovered and analyzed for the sugar content. Enzymatic hydrolysis was performed using a commercial enzyme mixture (Celluclast™, Novozymes) containing cellulase from Trichoderma reesei and β-glucosidase from Aspergillus niger. Assays were conducted in 250 mL Erlenmeyer flasks at 50°C under agitation (180 rpm) for 12 h, with the addition of 0.05 M citrate buffer (pH = 4.8). Then, approximately 2 mL of the enzyme suspension at a declared activity of 700 endoglucanase units (EGU)/g was added to 150 mL of the apple pomace sample (Zhang et al., 2017); the apple pomace hydrolysate was then analyzed for its sugar content.
2.4 Single substrate fermentation
Single substrate fermentations were performed for preliminary assessments of the fermentative capacities of L. casei on the selected carbon sources to define the baseline for further process development and to study the feasibility of sequentially using the various feedstocks. The fermentations were carried out in 100 mL Erlenmeyer flasks with 100 mL working volume. Approximately 100 mL of the various substrates, i.e., SCW, pear pomace, peach pomace, apple pomace, and melon wastes, were added to 10 mL of MSL and inoculated with 10% (v/v) of an exponentially growing inoculum of L. casei at 30°C and pH = 6.3 for 96 h at a stirring rate of 100 rpm on a rotary shaker for growth as single carbon sources. The samples were withdrawn at 0, 24, 48, 72, and 96 h, before being filtered and stored at 4°C until the analyses. All experiments were carried out in triplicate, and the standard deviation is reported as the measure of errors.
2.5 Sequential repeated batch fermentation
Repeated batch fermentation processes were carried out by considering five batch cycles (Figure 1), each using a different FW depending on seasonal availability, with SCW as the substrate available throughout the year and MSL as the nitrogen source. A thermoregulated autoclavable MiniforsTM bioreactor (Infors, Basel, Switzerland) (1 L working volume, 1.5 L overall capacity) equipped with probes for the pH, temperature, and dissolved oxygen (Mettler Toledo, Columbus, United States) was used. The pH values of the cultures were automatically maintained at 6.3 by the addition of 1 N NaOH solution.
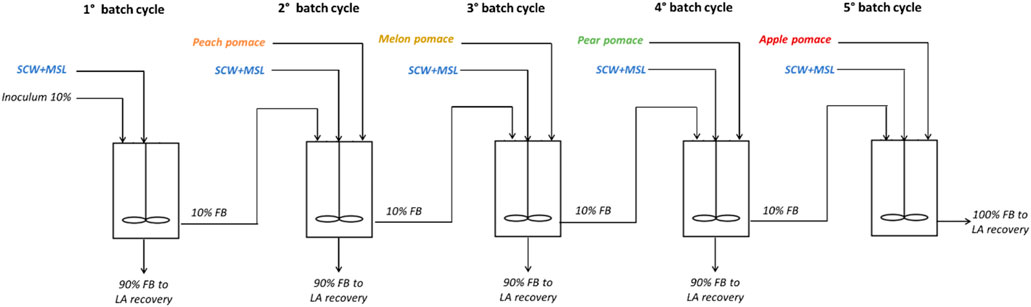
Figure 1. - Experimental scheme of the sequential repeated batch fermentation process based on seasonally available FWs, including SCW as an annual available waste and MSL as the nitrogen source (FB, fermentation broth).
Before inoculation, the fermenter was filled with a mixture of SCW and 10% MSL along with the various FWs at appropriate percentages depending on the cycles. The culture was stirred at 50 rpm with a mechanical stirrer. An airflow of 0.5 L/min was fluxed on the head space of the fermenter to preserve a slight overpressure. Each cycle was followed for 48 h and samples were retrieved at 0, 6, 12, 24, 36, and 48 h. About 90% of the working volume was discharged at the end of each cycle, while 10% of the fermentation broth from each cycle was retained in the fermenter as inoculum for the following cycle. The following ratio of substrates was added to the SCW:
- 1st batch: SCW-MSL 100% + FW 0%
- 2nd batch: SCW-MSL 30% + FW (peach pomace) 70%
- 3rd batch: SCW-MSL 30% + FW (melon pomace) 70%
- 4th batch: SCW-MSL 30% + FW (pear pomace) 70%
- 5th batch: SCW-MSL 30% + FW (apple pomace) 70%
2.6 Analytical assays
Sugars (glucose, fructose, sucrose, and lactose) were analyzed by high-performance liquid chromatography (HPLC; Jasco, Easton, MD, United States) equipped with refractive index and UV detectors (Jasco, Oklahoma City, OK, United States) as well as Rezex ROA-Organic Acid H+ (8%), 300 × 7.8 mm (Phenomenex, Torrance, CA, United States). Isocratic elution was conducted at 30°C with 0.6 mL/min of 0.01 M H2SO4. Before the HPLC analyses, the samples were centrifuged (6720 RCF; 10 min), maintained at 80°C for 10 min to eliminate possible interferences due to the microbial enzymes, and filtered using cellulose acetate filters (porosity of 0.2 µm). The overall yield of LA was calculated as the gram-produced LA per gram of available total sugars and gram per consumed total sugars. The maximum production rate was calculated as the mass of the maximum LA production per volume of the fermentation broth in time units (hours).
3 Results
3.1 Sugar contents of the substrates
The sugar contents of the different substrates used in this study are reported in Table 1 and Figure 2. The total sugar content of the FWs were 29.3 ± 1.21, 28.7 ± 0.87, and 58.2 ± 4.4 g/L for the melon, pear, and apple pomace, respectively, and 40.4 g/L for the peach pomace. The SCW sugar content was 46 g/L, and this amount was almost entirely due to lactose. The differences in the sugar contents of the FWs are attributable to the different compositions of pomace; peach pomace is principally composed of rotten fruit pulp without the peach pits and skins, which are usually removed by chemical treatment of the whole fruit before processing in the industry, except for fresh peaches destined for fresh consumption. Conversely, melon, pear, and apple pomace samples included the skins, kernels, stalks (for apple and pear), and thick peel (for melon), as well as all fibrous parts that decreased the fermentable sugar contents. The chemical composition of MSL was provided by the manufacturer (Table 2; Figure 3), and it was analyzed here for determination of the sugar and LA contents.
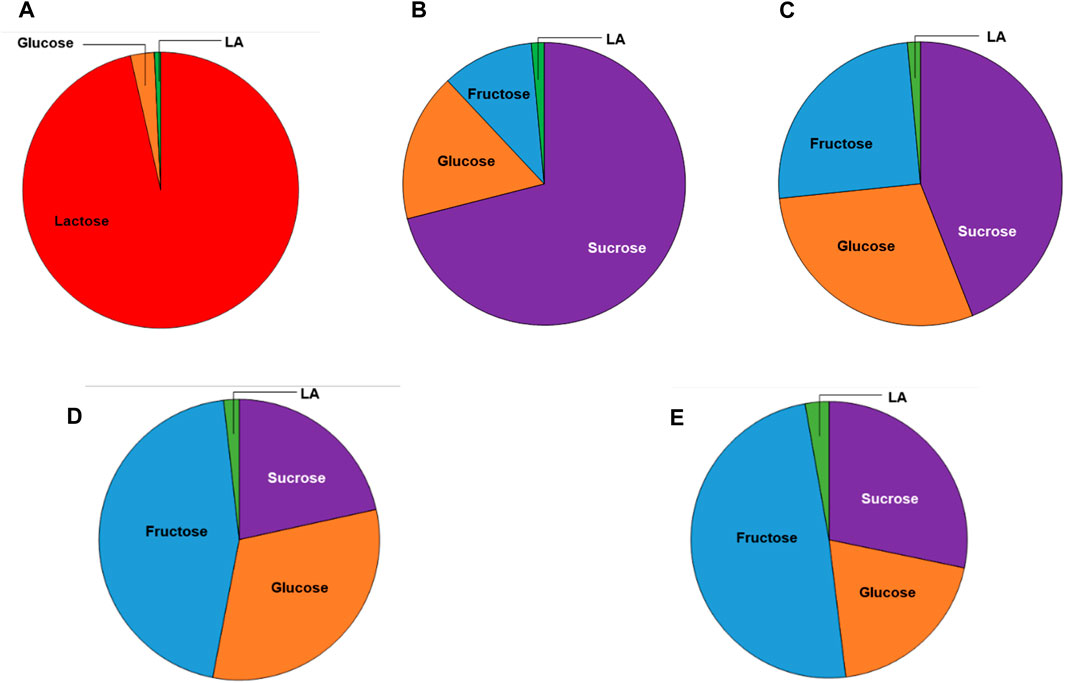
Figure 2. Pie charts of the sugar compositions (on a wet basis) of the substrates used in this study: (A) SCW; (B) peach pomace; (C) melon pomace; (D) pear pomace; (E) apple pomace. The green slices correspond to the initial LA concentrations due to spontaneous fermentation.
A small quantity of LA was already present in all FWs and SCW, probably due to the initial LA fermentation over the recovery, storage, and transportation durations probably due to the high summer temperatures. The occurrence of LA in MSL is well-established (Nisa et al., 2006; Kaur et al., 2020). The filtered apple pomace sample was characterized by a lower sugar content than apple pomace as a relevant amount of the soluble sugars remained in the filtration cake (Table 3). On the contrary, significant increases of glucose and fructose were evidenced in the hydrolysate, confirming the high amounts of polysaccharides, i.e., glucan, starch, and cellulose, in apple pomace (Gullón et al., 2008).
3.2 Fermentation and LA production by L. casei on non-hydrolyzed single substrates
The capacity of L. casei to grow and produce LA on FWs was tested preliminarily on the single substrates. LA production from SCW by L. casei has already been established in a previous work (Costa et al., 2021), along with microbial growth on MSL. The FW samples were only ground and homogenized because they contain promptly fermentable sugars as mono- and di-saccharides. Figure 4 shows the sugar depletion and LA production after 96 h of fermentation of each substrate.
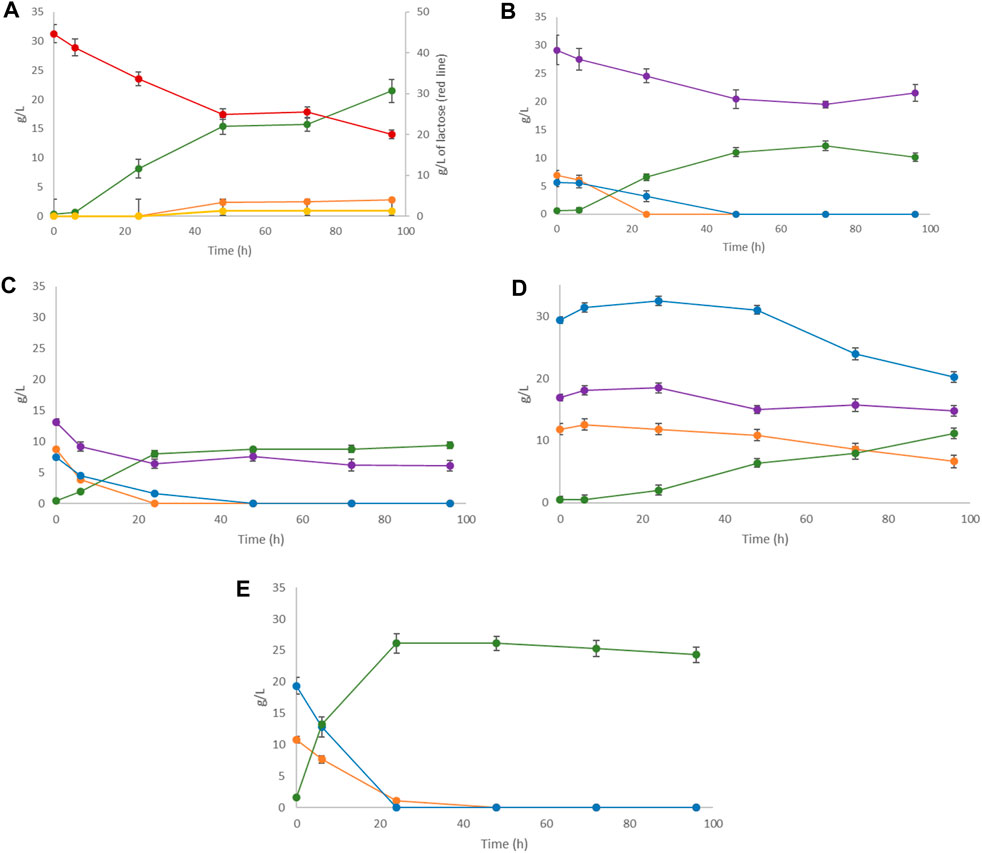
Figure 4. Single substrate LA fermentation by Lactobacillus casei with (A) SCW, (B) peach pomace, (C) melon pomace, (D) apple pomace, and (E) pear pomace as the carbon sources. Concentrations of LA (green line) along with lactose (where present, red line), sucrose (purple line), galactose (where present, yellow line), glucose (orange line), and fructose (blue line) were monitored for 96 h.
As seen, the LA yield was generally low due to the fact that the pH cannot be regulated in small-scale tests and spontaneously tends to reach acidic values, jeopardizing the biomass growth and provoking the cessation of fermentation. In fact, the main aim of these tests was to exclude the presence of toxic substances that could inhibit microbial growth, rather than optimizing the LA yield. The small-scale preliminary trials showed that all FWs had promising potential as substrates for LA fermentation except for apple pomace, as evidenced by the slow and low sugar utilization by L. casei. Of the overall sugar content (58.2 g/L), only 28% was consumed with low LA production (less than 20% yield from the initial sugar content) (Table 4). Apple pomace is mainly composed of skin, flesh (95%), and seeds (2%–4%) (Kammerer et al., 2014) and is characterized by its high content of polysaccharides, such as gluco-, xylo-, and arabino-oligosaccharides (Gullón et al., 2008), and polyphenols, such as procyanidin B2 and chlorogenic acid, that should act as growth stimulants for LAB (Duda-Chodak and Tarko, 2023). Attempts to improve the process efficiency of apple pomace have focused on the introduction of filtration or hydrolysis pretreatments prior to fermentation.
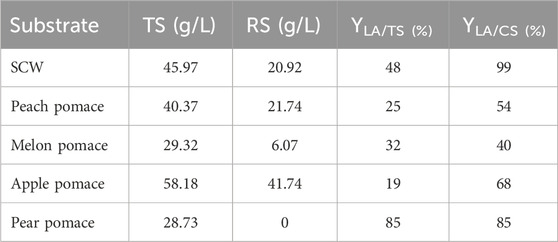
Table 4. Total sugar (TS, g/L), residual sugar (RS, g/L), and LA yields on TS (YLA/TS) and consumed sugar (CS) (YLA/CS) for single carbon source fermentations by L. casei.
L. casei fermentation on lactose clearly showed a strong dependence on product inhibition probably due to the concentration of LA, which resulted in a decrease in pH. When the pH is lower than the pKa value of LA (3.86), LA can diffuse into the cytoplasm through the plasma membrane and dissociate into lactate and protons (Maris et al., 2004). This leads to acidification of the cytoplasm and disruption of the proton motive force, resulting in inhibition of nutrient transport (Singhvi et al., 2018). Analogous effects were observed during growth for the peach and melon pomace samples after approximately 50 h of fermentation. In these cases, complete depletion of glucose and fructose was achieved, whereas sucrose remained unfermented. L. casei shows very short lag phases in all FW substrates (<6 h), as confirmed by Rezvani et al. (2017).
3.3 Fermentation and LA production during the 5-cycle repeated batch process
Repeated batch fermentation was used as the strategy based on the findings from the previously tested single substrate fermentation. The aim here was to simulate an all-season process using different FWs in series based on their specific seasonal availabilities. SCW is considered to be annually available and was consequently added in all cycles at 30% along with MSL at 10% as the nitrogen source. The overall process duration was 240 h, comprising 48 h for each cycle until complete depletion of the corresponding carbon source (Figure 5).
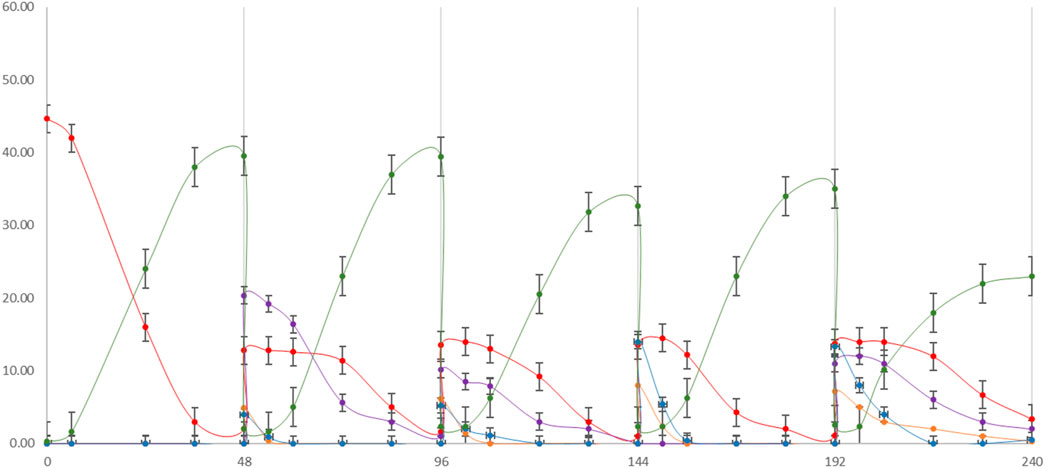
Figure 5. cycle repeated batch fermentation process: first cycle (0–48 h) SCW + MSL (10%); second cycle (48–96 h) SCW 30% + peach pomace 70%; third cycle (96–144 h) SCW 30% + melon pomace; fourth cycle (144–192 h) SCW 30% + 70% pear pomace; fifth cycle (192–240 h) SCW 30% + 70% apple pomace. LA production (green line) along with lactose (red line), sucrose (purple line), fructose (blue line), and glucose (orange line) depletions were monitored.
An inoculum of 10% fermentation broth (FB) was maintained for each batch from the previous cycle, based on the repeated batch strategy. At the beginning of fermentation, a lag phase of approximately 6 h was observed, which reduced to 1–2 h in the following batch. LA yield, substrate uptake, and LA volumetric (QLA) productivity in each batch cycle were recorded (Table 5). A total quantity of 180.56 g/L of LA was produced. In total, approximately 830 g of LA was cumulatively recovered from 4.6 L of the FB. The overall LA yields from the total sugar (TS) and consumed sugar (CS) were 88.0% and 92.9%, respectively; the average LA volumetric productivity expressed as QLA was estimated to be 0.88 g/L∙h. Although lactose, sucrose, glucose, and fructose are all present as substrates, the order of sugar utilization by L. casei is glucose ≥ fructose > sucrose > lactose, as has been generally reported for other Lactobacilli in complex media (Srinivas et al., 1990). The fifth batch cycle with the apple pomace substrate was confirmed to have the worst fermentation performance, similar to that in the single substrate process; its yield and productivity were the lowest, in addition to the low sugar consumption and LA production.
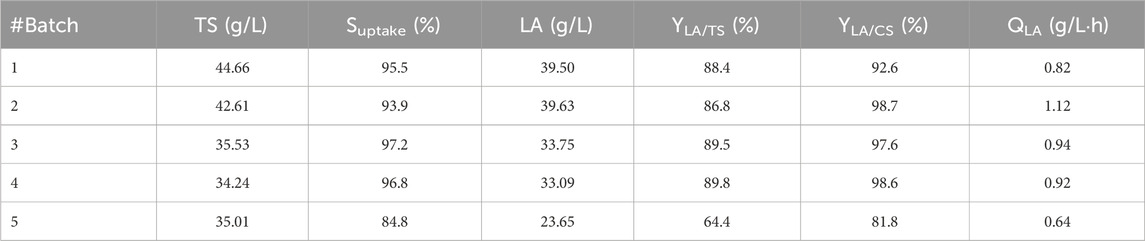
Table 5. Repeated batch fermentation parameters for each cycle. TS, total sugars; Suptake, substrate uptake percentage; LA concentration, LA yields on TS (YLA/TS) and consumed sugar (CS) (YLA/CS); and volumetric productivity (QLA) were calculated.
3.4 Fermentative performance of pretreated apple pomace
Untreated apple pomace showed low fermentative performance in the Erlenmeyer flask-scale fermentation as well as in the controlled fermenter, unlike the other FWs in this study. Therefore, two different pretreatments, i.e., mechanical filtration and enzymatic hydrolysis, were attempted. The pretreated samples were fermented by L. casei under the conditions reported in Section 3.1 as mechanically filtered apple pomace (Figure 6) and enzymatically hydrolyzed apple pomace (Figure 7).
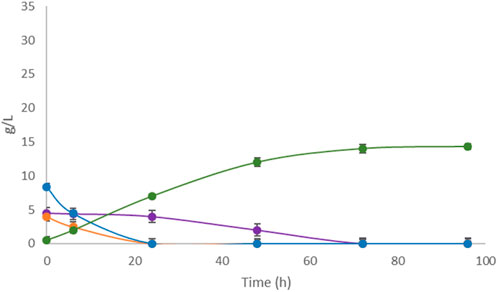
Figure 6. Single-substrate LA fermentation by L. casei with filtered apple pomace as the carbon source. Concentrations of LA (green line) along with sucrose (purple line), glucose (orange line), and fructose (blue line) were monitored for 96 h.
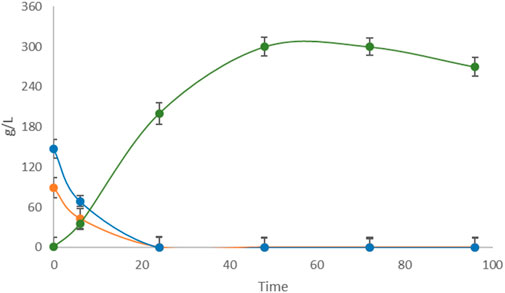
Figure 7. Single-substrate LA fermentation by L. casei with hydrolyzed apple pomace as the carbon source. Concentrations of LA (green line) along with sucrose (purple line), glucose (orange line), and fructose (blue line) were monitored for 96 h.
Complete sugar depletion occurred in the filtered medium, with a LA yield of 85.00%, which was significantly improved fermentation performance over the untreated sample. Otherwise, fermentation of the hydrolyzed apple pomace sample resulted in a yield of 115% on the initial amount of glucose and fructose, with a production of 270 g/L of LA on 235 g/L of TS, indicating that hydrolysis produced an unquantified amount of fermentable monosaccharides other than glucose and fructose, such as galactose and mannose derived from the hydrolysis of glucans, which were then used by L. casei as substrates for LA production. Finally, a second round of the 5-cycle repeated batch strategy was implemented, including the hydrolyzed apple pomace as the carbon source in the final step (data not shown). The fifth step was allowed to last 96 h, which resulted in volumetric productivities of 2.81 g/L∙h and 1.32 g/L∙h for the fifth cycle and entire process, respectively. The total amount of LA produced in the 5-cycle repeated batch process using different FWs in sequence was 397.1 g/L over 288 h.
4 Discussion
The main objective of this work was to study the use of FWs and SCW as substrates to establish an alternative and a more sustainable method of obtaining LA through the fermentative approach while reducing the environmental impacts of these residues. To assess whether the FWs and SCW could be efficiently fermented, the fermentative performances of the L. casei strain were first evaluated in media containing SCW, peach, melon, pear, and apple pomace separately. L. casei exhibits homofermentative metabolism of glucose, which primarily produces LA. Under anerobic conditions, one molecule of glucose is catabolized to two molecules of pyruvate via the EMP pathway. These pyruvate molecules are subsequently reduced to LA by lactate dehydrogenase (LDH) utilizing NADH+ H+ that generates two molecules of ATP per glucose molecule. Fructose metabolism in L. casei follows a similar route; after fructose is converted to fructose 1,6-bisphosphate, it is integrated into the glycolytic pathway, ultimately undergoing the same biochemical steps as glucose metabolism (Cui and Qu, 2021). Recent analyses of sugar utilization have demonstrated that L. casei can ferment a broad spectrum of monosaccharides and disaccharides, including glucose, fructose, galactose, mannose, ribose, sorbose, lactose, sucrose, maltose, trehalose, and cellobiose (Viana et al., 2000).
LA is one of the most important chemicals and a keystone product in industrial biotechnology; its market demand is increasing progressively and is not predicted to decline in the near future. However, the high costs of the raw materials and fermentation–separation processes have severely limited LA production (Ojo and de Smidt, 2023). For a low-priced commodity like LA, production from pure sugars is undoubtedly unsustainable for both environmental and economic reasons. However, two strategies are suggested to reduce the bioprocesses costs, i.e., selecting cheaper raw materials for fermentation and upgrading the production technologies. Several studies have focused on the development of consolidated bioprocesses for direct fermentation of complex and recalcitrant biomass, such as lignocellulose, through the design of specific microbial consortia and exploration of recombinant cellulolytic strains by metabolic engineering (Singhania et al., 2022). In both cases, the approaches for LA production remain highly challenging and far from being applicable at the industrial scale (Huang et al., 2023).
The results obtained from the single substrate fermentations suggest the feasibility of using L. casei to ferment FWs and SCW in the absence of inhibitor compounds that can have negative effects on fermentation. Moreover, pretreatments other than mixing and rough filtration appear to be necessary before the fermentations. It was confirmed at all FWs except for apple pomace, where the particular gel-like consistency of the slurry and presence of fibrous material necessitated additional mechanical filtration and hydrolysis, could be used to obtain significant LA yields. Theoretically, enzymatic hydrolysis treatments could be applied to all FWs with the prospect of increasing the fermentable sugars and consequently the LA from the given masses. However, in this study, pretreatments were relegated to a minimum and used only where strictly necessary to create a low-cost fermentation system. Pretreatments are one of the major cost categories in agri-food waste biorefinery processes, and the costs often involved in pretreatments are equivalent to the cost savings obtained by using waste biomass (Macedo et al., 2020). In single substrate fermentation, the rapid development of an acidic environment due to the lack of pH control is preserved from contamination, thus avoiding sterilization. On the other hand, the inhibitory effects of the end-product due to LA accumulation in the fermentation system can reduce the yield and productivity while increasing the fermentation time. Such inhibitory effects on the metabolic activity can be overcome by scaling-up to the fermenter, using a pH control system, and periodically removing the end-product accumulated in the medium. In the repeated batch experiments, controlling the pH can have significant effects on both LA production and sugar depletion. The repeated batch fermentation strategy was chosen here because of its advantages in terms of low investment costs, simple control and operations, and easy-to-maintain complete sterilization than the continuous strategy (Reddy et al., 2016). The repeated batch experiments conducted with various renewable substrates demonstrate the feasibility of producing large amounts of LA from different raw materials. Moreover, using seasonally available FWs as the substrates with repeated batches permits separate feeding of the fermenter with the various substrates based on their availabilities.
Many studies have highlighted the advantages of continuous and batch-fed fermentation modes on LA productivity, which are attributable to lower product inhibition, since LA is constantly removed from the bioreactor (Ahring et al., 2016; Olszewska-Widdrat et al., 2020). In this case, we applied simplicity of operation and process management as the criteria, especially without the obligation to maintain sterility. In fact, our experiments provide a small-scale simulation of the operations in a local biorefinery, which is located as close as possible to the waste production site and is supplied with wastes from primary agri-food manufacturing operations. Starting from the beginning of the seasonal campaign, with the pomace of early summer fruits to late summer and early fall productions, LA can be produced uninterruptedly for several months based on the substrate availabilities. In our simulated production process, each batch cycle was supplied with approximately 35–40 g/L of sugars, which were almost totally depleted in 48 h with 94% average yield and 0.9 g/L∙h productivity. With the hydrolyzed apple pomace, the average yield and productivity increased to about 99% based on the sugar consumed and 1.32 g/L∙h, respectively. This yield took into account the overall fermentable sugars, including the quantity released by hydrolysis. The total amount of LA produced in the five cycles was 397.1 g/L over 288 h and without apparent contamination.
Biomass concentration was not reported here, and LA production was instead used as an indicator of the good fermentation course. It is well known that LA production is partially associated with growth. After the lag phase, the metabolite production rate becomes proportional to the active biomass concentration in the reactor (Alvarez et al., 2010). Some of the sugars available in the FWs are glucose, fructose, sucrose, and lactose. Glucose and fructose are the most favorable types of sugars available for assimilation by L. casei for producing LA. Similar to the other LAB, L. casei follows a hierarchical pattern of sugar utilization. In particular, glucose is consumed faster than fructose because it has been demonstrated that the enzymes for glucose metabolism are constitutive, whereas fructose metabolism requires induction of certain enzymes in the initial stages before the sugars enter the metabolic pathway. Analogous reasons can also be used to explain how L. casei has a slightly higher preference for sucrose than lactose. Kachhy et al. (1977) and Mital et al. (1973) found that galactosidases and fructofuranosidases, which are required for lactose and sucrose hydrolysis, are inducible and constitutive enzymes, respectively. Moreover, the complexity of galactose metabolism, as one of the monomers of lactose, is reported to play a role in the slower utilization of lactose in L. casei (Srinivas et al., 1990). It is worth noting that some authors have reported that addition of sucrose to milk fermentation stimulates LA production by the lactobacilli (Nurhartadi et al., 2017) as well as the addition of glucose and fructose (Zielińska et al., 2021), confirming our results.
In this study, to further reduce the manufacturing costs of LA, MSL supplementation is proposed to replace expensive components like yeast extract or peptones. Li et al. (2006) evidenced that MSL is a good choice even if it does not fully substitute the vitamin and salt contents; they reported that the final LA concentrations in MSL-supplemented media were almost equal to those achieved with yeast extract, with only a slight decrease in productivity. The use of SCW as a fermentation substrate has been recently recommended by both the European Commission and FAO (Papirio et al., 2020). To the best of our knowledge, the results presented here are comparable to those reported by other authors (Table 6).
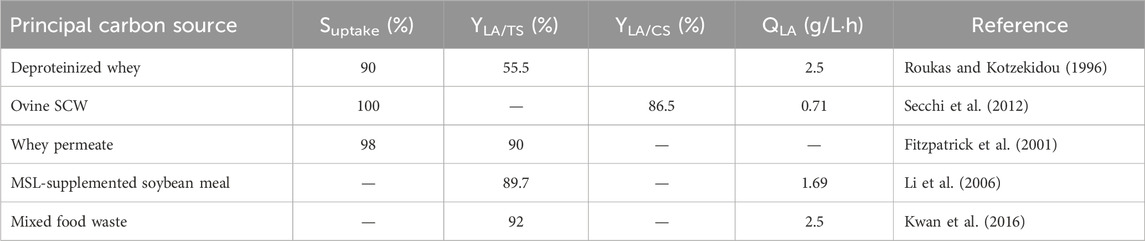
Table 6. Non-exhaustive comparison of the LA fermentation performances by L. casei on the raw substrates. Suptake, substrate uptake percentage; LA yields on TS (YLA/TS) and consumed sugar (CS) (YLA/CS); and volumetric productivity (QLA) are reported, when available.
Although our findings confirm that FWs and SCW have great potential as raw materials in biorefineries for producing value-added materials, their large-scale uses still show potential risks of economic and environmental unfeasibility, which are associated with their logistics, supply, and high perishability (Jiménez-Moreno et al., 2020). In fact, one of the major bottlenecks limiting the wide use of agri-food wastes at present is transportation from the site of production to the biorefinery, which accounts for a considerable proportion of the environmental impact (Ögmundarson et al., 2020). The option of building small local biorefineries, however, represents the problem of overall economic profitability that usually privileges large-scale plants.
5 Conclusion
Microbial fermentation of LA using agricultural and food wastes has emerged as a promising alternative to traditional chemical synthesis. This approach not only adds value to waste materials but also aligns with sustainable and ecofriendly production practices. In this study, fermentation of SCW, an annually available biomass, along with seasonally available FWs such as peach, melon, pear, and apple pomace, which are byproducts of the food processing industry, by L. casei was explored. Feedstock pretreatments were reduced and sterilization was avoided whenever possible to ensure that the process remained low-cost. Only apple pomace required preliminary enzymatic hydrolysis before fermentation. A repeated batch process was set up, yielding a maximum of 397.1 g/L of LA over 288 h, resulting in a volumetric productivity of 1.32 g/L∙h. However, for this process to be viable at a higher scale, further studies focused on upstream, scaling up, and downstream operations as well as economic feasibility analyses are essential. These findings represent a significant step forward in the sustainable production of LA, offering a practical solution for waste management while creating valuable bioproducts.
Data availability statement
The raw data supporting the conclusions of this article will be made available by the authors without undue reservation.
Author contributions
SC: methodology, writing–original draft, and writing–review and editing. DS: data curation, formal analysis, methodology, and writing–original draft. MR: methodology, and writing–original draft. SV: data curation and writing–original draft. SM: data curation and writing–original draft. ET: conceptualization, writing–original draft, and writing–review and editing.
Funding
The authors declare that financial support was received for the research, authorship, and/or publication of this article. This research was funded by the European Union Grant (no. FSE REACT-EU to SC), PON Research and Innovation 2014–2020 Grant (no. 2021: PON-DM-1062-SC-RIC CUP F71B21005820007 to SM), and National Recovery and Resilience Plan (NRRP), Mission 04 Component 2 Investment 1.5 (NextGenerationEU, call for tender no. 3277 dated 30 December 2021; award no. 0001052 dated 23 June 2022 to SM).
Conflict of interest
The authors declare that the research was conducted in the absence of any commercial or financial relationships that could be construed as a potential conflict of interest.
Publisher’s note
All claims expressed in this article are solely those of the authors and do not necessarily represent those of their affiliated organizations, or those of the publisher, the editors, and the reviewers. Any product that may be evaluated in this article, or claim that may be made by its manufacturer, is not guaranteed or endorsed by the publisher.
References
Abedi, E., and Hashemi, S. M. B. (2020). Lactic acid production–producing microorganisms and substrates sources-state of art. Heliyon 6 (10), e04974. doi:10.1016/j.heliyon.2020.e04974
Abedin, Md M., Chourasia, R., Phukon, L. C., Sarkar, P., Ray, R. C., Singh, S. P., et al. (2023). Lactic acid bacteria in the functional food industry: biotechnological properties and potential applications. Crit. Rev. Food Sci. Nutr. 0 (0), 1–19. doi:10.1080/10408398.2023.2227896
Aguirre-Garcia, Y. L., Nery-Flores, S. D., Campos-Muzquiz, L. G., Carolina Flores-Gallegos, A., Palomo-Ligas, L., Ascacio-Valdés, J. A., et al. (2024). Lactic acid fermentation in the food industry and bio-preservation of food. Fermentation 10 (3), 168. doi:10.3390/fermentation10030168
Ahmad, A., Banat, F., Alsafar, H., and Hasan, S. W. (2024). An overview of biodegradable poly (lactic acid) production from fermentative lactic acid for biomedical and bioplastic applications. Biomass Convers. Biorefinery 14 (3), 3057–3076. doi:10.1007/s13399-022-02581-3
Ahring, B. K., Traverso, J. J., Murali, N., and Srinivas, K. (2016). Continuous fermentation of clarified corn stover hydrolysate for the production of lactic acid at high yield and productivity. Biochem. Eng. J. 109, 162–169. doi:10.1016/j.bej.2016.01.012
Ajala, E. O., Olanrewaju Olonade, Y., Ajala, M. A., and Akinpelu, G. S. (2020). Lactic acid production from lignocellulose – a review of major challenges and selected solutions. ChemBioEng Rev. 7 (2), 38–49. doi:10.1002/cben.201900018
Alexandri, M., Schneider, R., Mehlmann, K., and Venus, J. (2019). Recent advances in D-lactic acid production from renewable resources: case studies on agro-industrial waste streams. Food Technol. Biotechnol. 57 (3), 293–304. doi:10.17113/ftb.57.03.19.6023
Alvarez, M. M., Aguirre-Ezkauriatza, E. J., Ramírez-Medrano, A., and Rodríguez-Sánchez, Á. (2010). Kinetic analysis and mathematical modeling of growth and lactic acid production of Lactobacillus casei var. Rhamnosus in milk whey. J. Dairy Sci. 93 (12), 5552–5560. doi:10.3168/jds.2010-3116
Awasthi, D., Wang, L., Rhee, M. S., Wang, Q., Chauliac, D., Ingram, L. O., et al. (2018). Metabolic engineering of Bacillus subtilis for production of D-lactic acid. Biotechnol. Bioeng. 115 (2), 453–463. doi:10.1002/bit.26472
Bosco, F., Cirrincione, S., Carletto, R., Marmo, L., Chiesa, F., Mazzoli, R., et al. (2021). PHA production from cheese whey and “scotta”: comparison between a consortium and a pure culture of leuconostoc mesenteroides. Microorganisms 9 (12), 2426. doi:10.3390/microorganisms9122426
Bustamante, D., Tortajada, M., Ramón, D., and Rojas, A. (2019). Production of D-lactic acid by the fermentation of orange peel waste hydrolysate by lactic acid bacteria. Fermentation 6 (1), 1. doi:10.3390/fermentation6010001
Caldeira, C., Vlysidis, A., Fiore, G., De Laurentiis, V., Vignali, G., and Sala, S. (2020). Sustainability of food waste biorefinery: a review on valorisation pathways, techno-economic constraints, and environmental assessment. Bioresour. Technol. 312, 123575. doi:10.1016/j.biortech.2020.123575
Carota, E., Crognale, S., D’Annibale, A., Gallo, A. M., Stazi, S. R., and Petruccioli, M. (2017). A sustainable use of ricotta cheese whey for microbial biodiesel production. Sci. Total Environ. 584, 554–560. doi:10.1016/j.scitotenv.2017.01.068
Catone, M. V., Palomino, M. M., Legisa, D. M., Martin, J. F., García, V. M., Ruzal, S. M., et al. (2021). Lactic acid production using cheese whey based medium in a stirred tank reactor by a ccpA mutant of lacticaseibacillus casei. World J. Microbiol. Biotechnol. 37 (4), 61. doi:10.1007/s11274-021-03028-z
Costa, S., Summa, D., Semeraro, B., Zappaterra, F., Rugiero, I., and Tamburini, E. (2021). Fermentation as a strategy for bio-transforming waste into resources: lactic acid production from agri-food residues. Fermentation 7 (1), 3. doi:10.3390/fermentation7010003
Cui, Y., and Qu, X. (2021). Genetic mechanisms of prebiotic carbohydrate metabolism in lactic acid bacteria: emphasis on lacticaseibacillus casei and lacticaseibacillus paracasei as flexible, diverse and outstanding prebiotic carbohydrate starters. Trends Food Sci. Technol. 115, 486–499. doi:10.1016/j.tifs.2021.06.058
Dedenaro, G., Costa, S., Rugiero, I., Pedrini, P., and Tamburini, E. (2016). Valorization of agri-food waste via fermentation: production of l-lactic acid as a building block for the synthesis of biopolymers. Appl. Sci. 6 (12), 379. doi:10.3390/app6120379
De Giorgi, S., Raddadi, N., Fabbri, A., Toschi, T. G., and Fava, F. (2018). Potential use of ricotta cheese whey for the production of lactobionic acid by Pseudomonas taetrolens strains. New Biotechnol. 42, 71–76. doi:10.1016/j.nbt.2018.02.010
Derabli, B., Nancib, A., Nancib, N., Aníbal, J., Raposo, S., Rodrigues, B., et al. (2022). Opuntia Ficus indica waste as a cost effective carbon source for lactic acid production by Lactobacillus plantarum. Food Chem. 370, 131005. doi:10.1016/j.foodchem.2021.131005
Duda-Chodak, A., and Tarko, T. (2023). Possible side effects of polyphenols and their interactions with medicines. Molecules 28 (6), 2536. doi:10.3390/molecules28062536
Fidan, H., Esatbeyoglu, T., Simat, V., Trif, M., Tabanelli, G., Kostka, T., et al. (2022). Recent developments of lactic acid bacteria and their metabolites on foodborne pathogens and spoilage bacteria: facts and gaps. Food Biosci. 47, 101741. doi:10.1016/j.fbio.2022.101741
Fitzpatrick, J. J., Ahrens, M., and Smith, S. (2001). Effect of manganese on Lactobacillus casei fermentation to produce lactic acid from whey permeate. Process Biochem. 36 (7), 671–675. doi:10.1016/S0032-9592(00)00265-X
Ganesh, K. S., Sridhar, A., and Vishali, S. (2022). Utilization of fruit and vegetable waste to produce value-added products: conventional utilization and emerging opportunities-A review. Chemosphere 287, 132221. doi:10.1016/j.chemosphere.2021.132221
Gao, C., Ma, C., and Xu, P. (2011). Biotechnological routes based on lactic acid production from biomass. Biotechnol. Adv. 29 (6), 930–939. doi:10.1016/j.biotechadv.2011.07.022
Garita-Cambronero, J., Hijosa-Valsero, M., Paniagua-García, A. I., and Díez-Antolínez, R. (2021). Revisiting the production of L(+)-Lactic acid from vine shoots: bioconversion improvements by employing thermotolerant bacteria. Appl. Microbiol. Biotechnol. 105 (24), 9385–9402. doi:10.1007/s00253-021-11693-1
Gullón, B., Yáñez, R., Alonso, J. L., and Parajó, J. C. (2008). L-lactic acid production from apple pomace by sequential hydrolysis and fermentation. Bioresour. Technol. 99 (2), 308–319. doi:10.1016/j.biortech.2006.12.018
Hossain, M. T. (2023). “2 - synthetic biology and metabolic engineering for improvement of lactic acid bacteria as cell factories,” in Lactic acid bacteria as cell factories, applied biotechnology reviews. Editors A. Poonia, D. Montet, R. C. Ray, V. A. De Carvalho Azevedo, and S. Paramithiotis (Amsterdam, Netherlands: Woodhead Publishing), 17–28. doi:10.1016/B978-0-323-91930-2.00006-7
Huang, Y., Wang, Y., Shang, N., and Li, P. (2023). Microbial fermentation processes of lactic acid: challenges, solutions, and future prospects. Foods 12 (12), 2311. doi:10.3390/foods12122311
Jawad, A. H., Alkarkhi, A. F. M., Jason, O. C., Easa, A. M., and Norulaini, N. A. N. (2013). Production of the lactic acid from mango peel waste–factorial experiment. J. King Saud University-Science 25 (1), 39–45. doi:10.1016/j.jksus.2012.04.001
Jiménez-Moreno, N., Esparza, I., Bimbela, F., Gandía, L. M., and Ancín-Azpilicueta, C. (2020). Valorization of selected fruit and vegetable wastes as bioactive compounds: opportunities and challenges. Crit. Rev. Environ. Sci. Technol. 50 (20), 2061–2108. doi:10.1080/10643389.2019.1694819
Kachhy, A. N., Modi, V. V., and Vaidya, H. C. (1977). Induction of beta-galactosidase in Lactobacillus acidophilus. Indian J. Exp. Biol. 15 (4), 271–273.
Kammerer, D. R., Kammerer, J., Valet, R., and Carle, R. (2014). Recovery of polyphenols from the by-products of plant food processing and application as valuable food ingredients. Food Res. Int. 7th World Congr. Polyphenols Appl. 65, 2–12. doi:10.1016/j.foodres.2014.06.012
Kandemir, K., Piskin, E., Xiao, J., Tomas, M., and Capanoglu, E. (2022). Fruit juice industry wastes as a source of bioactives. J. Agric. Food Chem. 70 (23), 6805–6832. doi:10.1021/acs.jafc.2c00756
Kaur, A., Rishi, V., Kumar Soni, S., and Rishi, P. (2020). A novel multi-enzyme preparation produced from Aspergillus Niger using biodegradable waste: a possible option to combat heterogeneous biofilms. Amb. Express 10 (1), 36. doi:10.1186/s13568-020-00970-3
Kumar, S., Yadav, N., Nain, L., and Khare, S. K. (2020). A simple downstream processing protocol for the recovery of lactic acid from the fermentation broth. Bioresour. Technol. 318, 124260. doi:10.1016/j.biortech.2020.124260
Kumar, V., Rana, A., Ahire, J. J., and Taneja, N. K. (2023). “Utilization of whey for production of bioenergy and biofuels,” in Whey valorization: innovations, technological advancements and sustainable exploitation. Editors A. Poonia, and A. T. Petkoska (Singapore: Springer Nature), 311–321. doi:10.1007/978-981-99-5459-9_15
Kwan, T. H., Hu, Y., and Lin, C. S.K. (2016). Valorisation of food waste via fungal hydrolysis and lactic acid fermentation with Lactobacillus casei shirota. Bioresour. Technol. Special Issue Bioenergy, Bioprod. Environ. Sustain. 217, 129–136. doi:10.1016/j.biortech.2016.01.134
Levit, R., Cortes-Perez, N. G., de Moreno de Leblanc, A., Loiseau, J., Aucouturier, A., Langella, P., et al. (2022). Use of genetically modified lactic acid bacteria and bifidobacteria as live delivery vectors for human and animal health. Gut Microbes 14 (1), 2110821. doi:10.1080/19490976.2022.2110821
Li, Z., Ding, S., Li, Z., and Tan, T. (2006). L-lactic acid production by Lactobacillus casei fermentation with corn steep liquor-supplemented acid-hydrolysate of soybean meal. Biotechnol. J. 1 (12), 1453–1458. doi:10.1002/biot.200600099
Macedo, C., Victor, J., Ranke, F. F. de B., Escaramboni, B., Campioni, T. S., Núñez, E. G. F., et al. (2020). Cost-effective lactic acid production by fermentation of agro-industrial residues. Biocatal. Agric. Biotechnol. 27, 101706. doi:10.1016/j.bcab.2020.101706
Marchesan, A. N., Silva, J. F. L., Maciel Filho, R., and Maciel, M. R. W. (2021). Techno-economic analysis of alternative designs for low-pH lactic acid production. ACS Sustain. Chem. Eng. 9 (36), 12120–12131. doi:10.1021/acssuschemeng.1c03447
Maris, A. J. A. van, Winkler, A. A., Porro, D., van Dijken, J. P., and Pronk, J. T. (2004). Homofermentative lactate production cannot sustain anaerobic growth of engineered Saccharomyces cerevisiae: possible consequence of energy-dependent lactate export. Appl. Environ. Microbiol. 70 (5), 2898–2905. doi:10.1128/AEM.70.5.2898-2905.2004
Mazzoli, R. (2021). Current progress in production of building-block organic acids by consolidated bioprocessing of lignocellulose. Fermentation 7 (4), 248. doi:10.3390/fermentation7040248
Mital, B. K., Shallenberger, R. S., and Steinkraus, K. H. (1973). α-Galactosidase activity of lactobacilli. Appl. Microbiol. 26 (5), 783–788. doi:10.1128/am.26.5.783-788.1973
Mittermeier, F., Bäumler, M., Arulrajah, P., García Lima, J. de J., Hauke, S., Stock, A., et al. (2023). Artificial microbial consortia for bioproduction processes. Eng. Life Sci. 23 (1), e2100152. doi:10.1002/elsc.202100152
Ngouénam, J. R., Kenfack, C. H. M., Kouam, E. M. F., Kaktcham, P. M., Maharjan, R., and Ngoufack, F. Z. (2021). Lactic acid production ability of Lactobacillus sp. from four tropical fruits using their by-products as carbon source. Heliyon 7 (5), e07079. doi:10.1016/j.heliyon.2021.e07079
Nisa, M. U., Ajmal Khan, M., Sarwar, M., Lee, W. S., Lee, H. J., Ki, K. S., et al. (2006). Influence of corn steep liquor on feeding value of urea treated wheat straw in buffaloes fed at restricted diets. Asian-Australasian J. Animal Sci. 19 (11), 1610–1616. doi:10.5713/ajas.2006.1610
Nurhartadi, E., Utami, R., Nursiwi, A., Sari, A. M., Widowati, E., Sanjaya, A. P., et al. (2017). Effect of incubation time and sucrose addition on the characteristics of cheese whey yoghurt. IOP Conf. Ser. Mater. Sci. Eng. 193 (1), 012008. doi:10.1088/1757-899X/193/1/012008
Ögmundarson, Ó., Sukumara, S., Laurent, A., and Fantke, P. (2020). Environmental hotspots of lactic acid production systems. GCB Bioenergy 12 (1), 19–38. doi:10.1111/gcbb.12652
Ojo, A. O., and de Smidt, O. (2023). Lactic acid: a comprehensive review of production to purification. Processes 11 (3), 688. doi:10.3390/pr11030688
Olszewska-Widdrat, A., Alexandri, M., López-Gómez, J. P., Schneider, R., and Venus, J. (2020). Batch and continuous lactic acid fermentation based on A multi-substrate approach. Microorganisms 8 (7), 1084. doi:10.3390/microorganisms8071084
Ordoñez-Araque, R., Quishpillo-Miranda, N., and Ramos-Guerrero, L. (2022). Edible insects for humans and animals: nutritional composition and an option for mitigating environmental damage. Insects 13 (10), 944. doi:10.3390/insects13100944
Ortiz-Sanchez, M., Inocencio-García, P.-J., Alzate-Ramírez, A. F., and Alzate, C. A. C. (2023). Potential and restrictions of food-waste valorization through fermentation processes. Fermentation 9 (3), 274. doi:10.3390/fermentation9030274
Osorio-González, C. S., Gómez-Falcon, N., Brar, S. K., and Ramírez, A. A. (2022). Cheese whey as a potential feedstock for producing renewable biofuels: a review. Energies 15 (18), 6828. doi:10.3390/en15186828
Papirio, S., Matassa, S., Pirozzi, F., and Esposito, G. (2020). Anaerobic Co-digestion of cheese whey and industrial hemp residues opens new perspectives for the valorization of agri-food waste. Energies 13 (11), 2820. doi:10.3390/en13112820
Pasta, C., Caccamo, M., Petriglieri, R., Difalco, A., Farina, G., Belvedere, G., et al. (2023). Sicilian whey: utilization of ricotta whey in the production of value-added artisanal beers. Fermentation 10 (1), 19. doi:10.3390/fermentation10010019
Pires, A. F., Garcia Marnotes, N., Rubio, O. D., Garcia, A. C., and Pereira, C. D. (2021). Dairy by-products: a review on the valorization of whey and second cheese whey. Foods 10 (5), 1067. doi:10.3390/foods10051067
Poništ, J., Dubšíková, V., Schwarz, M., and Samešová, D. (2021). Methods of processing whey waste from dairies. A review. Environ. Prot. Eng. 47 (4). doi:10.37190/epe210405
Rawoof, S. A. A., Senthil Kumar, P., Vo, D.-V. N., Devaraj, K., Mani, Y., Devaraj, T., et al. (2021). Production of optically pure lactic acid by microbial fermentation: a review. Environ. Chem. Lett. 19 (1), 539–556. doi:10.1007/s10311-020-01083-w
Read, Q. D., Brown, S., Cuéllar, A. D., Finn, S. M., Gephart, J. A., Marston, L. T., et al. (2020). Assessing the environmental impacts of halving food loss and waste along the food supply chain. Sci. Total Environ. 712, 136255. doi:10.1016/j.scitotenv.2019.136255
Reddy, L. V., Kim, Y.-M., Yun, J.-S., Ryu, H.-W., and Young-Jung, W. (2016). L-lactic acid production by combined utilization of agricultural bioresources as renewable and economical substrates through batch and repeated-batch fermentation of Enterococcus faecalis RKY1. Bioresour. Technol. 209, 187–194. doi:10.1016/j.biortech.2016.02.115
Ren, Y., Wang, X., Li, Y., Li, Y.-Y., and Wang, Q. (2022). Lactic acid production by fermentation of biomass: recent achievements and perspectives. Sustainability 14 (21), 14434. doi:10.3390/su142114434
Rezvani, F., Ardestani, F., and Najafpour, G. (2017). Growth kinetic models of five species of lactobacilli and lactose consumption in batch submerged culture. Braz. J. Microbiol. 48 (2), 251–258. doi:10.1016/j.bjm.2016.12.007
Ribeiro, J. C., Mota, V. T., Oliveira, V. M. de, and Zaiat, M. (2022). Hydrogen and organic acid production from dark fermentation of cheese whey without buffers under mesophilic condition. J. Environ. Manag. 304, 114253. doi:10.1016/j.jenvman.2021.114253
Roukas, T., and Kotzekidou, P. (1996). Continuous production of lactic acid from deproteinized whey by coimmobilized Lactobacillus casei and lactococcus lactis cells in a packed-bed reactor. Food Biotechnol. 10 (3), 231–242. doi:10.1080/08905439609549916
Ruiz-Ruiz, F., Mancera-Andrade, E. I., Parra-Saldivar, R., Keshavarz, T., and Iqbal, H. M. N. (2017). Drug delivery and cosmeceutical applications of poly- lactic acid based novel constructs - a review. Curr. Drug Metab. 18 (10), 914–925. doi:10.2174/1389200218666170919170335
Sakr, E. A. E., Massoud, M. I., and Ragaee, S. (2021). Food wastes as natural sources of lactic acid bacterial exopolysaccharides for the functional food industry: a review. Int. J. Biol. Macromol. 189, 232–241. doi:10.1016/j.ijbiomac.2021.08.135
Sansonetti, S., Curcio, S., Calabrò, V., and Iorio, G. (2009). Bio-ethanol production by fermentation of ricotta cheese whey as an effective alternative non-vegetable source. Biomass Bioenergy 33 (12), 1687–1692. doi:10.1016/j.biombioe.2009.09.002
Sayed, W. F., Salem, W. M., Zeinab, A. S., and Abdelmoneim, K. A. (2020). Production of lactic acid from whey permeates using lactic acid bacteria isolated from cheese. SVU-International J. Veterinary Sci. 3 (2), 78–95. doi:10.21608/svu.2020.35000.1064
Secchi, N., Giunta, D., Pretti, L., Ruiz García, M., Roggio, T., Mannazzu, I., et al. (2012). Bioconversion of ovine scotta into lactic acid with pure and mixed cultures of lactic acid bacteria. J. Industrial Microbiol. Biotechnol. 39 (1), 175–181. doi:10.1007/s10295-011-1013-9
Singhania, R. R., Patel, A. K., Singh, A., Haldar, D., Soam, S., Chen, C.-W., et al. (2022). Consolidated bioprocessing of lignocellulosic biomass: technological advances and challenges. Bioresour. Technol. 354, 127153. doi:10.1016/j.biortech.2022.127153
Singhvi, M., Zendo, T., and Sonomoto, K. (2018). Free lactic acid production under acidic conditions by lactic acid bacteria strains: challenges and future prospects. Appl. Microbiol. Biotechnol. 102 (14), 5911–5924. doi:10.1007/s00253-018-9092-4
Sommella, E., Pepe, G., Ventre, G., Pagano, F., Conte, G. M., Ostacolo, C., et al. (2016). Detailed peptide profiling of “scotta”: from a dairy waste to a source of potential health-promoting compounds. Dairy Sci. Technol. 96 (5), 763–771. doi:10.1007/s13594-016-0297-y
Srinivas, D., Mital, B. K., and Garg, S. K. (1990). Utilization of sugars by Lactobacillus acidophilus strains. Int. J. Food Microbiol. 10 (1), 51–57. doi:10.1016/0168-1605(90)90007-R
Sun, Y., Li, X., Wei, C., Qi, W., and Xiu, Z. (2021). An aptly industrialized bioprocess for lactic acid production from corn stover using thermotolerant microbial consortia. Bioprocess Biosyst. Eng. 44 (11), 2445–2454. doi:10.1007/s00449-021-02616-5
Sun, Y., Xu, Z., Zheng, Y., Zhou, J., and Xiu, Z. (2019). Efficient production of lactic acid from sugarcane molasses by a newly microbial consortium CEE-DL15. Process Biochem. 81, 132–138. doi:10.1016/j.procbio.2019.03.022
Suri, S., Singh, A., and Nema, P. K. (2022). Current applications of citrus fruit processing waste: a scientific outlook. Appl. Food Res. 2 (1), 100050. doi:10.1016/j.afres.2022.100050
Thygesen, A., Tsapekos, P., Alvarado-Morales, M., and Angelidaki, I. (2021). Valorization of municipal organic waste into purified lactic acid. Bioresour. Technol. 342, 125933. doi:10.1016/j.biortech.2021.125933
Tian, X., Chen, H., Liu, H., and Chen, J. (2021). Recent advances in lactic acid production by lactic acid bacteria. Appl. Biochem. Biotechnol. 193 (12), 4151–4171. doi:10.1007/s12010-021-03672-z
Tsermoula, P., Khakimov, B., Nielsen, J. H., and Engelsen, S. B. (2021). WHEY - the waste-stream that became more valuable than the food product. Trends Food Sci. Technol. 118, 230–241. doi:10.1016/j.tifs.2021.08.025
Viana, R., Vicente, M., Dossonnet, V., Vadeboncoeur, C., Pérez-Martínez, G., and Deutscher, J. (2000). Enzyme I and HPr from Lactobacillus casei: their role in sugar transport, carbon catabolite repression and inducer exclusion. Mol. Microbiol. 36 (3), 570–584. doi:10.1046/j.1365-2958.2000.01862.x
Vigneshwar, S. S., Swetha, A., Panchamoorthy Gopinath, K., Goutham, R., Pal, R., Arun, J., et al. (2022). Bioprocessing of biowaste derived from food supply chain side-streams for extraction of value added bioproducts through biorefinery approach. Food Chem. Toxicol. 165, 113184. doi:10.1016/j.fct.2022.113184
Wahjudi, S. M. W., Petrzik, T., Oudenne, F., Calvo, C. L., and Büchs, J. (2023). Unraveling the potential and constraints associated with corn steep liquor as a nutrient source for industrial fermentations. Biotechnol. Prog. 39 (6), e3386. doi:10.1002/btpr.3386
Walzberg, J., Lonca, G., Hanes, R. J., Eberle, A. L., Carpenter, A., and Heath, G. A. (2021). Do we need a new sustainability assessment method for the circular economy? A critical literature review. Front. Sustain. 1. doi:10.3389/frsus.2020.620047
Yankov, D. (2022). Fermentative lactic acid production from lignocellulosic feedstocks: from source to purified product. Front. Chem. 10, 823005. doi:10.3389/fchem.2022.823005
Zhang, W., Zhao, F., Yang, T., Zhao, F., and Liu, S. (2017). Celluclast 1.5L pretreatment enhanced aroma of palm kernels and oil after kernel roasting. J. Sci. Food Agric. 97 (15), 5146–5157. doi:10.1002/jsfa.8394
Zhou, K., Yu, J., Ma, Y., Cai, L., Zheng, L., Gong, W., et al. (2022). Corn steep liquor: green biological resources for bioindustry. Appl. Biochem. Biotechnol. 194 (7), 3280–3295. doi:10.1007/s12010-022-03904-w
Zielińska, D., Marciniak-Lukasiak, K., Karbowiak, M., and Lukasiak, P. (2021). Effects of fructose and oligofructose addition on milk fermentation using novel Lactobacillus cultures to obtain high-quality yogurt-like products. Molecules 26 (19), 5730. doi:10.3390/molecules26195730
Keywords: lactic acid, fruit waste, second cheese whey, fermentation, biorefinery
Citation: Costa S, Summa D, Radice M, Vertuani S, Manfredini S and Tamburini E (2024) Lactic acid production by Lactobacillus casei using a sequence of seasonally available fruit wastes as sustainable carbon sources. Front. Bioeng. Biotechnol. 12:1447278. doi: 10.3389/fbioe.2024.1447278
Received: 11 June 2024; Accepted: 12 July 2024;
Published: 02 August 2024.
Edited by:
Alessandro Concas, University of Cagliari, ItalyReviewed by:
Nikhil Gauravarapu Navlur, Dr. B. R. Ambedkar National Institute of Technology Jalandhar, IndiaDiego Armando Esquivel Hernandez, Metropolitan Autonomous University, Mexico
Zhongyang Qiu, Huaiyin Normal University, China
Copyright © 2024 Costa, Summa, Radice, Vertuani, Manfredini and Tamburini. This is an open-access article distributed under the terms of the Creative Commons Attribution License (CC BY). The use, distribution or reproduction in other forums is permitted, provided the original author(s) and the copyright owner(s) are credited and that the original publication in this journal is cited, in accordance with accepted academic practice. No use, distribution or reproduction is permitted which does not comply with these terms.
*Correspondence: Elena Tamburini, dG1lQHVuaWZlLml0
†These authors have contributed equally to this work and share first authorship