- 1Trauma Emergency Center, The Third Hospital of Hebei Medical University, Shijiazhuang, China
- 2Key Laboratory of Biomechanics of Hebei Province, Orthopaedic Research Institution of Hebei Province, Shijiazhuang, China
Bone defects can arise from trauma or pathological factors, resulting in compromised bone integrity and the loss or absence of bone tissue. As we are all aware, repairing bone defects is a core problem in bone tissue engineering. While minor bone defects can self-repair if the periosteum remains intact and normal osteogenesis occurs, significant defects or conditions such as congenital osteogenesis imperfecta present substantial challenges to self-healing. As research on mesenchymal stem cell (MSC) advances, new fields of application have emerged; however, their application in orthopedics remains one of the most established and clinically valuable directions. This review aims to provide a comprehensive overview of the research progress regarding MSCs in the treatment of diverse bone defects. MSCs, as multipotent stem cells, offer significant advantages due to their immunomodulatory properties and ability to undergo osteogenic differentiation. The review will encompass the characteristics of MSCs within the osteogenic microenvironment and summarize the research progress of MSCs in different types of bone defects, ranging from their fundamental characteristics and animal studies to clinical applications.
1 Introduction
With the modernization of society, skeletal injuries are becoming increasingly prevalent in advanced industrialized countries. An analysis of Global Burden of Disease (GBD) data indicates that musculoskeletal conditions are the leading contributors to disability worldwide. Bone defects are one of the important factors causing musculoskeletal conditions (Cieza et al., 2021). There are two types of bone defects: traumatic defects, which result from accidents, and pathological defects, which arise from bone diseases. When bone defects are extensive or the skeletal microenvironment is imbalanced, delayed union or nonunion would occur (Impieri et al., 2024). At present, the primary clinical methods for treating large bone defects include bone transplantation, Ilizarov technology, guided bone regeneration (GBR), and artificial bone substitute material transplantation (Semaya et al., 2016; Kim and Ku, 2020; Alford et al., 2021; Singh et al., 2021) (Figure 1). The above methods have their own advantages and disadvantages. As a primary treatment method, bone grafting with autologous or allogeneic bone can have certain effects on bone defect treatment. However, several notable limitations still exist, containing a shortage of donors, lengthy operation times, and complications (such as a high rate of bone resorption, risks of infection, and the possibility of immune rejection) during the procedure (Ferraz, 2023; Sivakumar et al., 2023). Continued research and innovation are vital to overcoming these obstacles to improve treatment status of bone defect. These challenges can also significantly impact the short-term and long-term outcome for bone defect patients.
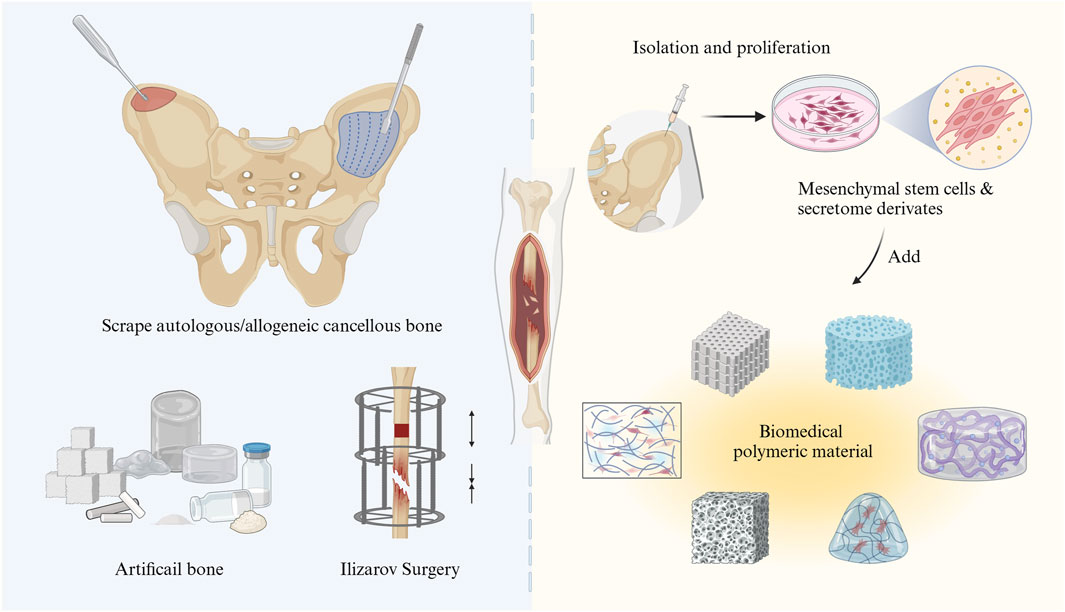
Figure 1. Schematic illustration of the treatment of bone tissue defects through traditional surgical strategies (left) and bone tissue engineering with MSCs (right).
The development of bone tissue engineering has introduced innovative ideas and strategies for the repair of tissue defects. Stem cell therapy is increasingly recognized as a promising approach in organic tissue repair, either when used alone or in combination with other treatment modalities (for instance, biomaterials and/or pharmaceuticals) (Riester et al., 2020). Mesenchymal stem cell (MSCs) are frequently utilized in both basic and clinical research due to their capacity for self-renew, repair, and differentiation into a wide range of cell types, such as osteoblasts, adipocytes, chondroblasts, neuroblasts, liver cells, and other endo- and ectodermal cells (Lee et al., 2004; Dominici et al., 2006; Bianco, 2014; Sheng, 2015). In the bone microenvironment, homing MSCs and bone marrow-derived mesenchymal stem cells (BM-MSCs) are of considerable interest in the maintenance of bone homeostasis. This interest is largely due to their key biological characteristics, including differentiation, secretion, and immunoregulation (Bruder et al., 1994; Chai et al., 2022). When a bone injury occurs, MSCs can differentiate into osteoblasts, which are the main local cells and one of the types of cells important for repairing bone damage in the bone microenvironment. Consequently, MSCs are considered ideal seeding cells for tissue engineering and regenerative medicine and have become promising candidates in the treatment of bone-related diseases. Unlike traditional surgical methods, MSC therapy leverages the regenerative potential of stem cells to culture and implant bone-like tissue in vitro, thereby enhancing the repair of bone defect areas and accelerating bone tissue regeneration. Furthermore, MSC therapy generally presents a lower risk of immune rejection and results in a shorter recovery time, which can reduce the incidence of complications (Aggarwal and Pittenger, 2005; Yi et al., 2022). These advantages, including enhanced biocompatibility, improved osteogenic potential, and the ability to support tissue regeneration, position MSC-based bone tissue engineering therapy as a promising and innovative treatment option for patients with bone defects or injuries (Figure 1). A comprehensive overview of the latest advancements and realistic applications of MSCs is therefore essential. This paper summarizes the use of MSCs in bone defect therapy by dividing the discussion into two parts: the fundamental roles and mechanisms of MSCs and their related applications in both basic and clinical research. Finally, the application prospects and future research directions for MSCs are explored.
2 Basic characteristics and cell markers of MSCs
MSCs have traditionally been considered as multipotent stem cells characterized by a spindle-shaped morphology and adherent properties (Dominici et al., 2006). They were discovered during bone marrow transplantation (Friedenstein et al., 1968) and are known to express specific surface antigens, including CD73 (with a positive rate >95%), CD90, and CD105, and to lack expression of hematopoietic cell-associated surface antigens (including CD45, CD34, CD11 or CD14, CD79a or CD19, and HLA-DR), in accordance with the identification criteria proposed by the International Society for Cell Therapy (ISCT) (Dominici et al., 2006).
Given the absence of major histocompatibility complex (MHC), Fas ligand (apoptosis-mediating surface antigen Fas) and T-cell costimulatory molecules, MSCs are not easily recognized by immune cells (Najar et al., 2012; Oh et al., 2022). Studies have found that human MSCs typically express low levels of MHC-I molecules and do not express MHC-II molecules or costimulatory molecules (such as CD86, CD80, CD40, and CD40L), which makes them excellent candidates for cell therapy (Chamberlain et al., 2007; Lechanteur et al., 2016; Keshavarz Shahbaz et al., 2022). Recent research further stated that MSCs enable the evasion of natural killer (NK) cell-mediated cytotoxicity and exert immunosuppressive activity with low levels of MHC-I molecules (Oh et al., 2022). However, in the presence of pro-inflammatory cytokines, such as interferon-gamma (IFN-γ), MHC-II molecules will be upregulated on MSCs (Cassano et al., 2018). MHC-II molecules are mainly involved in presenting antigens to helper T cells, which are essential for coordinating immune responses (Maizels and Withers, 2014). This upregulation of MHC-II allows MSCs to effectively present antigens to helper T cells and activate immune responses when necessary (Omoto et al., 2014). Studies have demonstrated that the intratumoral implantation of MSCs, combined with peripheral IFN-γ immunotherapy can cure glioma-bearing rats (Ströjby et al., 2014). Moreover, MSCs also exhibit immune rejection during allograft when MHC-I and MHC-II of the recipient mice are mismatched (Eliopoulos et al., 2005). Considering these points, the immune interactions of MSCs are complex and dependent on various factors. However, the unique MHC expression profile, characterized by low levels of MHC-I and inducible MHC-II expression, endows them with immunomodulatory properties that can be harnessed for therapeutic purposes.
MSCs can be isolated from many tissues, such as bone marrow, adipose tissue, muscle, synovial fluid (Alhadlaq and Mao, 2004), umbilical cord, chorion, placenta, amnion (Asgari et al., 2017), dental pulp, or gingiva (Paganelli et al., 2021). At present, BM-MSCs, adipose-derived mesenchymal stem cells (AD-MSCs), umbilical cord mesenchymal stem cells (UC-MSCs), amniotic membrane mesenchymal stem cells (AM-MSCs), and placental mesenchymal stem cells (PMSCs) are commonly used in the study of bone injury therapy. Nonetheless, MSC-based therapies encounter several challenges, including limited self-renewal capacities, variability in the availability of donor-derived MSCs, and the financial implications associated with donor screening processes (Zhou et al., 2021). As an alternative approach, MSCs derived from induced pluripotent stem cells (iPSCs), which offer the potential for more standardized cell preparations. MSCs have emerged as a particularly advantageous source of iPSCs due to their ease of isolation and cultivation, as well as their relatively straightforward reprogramming compared to terminally differentiated cells (Shao et al., 2013).Therefore, it is crucial to identify the commonalities and differences between various MSCs types in the treatment of bone defects.
Studies have shown that the different molecular antigens expressed on MSCs can be used to distinguish and identify their source and function (Table 1). The more commonly used BM-MSCs highly express numerous surface markers, such as CD10, CD13, CD29, CD44, CD49c, CD49f, CD59, CD73, CD81, CD90, CD105, CD106, CD143, CD146, CD147, CD151, CD166, CD271, CD276, low-affinity nerve growth factor receptor (LNGFR), podocalyxin-like protein 1 (PODXL), Stro-1, and bone morphogenetic protein receptor type 1A (BMPR-1A), with little or no expression of CD3, CD11b, CD14, CD19, CD34, CD36, CD45, CD79a, CD117, and CD133 (Jones et al., 2002; Shiota et al., 2007; Pachón-Peña et al., 2011; Amati et al., 2018). Nevertheless, significant differences in the percentage of surface expression were observed among MSCs from different sources. Compared with BM-MSCs, AD-MSCs showed surface positives for CD34 and CD36, while showing only weak positivity for CD49f, CD106 and PODXL (Pachón-Peña et al., 2011). More importantly, CD143 is specifically expressed on BM-MSCs and is restricted to only adult sources (Amati et al., 2018). Additionally, CD106 serves as a marker of placental chorionic plate-derived mesenchymal stem cells (CP-MSCs), which possess strong immune regulation functions (Fukiage et al., 2008; Yang et al., 2013). Moreover, CD271 has been identified as a crucial marker during the high-purity isolation of BM-MSC, as CD271high BM-MSCs contain colony-forming units-fibroblast (CFU-F) and other positive markers, including CD10, CD13, CD73, and CD105 (Bühring et al., 2007).
In addition to expressing specific markers, the cytokines secreted by different types of MSCs are also key in distinguishing MSC clusters. For instance, BM-MSCs, AD-MSCs, and UC-MSCs secrete varying levels of hepatocyte growth factor (HGF), transforming growth factor-β1 (TGF-β1), interleukin-6 (IL-6), interleukin-10 (IL-10), and prostaglandin E2 (PGE2) (Chen et al., 2015; Nakao et al., 2019). Granulocyte colony-stimulating factor (G-CSF) and granulocyte-macrophage colony-stimulating factor (GM-CSF) are predominantly produced by BM-MSCs, whereas vascular endothelial growth factor (VEGF) expression in BM-MSCs is relatively low (Ostanin et al., 2011; Dabrowski et al., 2017; Tyrina et al., 2023). A significantly higher 26S proteasome activity was detected in AD-MSCs than in BM-MSCs. Levels of intercellular cell adhesion molecule-1 (ICAM-1), integrin α5 and integrin α6 were significantly higher in AD-MSCs compared to BM-MSCs (Abu-El-Rub et al., 2024). Compared with BM-MSCs, AD-MSCs secrete higher levels of Th1/pro-inflammatory factors, such as IFN-γ, interleukin-2 (IL-2), interleukin-1β (IL-1β), and tumor necrosis factor-alpha (TNF-α). PMSCs produce higher levels of Th2/anti-inflammatory factors, such as interleukin-4 (IL-4), IL-6, and monocyte chemoattractant protein-1 (MCP-1), compared to BM-MSCs (Ostanin et al., 2011). These findings indicate that MSCs from different sources possess distinct immunomodulatory and adhesion profiles, which may significantly impact their roles in tissue repair and regeneration. Moreover, embryonic tissue-derived MSCs secrete low levels of matrix metalloproteinases (MMPs) (Bian et al., 2022) (Table 2). In pathological environments, cytokine secretion will change tremendously. For instance, the secretion of cytokines from BM-MSCs will increase after stimulation with high concentrations of lipopolysaccharide or endotoxin (Ostanin et al., 2011). Recent research has emphasized an even more nuanced aspect of MSC function: the release of extracellular vesicles (EVs). These nanoscale vesicles carry a cargo of bioactive molecules such as proteins, lipids, and RNAs that can influence recipient cells. EVs derived from BM-MSCs are enriched in anti-inflammatory proteins compared to EVs from other sources, which are associated with less organ damage (Blanco et al., 2023). Additionally, MSCs from different sources showed different differentiation capabilities. For example, muscle-derived MSCs regularly failed to form any histologically identifiable bone. In contrast, periosteum- and cord blood-derived MSCs can form bone, but they do not establish a hematopoietic microenvironment. BM-MSCs, however, are capable of forming bone and establishing the hematopoietic microenvironment (Sacchetti et al., 2016). Human BM-MSCs demonstrate the highest bone regenerative potential compared to those derived from adipose tissue, umbilical cord, mature chondrocytes, and skin fibroblasts. BMSCs were able to form cartilage discs in vitro and fully regenerate critical-size femoral defects mice. The binding sites of enhancers and promoter regions of ossification-related genes, which are commonly expressed transcription factors, are exclusively available in BM-MSCs. And the secretion of osteopontin initiates the healing of defects and the formation of bone hard calluses (Hochmann et al., 2023). These results suggest that MSCs with specific markers, distinct sources, and varying secretory factors can serve as multiple carriers for research and treatment, aiding scholars in constructing therapeutic complexes.
3 Origins and immunomodulatory role of MSCs in bone injury or healing
The healing process following bone injury involves a complex interaction between various cells and cytokines, with MSCs playing a crucial regulatory and reparative role (Figure 2). During the bone repair process, the recruitment of circulating stem/progenitor cells to the site of injury occurs as a normal biological response (Peihong et al., 2018). The ability of circulating or administered MSCs to migrate and integrate into the bone tissue environment is referred to as MSC homing (Ullah et al., 2019). The homing process is the initial step of bone formation, enabling MSCs to effectively migrate to the area of the bone defect, where they can repair and regenerate tissue as needed (Wang et al., 2013). This is an important factor in bone regeneration, as it indirectly reflects the ability of local bone to regenerate by recruiting progenitor cells (Liesveld et al., 2020; Guo et al., 2024). In the bone microenvironment, MSCs can originate from peripheral stromal vessel walls on the trabecular bone surface or in interfibrillar spaces (da Silva Meirelles et al., 2009). MSCs can differentiate into bone-related cells, such as osteoblasts and chondrocytes. Intravenous injection of CD271-selected MSCs within 24 h of a mouse femur fracture resulted in significant accumulation at the fracture sites for at least 7 days (Dreger et al., 2014). With technical advancements, research on MSCs homing has made substantial progress. Cytokines and chemokines, such as stromal cell-derived factor 1(SDF-1) and its receptor chemokine receptor type 4 (CXCR4) play important roles in maintaining mobilization, trafficking and homing of stem cells from bone marrow to the site of injury (Ye et al., 2020). After injury, such as fractures, the local hypoxic environment stimulates the secretion of these cytokines and chemokines, leading to the mobilization of MSCs. Lauer et al. reported that the application of SDF can promote MSCs homing to the fracture site, which could be conducive to fracture healing (Lauer et al., 2020). Furthermore, the upregulation of the chemokine C-X-C motif ligand 12 (CXCL12) at the bone injury site could promote the migration ability of MSCs expressing CXCR4 (Yellowley, 2013).The widely employed short-wave therapy in clinical practice enhances MSCs homing during fracture healing by upregulating the expression of cytokines such as SDF-1 and CXCR-4 in the callus (Ye et al., 2020). MSC homing marks the initiation of bone formation. Improving the migratory capacity of MSCs holds immense importance for the process of bone formation.
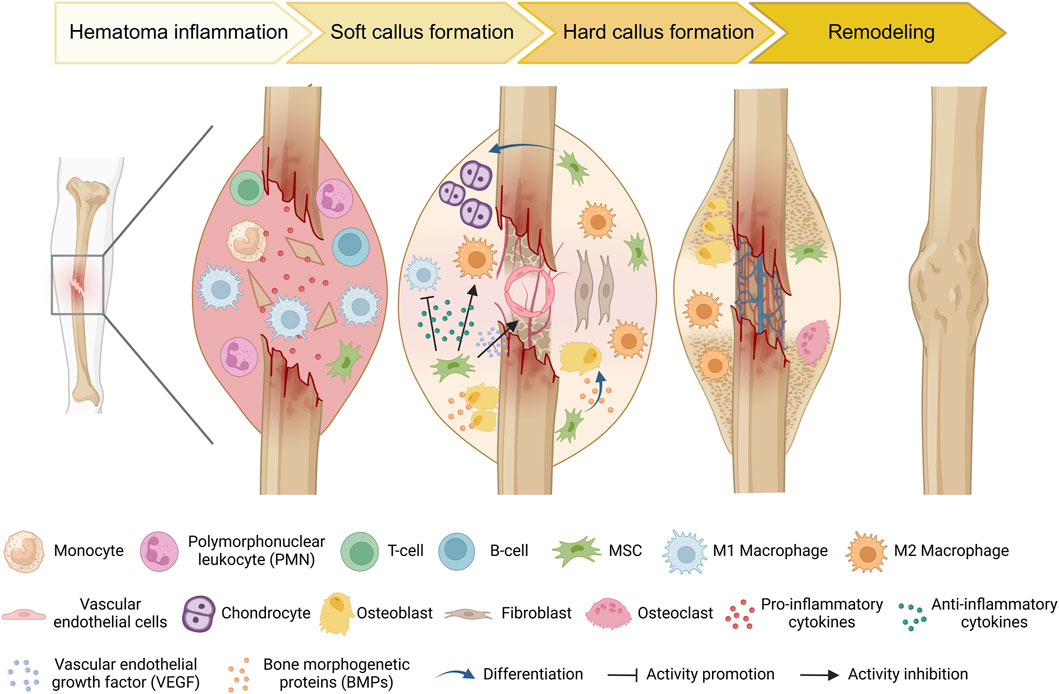
Figure 2. Schematic diagram of the typical natural bone healing process and cell dynamic transformation. During the inflammatory stage, injury triggers the recognition of damage-associated molecular patterns (DAMPs) by innate immune cells. These cells then secrete pro-inflammatory cytokines to recruit additional inflammatory cells and initiate an adaptive immune response. Concurrently, MSCs are recruited to the injury site. As necrotic debris is cleared and the immunomodulatory effects of MSCs, anti-inflammatory cells (e.g., M2 macrophages) increase, which promotes the differentiation of MSCs into osteoblasts and chondrocytes, thereby accelerating bone matrix mineralization and callus formation. As new bone tissue forms, the fracture site becomes more stable. Subsequently, osteoclasts begin the remodeling process by resorbing old and loose bone tissue, ultimately restoring bone shape and strength.
In the early stages of bone injury, cells in the bone microenvironment are damaged. Due to the release of injury-associated cytokines, neutrophils, monocytes, and lymphocytes successively infiltrate into the injury site (Chow et al., 2022). At this stage, homing MSCs mediates necrotic tissue clearance and bone regeneration through immune regulation. For example, during the early phases of bone infection, tissue-specific MSCs induce increased recruitment, activation, and sensitization of neutrophil granulocytes (Brandau et al., 2010). In the bone microenvironment, the level of type I interferon (IFN-I) will increase once MSCs are stimulated by injury cytokines. The IFN-I produced by MSCs can also enhance the effector function of NK cells in the early stage of bone repair (Petri et al., 2017). In addition, several studies have shown that as a type of multipotent stem cell, MSCs play an important role in the regulation of osteoimmunology. Bartholomew et al. was the first to discover the immunosuppressive ability of MSCs by demonstrating that MSCs inhibit lymphocyte proliferation in vitro and allogeneic skin transplantation rejection in vivo, thus confirming that MSCs have an immunoregulatory effect in bone (Bartholomew et al., 2002). In subsequent studies, it was found that MSCs could exercise immunomodulatory effects in both innate and adaptive ways (Glennie et al., 2005; Pan et al., 2024). Therefore, MSCs can serve as promising targets for early intervention therapies in tissue regeneration.
In the process of bone regeneration, the immunomodulatory properties of MSCs would be directly conducive to osteogenesis in inflammation conditions. MSCs regulate immune responses in the skeletal system via juxtacrine and paracrine signaling (Harrell et al., 2021). Preconditioning MSCs with pro-inflammatory cytokines alters their secretory profile and osteogenic potential. Ren and colleagues discovered that the immunosuppressive properties of MSCs are induced by IFN-γ, TNF-α, interleukin-1 alpha (IL-1α), and IL-1β (Ren et al., 2008). In vitro simulation of early pro-inflammatory conditions in fracture healing reveals that CD146-positive MSCs exhibit strong immunomodulatory and pro-angiogenic activities (Herrmann et al., 2019). Furthermore, the investigation reveals that the immunosuppressive function of MSCs is mainly mediated through the secretion of cytotoxic T lymphocyte antigen 4 (CTLA-4), especially under hypoxic conditions (Gaber et al., 2018). This immunomodulatory property can regulate local inflammation, creating a more favorable environment for bone healing and promoting the osteogenic differentiation of MSCs. Among the various elements influencing this process, the interaction between MSCs and macrophages is essential for maintaining bone homeostasis and facilitating regeneration (Hu et al., 2023). MSCs enhance healing by secreting a variety of cytokines and chemokines, such as VEGF-α, Insulin-like growth factor 1 (IGF-1), epidermal growth factor (EGF), keratinocyte growth factor, angiopoietin-1, SDF-1, macrophage inflammatory protein-1 (MIP-1), that promote the migration and proliferation of macrophages and endothelial cells (Chen et al., 2008). High IFN-γ and TNF-α levels could stimulate MSCs to secrete a large number of immunosuppressive cytokines, such as HGF, TGF-β, indoleamine 2,3-dioxygenase (IDO), PGE2, and nitrous oxide (NO) (Hackel et al., 2021). In addition, the specific C-C motif chemokine ligands 2 (CCL2) released from MSCs may facilitate their interaction with macrophages in the skeletal system (Shen et al., 2016; Toya et al., 2023). When MSCs are co-cultured with macrophages, the pro-inflammatory cytokines TNF-α, IL-1β, and IL-6 from the macrophages are suppressed while the anti-inflammatory cytokine IL-10 is produced (Hu et al., 2015; Chen et al., 2019). A study conducted by Heo et al. demonstrated that MSCs could induce macrophages to transit into the M2 phenotype through the action of exosomes in vitro (Heo et al., 2019). Mesenchymal stem cell-conditioned media (MSC-CM), particularly when stimulated with IL-4, exhibited anti-inflammatory effects by inhibiting the activation of lipopolysaccharide (LPS)-stimulated macrophages, downregulating inflammatory mediators, and suppressing key inflammatory pathways (Jin et al., 2022). Moreover, TNF-stimulated gene 6 protein (TSG-6), IL-6, and IL-10 from MSCs to macrophages exert similar effects as mentioned above (Lo Sicco et al., 2017; Arabpour et al., 2021). M2 macrophages could further enhance the proliferation of CD4+CD25+FOXP3+ T cells (Tregs), a subtype of immunosuppressive T cell (Tiemessen et al., 2007; Zhao W. et al., 2020). Correspondingly, the enhancement of M2 macrophages promotes the osteogenic differentiation of MSCs (Mahon et al., 2020). Additionally, the paracrine interactions between MSCs and macrophages are regulated by 1,25-dihydroxyvitamin D3 (Saldaña et al., 2017). Further experiments showed that macrophage mitochondrial transfer can promote the osteogenic differentiation of MSCs. Increased mitochondrial transfer of M1-like macrophages to MSCs triggers a reactive oxygen species (ROS) burst, which leads to metabolic remodeling (Cai et al., 2023; Qiu et al., 2024). In summary, the combined effects of MSCs on immune cells regulate the inflammatory response, particularly through the interplay and communication mechanisms between MSCs and macrophages, which contribute to the establishment of a more stable immune microenvironment, stimulate angiogenesis, and promote the proliferation and differentiation of osteoblasts.
Except for macrophages, MSCs can modulate local immune state indirectly through interactions with other immune cells. For instance, MSCs can induce the formation of Tregs via heme oxygenase-1 (HO-1) and IL-10. Scholars found that MSCs promote the proliferation of Th2 cells and Tregs by inhibiting Th1 cells, Th17 cells, and B cells (Schena et al., 2010; Fan et al., 2012; Luz-Crawford et al., 2013). Tregs are known to positively impact bone regeneration by stimulating the osteogenic differentiation of MSCs (Li et al., 2024). Moreover, the CD146high MSCs play an inhibitory role in host T cells, which is related to apoptosis of T cells (Bikorimana et al., 2022). In the regulation of dendritic cell (DC), both MSCs and their supernatants could decrease DC endocytosis by impairing IL-12 levels (Zhang et al., 2004). MSCs could elevate the population of CTLA-4+ DCs, which play a crucial role in inducing naïve T cells, mainly polarizing them into IL-10+IL-17+ T helper cells (Mázló et al., 2021). Exosomes derived from MSCs can also promote the maturation of DCs by reducing IL-6 levels while increasing IL-10 and TGF-β levels. This process enhances antigen presentation, initiates the immune response, and coordinates the repair process (Shahir et al., 2020).The MSCs could also increase the levels of CD24highCD38high B cells and IL-10-producing B cells while reducing the number of memory B-cells (Carreras-Planella et al., 2019) (Figure 3). Based on the findings of current studies, MSCs primarily exhibit anti-inflammatory properties and promote bone regeneration at defect sites by modulating their cytokines expression in the bone microenvironment. This suggests that MSCs can be multipotent seeds for bone tissue engineering. Given their numerous immunomodulatory effects, MSCs are crucial not only for the repair of bone defects but also for the management of various bone-related diseases, including osteoarthritis. MSCs can suppress the release of inflammatory mediators, attenuate the activation of macrophages, T and B cells, and facilitate cartilage regeneration and repair. These mechanisms collectively contribute to a reduction in inflammation and tissue damage, thereby enhancing joint function (Fahy et al., 2014; Fichadiya et al., 2016; Hu et al., 2018; Labinsky et al., 2020; Zhao X. et al., 2020; Zhu et al., 2020). MSCs play a crucial role in maintaining the homeostasis and equilibrium of the bone microenvironment (Lin et al., 2021; Shen and Shi, 2021). These findings open new ways for treatment options in bone regeneration processes. The potential for designing various types of MSC carriers to meet the requirements for bone defect healing within the skeletal system remains a significant area of exploration.
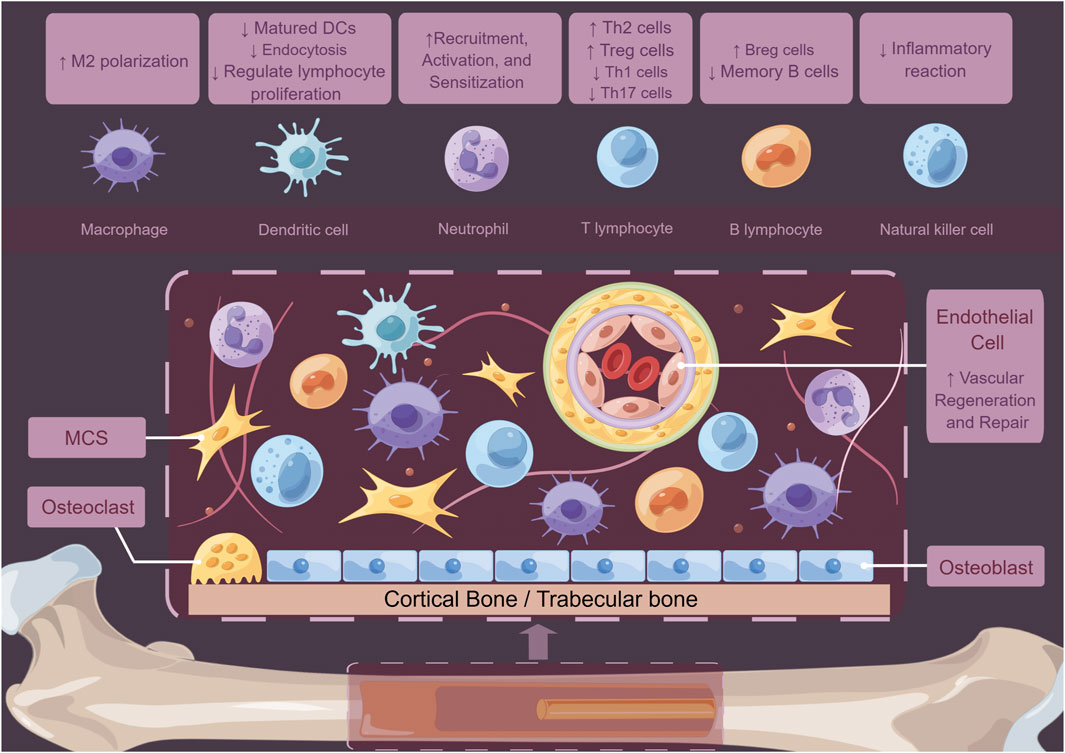
Figure 3. Schematic diagram of how MSCs regulate the bone marrow microenvironment. MSCs not only act on immune cells such as macrophages, DCs, neutrophils, T cells, B cells, and NK cells to play crucial immunomodulatory roles but also regulate tissue cell functions and mediate vascular repair and regeneration through autocrine and paracrine proteins.
4 MSCs in bone defect healing
Currently, MSCs have been used as therapeutic agents for a variety of disorders, particularly in bone regeneration, due to their capacity for self-renewal, migration, and immunomodulation (Song et al., 2020). Bone defects can generally be classified into two categories: traumatic and pathological. When bone defects occur, the release of inflammatory factors will attract MSCs to migrate into the injury sites. Subsequently, MSCs secrete cytokines locally to facilitate signaling communication essential for bone formation (Kang et al., 2022). Although significant advancements have been made in the treatment of bone defects within clinical orthopedics, large-scale bone defects--defined as those greater than 1–2 cm with a more than 50% loss in bone circumference--continue to pose a clinical challenge (Schemitsch, 2017). Thus, repairing large defects has been a high-interest topic in recent years. Notably, MSCs are regarded as ideal candidates for assisting in the repair of bone defects. Numerous studies have demonstrated that the combined application of MSCs with cell-secreted substances, such as exosomes and cytokines, along with genetic modification and preconditioning, could help to mitigate the limitations of these methods within the bone microenvironment (Huang et al., 2020; Pawitan et al., 2020; Nakao et al., 2021; Zou et al., 2023). The combination of bioactive materials and MSCs may further enhance osteogenic effect at bone defect sites (Niu et al., 2017; Xiongfa et al., 2020; Xie et al., 2022; Park et al., 2024). As of January 2024, over 70 clinical trials involving the use of MSCs in bone regeneration, bone tumors, osteogenic dysfunction and osteonecrosis have been carried out worldwide (https://trialsearch.who.int and http://www.clinicaltrials.gov, accessed by 30 January 2024) (Figure 4).
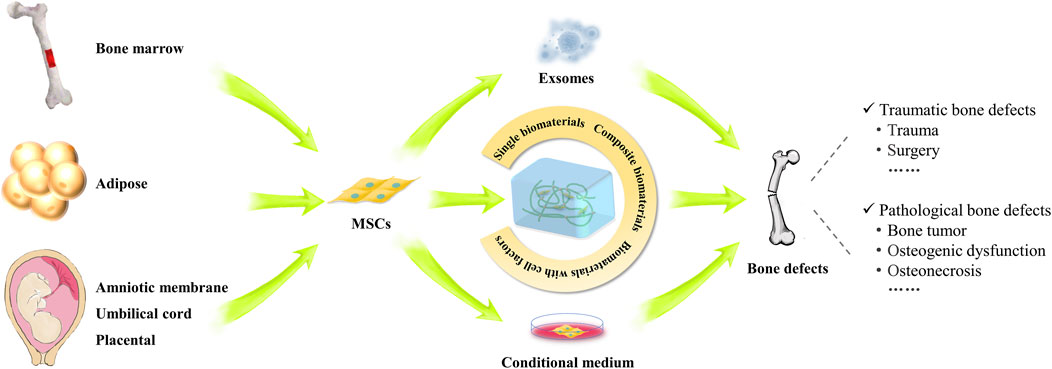
Figure 4. Study and applications of MSCs in the repair of various bone defects. The schematogram illustrates the use of MSCs from different sources in the treatment of bone defects. MSC implantation, conditioned medium, exosomes and MSC composite biomaterials can promote the repair of various types of bone defects.
4.1 MSCs in traumatic bone defects
Traumatic fractures and surgical osteotomies are common types of bone defects. In general, traumatic fracture repair can be divided into four phases: the initial inflammatory response, soft callus formation, hard callus formation, initial bony union and bone remodeling (Schindeler et al., 2008) (Figure 2). If patients present with a local or systemic infection, poor alignment, multiple combined injuries, or are in poor physical condition, delayed or nonunion of bone will occur (Hak et al., 2014; Tian et al., 2020). Thus far, there are many ways to efficiently resolve the above concerns. Various therapeutic strategies, including autologous bone grafting, allogeneic bone grafting, and the application of synthetic bioactive materials, have demonstrated substantial clinical potential for addressing bone defects. Despite these advancements, several limitations remain that impact their efficacy and applicability (Ferraz, 2023). In response to these challenges, the field of MSCs research has become an emerging trend. The focus of bone defect treatment has evolved from merely achieving bone continuity to employing bioengineering approaches aimed at optimizing the osteo-microenvironment.
MSCs can differentiate into osteoblasts to initiate local bone repair or secrete bioactive substances indirectly influence bone regeneration (Asgharzadeh et al., 2018). Huang et al. used femoral fracture models of mice to demonstrate that both systemic and local application of MSCs promoted fracture healing equally through direct differentiation into osteoblasts (Huang et al., 2015). Above all, scholars found that compared with MSCs, MSC-CM had higher concentrations of cytokines, which could accelerate bone healing in bone defect sites with cellular secretions (Osugi et al., 2012). The CM secreted by MSCs primarily consists of numerous active substances, including cytokines, immune factors, and other components (Al-Sharabi et al., 2023). MSC-CM enhances macrophage migration and induces the switching of macrophages from M1 to M2 types at an early stage of osteogenesis (Katagiri et al., 2022). Benavides-Castellanos et al. concluded that the application of MSC-CM in bone defects could have a beneficial effect on the repair and regeneration of bone tissue, which shows that paracrine effects play a crucial role in MSC treatment (Benavides-Castellanos et al., 2020). To further investigate the reason for this observation, scholars found that the exosomes in MSC-CM are critical effector substances in osteogenesis and angiogenesis (Hu et al., 2015; Furuta et al., 2016; Diomede et al., 2018). As the research progresses, exosomes derived from stem cells have been extensively studied for their role in promoting regeneration and reconstruction of various tissues as “cell-free” therapies. Li et al. (2022) used MSCs as mediators that were genetically engineered with the bone morphogenetic protein-2 (BMP2) gene to produce exosomes (MSC-BMP2-Exo), which have potential applications in the process of bone repair. The engineered MSCs function as cellular factories, and produced exosomes exhibit excellent biocompatibility and homing ability. Other than enhancing osteogenic differentiation, MSC-Exos increase the expression of VEGF and hypoxia inducible factor-1α (HIF-1α), which are crucial for promoting angiogenesis and accelerating fracture healing (Zhang et al., 2019). Liu et al. (2020) found that the hypoxic pretreatment of MSCs enhances the production of exosome miR-126 by activating HIF-1a, which mediates the SPRED1/Ras/Erk signaling pathway to promote fracture healing. In addition to directly regulating bone regeneration, MSCs can be systemically infused to reduce inflammation. Liu et al. found that inflammatory cytokines (IFN-γ and TNF-α) at implantation sites are downregulated by systemic infusion of MSCs, which upregulates Tregs (Liu et al., 2015). This approach can also enhance bone regeneration in MSC-seeded scaffolds. The results of the meta-analysis strengthen the evidence supporting the systemic application of MSCs in bone regeneration (Fu et al., 2021). However, detailed mechanisms should be explored in future studies. The diverse capabilities of MSCs, including direct osteogenic differentiation, paracrine effects through MSC-CM, and the use of engineered exosomes, underscore their significant potential for promoting bone regeneration and accelerating fracture healing. Their ability to enhance bone regeneration both directly and indirectly makes them ideal biotherapeutic agents.
With the development of osteogenesis-promoting biomaterials, the combination of biomaterials and MSCs has been precisely advanced to treat bone defects with suitable biocompatibility, physicochemical stability, and safety. In general, MSCs are seeded on scaffolds, which are then transplanted to bone defects for bone regeneration. Currently, delivering MSCs via biodegradable scaffolds is an important direction in the study of bone repair. Such bioactive scaffolds not only enhance the adhesion, growth, and survival of MSCs but also guide MSCs to differentiate into osteoconductive structures (Venkataiah et al., 2021). Marcacci and colleagues first reported the use of autologous MSCs expanded in vitro and inoculated onto a porous ceramic scaffold of hydroxyapatite (HA), a main inorganic component of bone (Marcacci et al., 2007). Wang et al. transplanted coral scaffolds with rabbit-derived AD-MSCs into bone defects in nude mice, and the rate of bone formation was significantly enhanced after 8 weeks (Wang et al., 2021). Moreover, bone defect healing can be improved by using nanomaterials to affect the polarization of macrophages and their interaction with MSCs. M1 macrophages initiate and maintain an inflammatory response in the early stages of bone healing to help remove damaged tissue; M2 macrophages promote the formation and remodeling of new bone in the middle and late stages of bone healing (Murayama et al., 2024). HA and poly (methyl methacrylate) (PMMA) particles could drive the polarization of macrophages towards an inflammatory phenotype, leading to an inflammatory cascade which culminates in periprosthetic osteolysis. Pre-treatment of macrophages with pharmacological inhibitors of these molecules in turn prevents macrophage polarization and dampens inflammatory cytokine production (Mahon et al., 2018). With the improvement of technology, according to current research reports, nano hydroxyapatite particles (BMnP) polarize human macrophages towards M2 phenotype, and further enhance MSC osteogenesis in an IL-10-dependent manner (Mahon et al., 2020). The GAD/Ag-pIO scaffold enhances osteogenic differentiation and fracture healing through immunomodulation and promotion of macrophage mitochondrial transfer (Qiu et al., 2024). Fibrin-MSC composites triggered rapid attraction of host cells into the hydrogel, forming a migration front dominated by M1 macrophages and endothelial progenitor cells, which stimulates early tissue maturation and primitive blood vessel formation, thereby promoting long bone healing in rats (Seebach et al., 2014). IL-4 overexpressing MSCs within macroporous gelatin-based microribbon (μRB) scaffold promoted the expression of M2 markers and increased the chemotaxis of macrophages into the scaffold, which contributes to MSC osteogenesis differentiation and helps bridge the long bone defect (Ueno et al., 2020).
To better improve the effectiveness of combination strategies, different kinds of active ingredients have been applied in bone defect treatment with a collaborative therapeutic effect. Xie et al. (2016) found that hydroxyapatite/collagen/chitosan (HAp/Col/CTS) could significantly promote the adhesion, proliferation, and osteogenesis of MSCs. When a 30% HAp/CTS scaffold combined with MSCs from second trimester human amniotic fluid was implanted into the bone defect in a rabbit tibia, the bone healing rate improved significantly (Mohammed et al., 2019). Kamali et al. prepared a gelatin/nanohydroxyapatite (G/nHAp) scaffold loaded with cannabidiol (CBD) and found that several MSCs migrated into the rat radial bone defect site (Kamali et al., 2019). Li et al. co-transplanted nano-HAp/polyurethane scaffolds with osteoblasts induced by rat-derived MSCs. The results showed that alkaline phosphatase (ALP), Col-I, osteocalcin (OCN), MSX2, and Runt-related transcription factor 2 (RUNX2) increased greatly (Li L. et al., 2019). Aslam et al. developed a tri-composite biomaterial with marine-derived chitosan, fucoidan, and HA, loaded with MSCs, showing prospects for fracture treatment, promoting cell proliferation and osteogenesis (Aslam et al., 2023). Zarei et al. reported the fabrication of poly (lactic acid)/Ti6Al4V@calcium phosphate core-shell nanocomposite scaffolds through fused deposition modeling (FDM). These scaffolds demonstrated enhanced mechanical properties and in vitro biocompatibility, supporting the attachment, differentiation, and proliferation of human AD-MSCs, making them a promising candidate for repairing critical-size bone defects (Zarei et al., 2023). Furthermore, combining bioactive materials and pro-osteogenic factors from MSCs also has a positive effect on bone regeneration. BMPs belong to the TGF-β family, which are particularly important in bone and cartilage formation, as they stimulate the proliferation and differentiation of osteoblasts (Mengrui et al., 2024). Sun et al. installed a recombinant adenovirus with BMP-2 into a hydrogel scaffold, and clear bone formation was observed after 6 weeks in a mouse model (Sun et al., 2020). The secretome of MSCs overexpressing BMP-9 enhanced the osteoblast differentiation and bone repair capabilities of MSC by upregulating RUNX2, ALP and osteopontin protein expression (Calixto et al., 2023). Schoonraad et al. used a matrix-metalloproteinase (MMP)-sensitive hydrogel nanocomposite, comprised of poly(ethylene glycol) crosslinked with MMP-sensitive peptides, tethered RGD, and entrapped HA nanoparticles, proving that rhBMP-2 and rhBMP-9 enhance the osteogenic potential of human MSC embedded in hydrogels (Schoonraad et al., 2023). He et al. developed an acoustically responsive biomimetic hydrogel scaffold complex that releases SDF-1/BMP-2 cytokines via pulsed ultrasound to promote the endogenous BMSCs recruiting and bone regeneration (He et al., 2023). These studies demonstrate that MSCs are currently a well-established option in the field of bone tissue engineering and regenerative medicine. However, their availability and capacity for self-renewal remain constrained in practice. Recent advances in somatic cell reprogramming offer a promising solution to these challenges (Takahashi and Yamanaka, 2006). The emergence of iPSCs has revolutionized the field of regenerative medicine, particularly in the realm of bone healing and regeneration. As researchers explore their capabilities, studies have confirmed that iPSC-derived MSCs demonstrate superior effectiveness in regenerating nonunion bone defects in murine models compared to traditional BM-MSCs (Sheyn et al., 2016). Transplantation of iPSC-seeded PLGA/aCaP scaffolds may improve bone regeneration in critical-size bone defects in mice (Kessler et al., 2024). Further research has demonstrated that iPSCs can generate high yields of osteogenic cell-matrix (OCM) in vitro, with osteogenic activity surpassing that of BMP-2. This development offers a biologically active and scalable biomaterial strategy without donor cells for enhancing bone regeneration in patients with delayed or failed bone healing. This donor-free cell products mitigates concerns related to donor variability and presents a higher safety profile compared to synthetic biomaterials (McNeill et al., 2020; Hanetseder et al., 2023). Therefore, the extending scope of bioactive material selection and the applications of various composite factors will lead to important developments and improvements in bone defect therapy soon.
Moreover, significant effectiveness of pure MSCs or the combination of MSCs and biological scaffolds has been shown in clinical trials. A systematic review and meta-analysis confirmed that MSCs therapy significantly augmented the progress of bone regeneration and significantly reduced the incidence of poor recovery (Yi et al., 2022). For example, scholars combined MSCs loaded on fibrin clots and implanted them into an upper bone nonunion. The remodeling rate of bone was significantly enhanced (Giannotti et al., 2013). Hernigou et al. implanted BM-MSCs from the posterior iliac ridge at the contact site between the acetabular graft and the host and observed a rapid increase in graft-host bone osseointegration (Hernigou et al., 2014). In tibial plateau collapse, the application of autologous MSCs/β-tricalcium phosphate (β-TCP) increased the bone regeneration ability and promoted patient rehabilitation (Gan et al., 2008; Chu et al., 2019). Additionally, MSC exosomes integrated into the β-TCP scaffold increased the osteogenic activity in bone defect sites (Zhang et al., 2016). The combination of amplified hBM-MSCs with biomaterials can effectively obtain bone consolidation (Gómez-Barrena et al., 2020). The combination treatment of WJ-MSCs and teriparatide has been shown to be feasible and tolerable in Phase I/IIa studies, and has a clinical benefit for fracture healing by promoting bone architecture (Shim et al., 2021). A case report has shown that the implantation of MSCs and secretome to treat osteoporotic compression fractures can improve the quality of bones and surrounding tissues (Rahyussalim et al., 2023). In short, patients with fractures benefit from MSCs administration. These findings demonstrate the clinical feasibility of combining MSCs with bioactive materials and are potentially useful drugs for bone regeneration. While these clinical applications have achieved satisfactory results, safety concerns and the proper screening needed have made determining how to accurately and suitably enrich cells in scaffolds and should be considered in bone regeneration.
4.2 MSCs in pathological bone defects
Pathological bone defects mainly refer to bone loss caused by congenital disease, deformity, bone tumors, osteonecrosis, osteogenic dysfunction, etc., These defects are typically characterized by a long cycle of slow repair and long-term nonhealing (Arrigoni et al., 2017). There remains an enormous need for effective strategies to repair pathological bone defects. Therefore, it is of great clinical value to study the application of MSCs in pathological defects.
4.2.1 Tumor defects
Bone tumors, especially those with malignant potential, frequently occur around the knee joint in the skeletal system. Osteosarcoma is one of the most prevalent malignant bone tumors, characterized by its rapid growth and high tendency for metastasis. (Shoaib et al., 2022). Surgery is a common treatment method; however, it often results in the retention of very large bone defect (Han et al., 2021; Machoň et al., 2022). Therefore, finding effective strategies to promote bone regeneration in bone tumors post operation remains a challenge for clinical experts. As pluripotent stem cells located in connective tissue, modified MSCs have the capability to not only promote osteogenesis and facilitate defect repair, but they also function as effective transport carriers for therapeutic agents. Additionally, modified MSCs exhibit antitumor properties by targeting and inhibiting tumor growth, offering a potential dual role in both regenerative medicine and cancer therapy (Qiao et al., 2015).
Osteolysis is a common pathological change of all osteosarcomas. It is a process of abnormal changes in physiological bone reconstruction and excessive bone resorption (deSantos and Edeiken, 1982; Julie et al., 2011). Nuclear factor κB receptor activator of nuclear factor ligand κB ligand (RANKL)/nuclear factor κB receptor activator of nuclear factor ligand κB (RANK)/osteoprotegerin (OPG) is a key pathway in the regulation of bone injury and osteolysis (Zhang et al., 2022). This pathway is important not only for bone homeostasis maintenance but also for pathological regulation. Previous studies found that increasing OPG expression could reduce tumor-induced osteolysis by inhibiting osteoclasts and weakening osteosarcoma (Lamoureux et al., 2007). Since transgenic MSCs can introduce antitumor factors into tumor sites, Qiao et al. overexpressed the OPG gene in MSCs and then applied them due to the aggregation of fluorescently labeled MSCs-OPG to osteosarcoma nude mice. The results showed that MSCs-OPG play an inhibitory role in osteosarcoma proliferation and osteolysis (Qiao et al., 2015).
MSCs could also serve as a promising platform for the targeted delivery of therapeutic nanomedicines. These medicines have the potential to regulate local immunity, enhance the osteogenic differentiation of MSCs and increase their immunomodulatory effects (Chang et al., 2021). This combination may yield a multifaceted approach to combating osteosarcoma. For example, aclitaxel on MSCs was accurately transported to the tumor site by MSC homing, showing a dose-dependent antitumor effect in vitro (Hu et al., 2022). Kalia et al. observed that when a HAp-coated circle was transplanted with autologous MSCs into a sheep bone tumor model, bone growth increased significantly, especially in the area near the coating circle, compared with the control group (Kalia et al., 2006). Lenna and workers performed photodynamic therapy (PDT) with MSCs loaded with AlPcS4@FNPs in vitro and in vivo, where AlPcS4 is a photosensitizer that can be activated with an LED source, and showed that MSCs can act as carriers to inhibit osteosarcoma growth (Lenna et al., 2020). In similar studies, it was found that MSCs loaded with a photosensitizer (TPPS@FNPs) could promote the release of ROS from osteosarcoma cells (Duchi et al., 2013). Suitable ROS stimulus can further induce macrophages polarization from M1 to M2 phenotype, which contributes to bone formation around implants (Tsai et al., 2021). Additionally, exosomes derived from MSCs also have therapeutic value (Nakao et al., 2021). Abello and colleagues found that labeled exosomes from human UC-MSCs could accumulate in the tumor site for more than 24–48 h after injection, suggesting that exosomes may be a prospective substance to treat bone tumors due to their tumor-targeting features (Abello et al., 2019).
However, it is unfortunate that some studies have also found that MSCs may promote osteosarcoma. For instance, a study by Avnet et al., MSCs were co-injected with osteosarcoma cells into subcutaneous and orthotopic models demonstrated that lung metastasis significantly increased because of the acidosis induced by MSCs (Avnet et al., 2021). Compared with osteoclast precursors, MSCs accelerated the local proliferation of osteosarcoma cells, but did not intensify the process of osteolysis and metastasis (Pierre et al., 2015). Moreover, exosomes derived from MSCs with overexpressed miR-21-5p can enhance the proliferation and invasion of osteosarcoma cells by activating the PI3K/Akt/mTOR signaling pathway, both in vitro and in vivo (Qi et al., 2021). It is astonished that MSCs could directly transform into Ewing sarcoma cells by engineering the t(11; 22) (q24; q12) translocation together with CDKN2A gene mutations (Sole et al., 2021). Thus, contradictory results have been reported, possibly due to nonstandard protocols, variations in tumor types and stages, the absence of specific cell markers for accurate identification, and the susceptibility of MSCs to their surrounding microenvironment. Consequently, a comprehensive analysis of the effects and underlying mechanisms of MSCs in bone tumors is warranted for future research.
4.2.2 Osteogenic dysfunction
Primary osteogenic dysfunction is often caused by inherited metabolic diseases. Osteogenesis imperfecta (OI), known as both brittle bone disease and china doll, is an autosomal inherited disorder that mainly manifests as generalized osteoporosis due to defects in osteogenesis and fibroblast function (Marom et al., 2016). OI-derived iPSCs exhibited decreased cell growth and impaired osteogenic differentiation and collagen expression (Duangchan et al., 2021). Otsuru et al. first reported that the use of allogeneic MSC transplantation in children with OI resulted in significant osteogenesis in osteoporosis locations (Otsuru et al., 2012). Furthermore, MSCs was injected into fetuses with OI during pregnancy and found significant improvements in bone line growth, reduced fracture incidence, and improved growth trajectory postpartum (Götherström et al., 2014). In the TERCELOI clinical trial, reiterative infusions of histocompatible MSCs in pediatric OI patients were safe, improved bone parameters, and elicited a pro-osteogenic paracrine response (Infante et al., 2021). It was observed that the expression of the TGF-β pathway was enhanced in the serum of the most severe pediatric patients with OI, which were modulated after MSCs therapy (Infante et al., 2022). Hypophosphatasia (HPP) is also a hereditary disease characterized by incomplete mineralization of bones and teeth (Khan et al., 2024). Clinical studies have reported that allogeneic MSCs injected into HPP patients significantly strengthened bone density (Taketani et al., 2015). Allogeneic MSC transplantation, along with the local implantation of osteogenic constructs incorporating MSCs and porous hydroxyapatite ceramics, demonstrated consistent improvement in respiratory and skeletal abnormalities in a patient with perinatal HPP (Tadokoro et al., 2009). Congenital pseudarthrosis of the tibia (CPT) is a rare orthopedic disorder characterized by a spontaneous nonhealing fracture, often associated with neurofibromatosis type I (NF1) (Van Royen et al., 2016, p. 1). Granchi et al. reported an effective achievement in bone tissue regeneration by using a regenerative strategy involving MSCs combined with platelet-rich fibrin (PRF) as a source of growth factors (Granchi et al., 2012). Moreover, the effectiveness of in situ injection of bone marrow aspirate concentrate (BMAC), as an adjunctive treatment for CPT, and autologous MSC injection combined with external fixation at the pseudarthrosis site resulted in faster bone healing in comparison to external fixation (Memeo et al., 2020). Kurniawan et al. explored the efficacy of combining UC-MSCs with secretome in treating CPT, revealing favorable outcomes such as high primary union rates and significant functional improvement in their case series (Kurniawan et al., 2023). Therefore, using MSC-based therapies might be a dependable way to treat both acquired and inherited bone defects. Advances in this field could lead to more effective treatments, potentially transforming the management of complex bone disorders and improving patient outcomes significantly.
4.2.3 Osteonecrosis
Osteonecrosis is typically caused by the lack of blood supply to bone tissue, leading to a pathological state characterized by cell death. This condition is often secondary to the effects of medications, radiation, or other chronic diseases (Shah et al., 2015). Jiang et al. investigated the efficacy of MSCs in a mouse model of glucocorticoid-induced osteonecrosis. They combined MSCs with Rab001, along with an MSC bone-targeted agent, and showed that this combination effectively directs MSCs to trabecular and endocortical bone surfaces and significantly inhibits the progression of osteonecrosis (Jiang et al., 2021). Additionally, the intravenous injection of MSCs into rabbits with hormonal femoral head necrosis resulted in an increased concentration of MSCs around the femoral blood vessels after 3 days and effectively alleviated the severity of osteonecrosis (Ueda et al., 2017). In several clinical trials with long-term follow-ups, the injection of autologous BM-MSCs into patients with femoral head necrosis significantly reduced the rate of artificial hip replacement and improved disease outcome compared to simple core decompression without cellular intervention (Daltro et al., 2015; Hernigou et al., 2018; Hernigou et al., 2021; Kang et al., 2018; Gómez-Barrena et al., 2021; Diri et al., 2023; Ulusoy et al., 2023). Recent studies emphasize the promising application of iPSCs in treating osteonecrosis of the femoral head through various mechanisms. iPSC-derived MSCs exhibit robust regenerative potential, promoting bone repair and angiogenesis in necrotic areas (Zhou et al., 2021). Additionally, exosomes secreted by human-iPSC-MSCs (hiPS-MSC-Exos) have demonstrated their potential as a therapeutic strategy for osteonecrosis of the femoral head by enhancing local angiogenesis and preventing bone loss through the activation of the PI3K/Akt signaling pathway in endothelial cells (Liu et al., 2017). Combining hiPS-MSC-Exos with miR-135b has been shown to further alleviate bone loss and promote osteoblast proliferation while inhibiting apoptosis (Xiang et al., 2020). These advancements underscore the transformative potential of MSCs therapies, particularly iPSCs, in not only halting the progression of osteonecrosis but also in fostering significant improvements in bone regeneration and overall patient outcomes.
In studies examining medication-related osteonecrosis of the jaw (MRONJ), the effects observed following treatment with MSCs were comparable to those found in therapies for hormone-related femoral head necrosis. For instance, Kaibuchi et al. observed that MSCs aggregated around new blood vessels after injecting MSCs labeled with fluorescent dyes into a rats model of MRONJ (Kaibuchi et al., 2016). While diseased MSCs from mice with MRONJ displayed inferior characteristics compared to MSCs from untreated mice, the exchange of cellular contents between control MSCs and diseased MSCs significantly contributed to the treatment potential of diseased MSCs (Matsuura et al., 2016). Moreover, the addition of CM from cultured MSCs in vitro to the model rats above promoted the migration, proliferation, and osteogenic differentiation of MSCs at osteonecrosis sites (Ogata et al., 2017; Ogata et al., 2018). Allogeneic BM-MSCs in extractions sites ameliorates MRONJ incidence in zoledronic acid-treated rats compared to non-MSC treatments (Rodríguez-Lozano et al., 2020). These patients with MRONJ exhibited varying degrees of pathological improvement. In addition to etiology-specific treatments, the use of MSCs has shown significant clinical outcomes for patients with bone necrosis. However, the detailed immune regulatory mechanisms of MSCs in treating osteonecrosis, as well as the interactions between allogeneic MSCs and various cell populations in the pathological microenvironment, need to be further elucidated.
5 Conclusion
Repairing bone defects caused by trauma, surgery, tumors, hypoplasia, and other factors has long posed a significant challenge in the field of bone tissue engineering. MSCs, a major type of adult stem cells, can be acquired from various tissues and exhibit low immunogenicity, multidirectional differentiation potential, and excellent compatibility and stability, making them ideal candidates for tissue repair and immune regulation. Currently, MSCs are drawing increasing attention for the treatment of bone defects. The regulated mechanisms underlying their bioactive behavior have been elucidated, revealing that these mechanisms involve differentiation, immune regulation, and paracrine effects. In this review, we primarily discussed the present application status of MSCs, emphasizing their regulatory effect in bone defects repair. MSCs affect osteogenesis through three-dimensional interactions with other cells, particularly macrophages. In summary, the applications of MSCs can be categorized into three aspects. First, there is the direct application of MSCs in bone healing, which remains the most common clinical treatment method. It also includes the use of MSCs as effective cells combined with bioactive materials, which can be applied via local or intravenous injection. Such approaches not only improve the biocompatibility of biomaterials but also improve the loading capacity and targeting of MSCs. Second, MSC-CM plays a crucial role by containing cytokines that activate specific signaling pathways to maintain balance in the bone microenvironment. Additionally, exosomes derived from MSCs also represent an important therapeutic component for bone defects because they carry multiple active factors, including DNA, RNA, and proteins. Third, MSCs can serve as vehicles or be modified into a specific complex to deliver one or more agents to the bone defect site through MSC homing. While MSCs have broad prospects in clinical bone defect therapy, further studies are necessary to investigate the underlying molecular mechanisms, their secretory factors and their influence on bone homeostasis and regeneration. It is imperative to expedite the development of combination therapies involving MSCs and biomaterials to accelerate their clinical translation. However, in extracting the clinical value of MSCs, it is equally necessary to recognize the importance of addressing regulatory considerations and potential obstacles, such as long-term safety and effectiveness.
Author contributions
YuZ: Writing–original draft, Writing–review and editing. MF: Writing–original draft, Writing–review and editing. YiZ: Formal Analysis, Funding acquisition, Project administration, Supervision, Writing–review and editing.
Funding
The author(s) declare that financial support was received for the research, authorship, and/or publication of this article. This research was funded by the Natural Science Foundation of Hebei Province “Precision Medicine” Joint Fund Key Project (No. H2020206456).
Conflict of interest
The authors declare that the research was conducted in the absence of any commercial or financial relationships that could be construed as a potential conflict of interest.
Publisher’s note
All claims expressed in this article are solely those of the authors and do not necessarily represent those of their affiliated organizations, or those of the publisher, the editors and the reviewers. Any product that may be evaluated in this article, or claim that may be made by its manufacturer, is not guaranteed or endorsed by the publisher.
References
Abello, J., Nguyen, T. D. T., Marasini, R., Aryal, S., and Weiss, M. L. (2019). Biodistribution of gadolinium- and near infrared-labeled human umbilical cord mesenchymal stromal cell-derived exosomes in tumor bearing mice. Theranostics 9, 2325–2345. doi:10.7150/thno.30030
Abu-El-Rub, E., Khaswaneh, R. R., Almahasneh, F. A., Almazari, R., and Alzu’bi, A. (2024). Adipose tissue and bone marrow-derived mesenchymal stem cells are not really the same: investigating the differences in their immunomodulatory, migratory, and adhesive profile. Biochem. Genet. doi:10.1007/s10528-024-10724-6
Aggarwal, S., and Pittenger, M. F. (2005). Human mesenchymal stem cells modulate allogeneic immune cell responses. Blood 105, 1815–1822. doi:10.1182/blood-2004-04-1559
Alford, A. I., Nicolaou, D., Hake, M., and McBride-Gagyi, S. (2021). Masquelet’s induced membrane technique: review of current concepts and future directions. J. Orthop. Res. 39, 707–718. doi:10.1002/jor.24978
Alhadlaq, A., and Mao, J. J. (2004). Mesenchymal stem cells: isolation and therapeutics. Stem Cells Dev. 13, 436–448. doi:10.1089/scd.2004.13.436
Al-Sharabi, N., Gruber, R., Sanz, M., Mohamed-Ahmed, S., Kristoffersen, E. K., Mustafa, K., et al. (2023). Proteomic analysis of mesenchymal stromal cells secretome in comparison to leukocyte- and platelet-rich fibrin. Int. J. Mol. Sci. 24, 13057. doi:10.3390/ijms241713057
Amati, E., Perbellini, O., Rotta, G., Bernardi, M., Chieregato, K., Sella, S., et al. (2018). High-throughput immunophenotypic characterization of bone marrow- and cord blood-derived mesenchymal stromal cells reveals common and differentially expressed markers: identification of angiotensin-converting enzyme (CD143) as a marker differentially expressed between adult and perinatal tissue sources. Stem Cell Res. Ther. 9, 10. doi:10.1186/s13287-017-0755-3
Arabpour, M., Saghazadeh, A., and Rezaei, N. (2021). Anti-inflammatory and M2 macrophage polarization-promoting effect of mesenchymal stem cell-derived exosomes. Int. Immunopharmacol. 97, 107823. doi:10.1016/j.intimp.2021.107823
Arrigoni, C., Gilardi, M., Bersini, S., Candrian, C., and Moretti, M. (2017). Bioprinting and organ-on-chip applications towards personalized medicine for bone diseases. Stem Cell Rev. Rep. 13, 407–417. doi:10.1007/s12015-017-9741-5
Asgari, H. R., Akbari, M., Yazdekhasti, H., Rajabi, Z., Navid, S., Aliakbari, F., et al. (2017). Comparison of human amniotic, chorionic, and umbilical cord multipotent mesenchymal stem cells regarding their capacity for differentiation toward female germ cells. Cell Reprogr. 19, 44–53. doi:10.1089/cell.2016.0035
Asgharzadeh, A., Alizadeh, S., Keramati, M. R., Soleimani, M., Atashi, A., Edalati, M., et al. (2018). Upregulation of miR-210 promotes differentiation of mesenchymal stem cells (MSCs) into osteoblasts. Bosn. J. Basic Med. Sci. 18, 328–335. doi:10.17305/bjbms.2018.2633
Aslam, B., Augustyniak, A., Clarke, S. A., and McMahon, H. (2023). Development of a novel marine-derived tricomposite biomaterial for bone regeneration. Mar. Drugs 21, 473. doi:10.3390/md21090473
Avila-Portillo, L. M., Aristizabal, F., Riveros, A., Abba, M. C., and Correa, D. (2020). Modulation of adipose-derived mesenchymal stem/stromal cell transcriptome by G-CSF stimulation. Stem Cells Int. 2020, 1–9. doi:10.1155/2020/5045124
Avnet, S., Lemma, S., Cortini, M., Di Pompo, G., Perut, F., Lipreri, M. V., et al. (2021). The release of inflammatory mediators from acid-stimulated mesenchymal stromal cells favours tumour invasiveness and metastasis in osteosarcoma. Cancers (Basel) 13, 5855. doi:10.3390/cancers13225855
Bai, L., Lennon, D. P., Caplan, A. I., DeChant, A., Hecker, J., Kranso, J., et al. (2012). Hepatocyte growth factor mediates mesenchymal stem cell–induced recovery in multiple sclerosis models. Nat. Neurosci. 15, 862–870. doi:10.1038/nn.3109
Bartholomew, A., Sturgeon, C., Siatskas, M., Ferrer, K., McIntosh, K., Patil, S., et al. (2002). Mesenchymal stem cells suppress lymphocyte proliferation in vitro and prolong skin graft survival in vivo. Exp. Hematol. 30, 42–48. doi:10.1016/s0301-472x(01)00769-x
Benavides-Castellanos, M. P., Garzón-Orjuela, N., and Linero, I. (2020). Effectiveness of mesenchymal stem cell-conditioned medium in bone regeneration in animal and human models: a systematic review and meta-analysis. Cell Regen. 9, 5. doi:10.1186/s13619-020-00047-3
Benkhoucha, M., Santiago-Raber, M.-L., Schneiter, G., Chofflon, M., Funakoshi, H., Nakamura, T., et al. (2010). Hepatocyte growth factor inhibits CNS autoimmunity by inducing tolerogenic dendritic cells and CD25+Foxp3+ regulatory T cells. Proc. Natl. Acad. Sci. U. S. A. 107, 6424–6429. doi:10.1073/pnas.0912437107
Bernardo, M. E., and Fibbe, W. E. (2013). Mesenchymal stromal cells: sensors and switchers of inflammation. Cell Stem Cell 13, 392–402. doi:10.1016/j.stem.2013.09.006
Bian, D., Wu, Y., Song, G., Azizi, R., and Zamani, A. (2022). The application of mesenchymal stromal cells (MSCs) and their derivative exosome in skin wound healing: a comprehensive review. Stem Cell Res. Ther. 13, 24. doi:10.1186/s13287-021-02697-9
Bianco, P. (2014). “Mesenchymal” stem cells. Annu. Rev. Cell Dev. Biol. 30, 677–704. doi:10.1146/annurev-cellbio-100913-013132
Bikorimana, J.-P., Saad, W., Abusarah, J., Lahrichi, M., Talbot, S., Shammaa, R., et al. (2022). CD146 defines a mesenchymal stromal cell subpopulation with enhanced suppressive properties. Cells 11, 2263. doi:10.3390/cells11152263
Blanco, N. G., Machado, N. M., Castro, L. L., Antunes, M. A., Takiya, C. M., Trugilho, M. R. O., et al. (2023). Extracellular vesicles from different sources of mesenchymal stromal cells have distinct effects on lung and distal organs in experimental sepsis. Int. J. Mol. Sci. 24, 8234. doi:10.3390/ijms24098234
Brandau, S., Jakob, M., Hemeda, H., Bruderek, K., Janeschik, S., Bootz, F., et al. (2010). Tissue-resident mesenchymal stem cells attract peripheral blood neutrophils and enhance their inflammatory activity in response to microbial challenge. J. Leukoc. Biol. 88, 1005–1015. doi:10.1189/jlb.0410207
Bruder, S. P., Fink, D. J., and Caplan, A. I. (1994). Mesenchymal stem cells in bone development, bone repair, and skeletal regenaration therapy. J. Cell Biochem. 56, 283–294. doi:10.1002/jcb.240560303
Bühring, H.-J., Battula, V. L., Treml, S., Schewe, B., Kanz, L., and Vogel, W. (2007). Novel markers for the prospective isolation of human MSC. Ann. N. Y. Acad. Sci. 1106, 262–271. doi:10.1196/annals.1392.000
Cai, W., Zhang, J., Yu, Y., Ni, Y., Wei, Y., Cheng, Y., et al. (2023). Mitochondrial transfer regulates cell fate through metabolic remodeling in osteoporosis. Adv. Sci. (Weinh) 10, e2204871. doi:10.1002/advs.202204871
Calixto, R. D., Freitas, G. P., Souza, P. G., Ramos, J. I. R., Santos, I. C., de Oliveira, F. S., et al. (2023). Effect of the secretome of mesenchymal stem cells overexpressing BMP-9 on osteoblast differentiation and bone repair. J. Cell Physiol. 238, 2625–2637. doi:10.1002/jcp.31115
Carreras-Planella, L., Monguió-Tortajada, M., Borràs, F. E., and Franquesa, M. (2019). Immunomodulatory effect of MSC on B cells is independent of secreted extracellular vesicles. Front. Immunol. 10, 1288. doi:10.3389/fimmu.2019.01288
Cassano, J. M., Schnabel, L. V., Goodale, M. B., and Fortier, L. A. (2018). Inflammatory licensed equine MSCs are chondroprotective and exhibit enhanced immunomodulation in an inflammatory environment. Stem Cell Res. Ther. 9, 82. doi:10.1186/s13287-018-0840-2
Chai, S., Huang, J., Mahmut, A., Wang, B., Yao, Y., Zhang, X., et al. (2022). Injectable photo-crosslinked bioactive BMSCs-BMP2-GelMA scaffolds for bone defect repair. Front. Bioeng. Biotechnol. 10, 875363. doi:10.3389/fbioe.2022.875363
Chamberlain, G., Fox, J., Ashton, B., and Middleton, J. (2007). Concise review: mesenchymal stem cells: their phenotype, differentiation capacity, immunological features, and potential for homing. Stem Cells 25, 2739–2749. doi:10.1634/stemcells.2007-0197
Chang, X., Ma, Z., Zhu, G., Lu, Y., and Yang, J. (2021). New perspective into mesenchymal stem cells: molecular mechanisms regulating osteosarcoma. J. Bone Oncol. 29, 100372. doi:10.1016/j.jbo.2021.100372
Chen, J.-Y., Mou, X.-Z., Du, X.-C., and Xiang, C. (2015). Comparative analysis of biological characteristics of adult mesenchymal stem cells with different tissue origins. Asian Pac J. Trop. Med. 8, 739–746. doi:10.1016/j.apjtm.2015.07.022
Chen, L., Tredget, E. E., Wu, P. Y. G., and Wu, Y. (2008). Paracrine factors of mesenchymal stem cells recruit macrophages and endothelial lineage cells and enhance wound healing. PLoS One 3, e1886. doi:10.1371/journal.pone.0001886
Chen, L., Zhang, Q., Chen, Q.-H., Ran, F.-Y., Yu, L.-M., Liu, X., et al. (2019). Combination of G-CSF and AMD3100 improves the anti-inflammatory effect of mesenchymal stem cells on inducing M2 polarization of macrophages through NF-κB-IL1RA signaling pathway. Front. Pharmacol. 10, 579. doi:10.3389/fphar.2019.00579
Chow, S. K.-H., Wong, C. H.-W., Cui, C., Li, M. M.-C., Wong, R. M. Y., and Cheung, W.-H. (2022). Modulating macrophage polarization for the enhancement of fracture healing, a systematic review. J. Orthop. Transl. 36, 83–90. doi:10.1016/j.jot.2022.05.004
Chu, W., Wang, X., Gan, Y., Zhuang, Y., Shi, D., Liu, F., et al. (2019). Screen-enrich-combine circulating system to prepare MSC/β-TCP for bone repair in fractures with depressed tibial plateau. Regen. Med. 14, 555–569. doi:10.2217/rme-2018-0047
Cieza, A., Causey, K., Kamenov, K., Hanson, S. W., Chatterji, S., and Vos, T. (2021). Global estimates of the need for rehabilitation based on the global burden of disease study 2019: a systematic analysis for the global burden of disease study 2019. Lancet 396, 2006–2017. doi:10.1016/S0140-6736(20)32340-0
Clarkin, C. E., King, A. J., Dhadda, P., Chagastelles, P., Nardi, N., Wheeler-Jones, C. P., et al. (2013). Activin receptor-like kinase 5 inhibition reverses impairment of endothelial cell viability by endogenous islet mesenchymal stromal cells. Stem Cells 31, 547–559. doi:10.1002/stem.1305
Dabrowski, F. A., Burdzinska, A., Kulesza, A., Sladowska, A., Zolocinska, A., Gala, K., et al. (2017). Comparison of the paracrine activity of mesenchymal stem cells derived from human umbilical cord, amniotic membrane and adipose tissue. J. Obstet. Gynaecol. Res. 43, 1758–1768. doi:10.1111/jog.13432
Daltro, G. C., Fortuna, V., de Souza, E. S., Salles, M. M., Carreira, A. C., Meyer, R., et al. (2015). Efficacy of autologous stem cell-based therapy for osteonecrosis of the femoral head in sickle cell disease: a five-year follow-up study. Stem Cell Res. Ther. 6, 110. doi:10.1186/s13287-015-0105-2
da Silva Meirelles, L., Sand, T. T., Harman, R. J., Lennon, D. P., and Caplan, A. I. (2009). MSC frequency correlates with blood vessel density in equine adipose tissue. Tissue Eng. Part A 15, 221–229. doi:10.1089/ten.tea.2008.0103
Deng, B., Wehling-Henricks, M., Villalta, S. A., Wang, Y., and Tidball, J. G. (2012). IL-10 triggers changes in macrophage phenotype that promote muscle growth and regeneration. J. Immunol. 189, 3669–3680. doi:10.4049/jimmunol.1103180
deSantos, L. A., and Edeiken, B. (1982). Purely lytic osteoasarcoma. Skelet. Radiol. 9, 1–7. doi:10.1007/BF00367373
Diomede, F., D’Aurora, M., Gugliandolo, A., Merciaro, I., Orsini, T., Gatta, V., et al. (2018). Biofunctionalized scaffold in bone tissue repair. Int. J. Mol. Sci. 19, 1022. doi:10.3390/ijms19041022
Diri, D., Alasaad, H., Muhammed, H., and Ibrahim, J. (2023). Case report: adipose-derived mesenchymal stem cells combined with core decompression in the treatment of early-stage avascular necrosis of the femoral head. Int. J. Surg. Case Rep. 102, 107861. doi:10.1016/j.ijscr.2022.107861
Dn, S., Bsf, S., Cm, A., Jf, V., Pg, de J., Ms, F., et al. (2018). IGF-1-Overexpressing mesenchymal stem/stromal cells promote immunomodulatory and proregenerative effects in chronic experimental chagas disease. Stem cells Int. 2018, 1–11. doi:10.1155/2018/9108681
Dominici, M., Le Blanc, K., Mueller, I., Slaper-Cortenbach, I., Marini, F., Krause, D., et al. (2006). Minimal criteria for defining multipotent mesenchymal stromal cells. The International Society for Cellular Therapy position statement. Cytotherapy 8, 315–317. doi:10.1080/14653240600855905
Dreger, T., Watson, J. T., Akers, W., Molligan, J., Achilefu, S., Schon, L. C., et al. (2014). Intravenous application of CD271-selected mesenchymal stem cells during fracture healing. J. Orthop. Trauma 28 (Suppl. 1), S15–S19. doi:10.1097/BOT.0000000000000063
Du, W. J., Chi, Y., Yang, Z. X., Li, Z. J., Cui, J. J., Song, B. Q., et al. (2016). Heterogeneity of proangiogenic features in mesenchymal stem cells derived from bone marrow, adipose tissue, umbilical cord, and placenta. Stem Cell Res. Ther. 7, 163. doi:10.1186/s13287-016-0418-9
Duangchan, T., Tawonsawatruk, T., Angsanuntsukh, C., Trachoo, O., Hongeng, S., Kitiyanant, N., et al. (2021). Amelioration of osteogenesis in iPSC-derived mesenchymal stem cells from osteogenesis imperfecta patients by endoplasmic reticulum stress inhibitor. Life Sci. 278, 119628. doi:10.1016/j.lfs.2021.119628
Duchi, S., Sotgiu, G., Lucarelli, E., Ballestri, M., Dozza, B., Santi, S., et al. (2013). Mesenchymal stem cells as delivery vehicle of porphyrin loaded nanoparticles: effective photoinduced in vitro killing of osteosarcoma. J. Control Release 168, 225–237. doi:10.1016/j.jconrel.2013.03.012
Eliopoulos, N., Stagg, J., Lejeune, L., Pommey, S., and Galipeau, J. (2005). Allogeneic marrow stromal cells are immune rejected by MHC class I- and class II-mismatched recipient mice. Blood 106, 4057–4065. doi:10.1182/blood-2005-03-1004
Fahy, N., de Vries-van Melle, M. L., Lehmann, J., Wei, W., Grotenhuis, N., Farrell, E., et al. (2014). Human osteoarthritic synovium impacts chondrogenic differentiation of mesenchymal stem cells via macrophage polarisation state. Osteoarthr. Cartil. 22, 1167–1175. doi:10.1016/j.joca.2014.05.021
Fan, H., Zhao, G., Liu, L., Liu, F., Gong, W., Liu, X., et al. (2012). Pre-treatment with IL-1β enhances the efficacy of MSC transplantation in DSS-induced colitis. Cell Mol. Immunol. 9, 473–481. doi:10.1038/cmi.2012.40
Fan, W., Li, J., Wang, Y., Pan, J., Li, S., Zhu, L., et al. (2016). CD105 promotes chondrogenesis of synovium-derived mesenchymal stem cells through Smad2 signaling. Biochem. Biophys. Res. Commun. 474, 338–344. doi:10.1016/j.bbrc.2016.04.101
Ferraz, M. P. (2023). Bone grafts in dental medicine: an overview of autografts, allografts and synthetic materials. Mater. (Basel) 16, 4117. doi:10.3390/ma16114117
Fichadiya, A., Bertram, K. L., Ren, G., Yates, R. M., and Krawetz, R. J. (2016). Characterizing heterogeneity in the response of synovial mesenchymal progenitor cells to synovial macrophages in normal individuals and patients with osteoarthritis. J. Inflamm. (Lond) 13, 12. doi:10.1186/s12950-016-0120-9
Friedenstein, A. J., Petrakova, K. V., Kurolesova, A. I., and Frolova, G. P. (1968). Heterotopic of bone marrow. Analysis of precursor cells for osteogenic and hematopoietic tissues. Available at: https://pubmed.ncbi.nlm.nih.gov/5654088/(Accessed March 15, 2023).
Fu, J., Wang, Y., Jiang, Y., Du, J., Xu, J., and Liu, Y. (2021). Systemic therapy of MSCs in bone regeneration: a systematic review and meta-analysis. Stem Cell Res. Ther. 12, 377. doi:10.1186/s13287-021-02456-w
Fukiage, K., Aoyama, T., Shibata, K. R., Otsuka, S., Furu, M., Kohno, Y., et al. (2008). Expression of vascular cell adhesion molecule-1 indicates the differentiation potential of human bone marrow stromal cells. Biochem. Biophys. Res. Commun. 365, 406–412. doi:10.1016/j.bbrc.2007.10.149
Furuta, T., Miyaki, S., Ishitobi, H., Ogura, T., Kato, Y., Kamei, N., et al. (2016). Mesenchymal stem cell-derived exosomes promote fracture healing in a mouse model. Stem Cells Transl. Med. 5, 1620–1630. doi:10.5966/sctm.2015-0285
Gaber, T., Schönbeck, K., Hoff, H., Tran, C. L., Strehl, C., Lang, A., et al. (2018). CTLA-4 mediates inhibitory function of mesenchymal stem/stromal cells. Int. J. Mol. Sci. 19, 2312. doi:10.3390/ijms19082312
Gan, Y., Dai, K., Zhang, P., Tang, T., Zhu, Z., and Lu, J. (2008). The clinical use of enriched bone marrow stem cells combined with porous beta-tricalcium phosphate in posterior spinal fusion. Biomaterials 29, 3973–3982. doi:10.1016/j.biomaterials.2008.06.026
Giannotti, S., Trombi, L., Bottai, V., Ghilardi, M., D’Alessandro, D., Danti, S., et al. (2013). Use of autologous human mesenchymal stromal cell/fibrin clot constructs in upper limb non-unions: long-term assessment. PLoS One 8, e73893. doi:10.1371/journal.pone.0073893
Glennie, S., Soeiro, I., Dyson, P. J., Lam, E. W.-F., and Dazzi, F. (2005). Bone marrow mesenchymal stem cells induce division arrest anergy of activated T cells. Blood 105, 2821–2827. doi:10.1182/blood-2004-09-3696
Gomez, I., Foudi, N., Longrois, D., and Norel, X. (2013). The role of prostaglandin E2 in human vascular inflammation. Prostagl. Leukot. Essent. Fat. Acids 89, 55–63. doi:10.1016/j.plefa.2013.04.004
Gómez-Barrena, E., Padilla-Eguiluz, N., Rosset, P., Gebhard, F., Hernigou, P., Baldini, N., et al. (2020). Early efficacy evaluation of mesenchymal stromal cells (MSC) combined to biomaterials to treat long bone non-unions. Injury 51 (Suppl. 1), S63–S73. doi:10.1016/j.injury.2020.02.070
Gómez-Barrena, E., Padilla-Eguiluz, N. G., Rosset, P., Hernigou, P., Baldini, N., Ciapetti, G., et al. (2021). Osteonecrosis of the femoral head safely healed with autologous, expanded, bone marrow-derived mesenchymal stromal cells in a multicentric trial with minimum 5 Years follow-up. J. Clin. Med. 10, 508. doi:10.3390/jcm10030508
Götherström, C., Westgren, M., Shaw, S. W. S., Aström, E., Biswas, A., Byers, P. H., et al. (2014). Pre- and postnatal transplantation of fetal mesenchymal stem cells in osteogenesis imperfecta: a two-center experience. Stem Cells Transl. Med. 3, 255–264. doi:10.5966/sctm.2013-0090
Granchi, D., Devescovi, V., Baglio, S. R., Magnani, M., Donzelli, O., and Baldini, N. (2012). A regenerative approach for bone repair in congenital pseudarthrosis of the tibia associated or not associated with type 1 neurofibromatosis: correlation between laboratory findings and clinical outcome. Cytotherapy 14, 306–314. doi:10.3109/14653249.2011.627916
Guo, Y., Ma, S., Wang, D., Mei, F., Guo, Y., Heng, B. C., et al. (2024). HtrA3 paves the way for MSC migration and promotes osteogenesis. Bioact. Mater 38, 399–410. doi:10.1016/j.bioactmat.2024.05.016
Hackel, A., Aksamit, A., Bruderek, K., Lang, S., and Brandau, S. (2021). TNF-α and IL-1β sensitize human MSC for IFN-γ signaling and enhance neutrophil recruitment. Eur. J. Immunol. 51, 319–330. doi:10.1002/eji.201948336
Hak, D. J., Fitzpatrick, D., Bishop, J. A., Marsh, J. L., Tilp, S., Schnettler, R., et al. (2014). Delayed union and nonunions: epidemiology, clinical issues, and financial aspects. Injury 45 (Suppl. 2), S3–S7. doi:10.1016/j.injury.2014.04.002
Han, J., Wang, W., Yu, X., Li, X., Wang, Z., Zhao, R., et al. (2021). Application and improvement of ipsilateral clavicle turnover during proximal humeral tumorous bone defect repair. Injury 52, 2941–2946. doi:10.1016/j.injury.2021.04.063
Hanetseder, D., Levstek, T., Teuschl-Woller, A. H., Frank, J. K., Schaedl, B., Redl, H., et al. (2023). Engineering of extracellular matrix from human iPSC-mesenchymal progenitors to enhance osteogenic capacity of human bone marrow stromal cells independent of their age. Front. Bioeng. Biotechnol. 11, 1214019. doi:10.3389/fbioe.2023.1214019
Harrell, C. R., Djonov, V., and Volarevic, V. (2021). The cross-talk between mesenchymal stem cells and immune cells in tissue repair and regeneration. Int. J. Mol. Sci. 22, 2472. doi:10.3390/ijms22052472
Harrell, C. R., Markovic, B. S., Fellabaum, C., Arsenijevic, N., Djonov, V., and Volarevic, V. (2020). The role of Interleukin 1 receptor antagonist in mesenchymal stem cell-based tissue repair and regeneration. Biofactors 46, 263–275. doi:10.1002/biof.1587
He, Y., Li, F., Jiang, P., Cai, F., Lin, Q., Zhou, M., et al. (2023). Remote control of the recruitment and capture of endogenous stem cells by ultrasound for in situ repair of bone defects. Bioact. Mater 21, 223–238. doi:10.1016/j.bioactmat.2022.08.012
Heo, J. S., Choi, Y., and Kim, H. O. (2019). Adipose-derived mesenchymal stem cells promote M2 macrophage phenotype through exosomes. Stem Cells Int. 2019, 1–10. doi:10.1155/2019/7921760
Hernigou, P., Dubory, A., Homma, Y., Guissou, I., Flouzat Lachaniette, C. H., Chevallier, N., et al. (2018). Cell therapy versus simultaneous contralateral decompression in symptomatic corticosteroid osteonecrosis: a thirty year follow-up prospective randomized study of one hundred and twenty five adult patients. Int. Orthop. 42, 1639–1649. doi:10.1007/s00264-018-3941-8
Hernigou, P., Hernigou, J., and Scarlat, M. (2021). Mesenchymal stem cell therapy improved outcome of early post-traumatic shoulder osteonecrosis: a prospective randomized clinical study of fifty patients with over ten year follow-up. Int. Orthop. 45, 2643–2652. doi:10.1007/s00264-021-05160-9
Hernigou, P., Pariat, J., Queinnec, S., Homma, Y., Flouzat Lachaniette, C. H., Chevallier, N., et al. (2014). Supercharging irradiated allografts with mesenchymal stem cells improves acetabular bone grafting in revision arthroplasty. Int. Orthop. 38, 1913–1921. doi:10.1007/s00264-014-2285-2
Herrmann, M., Stanić, B., Hildebrand, M., Alini, M., and Verrier, S. (2019). In vitro simulation of the early proinflammatory phase in fracture healing reveals strong immunomodulatory effects of CD146-positive mesenchymal stromal cells. J. Tissue Eng. Regen. Med. 13, 1466–1481. doi:10.1002/term.2902
Hochmann, S., Ou, K., Poupardin, R., Mittermeir, M., Textor, M., Ali, S., et al. (2023). The enhancer landscape predetermines the skeletal regeneration capacity of stromal cells. Sci. Transl. Med. 15, eabm7477. doi:10.1126/scitranslmed.abm7477
Hu, B., Zhang, Y., Zhang, G., Li, Z., Jing, Y., Yao, J., et al. (2022). Research progress of bone-targeted drug delivery system on metastatic bone tumors. J. Control Release 350, 377–388. doi:10.1016/j.jconrel.2022.08.034
Hu, G., Li, Q., Niu, X., Hu, B., Liu, J., Zhou, S., et al. (2015). Exosomes secreted by human-induced pluripotent stem cell-derived mesenchymal stem cells attenuate limb ischemia by promoting angiogenesis in mice. Stem Cell Res. Ther. 6, 10. doi:10.1186/scrt546
Hu, K., Shang, Z., Yang, X., Zhang, Y., and Cao, L. (2023). Macrophage polarization and the regulation of bone immunity in bone homeostasis. J. Inflamm. Res. 16, 3563–3580. doi:10.2147/JIR.S423819
Hu, T., Xu, H., Wang, C., Qin, H., and An, Z. (2018). Magnesium enhances the chondrogenic differentiation of mesenchymal stem cells by inhibiting activated macrophage-induced inflammation. Sci. Rep. 8, 3406. doi:10.1038/s41598-018-21783-2
Huang, C.-C., Kang, M., Lu, Y., Shirazi, S., Diaz, J. I., Cooper, L. F., et al. (2020). Functionally engineered extracellular vesicles improve bone regeneration. Acta Biomater. 109, 182–194. doi:10.1016/j.actbio.2020.04.017
Huang, S., Xu, L., Sun, Y., Zhang, Y., and Li, G. (2015). The fate of systemically administrated allogeneic mesenchymal stem cells in mouse femoral fracture healing. Stem Cell Res. Ther. 6, 206. doi:10.1186/s13287-015-0198-7
Ikeda, Y., Sakaue, M., Chijimatsu, R., Hart, D. A., Otsubo, H., Shimomura, K., et al. (2017). IGF-1 gene transfer to human synovial MSCs promotes their chondrogenic differentiation potential without induction of the hypertrophic phenotype. Stem Cells Int. 2017, 1–10. doi:10.1155/2017/5804147
Impieri, L., Pezzi, A., Hadad, H., Peretti, G. M., Mangiavini, L., and Rossi, N. (2024). Orthobiologics in delayed union and non-union of adult long bones fractures: a systematic review. Bone Rep. 21, 101760. doi:10.1016/j.bonr.2024.101760
Infante, A., Cabodevilla, L., Gener, B., and Rodríguez, C. I. (2022). Circulating TGF-β pathway in osteogenesis imperfecta pediatric patients subjected to MSCs-based cell therapy. Front. Cell Dev. Biol. 10, 830928. doi:10.3389/fcell.2022.830928
Infante, A., Gener, B., Vázquez, M., Olivares, N., Arrieta, A., Grau, G., et al. (2021). Reiterative infusions of MSCs improve pediatric osteogenesis imperfecta eliciting a pro-osteogenic paracrine response: TERCELOI clinical trial. Clin. Transl. Med. 11, e265. doi:10.1002/ctm2.265
Ito, W., Kanehiro, A., Matsumoto, K., Hirano, A., Ono, K., Maruyama, H., et al. (2005). Hepatocyte growth factor attenuates airway hyperresponsiveness, inflammation, and remodeling. Am. J. Respir. Cell Mol. Biol. 32, 268–280. doi:10.1165/rcmb.2004-0058OC
Jiang, M., Liu, L., Xiang, X., Liang, R., Qin, X., Zhao, J., et al. (2021). An MSC bone-homing compound, Rab001, increases bone mass and reduces the incidence of osteonecrosis in a glucocorticoid-induced osteonecrosis mouse model. Clin. Exp. Pharmacol. Physiol. 48, 770–781. doi:10.1111/1440-1681.13441
Jin, Q.-H., Kim, H.-K., Na, J.-Y., Jin, C., and Seon, J.-K. (2022). Anti-inflammatory effects of mesenchymal stem cell-conditioned media inhibited macrophages activation in vitro. Sci. Rep. 12, 4754. doi:10.1038/s41598-022-08398-4
Jones, E. A., Kinsey, S. E., English, A., Jones, R. A., Straszynski, L., Meredith, D. M., et al. (2002). Isolation and characterization of bone marrow multipotential mesenchymal progenitor cells. Arthritis Rheum. 46, 3349–3360. doi:10.1002/art.10696
Julie, R., Virginie, E., François, L., Régis, B., Julie, C., Séverine, B., et al. (2011). Formulated siRNAs targeting Rankl prevent osteolysis and enhance chemotherapeutic response in osteosarcoma models. J. bone mineral Res. 26, 2452–2462. doi:10.1002/jbmr.455
Kaibuchi, N., Iwata, T., Yamato, M., Okano, T., and Ando, T. (2016). Multipotent mesenchymal stromal cell sheet therapy for bisphosphonate-related osteonecrosis of the jaw in a rat model. Acta Biomater. 42, 400–410. doi:10.1016/j.actbio.2016.06.022
Kalia, P., Blunn, G. W., Miller, J., Bhalla, A., Wiseman, M., and Coathup, M. J. (2006). Do autologous mesenchymal stem cells augment bone growth and contact to massive bone tumor implants? Tissue Eng. 12, 1617–1626. doi:10.1089/ten.2006.12.1617
Kamali, A., Oryan, A., Hosseini, S., Ghanian, M. H., Alizadeh, M., Baghaban Eslaminejad, M., et al. (2019). Cannabidiol-loaded microspheres incorporated into osteoconductive scaffold enhance mesenchymal stem cell recruitment and regeneration of critical-sized bone defects. Mater Sci. Eng. C Mater Biol. Appl. 101, 64–75. doi:10.1016/j.msec.2019.03.070
Kang, J. S., Suh, Y. J., Moon, K. H., Park, J. S., Roh, T. H., Park, M. H., et al. (2018). Clinical efficiency of bone marrow mesenchymal stem cell implantation for osteonecrosis of the femoral head: a matched pair control study with simple core decompression. Stem Cell Res. Ther. 9, 274. doi:10.1186/s13287-018-1030-y
Kang, M., Huang, C.-C., Gajendrareddy, P., Lu, Y., Shirazi, S., Ravindran, S., et al. (2022). Extracellular vesicles from TNFα preconditioned MSCs: effects on immunomodulation and bone regeneration. Front. Immunol. 13, 878194. doi:10.3389/fimmu.2022.878194
Katagiri, W., Takeuchi, R., Saito, N., Suda, D., and Kobayashi, T. (2022). Migration and phenotype switching of macrophages at early-phase of bone-formation by secretomes from bone marrow derived mesenchymal stem cells using rat calvaria bone defect model. J. Dent. Sci. 17, 421–429. doi:10.1016/j.jds.2021.08.012
Keshavarz Shahbaz, S., Mansourabadi, A. H., and Jafari, D. (2022). Genetically engineered mesenchymal stromal cells as a new trend for treatment of severe acute graft-versus-host disease. Clin. Exp. Immunol. 208, 12–24. doi:10.1093/cei/uxac016
Kessler, F., Arnke, K., Eggerschwiler, B., Neldner, Y., Märsmann, S., Gröninger, O., et al. (2024). Murine iPSC-loaded scaffold grafts improve bone regeneration in critical-size bone defects. Int. J. Mol. Sci. 25, 5555. doi:10.3390/ijms25105555
Khan, A. A., Brandi, M. L., Rush, E. T., Ali, D. S., Al-Alwani, H., Almonaei, K., et al. (2024). Hypophosphatasia diagnosis: current state of the art and proposed diagnostic criteria for children and adults. Osteoporos. Int. 35, 431–438. doi:10.1007/s00198-023-06844-1
Kim, D. S., Jang, I. K., Lee, M. W., Ko, Y. J., Lee, D.-H., Lee, J. W., et al. (2018). Enhanced immunosuppressive properties of human mesenchymal stem cells primed by interferon-γ. EBioMedicine 28, 261–273. doi:10.1016/j.ebiom.2018.01.002
Kim, J., Kim, N. K., Park, S. R., and Choi, B. H. (2019). GM-CSF enhances mobilization of bone marrow mesenchymal stem cells via a CXCR4-medicated mechanism. Tissue Eng. Regen. Med. 16, 59–68. doi:10.1007/s13770-018-0163-5
Kim, Y.-K., and Ku, J.-K. (2020). Guided bone regeneration. J. Korean Assoc. Oral Maxillofac. Surg. 46, 361–366. doi:10.5125/jkaoms.2020.46.5.361
Kota, D. J., Prabhakara, K. S., Toledano-Furman, N., Bhattarai, D., Chen, Q., DiCarlo, B., et al. (2017). Prostaglandin E2 indicates therapeutic efficacy of mesenchymal stem cells in experimental traumatic brain injury. Stem Cells 35, 1416–1430. doi:10.1002/stem.2603
Kurniawan, A., Ivansyah, M. D., Dilogo, I. H., and Hutami, W. D. (2023). Umbilical cord mesenchymal stem cells combined with secretome for treating congenital pseudarthrosis of the Tibia: a case series. Eur. J. Orthop. Surg. Traumatol. 33, 2881–2888. doi:10.1007/s00590-023-03511-3
Labinsky, H., Panipinto, P. M., Ly, K. A., Khuat, D. K., Madarampalli, B., Mahajan, V., et al. (2020). Multiparameter analysis identifies heterogeneity in knee osteoarthritis synovial responses. Arthritis Rheumatol. 72, 598–608. doi:10.1002/art.41161
Lamoureux, F., Richard, P., Wittrant, Y., Battaglia, S., Pilet, P., Trichet, V., et al. (2007). Therapeutic relevance of osteoprotegerin gene therapy in osteosarcoma: blockade of the vicious cycle between tumor cell proliferation and bone resorption. Cancer Res. 67, 7308–7318. doi:10.1158/0008-5472.CAN-06-4130
Lauer, A., Wolf, P., Mehler, D., Götz, H., Rüzgar, M., Baranowski, A., et al. (2020). Biofabrication of SDF-1 functionalized 3D-printed cell-free scaffolds for bone tissue regeneration. Int. J. Mol. Sci. 21, 2175. doi:10.3390/ijms21062175
Lechanteur, C., Briquet, A., Giet, O., Delloye, O., Baudoux, E., and Beguin, Y. (2016). Clinical-scale expansion of mesenchymal stromal cells: a large banking experience. J. Transl. Med. 14, 145. doi:10.1186/s12967-016-0892-y
Lee, K.-D., Kuo, T. K.-C., Whang-Peng, J., Chung, Y.-F., Lin, C.-T., Chou, S.-H., et al. (2004). In vitro hepatic differentiation of human mesenchymal stem cells. Hepatology 40, 1275–1284. doi:10.1002/hep.20469
Lenna, S., Bellotti, C., Duchi, S., Martella, E., Columbaro, M., Dozza, B., et al. (2020). Mesenchymal stromal cells mediated delivery of photoactive nanoparticles inhibits osteosarcoma growth in vitro and in a murine in vivo ectopic model. J. Exp. Clin. Cancer Res. 39, 40. doi:10.1186/s13046-020-01548-4
Li, F., Wu, J., Li, D., Hao, L., Li, Y., Yi, D., et al. (2022). Engineering stem cells to produce exosomes with enhanced bone regeneration effects: an alternative strategy for gene therapy. J. Nanobiotechnology 20, 135. doi:10.1186/s12951-022-01347-3
Li, J., Xia, T., Zhao, Q., Wang, C., Fu, L., Zhao, Z., et al. (2024). Biphasic calcium phosphate recruits Tregs to promote bone regeneration. Acta Biomater. 176, 432–444. doi:10.1016/j.actbio.2024.01.001
Li, L., Li, J., Zou, Q., Zuo, Y., Cai, B., and Li, Y. (2019a). Enhanced bone tissue regeneration of a biomimetic cellular scaffold with co-cultured MSCs-derived osteogenic and angiogenic cells. Cell Prolif. 52, e12658. doi:10.1111/cpr.12658
Li, P., Li, S.-H., Wu, J., Zang, W.-F., Dhingra, S., Sun, L., et al. (2013). Interleukin-6 downregulation with mesenchymal stem cell differentiation results in loss of immunoprivilege. J. Cell Mol. Med. 17, 1136–1145. doi:10.1111/jcmm.12092
Li, W., Zhang, X., Wu, F., Zhou, Y., Bao, Z., Li, H., et al. (2019b). Gastric cancer-derived mesenchymal stromal cells trigger M2 macrophage polarization that promotes metastasis and EMT in gastric cancer. Cell Death Dis. 10, 918. doi:10.1038/s41419-019-2131-y
Liesveld, J. L., Sharma, N., and Aljitawi, O. S. (2020). Stem cell homing: from physiology to therapeutics. Stem Cells 38, 1241–1253. doi:10.1002/stem.3242
Lin, W., Li, Q., Zhang, D., Zhang, X., Qi, X., Wang, Q., et al. (2021). Mapping the immune microenvironment for mandibular alveolar bone homeostasis at single-cell resolution. Bone Res. 9, 17. doi:10.1038/s41413-021-00141-5
Liu, W., Li, L., Rong, Y., Qian, D., Chen, J., Zhou, Z., et al. (2020). Hypoxic mesenchymal stem cell-derived exosomes promote bone fracture healing by the transfer of miR-126. Acta Biomater. 103, 196–212. doi:10.1016/j.actbio.2019.12.020
Liu, X., Li, Q., Niu, X., Hu, B., Chen, S., Song, W., et al. (2017). Exosomes secreted from human-induced pluripotent stem cell-derived mesenchymal stem cells prevent osteonecrosis of the femoral head by promoting angiogenesis. Int. J. Biol. Sci. 13, 232–244. doi:10.7150/ijbs.16951
Liu, Y., Yang, R., and Shi, S. (2015). Systemic infusion of mesenchymal stem cells improves cell-based bone regeneration via upregulation of regulatory T cells. Tissue Eng. Part A 21, 498–509. doi:10.1089/ten.TEA.2013.0673
Lo Sicco, C., Reverberi, D., Balbi, C., Ulivi, V., Principi, E., Pascucci, L., et al. (2017). Mesenchymal stem cell-derived extracellular vesicles as mediators of anti-inflammatory effects: endorsement of macrophage polarization. Stem Cells Transl. Med. 6, 1018–1028. doi:10.1002/sctm.16-0363
Luz-Crawford, P., Kurte, M., Bravo-Alegría, J., Contreras, R., Nova-Lamperti, E., Tejedor, G., et al. (2013). Mesenchymal stem cells generate a CD4+CD25+Foxp3+ regulatory T cell population during the differentiation process of Th1 and Th17 cells. Stem Cell Res. Ther. 4, 65. doi:10.1186/scrt216
Machoň, V., Vlachopulos, V., and Beňo, M. (2022). Reconstruction of temporomandibular joint and skull base defect following osteosarcoma resection. J. Craniofac Surg. 33, e667–e669. doi:10.1097/SCS.0000000000008564
Mahon, O. R., Browe, D. C., Gonzalez-Fernandez, T., Pitacco, P., Whelan, I. T., Von Euw, S., et al. (2020). Nano-particle mediated M2 macrophage polarization enhances bone formation and MSC osteogenesis in an IL-10 dependent manner. Biomaterials 239, 119833. doi:10.1016/j.biomaterials.2020.119833
Mahon, O. R., O’Hanlon, S., Cunningham, C. C., McCarthy, G. M., Hobbs, C., Nicolosi, V., et al. (2018). Orthopaedic implant materials drive M1 macrophage polarization in a spleen tyrosine kinase- and mitogen-activated protein kinase-dependent manner. Acta Biomater. 65, 426–435. doi:10.1016/j.actbio.2017.10.041
Maizels, R. M., and Withers, D. R. (2014). MHC-II: a mutual support system for ILCs and T cells? Immunity 41, 174–176. doi:10.1016/j.immuni.2014.07.006
Marcacci, M., Kon, E., Moukhachev, V., Lavroukov, A., Kutepov, S., Quarto, R., et al. (2007). Stem cells associated with macroporous bioceramics for long bone repair: 6- to 7-year outcome of a pilot clinical study. Tissue Eng. 13, 947–955. doi:10.1089/ten.2006.0271
Marom, R., Lee, Y.-C., Grafe, I., and Lee, B. (2016). Pharmacological and biological therapeutic strategies for osteogenesis imperfecta. Am. J. Med. Genet. C Semin. Med. Genet. 172, 367–383. doi:10.1002/ajmg.c.31532
Matsuura, Y., Atsuta, I., Ayukawa, Y., Yamaza, T., Kondo, R., Takahashi, A., et al. (2016). Therapeutic interactions between mesenchymal stem cells for healing medication-related osteonecrosis of the jaw. Stem Cell Res. Ther. 7, 119. doi:10.1186/s13287-016-0367-3
Mázló, A., Kovács, R., Miltner, N., Tóth, M., Veréb, Z., Szabó, K., et al. (2021). MSC-like cells increase ability of monocyte-derived dendritic cells to polarize IL-17-/IL-10-producing T cells via CTLA-4. iScience 24, 102312. doi:10.1016/j.isci.2021.102312
McNeill, E. P., Zeitouni, S., Pan, S., Haskell, A., Cesarek, M., Tahan, D., et al. (2020). Characterization of a pluripotent stem cell-derived matrix with powerful osteoregenerative capabilities. Nat. Commun. 11, 3025. doi:10.1038/s41467-020-16646-2
Memeo, A., Verdoni, F., Minoli, C. F., Voto, A., D’Amato, R. D., Formiconi, F., et al. (2020). Effectiveness of bone marrow aspirate concentrate (BMAC) as adjuvant therapy in the surgical treatment of congenital pseudoarthrosis of the tibia: a retrospective comparative study. J. Biol. Regul. Homeost. Agents 34, 431–440. Congress of the Italian Orthopaedic Research Society.
Mengrui, W., Shali, W., Wei, C., and Yi-Ping, L. (2024). The roles and regulatory mechanisms of TGF-β and BMP signaling in bone and cartilage development, homeostasis and disease. Cell Res. 34, 101–123. doi:10.1038/s41422-023-00918-9
Mifune, Y., Matsumoto, T., Murasawa, S., Kawamoto, A., Kuroda, R., Shoji, T., et al. (2013). Therapeutic superiority for cartilage repair by CD271-positive marrow stromal cell transplantation. Cell Transpl. 22, 1201–1211. doi:10.3727/096368912X657378
Mohammed, E. E. A., Beherei, H. H., El-Zawahry, M., Farrag, A. R. H., Kholoussi, N., Helwa, I., et al. (2019). Combination of human amniotic fluid derived-mesenchymal stem cells and nano-hydroxyapatite scaffold enhances bone regeneration. Open Access Maced. J. Med. Sci. 7, 2739–2750. doi:10.3889/oamjms.2019.730
Muñoz, J., Akhavan, N. S., Mullins, A. P., and Arjmandi, B. H. (2020). Macrophage polarization and osteoporosis: a review. Nutrients 12, 2999. doi:10.3390/nu12102999
Murayama, M., Chow, S. K., Lee, M. L., Young, B., Ergul, Y. S., Shinohara, I., et al. (2024). The interactions of macrophages, lymphocytes, and mesenchymal stem cells during bone regeneration. Bone Jt. Res. 13, 462–473. doi:10.1302/2046-3758.139.BJR-2024-0122.R1
Murray, P. J. (2017). Macrophage polarization. Annu. Rev. Physiol. 79, 541–566. doi:10.1146/annurev-physiol-022516-034339
Najar, M., Raicevic, G., Fayyad-Kazan, H., De Bruyn, C., Bron, D., Toungouz, M., et al. (2012). Immune-related antigens, surface molecules and regulatory factors in human-derived mesenchymal stromal cells: the expression and impact of inflammatory priming. Stem Cell Rev. Rep. 8, 1188–1198. doi:10.1007/s12015-012-9408-1
Nakao, M., Inanaga, D., Nagase, K., and Kanazawa, H. (2019). Characteristic differences of cell sheets composed of mesenchymal stem cells with different tissue origins. Regen. Ther. 11, 34–40. doi:10.1016/j.reth.2019.01.002
Nakao, Y., Fukuda, T., Zhang, Q., Sanui, T., Shinjo, T., Kou, X., et al. (2021). Exosomes from TNF-α-treated human gingiva-derived MSCs enhance M2 macrophage polarization and inhibit periodontal bone loss. Acta Biomater. 122, 306–324. doi:10.1016/j.actbio.2020.12.046
Németh, K., Leelahavanichkul, A., Yuen, P. S. T., Mayer, B., Parmelee, A., Doi, K., et al. (2009). Bone marrow stromal cells attenuate sepsis via prostaglandin E(2)-dependent reprogramming of host macrophages to increase their interleukin-10 production. Nat. Med. 15, 42–49. doi:10.1038/nm.1905
Niu, Y., Li, Q., Xie, R., Liu, S., Wang, R., Xing, P., et al. (2017). Modulating the phenotype of host macrophages to enhance osteogenesis in MSC-laden hydrogels: design of a glucomannan coating material. Biomaterials 139, 39–55. doi:10.1016/j.biomaterials.2017.05.042
Ode, A., Schoon, J., Kurtz, A., Gaetjen, M., Ode, J. E., Geissler, S., et al. (2013). CD73/5’-ecto-nucleotidase acts as a regulatory factor in osteo-/chondrogenic differentiation of mechanically stimulated mesenchymal stromal cells. Eur. Cell Mater 25, 37–47. doi:10.22203/ecm.v025a03
Ogata, K., Katagiri, W., and Hibi, H. (2017). Secretomes from mesenchymal stem cells participate in the regulation of osteoclastogenesis in vitro. Clin. Oral Investig. 21, 1979–1988. doi:10.1007/s00784-016-1986-x
Ogata, K., Matsumura, M., Moriyama, M., Katagiri, W., Hibi, H., and Nakamura, S. (2018). Cytokine mixtures mimicking secretomes from mesenchymal stem cells improve medication-related osteonecrosis of the jaw in a rat model. JBMR Plus 2, 69–80. doi:10.1002/jbm4.10013
Oh, J. Y., Kim, H., Lee, H. J., Lee, K., Barreda, H., Kim, H. J., et al. (2022). MHC class I enables MSCs to evade NK-Cell-Mediated cytotoxicity and exert immunosuppressive activity. Stem Cells 40, 870–882. doi:10.1093/stmcls/sxac043
Omoto, M., Katikireddy, K. R., Rezazadeh, A., Dohlman, T. H., and Chauhan, S. K. (2014). Mesenchymal stem cells home to inflamed ocular surface and suppress allosensitization in corneal transplantation. Invest. Ophthalmol. Vis. Sci. 55, 6631–6638. doi:10.1167/iovs.14-15413
Ostanin, A. A., Petrovskii, Y. L., Shevela, E. Y., and Chernykh, E. R. (2011). Multiplex analysis of cytokines, chemokines, growth factors, MMP-9 and TIMP-1 produced by human bone marrow, adipose tissue, and placental mesenchymal stromal cells. Bull. Exp. Biol. Med. 151, 133–141. doi:10.1007/s10517-011-1275-2
Osugi, M., Katagiri, W., Yoshimi, R., Inukai, T., Hibi, H., and Ueda, M. (2012). Conditioned media from mesenchymal stem cells enhanced bone regeneration in rat calvarial bone defects. Tissue Eng. Part A 18, 1479–1489. doi:10.1089/ten.TEA.2011.0325
Otsuru, S., Gordon, P. L., Shimono, K., Jethva, R., Marino, R., Phillips, C. L., et al. (2012). Transplanted bone marrow mononuclear cells and MSCs impart clinical benefit to children with osteogenesis imperfecta through different mechanisms. Blood 120, 1933–1941. doi:10.1182/blood-2011-12-400085
Pachón-Peña, G., Yu, G., Tucker, A., Wu, X., Vendrell, J., Bunnell, B. A., et al. (2011). Stromal stem cells from adipose tissue and bone marrow of age-matched female donors display distinct immunophenotypic profiles. J. Cell Physiol. 226, 843–851. doi:10.1002/jcp.22408
Paganelli, A., Trubiani, O., Diomede, F., Pisciotta, A., and Paganelli, R. (2021). Immunomodulating profile of dental mesenchymal stromal cells: a comprehensive overview. Front. Oral Health 2, 635055. doi:10.3389/froh.2021.635055
Pan, W., Li, S., Li, K., and Zhou, P. (2024). Mesenchymal stem cells and extracellular vesicles: therapeutic potential in organ transplantation. Stem Cells Int. 2024, 2043550. doi:10.1155/2024/2043550
Park, M.-J., Shin, J.-S., Kim, Y.-H., Hong, S.-H., Yang, S.-H., Shin, J.-Y., et al. (2011). Murine mesenchymal stem cells suppress T lymphocyte activation through IL-2 receptor α (CD25) cleavage by producing matrix metalloproteinases. Stem Cell Rev. Rep. 7, 381–393. doi:10.1007/s12015-010-9203-9
Park, S., Rahaman, K. A., Kim, Y.-C., Jeon, H., and Han, H.-S. (2024). Fostering tissue engineering and regenerative medicine to treat musculoskeletal disorders in bone and muscle. Bioact. Mater 40, 345–365. doi:10.1016/j.bioactmat.2024.06.022
Pawitan, J. A., Bui, T. A., Mubarok, W., Antarianto, R. D., Nurhayati, R. W., Dilogo, I. H., et al. (2020). Enhancement of the therapeutic capacity of mesenchymal stem cells by genetic modification: a systematic review. Front. Cell Dev. Biol. 8, 587776. doi:10.3389/fcell.2020.587776
Peihong, S., Ye, T., Chaofei, Y., Xiaoli, M., Xue, W., Jiawei, P., et al. (2018). Mesenchymal stem cell migration during bone formation and bone diseases therapy. Int. J. Mol. Sci. 19, 2343. doi:10.3390/ijms19082343
Petri, R. M., Hackel, A., Hahnel, K., Dumitru, C. A., Bruderek, K., Flohe, S. B., et al. (2017). Activated tissue-resident mesenchymal stromal cells regulate natural killer cell immune and tissue-regenerative function. Stem Cell Rep. 9, 985–998. doi:10.1016/j.stemcr.2017.06.020
Picke, A.-K., Campbell, G. M., Blüher, M., Krügel, U., Schmidt, F. N., Tsourdi, E., et al. (2018). Thy-1 (CD90) promotes bone formation and protects against obesity. Sci. Transl. Med. 10, eaao6806. doi:10.1126/scitranslmed.aao6806
Pierre, A., Louis-Romée, L. N., Meadhbh Á, B., Philippe, R., Gonzague, D. P., Pierre, L., et al. (2015). Mesenchymal stem cells increase proliferation but do not change quiescent state of osteosarcoma cells: potential implications according to the tumor resection status. J. bone Oncol. 5. doi:10.1016/j.jbo.2015.11.002
Qi, J., Zhang, R., and Wang, Y. (2021). Exosomal miR-21-5p derived from bone marrow mesenchymal stem cells promote osteosarcoma cell proliferation and invasion by targeting PIK3R1. J. Cell Mol. Med. 25, 11016–11030. doi:10.1111/jcmm.17024
Qiao, B., Shui, W., Cai, L., Guo, S., and Jiang, D. (2015). Human mesenchymal stem cells as delivery of osteoprotegerin gene: homing and therapeutic effect for osteosarcoma. DDDT 9, 969–976. doi:10.2147/DDDT.S77116
Qiu, S., Cao, L., Xiang, D., Wang, S., Wang, D., Qian, Y., et al. (2024). Enhanced osteogenic differentiation in 3D hydrogel scaffold via macrophage mitochondrial transfer. J. Nanobiotechnology 22, 540. doi:10.1186/s12951-024-02757-1
Rahyussalim, A. J., Al As’ady, F. M., Nasser, M. K., and Kurniawati, T. (2023). Comprehensive approach to degenerative spine with fragility spine fracture to improve quality of life: a case report study. Int. J. Surg. Case Rep. 105, 107978. doi:10.1016/j.ijscr.2023.107978
Ren, G., Zhang, L., Zhao, X., Xu, G., Zhang, Y., Roberts, A. I., et al. (2008). Mesenchymal stem cell-mediated immunosuppression occurs via concerted action of chemokines and nitric oxide. Cell Stem Cell 2, 141–150. doi:10.1016/j.stem.2007.11.014
Riester, O., Borgolte, M., Csuk, R., and Deigner, H.-P. (2020). Challenges in bone tissue regeneration: stem cell therapy, biofunctionality and antimicrobial properties of novel materials and its evolution. Int. J. Mol. Sci. 22, 192. doi:10.3390/ijms22010192
Rodríguez-Lozano, F. J., Oñate-Sánchez, R., Gonzálvez-García, M., Vallés-Bergadá, M., Martínez, C. M., Revilla-Nuin, B., et al. (2020). Allogeneic bone marrow mesenchymal stem cell transplantation in tooth extractions sites ameliorates the incidence of osteonecrotic jaw-like lesions in zoledronic acid-treated rats. J. Clin. Med. 9, 1649. doi:10.3390/jcm9061649
Sacchetti, B., Funari, A., Remoli, C., Giannicola, G., Kogler, G., Liedtke, S., et al. (2016). No identical “mesenchymal stem cells” at different times and sites: human committed progenitors of distinct origin and differentiation potential are incorporated as adventitial cells in microvessels. Stem Cell Rep. 6, 897–913. doi:10.1016/j.stemcr.2016.05.011
Saldaña, L., Vallés, G., Bensiamar, F., Mancebo, F. J., García-Rey, E., and Vilaboa, N. (2017). Paracrine interactions between mesenchymal stem cells and macrophages are regulated by 1,25-dihydroxyvitamin D3. Sci. Rep. 7, 14618. doi:10.1038/s41598-017-15217-8
Sasaki, T., Akagi, R., Akatsu, Y., Fukawa, T., Hoshi, H., Yamamoto, Y., et al. (2017). The effect of systemic administration of G-CSF on a full-thickness cartilage defect in a rabbit model MSC proliferation as presumed mechanism: G-CSF for cartilage repair. Bone and Jt. Res. 6, 123–131. doi:10.1302/2046-3758.63.BJR-2016-0083
Schemitsch, E. H. (2017). Size matters: defining critical in bone defect size. J. Orthop. Trauma 31 (Suppl. 5), S20–S22. doi:10.1097/BOT.0000000000000978
Schena, F., Gambini, C., Gregorio, A., Mosconi, M., Reverberi, D., Gattorno, M., et al. (2010). Interferon-γ-dependent inhibition of B cell activation by bone marrow-derived mesenchymal stem cells in a murine model of systemic lupus erythematosus. Arthritis Rheum. 62, 2776–2786. doi:10.1002/art.27560
Schindeler, A., McDonald, M. M., Bokko, P., and Little, D. G. (2008). Bone remodeling during fracture repair: the cellular picture. Semin. Cell Dev. Biol. 19, 459–466. doi:10.1016/j.semcdb.2008.07.004
Schoonraad, S. A., Jaimes, A. A., Singh, A. J. X., Croland, K. J., and Bryant, S. J. (2023). Osteogenic effects of covalently tethered rhBMP-2 and rhBMP-9 in an MMP-sensitive PEG hydrogel nanocomposite. Acta Biomater. 170, 53–67. doi:10.1016/j.actbio.2023.08.045
Seebach, E., Freischmidt, H., Holschbach, J., Fellenberg, J., and Richter, W. (2014). Mesenchymal stroma cells trigger early attraction of M1 macrophages and endothelial cells into fibrin hydrogels, stimulating long bone healing without long-term engraftment. Acta Biomater. 10, 4730–4741. doi:10.1016/j.actbio.2014.07.017
Semaya, A. E.-S., Badawy, E., Hasan, M., and El-Nakeeb, R. M. (2016). Management of post-traumatic bone defects of the tibia using vascularised fibular graft combined with Ilizarov external fixator. Injury 47, 969–975. doi:10.1016/j.injury.2016.01.033
Shah, K. N., Racine, J., Jones, L. C., and Aaron, R. K. (2015). Pathophysiology and risk factors for osteonecrosis. Curr. Rev. Musculoskelet. Med. 8, 201–209. doi:10.1007/s12178-015-9277-8
Shahir, M., Mahmoud Hashemi, S., Asadirad, A., Varahram, M., Kazempour-Dizaji, M., Folkerts, G., et al. (2020). Effect of mesenchymal stem cell-derived exosomes on the induction of mouse tolerogenic dendritic cells. J. Cell Physiol. 235, 7043–7055. doi:10.1002/jcp.29601
Shao, K., Koch, C., Gupta, M. K., Lin, Q., Lenz, M., Laufs, S., et al. (2013). Induced pluripotent mesenchymal stromal cell clones retain donor-derived differences in DNA methylation profiles. Mol. Ther. 21, 240–250. doi:10.1038/mt.2012.207
Shen, B., Liu, J., Zhang, F., Wang, Y., Qin, Y., Zhou, Z., et al. (2016). CCR2 positive exosome released by mesenchymal stem cells suppresses macrophage functions and alleviates ischemia/reperfusion-induced renal injury. Stem Cells Int. 2016, 1240301. doi:10.1155/2016/1240301
Shen, F., and Shi, Y. (2021). Recent advances in single-cell view of mesenchymal stem cell in osteogenesis. Front. Cell Dev. Biol. 9, 809918. doi:10.3389/fcell.2021.809918
Sheng, G. (2015). The developmental basis of mesenchymal stem/stromal cells (MSCs). BMC Dev. Biol. 15, 44. doi:10.1186/s12861-015-0094-5
Sheyn, D., Ben-David, S., Shapiro, G., De Mel, S., Bez, M., Ornelas, L., et al. (2016). Human induced pluripotent stem cells differentiate into functional mesenchymal stem cells and repair bone defects. Stem Cells Transl. Med. 5, 1447–1460. doi:10.5966/sctm.2015-0311
Shim, J., Kim, K.-T., Kim, K. G., Choi, U.-Y., Kyung, J. W., Sohn, S., et al. (2021). Safety and efficacy of Wharton’s jelly-derived mesenchymal stem cells with teriparatide for osteoporotic vertebral fractures: a phase I/IIa study. Stem Cells Transl. Med. 10, 554–567. doi:10.1002/sctm.20-0308
Shiota, M., Heike, T., Haruyama, M., Baba, S., Tsuchiya, A., Fujino, H., et al. (2007). Isolation and characterization of bone marrow-derived mesenchymal progenitor cells with myogenic and neuronal properties. Exp. Cell Res. 313, 1008–1023. doi:10.1016/j.yexcr.2006.12.017
Shoaib, Z., Fan, T. M., and Irudayaraj, J. M. K. (2022). Osteosarcoma mechanobiology and therapeutic targets. Br. J. Pharmacol. 179, 201–217. doi:10.1111/bph.15713
Singh, V., Singh, M., Belbase, R. J., and Rastogi, A. (2021). Healing of artificially created gap non-union using autologous cultured osteoblasts impregnated over three-dimensional biodegradable scaffold: an experimental study (rabbit). Indian J. Orthop. 55, 460–465. doi:10.1007/s43465-020-00288-z
Sivakumar, P. M., Yetisgin, A. A., Demir, E., Sahin, S. B., and Cetinel, S. (2023). Polysaccharide-bioceramic composites for bone tissue engineering: a review. Int. J. Biol. Macromol. 250, 126237. doi:10.1016/j.ijbiomac.2023.126237
Sole, A., Grossetête, S., Heintzé, M., Babin, L., Zaïdi, S., Revy, P., et al. (2021). Unraveling ewing sarcoma tumorigenesis originating from patient-derived mesenchymal stem cells. Cancer Res. 81, 4994–5006. doi:10.1158/0008-5472.CAN-20-3837
Song, N., Scholtemeijer, M., and Shah, K. (2020). Mesenchymal stem cell immunomodulation: mechanisms and therapeutic potential. Trends Pharmacol. Sci. 41, 653–664. doi:10.1016/j.tips.2020.06.009
Ströjby, S., Eberstål, S., Svensson, A., Fritzell, S., Bexell, D., Siesjö, P., et al. (2014). Intratumorally implanted mesenchymal stromal cells potentiate peripheral immunotherapy against malignant rat gliomas. J. Neuroimmunol. 274, 240–243. doi:10.1016/j.jneuroim.2014.07.014
Sun, K., Lin, H., Tang, Y., Xiang, S., Xue, J., Yin, W., et al. (2020). Injectable BMP-2 gene-activated scaffold for the repair of cranial bone defect in mice. Stem Cells Transl. Med. 9, 1631–1642. doi:10.1002/sctm.19-0315
Tadokoro, M., Kanai, R., Taketani, T., Uchio, Y., Yamaguchi, S., and Ohgushi, H. (2009). New bone formation by allogeneic mesenchymal stem cell transplantation in a patient with perinatal hypophosphatasia. J. Pediatr. 154, 924–930. doi:10.1016/j.jpeds.2008.12.021
Takahashi, K., and Yamanaka, S. (2006). Induction of pluripotent stem cells from mouse embryonic and adult fibroblast cultures by defined factors. Cell 126, 663–676. doi:10.1016/j.cell.2006.07.024
Taketani, T., Oyama, C., Mihara, A., Tanabe, Y., Abe, M., Hirade, T., et al. (2015). Ex vivo expanded allogeneic mesenchymal stem cells with bone marrow transplantation improved osteogenesis in infants with severe hypophosphatasia. Cell Transpl. 24, 1931–1943. doi:10.3727/096368914X685410
Tian, R., Zheng, F., Zhao, W., Zhang, Y., Yuan, J., Zhang, B., et al. (2020). Prevalence and influencing factors of nonunion in patients with tibial fracture: systematic review and meta-analysis. J. Orthop. Surg. Res. 15, 377. doi:10.1186/s13018-020-01904-2
Tiemessen, M. M., Jagger, A. L., Evans, H. G., van Herwijnen, M. J. C., John, S., and Taams, L. S. (2007). CD4+CD25+Foxp3+ regulatory T cells induce alternative activation of human monocytes/macrophages. Proc. Natl. Acad. Sci. U. S. A. 104, 19446–19451. doi:10.1073/pnas.0706832104
Toya, M., Zhang, N., Tsubosaka, M., Kushioka, J., Gao, Q., Li, X., et al. (2023). CCL2 promotes osteogenesis by facilitating macrophage migration during acute inflammation. Front. Cell Dev. Biol. 11, 1213641. doi:10.3389/fcell.2023.1213641
Tsai, M.-L., Tsai, Y.-G., Lin, Y.-C., Hsu, Y.-L., Chen, Y.-T., Tsai, M.-K., et al. (2021). IL-25 induced ROS-mediated M2 macrophage polarization via AMPK-associated mitophagy. Int. J. Mol. Sci. 23, 3. doi:10.3390/ijms23010003
Tyrina, E. A., Andreeva, E. R., and Buravkova, L. B. (2023). Simulated microgravity affects stroma-dependent ex vivo myelopoiesis. Tissue Cell 80, 101987. doi:10.1016/j.tice.2022.101987
Ueda, S., Shimasaki, M., Ichiseki, T., Ueda, Y., Tsuchiya, M., Kaneuji, A., et al. (2017). Prevention of glucocorticoid-associated osteonecrosis by intravenous administration of mesenchymal stem cells in a rabbit model. BMC Musculoskelet. Disord. 18, 480. doi:10.1186/s12891-017-1837-1
Ueno, M., Lo, C.-W., Barati, D., Conrad, B., Lin, T., Kohno, Y., et al. (2020). Interleukin-4 overexpressing mesenchymal stem cells within gelatin-based microribbon hydrogels enhance bone healing in a murine long bone critical-size defect model. J. Biomed. Mater Res. A 108, 2240–2250. doi:10.1002/jbm.a.36982
Ullah, M., Liu, D. D., and Thakor, A. S. (2019). Mesenchymal stromal cell homing: mechanisms and strategies for improvement. iScience 15, 421–438. doi:10.1016/j.isci.2019.05.004
Ulusoy, İ., Yılmaz, M., and Kıvrak, A. (2023). Efficacy of autologous stem cell therapy in femoral head avascular necrosis: a comparative study. J. Orthop. Surg. Res. 18, 799. doi:10.1186/s13018-023-04297-0
Van Royen, K., Brems, H., Legius, E., Lammens, J., and Laumen, A. (2016). Prevalence of neurofibromatosis type 1 in congenital pseudarthrosis of the tibia. Eur. J. Pediatr. 175, 1193–1198. doi:10.1007/s00431-016-2757-z
Venkataiah, V. S., Yahata, Y., Kitagawa, A., Inagaki, M., Kakiuchi, Y., Nakano, M., et al. (2021). Clinical applications of cell-scaffold constructs for bone regeneration therapy. Cells 10, 2687. doi:10.3390/cells10102687
Waghray, M., Yalamanchili, M., Dziubinski, M., Zeinali, M., Erkkinen, M., Yang, H., et al. (2016). GM-CSF mediates mesenchymal-epithelial cross-talk in pancreatic cancer. Cancer Discov. 6, 886–899. doi:10.1158/2159-8290.CD-15-0947
Wahl, S. M., Orenstein, J. M., and Chen, W. (2000). TGF-β influences the life and death decisions of T lymphocytes. Cytokine Growth Factor Rev. 11, 71–79. doi:10.1016/s1359-6101(99)00030-1
Wang, X., Wang, Y., Gou, W., Lu, Q., Peng, J., and Lu, S. (2013). Role of mesenchymal stem cells in bone regeneration and fracture repair: a review. Int. Orthop. 37, 2491–2498. doi:10.1007/s00264-013-2059-2
Wang, Z., Han, L., Sun, T., Wang, W., Li, X., and Wu, B. (2021). Construction of tissue-engineered bone with differentiated osteoblasts from adipose-derived stem cell and coral scaffolds at an ectopic site. Br. J. Oral Maxillofac. Surg. 59, 46–51. doi:10.1016/j.bjoms.2020.07.006
Whelan, D. S., Caplice, N. M., and Clover, A. J. P. (2020). Mesenchymal stromal cell derived CCL2 is required for accelerated wound healing. Sci. Rep. 10, 2642. doi:10.1038/s41598-020-59174-1
Xiang, Z., Jiong-Ming, Y., Xiao-Jun, D., and Yang, W. (2020). Administration of mircoRNA-135b-reinforced exosomes derived from MSCs ameliorates glucocorticoid-induced osteonecrosis of femoral head (ONFH) in rats. J. Cell. Mol. Med. 24, 13973–13983. doi:10.1111/jcmm.16006
Xie, C., Liang, R., Ye, J., Peng, Z., Sun, H., Zhu, Q., et al. (2022). High-efficient engineering of osteo-callus organoids for rapid bone regeneration within one month. Biomaterials 288, 121741. doi:10.1016/j.biomaterials.2022.121741
Xie, J., Peng, C., Zhao, Q., Wang, X., Yuan, H., Yang, L., et al. (2016). Osteogenic differentiation and bone regeneration of iPSC-MSCs supported by a biomimetic nanofibrous scaffold. Acta Biomater. 29, 365–379. doi:10.1016/j.actbio.2015.10.007
Xiongfa, J., Xi, Y., Limin, M., Bo, B., Hao, Z., Zehua, L., et al. (2020). Mesenchymal stem cell-loaded thermosensitive hydroxypropyl chitin hydrogel combined with a three-dimensional-printed poly(ε-caprolactone)/nano-hydroxyapatite scaffold to repair bone defects via osteogenesis, angiogenesis and immunomodulation. Theranostics 10, 725–740. doi:10.7150/thno.39167
Yang, S.-H., Park, M.-J., Yoon, I.-H., Kim, S.-Y., Hong, S.-H., Shin, J.-Y., et al. (2009). Soluble mediators from mesenchymal stem cells suppress T cell proliferation by inducing IL-10. Exp. Mol. Med. 41, 315–324. doi:10.3858/emm.2009.41.5.035
Yang, Y., Otte, A., and Hass, R. (2015). Human mesenchymal stroma/stem cells exchange membrane proteins and alter functionality during interaction with different tumor cell lines. Stem Cells Dev. 24, 1205–1222. doi:10.1089/scd.2014.0413
Yang, Z. X., Han, Z.-B., Ji, Y. R., Wang, Y. W., Liang, L., Chi, Y., et al. (2013). CD106 identifies a subpopulation of mesenchymal stem cells with unique immunomodulatory properties. PLoS One 8, e59354. doi:10.1371/journal.pone.0059354
Ye, D., Chen, C., Wang, Q., Zhang, Q., Li, S., and Liu, H. (2020). Short-wave enhances mesenchymal stem cell recruitment in fracture healing by increasing HIF-1 in callus. Stem Cell Res. Ther. 11, 382. doi:10.1186/s13287-020-01888-0
Yellowley, C. (2013). CXCL12/CXCR4 signaling and other recruitment and homing pathways in fracture repair. Bonekey Rep. 2, 300. doi:10.1038/bonekey.2013.34
Yi, H., Wang, Y., Liang, Q., and Mao, X. (2022). Preclinical and clinical amelioration of bone fractures with mesenchymal stromal cells: a systematic review and meta-analysis. Cell Transpl. 31, 9636897211051743. doi:10.1177/09636897211051743
Zarei, M., Hasanzadeh Azar, M., Sayedain, S. S., Shabani Dargah, M., Alizadeh, R., Arab, M., et al. (2023). Material extrusion additive manufacturing of poly(lactic acid)/Ti6Al4V@calcium phosphate core-shell nanocomposite scaffolds for bone tissue applications. Int. J. Biol. Macromol. 255, 128040. doi:10.1016/j.ijbiomac.2023.128040
Zhang, J., Liu, X., Li, H., Chen, C., Hu, B., Niu, X., et al. (2016). Exosomes/tricalcium phosphate combination scaffolds can enhance bone regeneration by activating the PI3K/Akt signaling pathway. Stem Cell Res. Ther. 7, 136. doi:10.1186/s13287-016-0391-3
Zhang, W., Ge, W., Li, C., You, S., Liao, L., Han, Q., et al. (2004). Effects of mesenchymal stem cells on differentiation, maturation, and function of human monocyte-derived dendritic cells. Stem Cells Dev. 13, 263–271. doi:10.1089/154732804323099190
Zhang, Y., Hao, Z., Wang, P., Xia, Y., Wu, J., Xia, D., et al. (2019). Exosomes from human umbilical cord mesenchymal stem cells enhance fracture healing through HIF-1α-mediated promotion of angiogenesis in a rat model of stabilized fracture. Cell Prolif. 52, e12570. doi:10.1111/cpr.12570
Zhang, Y., Liang, J., Liu, P., Wang, Q., Liu, L., and Zhao, H. (2022). The RANK/RANKL/OPG system and tumor bone metastasis: potential mechanisms and therapeutic strategies. Front. Endocrinol. (Lausanne) 13, 1063815. doi:10.3389/fendo.2022.1063815
Zhao, W., Beers, D. R., Thonhoff, J. R., Thome, A. D., Faridar, A., Wang, J., et al. (2020a). Immunosuppressive functions of M2 macrophages derived from iPSCs of patients with ALS and healthy controls. iScience 23, 101192. doi:10.1016/j.isci.2020.101192
Zhao, X., Zhao, Y., Sun, X., Xing, Y., Wang, X., and Yang, Q. (2020b). Immunomodulation of MSCs and MSC-derived extracellular vesicles in osteoarthritis. Front. Bioeng. Biotechnol. 8, 575057. doi:10.3389/fbioe.2020.575057
Zhou, M., Xi, J., Cheng, Y., Sun, D., Shu, P., Chi, S., et al. (2021). Reprogrammed mesenchymal stem cells derived from iPSCs promote bone repair in steroid-associated osteonecrosis of the femoral head. Stem Cell Res. Ther. 12, 175. doi:10.1186/s13287-021-02249-1
Zhu, W., Zhang, X., Jiang, Y., Liu, X., Huang, L., Wei, Q., et al. (2020). Alterations in peripheral T cell and B cell subsets in patients with osteoarthritis. Clin. Rheumatol. 39, 523–532. doi:10.1007/s10067-019-04768-y
Keywords: mesenchymal stem cells, bone defect, bone microenvironment, biomaterial, bone tissue engineering
Citation: Zhang Y, Fan M and Zhang Y (2024) Revolutionizing bone defect healing: the power of mesenchymal stem cells as seeds. Front. Bioeng. Biotechnol. 12:1421674. doi: 10.3389/fbioe.2024.1421674
Received: 22 April 2024; Accepted: 10 October 2024;
Published: 21 October 2024.
Edited by:
Fei Liu, Texas A and M University, United StatesReviewed by:
Simin Pan, Texas A and M University, United StatesArash Shahsavari, Texas A and M University, United States
Copyright © 2024 Zhang, Fan and Zhang. This is an open-access article distributed under the terms of the Creative Commons Attribution License (CC BY). The use, distribution or reproduction in other forums is permitted, provided the original author(s) and the copyright owner(s) are credited and that the original publication in this journal is cited, in accordance with accepted academic practice. No use, distribution or reproduction is permitted which does not comply with these terms.
*Correspondence: Yingze Zhang, eXpsaW5nX2xpdUAxNjMuY29t
†These authors have contributed equally to this work and share first authorship