- 1State Research Institute Centre for Innovative Medicine, Department of Immunology and Bioelectrochemistry, Vilnius, Lithuania
- 2Faculty of Electronics, Vilnius Gediminas Technical University, Vilnius, Lithuania
- 3Faculty of Pharmacy, Department of Molecular and Cellular Biology, Wroclaw Medical University, Wroclaw, Poland
Gene electrotransfer (GET) is non-viral gene delivery technique, also known as electroporation-mediated gene delivery or electrotransfection. GET is a method used to introduce foreign genetic material (such as DNA or RNA) into cells by applying external pulsed electric fields (PEFs) to create temporary pores in the cell membrane. This study was undertaken to examine the impact of buffer composition on the efficiency of GET in mammalian cells Also, we specifically compared the effectiveness of high-frequency nanosecond (ns) pulses with standard microsecond (µs) pulses. For the assessment of cell transfection efficiency and viability, flow cytometric analysis, luminescent assays, and measurements of metabolic activity were conducted. The efficiency of electrotransfection was evaluated using two different proteins encoding plasmids (pEGFP-N1 and Luciferase-pcDNA3). The investigation revealed that the composition of the electroporation buffer significantly influences the efficacy of GET in CHO-K1 cell line. The different susceptibility of cell lines to the electric field and the plasmid cytotoxicity were reported. It was also shown that electroporation with nanosecond duration PEF protocols ensured equivalent or even better transfection efficiency than standard µsPEF. Additionally, we successfully performed long-term transfection of the murine 4T1 cell line using high-frequency nanosecond PEFs and confirmed its’ applicability in an in vivo model. The findings from the study can be applied to optimize electrotransfection conditions.
1 Introduction
Electroporation (EP) is a physical phenomenon in which cell membranes polarize due to an external electric field and nanoscale aqueous pores in a phospholipid bilayer are formed. This process facilitates the delivery of usually impermeable molecules into the cell (Kotnik et al., 2019; Tylewicz, 2020). EP is widely used as a non-viral gene delivery technique, termed gene electrotransfer (GET). This physical method improves cellular uptake of DNA, plasmid DNA (pDNA), and RNA and can be applied to both in vitro and in vivo models (Lambricht et al., 2015; Cervia and Yuan, 2018; Rakoczy et al., 2022). The wide applicability of GET involves treatment of cancer and other diseases (Li and Huang, 2000; Fewell et al., 2005) and DNA vaccination (Chattergoon et al., 1997). Electroporation presents numerous advantages in comparison to viral gene transfer methodology, including the ability to repeatedly administer to tissues without triggering substantial immune responses or toxicity, minimizing the occurrence of transgene expression at non-target sites, good stability, the capability to carry large amounts of material, high therapeutic efficacy, and low cost. While the standard viral gene transfer technique is limited by significant health risk associated with viral components in vectors cytotoxicity, immunogenicity and the potential for mutagenesis through chromosomal integration (Henshaw and Yuan, 2012; Li et al., 2012; Karagöz et al., 2018; Kumar et al., 2021). Nevertheless, the electroporation-based gene transfer methodology also faces limitations. These include relatively low transfection efficiency compared to viral delivery techniques, as well as compromised cell viability following treatment, particularly when employing high-energy electric pulses (Henshaw and Yuan, 2012). Therefore, there is a significant emphasis on the importance of research in electroporation-based gene electrotransfer, particularly in parametric optimization to ensure higher transfection efficacy.
In addition, it is important to consider that GET is a multistep process that includes electropermeabilization of the cell membrane lipid bilayer, electrophoretic migration of DNA toward the membrane with subsequent interaction, translocation across the membrane, migration toward the nucleus, and passage through it to ensure gene expression (Gong et al., 2022; Rakoczy et al., 2022), where the interaction of a DNA molecule with the cell membrane was marked as one of the key steps (Neumann et al., 1996; Golzio et al., 2002). Moreover, maintaining high viability and ensuring adequate plasmid quality are imperative for successful cell electrotransfection.
It is widely acknowledged that primary factors influencing the effectiveness of electroporation include electric field strength, pulse number, pulse duration, and applied frequency. Additionally, the efficiency can be influenced by temperature, plasmid quality, molecular size, and concentration as well as properties of the targeted cells (shape, cell membrane charge, cell density) (Golzio et al., 1998; Čegovnik and Novaković, 2004; Lesueur et al., 2016; Desai et al., 2017; Chopra et al., 2020; Sherba et al., 2020; Radzevičiūtė et al., 2022a). Studies have additionally shown that the characteristics of the electroporation buffer, including conductivity, osmolarity, and ionic composition, used during and post-electroporation, greatly influence gene electrotransfer efficiency (Neumann et al., 1996; Hristova et al., 1997; Flanagan et al., 2011; Haberl et al., 2013; Chopra et al., 2020).
In the matter of buffer ionic composition, it is noted that divalent cations such as Ca2+ and Mg2+ are necessary for the formation of DNA–membrane complex during the PEFs application. They act as a bridge between negatively-charged DNA and the negatively-charged cell plasma membrane and thus improve DNA–membrane binding (Wong and Neumann, 1982; Haberl et al., 2013). Other study results revealed that increased concentration of Mg2+ ions in electroporation buffer results in stronger interaction of DNA with the membrane and higher cell viability post-treatment. However, lower gene electrotransfer efficiency was reported (Haberl et al., 2010). Furthermore, the presence of Mg2+ in electroporation buffers may enhance cell viability by accelerating the restoration of ion homeostasis, even with higher energy pulses used (Sherba et al., 2020). The lower electrotransfection efficiency when higher concentration of Mg2+ ions is used can be explained by the increased activity of DNAse enzyme (Tullis and Price, 1974) or it can be hypothesized that Mg2+ ions bind DNA to the cell surface with such strength that it cannot pass into the cell during EP (Haberl et al., 2010). The role of Ca2+ ions in the EP buffer has also been reported. Data from in vivo studies demonstrated that the peak of transgene expression was achieved when Ca2+ levels were optimized (20–100 mmol/L) (Suzuki et al., 2003). Contrastingly, another group reported that the presence of Ca2+ ions in the DNA solution significantly inhibited transgene expression following EP procedure (Zhao et al., 2006). The inhibitory effect of Ca2+ ions may be associated with the properties of the cell membrane following electroporation and the subsequent resealing process (Ciobanu et al., 2018; Shi et al., 2022; Li and Shaw, 2023). Thus, to achieve high efficiency mammalian cell transfection additional optimization experiments need to be performed.
Most electric pulse protocols utilized for gene electrotransfer typically involve the application of long-duration electrical pulses (micro-millisecond range) (Potter and Heller, 2003; Cemazar et al., 2009; Kandušer et al., 2009; Nomura et al., 2016) or treatment modalities like European Standard Operating Procedures for Electrochemotherapy (ESOPE) (Cukjati et al., 2007; Miklavčič et al., 2012). Experimental data demonstrates that even longer than microsecond duration pulses of higher amplitude ensured better gene electrotransfer and longer protein expression in vivo model (Zampaglione et al., 2005; Prud’homme et al., 2006; Mir, 2009). This can be attributed to the increased electrophoretic effect observed when longer duration electric pulses are applied (Sukharev et al., 1992). However, it is noted that longer-duration pulses cause undesirable muscle contractions (Golberg and Rubinsky, 2012) or electrochemical reactions (Rodaite-Riseviciene et al., 2014), which could potentially impact the quality of plasmid DNA and consequently have a negative effect on GET efficiency (Cukjati et al., 2007; Arkhangelsky et al., 2008; Zhao et al., 2021). One potential method to mitigate these adverse effects is through the application of shorter pulsed electric fields in the nanosecond range (nsPEFs). Recent studies revealed that pre-treatment with nsPEF before msPEF increased transfected cells viability and gene expression (Guo et al., 2014). Additionally, we presented a proof of concept (Ruzgys et al., 2018) and published experimental data that high-frequency sub-microsecond PEF protocols can ensure high transfection efficiency and cell viability (Novickij et al., 2020; Radzevičiūtė et al., 2022b). Further advantages of applying high-frequency nsPEFs include the reduction in the generation of reactive oxygen species (ROS) (Radzevičiūtė-Valčiukė et al., 2023). The absence of an electrophoretic component in the pulse itself is compensated by the accumulation of residual transmembrane potential (TMP) during the bursts, leaving the cells polarized. This polarization may enhance the stability and/or size of the pores (Radzevičiūtė et al., 2022a). This is a relatively new (first works occurring in 2018) and evolving field that necessitates more applied research to further substantiate the feasibility of nsPEF in GET. It is also necessary to study how electroporation buffer composition as well as different EP parametric protocols affects GET efficacy.
Therefore, in this study, we focused on the effects of buffer composition on GET efficiency in mammalian cells using high-frequency nanosecond (ns) in comparison to standard microsecond (µs) pulses. The main goal of this study was to define electroporation buffer composition and develop long-term transfected murine (4T1) cancer cell line, as a proof of concept.
2 Materials and methods
2.1 Electroporation setup and parameters
Electroctroporation experiments were performed using a 3 kV, 100 ns–1 m square-wave pulse generator (VilniusTECH, Vilnius, Lithuania) (Novickij et al., 2016) and a commercially available electroporation cuvette with a 1 mm gap between electrodes (Biorad, Hercules, United States). The voltage applied to the cuvette varied according to selected protocols. For evaluation of electrotransfection (pEGFP-N1 plasmid) efficacy dependency on EP buffer (1–8) and cell viability, we have conducted tests with ESOPE-like microsecond (µsPEF) and nanosecond (nsPEF) pulsing protocols. The µsPEF protocol involved pulses of 100 µs duration and electric field strength values of 1.2 kV/cm and 1.5 kV/cm, using four and eight pulses at a pulse repetition frequency of 1 Hz. For nsPEF, we applied amplitudes of 4 kV/cm and 5 kV/cm with 500 and 250 pulses, respectively, at a repetition frequency of 1 MHz. Permeabilization assay was performed for both µsPEF and nsPEF using 1.2 kV/cm and 2.5–10 kV/cm pulse amplitudes, respectively. Finally, only two electric protocols [µsPEF (1.2 kV/cm × 100 µs × 8, 1 Hz) and nsPEF (2.5–10 kV/cm × 300 ns × 250, 1 MHz)], were used in electrotransfection with Luciferase-pcDNA3 plasmid.
2.2 Cell lines
Murine 4T1 (ATCC-CRL-2539), a mammary gland tumor cell line of BALB/c origin and Chinese Hamster Ovary CHO-K1 (ATCC-CCL-61) cell lines were grown in RPMI 1640 medium supplemented with glutamine, 100 U/mL of penicillin, 100 mg/mL of streptomycin, and 10% of fetal bovine serum (FBS). Cultivation of cells was carried out at 37°C with a 5% CO2 atmosphere. The cell culture reagents were obtained from Gibco, Thermo Fisher Scientific, Waltham, MA, United States.
The experimental day the cells were detached from the cell cultivation dish using Trypsin-EDTA solution (Thermo Fisher Scientific, Waltham, MA, United States) incubating for 3–10 min, centrifuged, and resuspended in the electroporation buffer (Table 1). For the gene electrotransfer experiments, a concentration of 6 × 106 cells/mL was used. The cell membrane permeabilization and viability assays were performed at a concentration of 2 × 106 cells/mL.
Various electroporation buffers have been selected based on a review of various available electroporation-associated works involving gene transfer (Table 1). The buffers include standard sucrose and HEPES-based buffers for electroporation research (e.g., No. 1, 2, and 5) and commercially recommended buffers (e.g., No. 4), also less popular buffers from other studies focused on nucleofection are included to enable better repeatability and consolidation of knowledge (e.g., No. 3, 6–8) (Pakhomov et al., 2014; Parreno et al., 2015; Zhou et al., 2016; Chopra et al., 2019; Gibot et al., 2020)
2.3 Cell membrane permeabilization and detection
Cell membrane permeabilization post-electroporation was assessed with BD Accuri C6 flow cytometer (BD Biosciences, San Jose, CA, United States) using fluorescent stain Yo-Pro1 (YP1; Thermo Fisher Scientific, Waltham, MA, United States). The cells in the electroporation buffer were mixed with YP1 stain for the final stain concentration of 1 μM. The 50 μL of mixed solution were positioned between the electrodes and subjected to various EP protocols. Subsequently, they were incubated at room temperature for 3 min. Following this, 150 μL of 0.9% NaCl solution was added, and the samples were analyzed using flow cytometry. YP1 fluorescence (491⁄509) was detected using Channel FL1 (533/30 nm BPF). The results were analyzed using FlowJo software (BD, Becton Drive Franklin Lakes, NJ, United States).
2.4 Viability determination
After the EP treatment the cells were transferred into a flat-bottom 96-well plate, incubated for 10 min and growth media to the final 200 μL volume in each well was added. Additionally, untreated cells were used as a control for data normalization. After 24 h cell viability was assessed using PrestoBlue cell viability reagent (Thermo Fisher Scientific, Waltham, MA, United States). First, all the wells were gently washed twice with phosphate-buffered saline (PBS). Afterward, 90 µL of PBS and 10 µL of cell viability reagent were added to each well, followed by 2 h incubation. The fluorescence (Ex. 540/20 nm; Em. 620/40 nm) was measured using a Synergy two microplate reader and Gen5 software (BioTek, Shoreline, WA, United States).
2.5 Plasmids
Plasmids construct pEGFP-N1 (4,733 bp) carrying the gene of GFP and Luciferase-pcDNA3 [7,040 bp; Addgene plasmid #18964, a kind gift from William Kaelin, Harvard Medical School, Boston, MA, United States (Safran et al., 2006)] encoding luciferase were used. Plasmids were prepared from transfected E. Coli using the Plasmid Plus Giga Kit (Qiagen, Hilden, Germany), according to the manufacturer’s instructions and diluted in sterile H2O to a 2 mg/mL concentration.
For the long-term transfection experiments, the luciferase-pcDNA3 plasmid was restricted with Bgl II enzyme. The digestion mix with plasmid DNA was incubated for 4 h at 37°C. Afterward, the linearized plasmid was concentrated by EtOH precipitation: 0.2 volumes of CH3COONa (3 M, pH 4.8) and three volumes of 96% EtOH were added. Following a 20-min incubation on ice, the vial containing the precipitated DNA was centrifugation (31,840 × g for 15 min). Subsequently, the plasmid was subjected to a wash with 70% ethanol and centrifuged once more. Finally, the resulting pellet was resuspended in sterile water.
After 20 min of incubation on ice, the vial with precipitated DNA was centrifuged (31,840 × g, 15 min). Afterward, the plasmid was washed with 70% EtOH and centrifuged again. The pellet was resuspended in sterile H2O. The linearized plasmid (0.5 μg/μL) were used for long-term transfection.
2.6 Gene electrotransfer
Each electrotransfection sample was performed by mixing 30 μL of ice-cold cell suspension and 4 μL corresponding plasmid DNA. Right after electroporation, the cells were moved to a 48-well plate and kept on ice for 10 min. Subsequently, 0.5 mL of cell culture growth media was added, and cells were left for the 24 h incubation period.
Next day cells electrotransfected with pEGFP-N1 plasmid were detached using Trypsin-EDTA solution, centrifuged (400 × g for 5 min), resuspended with 70 μL PBS and further assessed by flow cytometry (BD Accuri C6). The transfected cells fluorescence of GFP (Ex. 491⁄509) was detected using Channel FL1 (533/30 nm BPF). A shift of fluorescence spectra and the cells in the defined gate (which was defined based on the untreated, negative control) have been interpreted as fluorescence positive (transfected), while the cells outside the gate have been interpreted as non-fluorescent (nontransfected). The gating strategy for electrotransfection analysis is presented in Figure 1. The results were analysed using FlowJo software (BD, Becton Drive Franklin Lakes, NJ, United States).
The luciferase-pcDNA3 plasmid transfected cells were detached using Trypsin-EDTA solution, centrifuged (400 × g for 5 min), resuspended with 90 μL growth media and transferred into the white 96-well plates. D-Luciferin (Promega, Madison, WI, United States) was added to the cells at a final concentration of 150 μg/mL. The luminescence of luciferase-pcDNA3 plasmid expressing firefly luciferase was evaluated using a Synergy two microplate reader and Gen5 software (BioTek, Shoreline, WA, United States).
2.7 Long-term transfection
The 4T1 cells were electrotransfected with linearized luciferase-pcDNA3 plasmid as described above. However, after electroporation 30 μL of the sample was transferred to a Petri dish where the cells were incubated for 10 min. After incubation, 12 mL of the cell culture medium was added, and a 48-h incubation period followed. G418 Sulphate (200 μg/mL; Carl Roth GmbH, Karlsruhe, Germany) was used to select transfected 4T1 cells. Only the cells resistant to G418 remained viable and were subsequently cloned by transferring them into individual 96-well plates. Then, clones were named and grown up to a week. The optimal luminescent cell clones were chosen by comparing the maximum luminescence (in RLU) observed across the clone’s complete kinetic readings. Long-term transfected cells expressing luciferase were cultured and preserved by freezing them in a medium consisting of 10% DMSO and 90% FBS. These cells were then stored in liquid nitrogen until needed. The luciferase-expressing cell line that was established was named 4T1-Luc.
2.8 In Vivo bioluminescence assay
Female Balb/c 6–8-week-old mice were bred and housed in the mouse facility of the State Research Institute Centre for Innovative Medicine (Vilnius, Lithuania). 2 × 106 4T1-Luc cells were injected subcutaneously (s.c.) on the left flank to establish tumors.
The luminescence of established tumors was imaged by IVIS Spectrum and Living Image Software (Caliper/Perkin Elmer, Akron, OH, United States) when tumor volume reached 50 mm3. First, mice intraperitoneally received 15 mg/mL D-luciferin (Promega, Madison, WI, United States) solution (150 µL/mouse) and after 10–15 min luminescence intensity was visualized. The bioluminescence is proportional to the number of live, growing 4T1-Luc cells. Images were taken when the animal was under anesthesia (3% isoflurane and oxygen gas mixture).
The consent to perform animal experiments was obtained from the State Food and Veterinary Service (approval no. G2-266), carrying out the study strictly according to the Guide for the Care and Use of Laboratory Animals.
2.9 Statistical analysis
One-way analysis of variance (ANOVA) was used to compare different treatments. Tukey’s HSD multiple comparison test for the evaluation of the difference was used when ANOVA indicated a statistically significant result (p < 0.05 was considered as statistically significant). The data were post-processed using the OriginPro software program (OriginLab Corporation, Northampton, MA, United States). Each experimental point was obtained from at least three independent experiments, and results are represented as mean ± standard deviation.
3 Results
3.1 The influence of electroporation buffer composition on GET using CHO-K1 cell line
Previously, as a proof of concept we have determined that 300–700 ns duration electric pulses can be used for efficient transfection (Novickij et al., 2020), therefore, we have selected optimal ns protocols (4 kV/cm × 300 ns × 500) and (5 kV/cm × 300 ns × 250) delivered at 1 MHz and compared the efficiency to microsecond procedures (100 µs). The influence of eight distinct buffer solutions (refer to Table 1) on gene electrotransfer efficiency in the CHO-K1 cell line, utilizing the pEGFP-N1 plasmid, is illustrated in Figure 2.
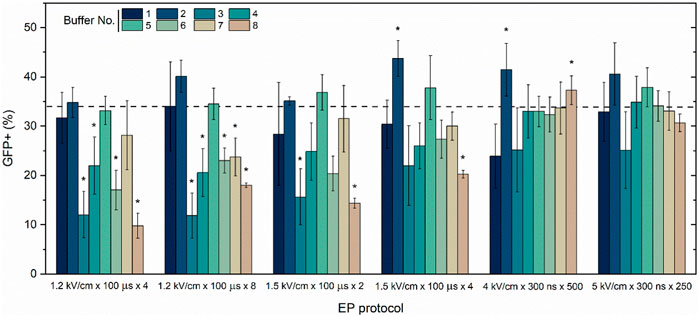
Figure 2. CHO-K1 cell line electrotransfection efficiency dependence on EP buffers composition (No. 1–8) and applied protocols with pEGFP-N1 plasmid encoding green fluorescent protein (GFP). Dashed line indicates average transfection efficacy using 1.2 kV/cm × 100 μs × 8 protocol and electroporation buffer No.1, which serves as a positive control. Asterisk (*) corresponds to statistically significant differences (p < 0.05) versus positive control.
The CHO-K1 cell line and the standard pEGFP-N1 plasmid were selected as well-described models to ensure data repeatability and the consolidation of knowledge. The transfection efficiency is highly dependent on buffer composition, while buffer (1, 2, and 5) are the most suitable for microsecond pulses. Interestingly, the influence of buffers on transfection efficiency is significantly diminished in case of nanosecond protocols. Both ns protocols result in comparable efficiency of electrotrasfection independently on the used buffer, however, there is a clear tendency that buffer No. Three is the least suitable for GET in vitro.
While successful plasmid DNA entry into the cell and protein production are important for transfection, viability is another crucial parameter characterizing treatment efficiency. Therefore, the study was further limited to the most suitable buffers (i.e., 1, 2, 5, and 7) and the toxicity of the treatment (PEF + toxicity of the plasmid) was evaluated. The results are summarized in Figure 3.
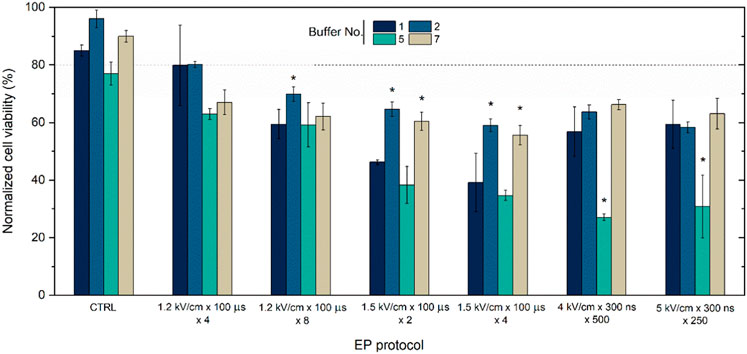
Figure 3. CHO-K1 cells viability dependence on EP buffers composition (No. 1, 2, 5, and 7) and applied protocols with pEGFP-N1 plasmid encoding green fluorescent protein (GFP). Dot line indicates transfection efficacy using 1.2 kV/cm × 100 μs × 8 protocol. CTRL refers to cells with plasmid in different buffer without electroporation treatment. Asterisk (*) corresponds to statistically significant differences (p < 0.05).
It can be seen (Figure 3) that the buffers are not toxic by themselves, however, when combined with electric field the overall toxicity of the treatment starts to rise and is also are highly dependent on buffer composition. Primarily, buffers numbered 1, 2, and seven reliably maintain the highest cellular viability levels, averaging over 60%. Conversely, buffer No. Five exhibits a negative impact on cell viability during GET, potentially decreasing to approximately 30%. The conventional electroporation solution, characterized by a sucrose-based composition (buffer No. 1), yields outcomes akin to buffers No. Two and seven when subjected to a voltage of 1.2 kV/cm or to nanosecond protocols. However, when exposed to increased amplitudes, such as 1.5 kV/cm with 100 µs pulses, a notable reduction in viability can be seen (Figure 3).
Usually, the GFP plasmid and CHO-K1 cell line serve as a model to predict electrotransfection efficiency and further to be used as protocol with other cell lines for long-term transfection. However, various cell lines exhibit different susceptibility to PEF and certain cell lines present challenges in achieving long-term transfected clones.
Therefore, to determine if the same tendency occurs with other cell lines and plasmids, the research was limited to marginal cases (i.e., buffers No. 1, 2, and 5), and long-term transfection with the 4T1 cell line was performed to develop a luminescent 4T1 cell line clone. However, firstly the efficiency of permeabilization to (YP) following PEF was characterized and compared with CHO-K1 cell line.
3.2 The influence of electroporation buffer composition on GET using 4T1 cell line
Both cell lines have been subjected to PEF and the electrotransfer of YP was evaluated. Based on data from Figure 2 it can be seen that bursts of 250 nanosecond pulses return similar results as bursts of 500 pulses, indicating the lack of rationale to use more pulses. Therefore, in order to augment the study’s versatility, we have constrained the protocols to employ 250 pulses but characterized the effects of pulse amplitude in 2.5–10 kV/cm range (standard electroporation buffer was used No. 1). The results are summarized in Figure 4.
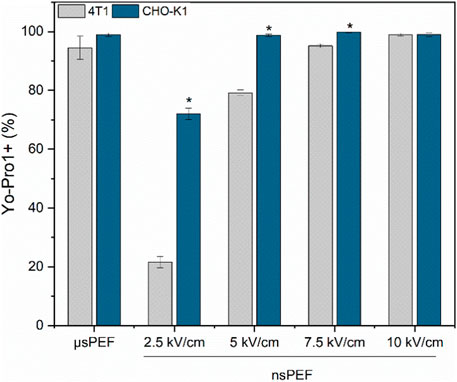
Figure 4. 4T1 (grey) and CHO-K1 (blue) cell membrane permeabilization to Yo-Pro1 fluorescent marker. Experiments were performed using No.1 EP buffer. μs PEF—1.2 kV/cm × 100 μs × 8, 1 Hz and nsPEF—2.5–10 kV/cm × 300 ns × 250, 1 MHz. Asterisk (*) corresponds to statistically significant (p < 0.05) difference.
It is apparent that the susceptibility of 4T1 cells to PEF is lower compared to the CHO-K1 cell line. For the ESOPE protocol (1.2 kV/cm x 100 µs × 8) the differences are low with both cell lines experiencing high permeabilization, although the difference is more profound in the nanosecond range. It is indicatory that the same nanosecond protocols will trigger lower GET efficiency for 4T1 cell line if the PEF parameters are not adjusted.
Further, the efficiency of GET with the Luciferase-pcDNA3 plasmid and three buffers (No. 1, 2, 5) was characterized using 1.2 kV/cm × 100 μs × 8, 1 Hz and nsPEF 5 kV/cm × 300 ns × 250, 1 MHz protocols and CHO-K1 cell line. The Luciferase-pcDNA3 plasmid was further utilized due to its applicability in developing a long-term transfected cell line (Figure 5A).
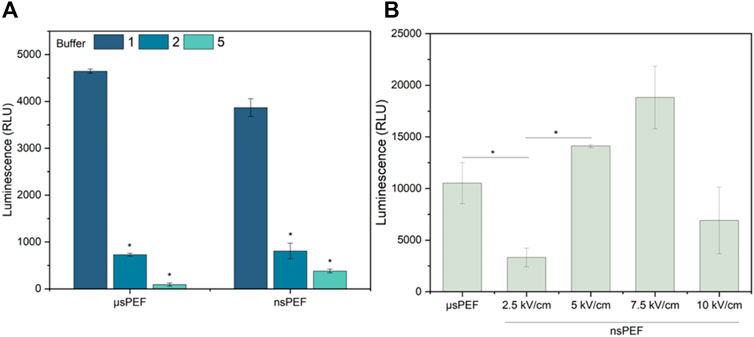
Figure 5. (A) CHO-K1 cell line electrotransfection efficiency dependence on EP buffers composition (No. 1, two and 5) with Luciferase-pcDNA3 plasmid, where µsPEF 1.2 kV/cm × 100 μs × 8, 1 Hz and nsPEF 5 kV/cm × 300 ns × 250, 1 MHz. (B) 4T1 cell line electrotransfection efficiency during µsPEF (1.2 kV/cm × 100 μs × 8, 1 Hz) and nsPEF (2.5–10 kV/cm × 300 ns × 250, 1 MHz), with Luciferase-pcDNA3 plasmid (EP buffer No.1). Asterisk (*) corresponds to statistically significant (p < 0.05) difference.
As can be seen (Figure 5A) the influence of buffer is more profound when another plasmid is used, thus, the results can hardly be superpositioned with standard GET characterization procedures using typical model plasmid (Figure 2). Unexpectedly, buffer No. 1 (standard electroporation buffer) is several-fold superior to buffer No. Two and five, which was not the case with GFP-encoding plasmid. Based on the dramatic results, the GET experiments with 4T1 cell line were limited to buffer No. 1, but GET with luciferase-pcDNA3 plasmid were performed in the whole range of PEF amplitudes (2.5–10 kV/cm). The results are summarized in Figure 5B.
As predicted, by the YP data (Figure 4) nanosecond duration (300 ns) 5 kV/cm protocol is not optimal since does not trigger saturated permeabilization. At the same time 7.5 kV/cm protocol is the best, which is in perfect agreement with permeabilization data. The 10 kV/cm protocol results in GET efficiency decline, which is attributed to significant viability loss due to irreversible electroporation. The results confirming this hypothesis are presented in Figure 6.
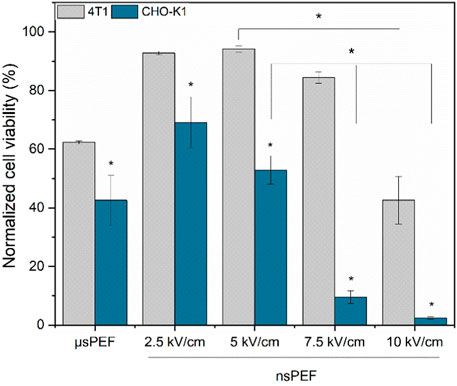
Figure 6. CHO-K1 (blue) and 4T1 (grey) normalized cell viability after electroporation with Luciferase-pcDNA3 plasmid. Both experiments were performed using No.1 EP buffer. Asterisk (*) corresponds to statistically significant (p < 0.05) difference.
As it can be seen, CHO-K1 cell line is significantly more susceptible to the treatment resulting in a rapid decline of viability following the GET procedure. It’s not the case with the 4T1 cell line, which in all cases shows higher viability when compared to CHO-K1, which again proves the hypothesis that for each specific cell line protocols should be checked and adjusted to ensure high GET efficiency.
Based on the results, the 7.5 kV/cm × 300 ns × 250 pulses bursts delivered at 1 MHz using a standard electroporation buffer (No. 1) have been selected as an optimal protocol for long-term transfection to develop a luminescent clone for in vivo research and tumor visualization, which was a success. The exemplary images of animals featuring 4T1-Luc tumors are shown in Figure 7.
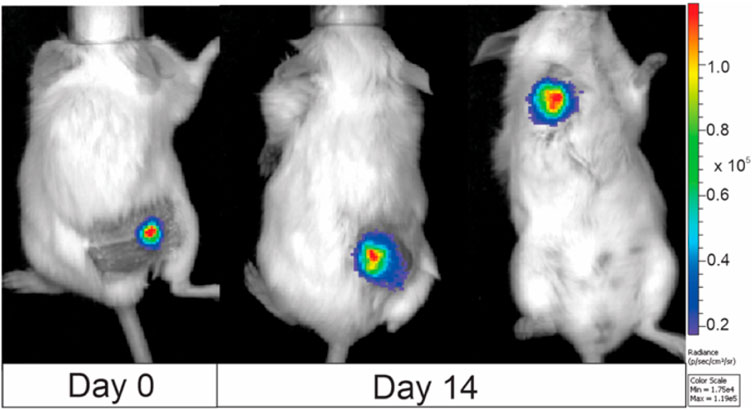
Figure 7. BALB/c murine imaging, where 4T1-luc clone was used for tumor induction. Images were taken at day 0 (when tumor volume reached 50 mm3) and day 14. The color bar on the right side of the image illustrates the correlation between color and light intensity, measured in arbitrary units (counts), for the entire animal images. Images were taken with IVIS Spectrum device and analyzed by Living Image software.
It is evidential that the developed luminescent 4T1 cell clone can be used for live tumor visualization in an in vivo model, and the dynamics of tumor development can be monitored (Refer to Figure 7). Also, the extent and localization of metastases (Figure 7; third mouse on the right-hand side) can be characterized both quantitatively (intensity of luminescence) and qualitatively. It also offers an excellent tool for the study of electrochemotherapy and other PEF-based techniques since it enables more precise electrode positioning and characterization of the effects after the treatment.
4 Discussion
The primary objective of this study was to characterize the effects of electroporation buffers on GET efficiency across both nanosecond and microsecond pulse duration ranges. Additionally, the study aimed to emphasize that using the model cell line (CHO-K1) and a standard GFP-encoding plasmid presents significant limitations when predicting GET efficiency with other plasmids or cell lines.
As already mentioned, in vitro GET efficiency depends on many factors, ranging from parametric electroporation conditions to the quality of the plasmid and cell line used (Golzio et al., 1998; Čegovnik and Novaković, 2004; Lesueur et al., 2016; Desai et al., 2017; Chopra et al., 2020; Sherba et al., 2020; Radzevičiūtė et al., 2022b). To evaluate transfection efficacy, we employed two distinct plasmids encoding different proteins: green fluorescent protein (pEGFP-N1) and luciferase (Luciferase-pcDNA3). The application of CHO-K1 cell line (Golzio et al., 1998; Čegovnik and Novaković, 2004; Desai et al., 2017), pEGFP-N1 plasmid (Chopinet et al., 2013; Novickij et al., 2020), and microsecond duration EP protocols was selected based on the literature as most commonly used in the in vitro GET context, serving as standard reference for efficiency comparison.
First, we tested the transfection efficacy of the standard CHO-K1 cell line and with pEGFP-N1 plasmid encoding green fluorescent protein (GFP) using eight different composition EP buffers and both microsecond and nanosecond range EP protocols. The highest transfection efficiency was achieved when No. 1, 2, 5, and 7 composition buffers were used. This can be attributed to the presence of divalent cations (Ca2+ and Mg2+), which are known to have a significant impact on GET efficiency (Neumann et al., 1996; Hristova et al., 1997; Haberl et al., 2013; Chopra et al., 2020). Reported electrotransfection efficacy results of GFP-encoding plasmid with the CHO-K1 cell line and standard microsecond EP protocol are in good agreement with existing knowledge (Chopinet et al., 2013; Novickij et al., 2020). Regarding sub-microsecond duration EP for gene electrotransfer, based on available knowledge, our group was the first and only one to apply high-frequency sub-microsecond protocols, and the reported data here are in agreement with our previously conducted studies (Novickij et al., 2020; Radzevičiūtė et al., 2022a). Higher transfection efficiency of the GFP protein-encoding plasmid was reached when sub-microsecond duration protocols (nsPEF) were used. Chopinet and colleagues combined classical microsecond GET protocols with nsPEFs, and their study results revealed that neither the percentage of electrotransfected cells nor the amount of GFP expressed was increased by combination with short duration nsPEF (10, 15 and 18 ns) (Chopinet et al., 2013).
Furthermore, EP buffers were narrowed down to those most effective, and we tested how they influence cell viability post-treatment, as this is another very important characteristic in GET context. Studies showed that EP No. Five buffer composition negatively affects cell viability, although it achieves sufficient electrotransfection rates. These results might be explained due to the absence of Ca2+ ions in the No. Five EP buffer composition, which is reported to play a crucial role (Suzuki et al., 2003; Ciobanu et al., 2018; Shi et al., 2022; Li and Shaw, 2023). Additionally, we demonstrated that the buffers containing the pEGFP-N1 plasmid are not inherently toxic to the cells. This is a favorable outcome, considering that only the plasmid’s toxicity to the cells has been previously reported (Lesueur et al., 2016). However, when combined with an PEF, the overall toxicity of the treatment increases significantly, and this increase is highly dependent on the buffer composition (Figure 3). It might be related to.
Nevertheless, to develop a luminescent murine cancer cell line for in vivo studies, we carried out electrotransfection protocol optimization with the Luciferase-pcDNA3 plasmid encoding luciferase protein. First, the luciferase-pcDNA3 plasmid electrotransfection efficiency of the model CHO-K1 cell line with three different composition EP buffers was tested (No. 1, 2, and 5) (Figure 4A). It should be noted that standard electroporation buffers (No. 1, 2, and 5) showed the best efficacy of transfection and competitive viability results when compared to commercial or other buffers involved in the study. Other studies showed that significantly higher transfection efficiency can be achieved when sucrose, as the osmotic balancing agent, in EP buffer is used (No.1). Our findings align with existing knowledge in the field (Sherba et al., 2020). The same electrotransfection tendencies can be observed regardless of the pulsed electric field protocol used (µsPEF or nsPEF). Furthermore, electrotransfection studies with murine 4T1 cell line were narrowed to one EP buffer composition but expanded in terms of PEFs amplitude flexibility. As seen in Figure 4B, better transfection efficiency and higher luminescence were achieved compared to standard GET protocols when applying a high-frequency nanosecond electroporation protocol with a higher amplitude (7.5 kV/cm). In the case of the highest amplitude (10 kV/cm), diminished luminescence can be attributed to potential negative effects on cell viability. Previously we reported that electrotransfection efficiency as well as cell viability is directly related to the used PEFs amplitude (Novickij et al., 2020).
However, to determine the optimal long-term transfection protocol, not only does the transfection efficacy need to be elevated, but also high permeabilization and cell viability must be ensured (Figure 5). Therefore, long-term transfection was performed utilizing nanosecond duration (7.5 kV/cm × 300 ns × 250, 1 MHz) EP protocol that resulted in a highly luminescent clone of the 4T1 cell line, which was subsequently tested in an in vivo murine model. As illustrated in Figure 7, even 14 days after the tumor reaches a volume of 50 mm³, the luminescence of the cancerous cells, as well as the presence of metastases, can still be detected. To the best of our knowledge, it’s the first murine cancer cell line study to confirm the successful applicability of high frequency nsPEFs in long-term electrotransfection context. The scientific data confirm the ability to long-term transfect the 4T1 mouse mammary tumor cell line with a luciferase-expressing plasmid; however, only when applying viral transfection methodology (Tao et al., 2008; Baklaushev et al., 2015; Baklaushev et al., 2017). Tao and colleagues characterized the luciferase-expressing 4T1 cell line transfected with vectors in a female BALB/c mice model by conducting a 6-week study of primary tumor growth and metastasis. As in the case of our study, the transfected 4T1 cell line allowed for the assessment of tumor growth and metastasis progression (Tao et al., 2008). Additionally, we have previously proved that high-frequency nanosecond duration EP protocols can be used for long-term transfection, ensuring significantly higher luminescence compared to standard GET protocol (μsPEF). However, our previous study focused on CHO-K1 cell line, which is the most commonly utilized platform for recombinant protein expression (Radzevičiūtė et al., 2022b), while most of the time transfection of cancerous cell lines is more challenging due to genetic instability. Thus, we demonstrate an efficient electrotransfection methodology for mammalian cells using high-frequency pulsed electric fields (PEFs), which represents a significant enhancement over standard existing GET methodologies.
5 Conclusion
In summary it has been demonstrated that the properties of the electroporation buffer, such as ionic composition, used during EP treatment, have a significant impact on gene electrotransfer efficiency. Additionally, we showed that sub-microsecond duration protocols reached higher, or on-par transfection efficiency and cell viability when compared to standard microsecond modality. As a proof of concept, we established long-term transfected luminescent cell line using high-frequency nanosecond EP. Also, the developed murine 4T1-Luc cell line successfully validated its suitability for utilization in an in vivo model for tumor and metastasis evaluation.
Data availability statement
The original contributions presented in the study are included in the article/Supplementary Material, further inquiries can be directed to the corresponding authors.
Ethics statement
The animal study was approved by The consent to perform animal experiments was obtained from the State Food and Veterinary Service (approval no. G2-266), carrying out the study strictly according to the Guide for the Care and Use of Laboratory Animals. The study was conducted in accordance with the local legislation and institutional requirements.
Author contributions
ER-V: Conceptualization, Data curation, Formal Analysis, Investigation, Methodology, Project administration, Validation, Visualization, Writing–original draft, Writing–review and editing. JG: Formal Analysis, Investigation, Methodology, Validation, Writing–original draft, Writing–review and editing. AB: Data curation, Formal Analysis, Investigation, Methodology, Visualization, Writing–original draft, Writing–review and editing. AS: Investigation, Methodology, Validation, Visualization, Writing–original draft, Writing–review and editing. AZ: Investigation, Methodology, Validation, Visualization, Writing–original draft, Writing–review and editing. BL: Formal Analysis, Investigation, Validation, Visualization, Writing–original draft, Writing–review and editing. VM-P: Data curation, Formal Analysis, Investigation, Resources, Validation, Visualization, Writing–original draft, Writing–review and editing. EM: Formal Analysis, Investigation, Methodology, Writing–original draft, Writing–review and editing. PM: Visualization, Writing–original draft, Writing–review and editing, Investigation, Methodology. JK: Conceptualization, Data curation, Resources, Supervision, Validation, Writing–original draft, Writing–review and editing. VN: Funding acquisition, Supervision, Validation, Writing–original draft, Writing–review and editing.
Funding
The author(s) declare that financial support was received for the research, authorship, and/or publication of this article. The research was funded by Research Council of Lithuania, Grant No. S-PD-24-5.
Conflict of interest
The authors declare that the research was conducted in the absence of any commercial or financial relationships that could be construed as a potential conflict of interest.
Publisher’s note
All claims expressed in this article are solely those of the authors and do not necessarily represent those of their affiliated organizations, or those of the publisher, the editors and the reviewers. Any product that may be evaluated in this article, or claim that may be made by its manufacturer, is not guaranteed or endorsed by the publisher.
References
Arkhangelsky, E., Steubing, B., Ben-Dov, E., Kushmaro, A., and Gitis, V. (2008). Influence of pH and ionic strength on transmission of plasmid DNA through ultrafiltration membranes. Desalination 227 (1–3), 111–119. doi:10.1016/J.DESAL.2007.07.017
Baklaushev, V. P., Grinenko, N. F., Yusubalieva, G. M., Abakumov, M. A., Gubskii, I. L., Cherepanov, S. A., et al. (2015). Modeling and integral X-ray, optical, and MRI visualization of multiorgan metastases of orthotopic 4T1 breast carcinoma in BALB/c mice. Bull. Exp. Biol. Med. 158 (4), 581–588. doi:10.1007/s10517-015-2810-3
Baklaushev, V. P., Kilpeläinen, A., Petkov, S., Abakumov, M. A., Grinenko, N. F., Yusubalieva, G. M., et al. (2017). Luciferase expression allows bioluminescence imaging but imposes limitations on the orthotopic mouse (4T1) model of breast cancer. Sci. Rep. 7 (1), 7715. doi:10.1038/s41598-017-07851-z
Čegovnik, U., and Novaković, S. (2004). Setting optimal parameters for in vitro electrotransfection of B16F1, SA1, LPB, SCK, L929 and CHO cells using predefined exponentially decaying electric pulses. Bioelectrochemistry 62 (1), 73–82. doi:10.1016/J.BIOELECHEM.2003.10.009
Cemazar, M., Golzio, M., Sersa, G., Hojman, P., Kranjc, S., Mesojednik, S., et al. (2009). Control by pulse parameters of DNA electrotransfer into solid tumors in mice. Gene Ther. 16 (5), 635–644. doi:10.1038/gt.2009.10
Cervia, L. D., and Yuan, F. (2018). Current progress in electrotransfection as a nonviral method for gene delivery. Mol. Pharm. 15 (9), 3617–3624. doi:10.1021/acs.molpharmaceut.8b00207
Chattergoon, M., Boyer, J., and Weiner, D. B. (1997). Genetic immunization: a new era in vaccines and immune therapeutics. FASEB J. 11 (10), 753–763. doi:10.1096/FASEBJ.11.10.9271360
Chopinet, L., Batista-Napotnik, T., Montigny, A., Rebersek, M., Teissié, J., Rols, M. P., et al. (2013). Nanosecond electric pulse effects on gene expression. J. Membr. Biol. 246 (11), 851–859. doi:10.1007/S00232-013-9579-Y
Chopra, S., Ruzgys, P., Jakutaviciute, M., Rimgailaite, A., Navickaitė, D., and Satkauskas, S. (2019). A novel method for controlled gene expression via combined Bleomycin and Plasmid DNA Electrotransfer. Int. J. Mol. Sci. 20, 4047. doi:10.3390/ijms20164047
Chopra, S., Ruzgys, P., Maciulevičius, M., Jakutavičiūtė, M., and Šatkauskas, S. (2020). Investigation of plasmid DNA delivery and cell viability dynamics for optimal cell electrotransfection in vitro. Appl. Sci. Switz. 10 (17), 6070. doi:10.3390/app10176070
Ciobanu, F., Golzio, M., Kovacs, E., and Teissié, J. (2018). Control by low levels of calcium of mammalian cell membrane electropermeabilization. J. Membr. Biol. 251 (2), 221–228. doi:10.1007/S00232-017-9981-Y
Cukjati, D., Batiuskaite, D., André, F., Miklavčič, D., and Mir, L. M. (2007). Real time electroporation control for accurate and safe in vivo non-viral gene therapy. Bioelectrochemistry 70 (2), 501–507. doi:10.1016/J.BIOELECHEM.2006.11.001
Desai, P., Yagnik, B., Sharma, D., Khan, A., Desai, N., and Padh, H. (2017). Transfecting CHO-K1 cells: comparison of CaPO4, electroporation and lipoplex method with in-house prepared polyplex. OMICS Int. 79 (4), 655–662. doi:10.4172/PHARMACEUTICAL-SCIENCES.1000276
Fewell, J. G., Matar, M., Slobodkin, G., Han, S. O., Rice, J., Hovanes, B., et al. (2005). Synthesis and application of a non-viral gene delivery system for immunogene therapy of cancer. J. Control. Release 109 (1–3), 288–298. doi:10.1016/J.JCONREL.2005.09.024
Flanagan, M., Gimble, J. M., Yu, G., Wu, X., Xia, X., Hu, J., et al. (2011). Competitive electroporation formulation for cell therapy. Cancer Gene Ther. 18 (8), 579–586. doi:10.1038/cgt.2011.27
Gibot, L., Montigny, A., Baaziz, H., Fourquaux, I., Audebert, M., and Rols, M. P. (2020). Calcium delivery by electroporation induces in vitro cell death through mitochondrial dysfunction without DNA damages. Cancers 12 (2), 425. doi:10.3390/cancers12020425
Golberg, A., and Rubinsky, B. (2012). Towards electroporation based treatment planning considering electric field induced muscle contractions. Technol. Cancer Res. Treat. 11 (2), 189–201. doi:10.7785/TCRT.2012.500249
Golzio, M., Mora, M. P., Raynaud, C., Delteil, C., Teissié, J., and Rols, M. P. (1998). Control by osmotic pressure of voltage-induced permeabilization and gene transfer in mammalian cells. Biophysical J. 74 (6), 3015–3022. doi:10.1016/S0006-3495(98)78009-9
Golzio, M., Teissié, J., and Rols, M. P. (2002). Direct visualization at the single-cell level of electrically mediated gene delivery. Proc. Natl. Acad. Sci. 99 (3), 1292–1297. doi:10.1073/PNAS.022646499
Gong, X., Chen, Z., Hu, J. J., and Liu, C. (2022). Advances of electroporation-related therapies and the Synergy with immunotherapy in cancer treatment. Vaccines 10 (11), 1942–2018. doi:10.3390/vaccines10111942
Guo, S., Jackson, D. L., Burcus, N. I., Chen, Y. J., Xiao, S., and Heller, R. (2014). Gene electrotransfer enhanced by nanosecond pulsed electric fields. Mol. Ther. Methods and Clin. Dev. 1, 14043. doi:10.1038/MTM.2014.43
Haberl, S., Kandušer, M., Flisar, K., Hodžić, D., Bregar, V. B., Miklavčič, D., et al. (2013). Effect of different parameters used for in vitro gene electrotransfer on gene expression efficiency, cell viability and visualization of plasmid DNA at the membrane level. J. Gene Med. 15 (5), 169–181. doi:10.1002/JGM.2706
Haberl, S., Miklavčič, D., and Pavlin, M. (2010). Effect of Mg ions on efficiency of gene electrotransfer and on cell electropermeabilization. Bioelectrochemistry 79 (2), 265–271. doi:10.1016/J.BIOELECHEM.2010.04.001
Henshaw, J. W., and Yuan, F. (2012). Field distribution and DNA transport in solid tumors during electric field-mediated gene delivery. J. Pharm. Sci. 101 (7), 2271–2280. doi:10.1002/jps
Hristova, N. I., Tsoneva, I., and Neumann, E. (1997). Sphingosine-mediated electroporative DNA transfer through lipid bilayers. FEBS Lett. 415 (1), 81–86. doi:10.1016/S0014-5793(97)01097-1
Kandušer, M., Miklavčič, D., and Pavlin, M. (2009). Mechanisms involved in gene electrotransfer using high- and low-voltage pulses — an in vitro study. Bioelectrochemistry 74 (2), 265–271. doi:10.1016/J.BIOELECHEM.2008.09.002
Karagöz, U., Kotmakçı, M., Akbaba, H., Çetintaş, V. B., and Kantarcı, G. (2018). Preparation and characterization of non-viral gene delivery systems with pEGFP-C1 Plasmid DNA. Braz. J. Pharm. Sci. 54 (1), 1–11. doi:10.1590/s2175-97902018000100265
Kotnik, T., Rems, L., Tarek, M., and Miklavčič, D. (2019). Membrane electroporation and electropermeabilization: mechanisms and models. Annu. Rev. Biophys. 48, 63–91. doi:10.1146/ANNUREV-BIOPHYS-052118-115451
Kumar, A. R. K., Shou, Y., Chan, B., L., K., and Tay, A. (2021). Materials for improving immune cell transfection. Adv. Mater. 33 (21), 2007421. doi:10.1002/ADMA.202007421
Lambricht, L., Lopes, A., Kos, S., Sersa, G., Préat, V., and Vandermeulen, G. (2015). Clinical potential of electroporation for gene therapy and DNA vaccine delivery. Expert Opin. Drug Deliv. 5247, 295–310. doi:10.1517/17425247.2016.1121990
Lesueur, L. L., Mir, L. M., and André, F. M. (2016). Overcoming the specific toxicity of large plasmids electrotransfer in primary cells in vitro. Mol. Ther. 5 (3), e291. doi:10.1038/MTNA.2016.4
Li, S., and Huang, L. (2000). Nonviral gene therapy: promises and challenges. Gene Ther. 7 (1), 31–34. doi:10.1038/sj.gt.3301110
Li, Y., Wang, J., Satterle, A., Wu, Q., Wang, J., and Liu, F. (2012). Gene transfer to skeletal muscle by site-specific delivery of electroporation and ultrasound. Biochem. Biophysical Res. Commun. 424 (2), 203–207. doi:10.1016/j.bbrc.2012.06.090
Li, Z. W., and Shaw, G. S. (2023). Role of calcium-sensor proteins in cell membrane repair. Biosci. Rep. 43 (2), 20220765. doi:10.1042/BSR20220765
Miklavčič, D., Serša, G., Brecelj, E., Gehl, J., Soden, D., Bianchi, G., et al. (2012). Electrochemotherapy: technological advancements for efficient electroporation-based treatment of internal tumors. Med. Biol. Eng. Comput. 50 (12), 1213–1225. doi:10.1007/S11517-012-0991-8
Mir, L. M. (2009). Nucleic acids electrotransfer-based gene therapy (electrogenetherapy): past, current, and future. Mol. Biotechnol. 43 (2), 167–176. doi:10.1007/s12033-009-9192-6
Neumann, E., Kakorin, S., Tsoneva, I., Nikolova, B., and Tomov, T. (1996). Calcium-mediated DNA adsorption to yeast cells and kinetics of cell transformation by electroporation. Biophysical J. 71, 868–877. doi:10.1016/S0006-3495(96)79288-3
Nomura, T., Nishimura, Y., Gotoh, H., and Ono, K. (2016). Rapid and efficient gene delivery into the adult mouse brain via focal electroporation. Sci. Rep. 6 (1), 29817–29819. doi:10.1038/srep29817
Novickij, V., Balevičiūtė, A., Ruzgys, P., Šatkauskas, S., Novickij, J., Zinkevičienė, A., et al. (2020). Sub-microsecond electrotransfection using new modality of high frequency electroporation. Bioelectrochemistry 136, 107594. doi:10.1016/j.bioelechem.2020.107594
Novickij, V., Grainys, A., Butkus, P., Tolvaišienė, S., Švedienė, J., Paškevičius, A., et al. (2016). High-frequency submicrosecond electroporator. Taylor Francis 30 (3), 607–613. doi:10.1080/13102818.2016.1150792
Pakhomov, A. G., Semenov, I., Xiao, S., Pakhomova, O. N., Gregory, B., Schoenbach, K. H., et al. (2014). Cancellation of cellular responses to nanoelectroporation by reversing the stimulus polarity. Cell. Mol. Life Sci. 71 (22), 4431–4441. doi:10.1007/s00018-014-1626-z
Parreno, J., Delve, E., Andrejevic, K., Paez-Parent, S., Wu, P. h., and Kandel, R. (2015). Efficient, low-cost nucleofection of passaged chondrocytes. CARTILAGE 7 (1), 82–91. doi:10.1177/1947603515609399
Potter, H., and Heller, R. (2003). Transfection by electroporation. Curr. Protoc. Mol. Biol. 9, Unit 9.3. doi:10.1002/0471142727.mb0903s92
Prud’homme, G., Glinka, Y., Khan, A., and Draghia-Akli, R. (2006). Electroporation-enhanced nonviral gene transfer for the prevention or treatment of immunological, endocrine and neoplastic diseases. Curr. Gene Ther. 6 (2), 243–273. doi:10.2174/156652306776359504
Radzevičiūtė, E., Malyško-Ptašinskė, V., Kulbacka, J., Rembiałkowska, N., Novickij, J., Girkontaitė, I., et al. (2022a). Nanosecond electrochemotherapy using bleomycin or doxorubicin: influence of pulse amplitude, duration and burst frequency. Bioelectrochemistry 148, 108251. doi:10.1016/j.bioelechem.2022.108251
Radzevičiūtė, E., Malyško-Ptašinskė, V., Novickij, J., and Girkontaitė, I. (2022b). Transfection by electroporation of cancer and primary cells using nanosecond and microsecond electric fields. Pharmaceutics 14 (6), 1239. doi:10.3390/pharmaceutics14061239
Radzevičiūtė-Valčiukė, E., Gečaitė, J., Želvys, A., Zinkevičienė, A., Žalnėravičius, R., Malyško-Ptašinskė, V., et al. (2023). Improving NonViral gene delivery using MHz bursts of nanosecond pulses and gold nanoparticles for electric field amplification. Pharmaceutics 15 (4), 1178. doi:10.3390/pharmaceutics15041178
Rakoczy, K., Kisielewska, M., Sędzik, M., Jonderko, L., Celińska, J., Sauer, N., et al. (2022). Electroporation in clinical applications—the potential of gene electrotransfer and electrochemotherapy. Appl. Sci. Switz. 12 (21), 10821. doi:10.3390/app122110821
Rodaite-Riseviciene, R., Saule, R., Snitka, V., and Saulis, G. (2014). Release of iron ions from the stainless steel anode occurring during high-voltage pulses and its consequences for cell electroporation technology. IEEE Trans. Plasma Sci. 42 (1), 249–254. doi:10.1109/TPS.2013.2287499
Ruzgys, P., Novickij, V., Novickij, J., and Šatkauskas, S. (2018). Nanosecond range electric pulse application as a non-viral gene delivery method: proof of concept. Sci. Rep. 8 (1), 15502–15508. doi:10.1038/s41598-018-33912-y
Safran, M., Kim, W. Y., O'Connell, F., Flippin, L., Günzler, V., Horner, J. W., et al. (2006). Mouse model for noninvasive imaging of HIF prolyl hydroxylase activity: assessment of an oral agent that stimulates erythropoietin production. Proc. Natl. Acad. Sci. U. S. A. 103 (1), 105–110. doi:10.1073/PNAS.0509459103
Sherba, J. J., Hogquist, S., Lin, H., Shan, J. W., Shreiber, D. I., and Zahn, J. D. (2020). The effects of electroporation buffer composition on cell viability and electro-transfection efficiency. Sci. Rep. 10 (1), 3053–3059. doi:10.1038/s41598-020-59790-x
Shi, J., Han, T., Yu, A. C., and Qin, P. (2022). Faster calcium recovery and membrane resealing in repeated sonoporation for delivery improvement. J. Control. Release 352, 385–398. doi:10.1016/J.JCONREL.2022.10.027
Sukharev, S. I., Klenchin, V., Serov, S., Chernomordik, L., and Chizmadzhev, Y. A. (1992). Electroporation and electrophoretic DNA transfer into cells. The effect of DNA interaction with electropores. Biophys. J. 63 (5), 1320–1327. doi:10.1016/S0006-3495(92)81709-5
Suzuki, T., Tsunekawa, J., Murai, A., and Muramatsu, T. (2003). Effect of CaCl2 concentration on the rate of foreign gene transfer and expression by in vivo electroporation in the mouse ovary. Int. J. Mol. Med. 12 (3), 365–368. doi:10.3892/ijmm.12.3.365
Tao, K., Fang, M., Alroy, J., and Sahagian, G. G. (2008). Imagable 4T1 model for the study of late stage breast cancer. BMC Cancer 8, 228. doi:10.1186/1471-2407-8-228
Tullis, R., and Price, P. A. (1974). The effect of calcium and magnesium on the ultraviolet Spectrum of bovine pancreatic deoxyribonuclease A. J. Biol. Chem. 249 (16), 5033–5037. doi:10.1016/S0021-9258(19)42324-7
Tylewicz, U. (2020). How does pulsed electric field work? Pulsed electric fields to obtain healthier and sustainable Food for tomorrow. Elsevier. doi:10.1016/B978-0-12-816402-0.00001-X
Wong, T. K., and Neumann, E. (1982). Electric field mediated gene transfer. Biochem. Biophysical Res. Commun. 107 (2), 584–587. doi:10.1016/0006-291X(82)91531-5
Zampaglione, I., Arcuri, M., Cappelletti, M., Ciliberto, G., Perretta, G., Nicosia, A., et al. (2005). In vivo DNA gene electro-transfer: a systematic analysis of different electrical parameters. J. Gene Med. 7 (11), 1475–1481. doi:10.1002/JGM.774
Zhao, J., Chen, S., Zhu, L., Zhang, L., Liu, J., Xu, D., et al. (2021). Antitumor effect and immune response of nanosecond pulsed electric fields in pancreatic cancer. Front. Oncol. 10, 621092–621113. doi:10.3389/fonc.2020.621092
Zhao, Y. G., Lu, H. l., Peng, J. l., and Xu, Y. h. (2006). Inhibitory effect of Ca2+ on in vivo gene transfer by electroporation. Acta Pharmacol. Sin. 27 (3), 307–310. doi:10.1111/j.1745-7254.2006.00280.x
Keywords: transfection, electroporation, GFP, luminescence, in vitro, in vivo, 4T1
Citation: Radzevičiūtė-Valčiukė E, Gečaitė J, Balevičiūtė A, Szewczyk A, Želvys A, Lekešytė B, Malyško-Ptašinskė V, Mickevičiūtė E, Malakauskaitė P, Kulbacka J and Novickij V (2024) Effects of buffer composition and plasmid toxicity on electroporation-based non-viral gene delivery in mammalian cells using bursts of nanosecond and microsecond pulses. Front. Bioeng. Biotechnol. 12:1430637. doi: 10.3389/fbioe.2024.1430637
Received: 10 May 2024; Accepted: 17 June 2024;
Published: 10 July 2024.
Edited by:
Meiyappan Lakshmanan, Indian Institute of Technology Madras, IndiaReviewed by:
Irene Maier, Medical University of Vienna, AustriaPablo Di Giusto, University of California, United States
Copyright © 2024 Radzevičiūtė-Valčiukė, Gečaitė, Balevičiūtė, Szewczyk, Želvys, Lekešytė, Malyško-Ptašinskė, Mickevičiūtė, Malakauskaitė, Kulbacka and Novickij. This is an open-access article distributed under the terms of the Creative Commons Attribution License (CC BY). The use, distribution or reproduction in other forums is permitted, provided the original author(s) and the copyright owner(s) are credited and that the original publication in this journal is cited, in accordance with accepted academic practice. No use, distribution or reproduction is permitted which does not comply with these terms.
*Correspondence: Eivina Radzevičiūtė-Valčiukė, ZWl2aW5hLnJhZHpldmljaXV0ZUBpbWNlbnRyYXMubHQ=; Vitalij Novickij, dml0YWxpai5ub3ZpY2tpakB2aWxuaXVzdGVjaC5sdA==