- 1The First Outpatient Department, Jilin Provincial Key Laboratory of Tooth Development and Bone Remodeling, School and Hospital of Stomatology, Jilin University, Changchun, China
- 2Foshan Stomatology Hospital and School of Medicine, Foshan, Guangdong, China
Periodontal defects involve the damage and loss of periodontal tissue, primarily caused by periodontitis. This inflammatory disease, resulting from various factors, can lead to irreversible harm to the tissues supporting the teeth if not treated effectively, potentially resulting in tooth loss or loosening. Such outcomes significantly impact a patient’s facial appearance and their ability to eat and speak. Current clinical treatments for periodontitis, including surgery, root planing, and various types of curettage, as well as local antibiotic injections, aim to mitigate symptoms and halt disease progression. However, these methods fall short of fully restoring the original structure and functionality of the affected tissue, due to the complex and deep structure of periodontal pockets and the intricate nature of the supporting tissue. To overcome these limitations, numerous biomaterials have been explored for periodontal tissue regeneration, with hydrogels being particularly noteworthy. Hydrogels are favored in research for their exceptional absorption capacity, biodegradability, and tunable mechanical properties. They have shown promise as barrier membranes, scaffolds, carriers for cell transplantation and drug delivery systems in periodontal regeneration therapy. The review concludes by discussing the ongoing challenges and future prospects for hydrogel applications in periodontal treatment.
1 Introduction
Periodontitis, a chronic condition leading to the inflammatory destruction of periodontal supporting tissues, is a primary cause of tooth loss in adults. With an estimated 1.1 billion people worldwide suffering from severe periodontitis, the disease significantly compromises chewing efficiency and causes eating difficulties. Additionally, the cosmetic effects of periodontitis, such as changes to the jawline and appearance when smiling due to receding gums and loose teeth, can adversely affect social interactions and the patient’s quality of life (Kononen et al., 2019; Wen et al., 2021). Despite current clinical therapies for periodontitis, including surgical interventions, root planing, and various scaling techniques, along with local antibiotic injections, aiming to alleviate symptoms and manage the disease progression (Deas et al., 2016; Slots, 2017; Kwon et al., 2021; Wadia, 2021), a full restoration of periodontal tissue function and structure remains unachieved. Consequently, there is an ongoing search for innovative therapeutic strategies, such as guided tissue regeneration (GTR)/guided bone regeneration (GBR), bone grafts, growth factors, stem cell therapy, and periodontal tissue engineering, to repair the structure and function of damaged periodontal tissues. While there have been advancements, challenges remain in enhancing the success and predictability of these treatments (Sam and Pillai, 2014; Giannobile and McClain, 2015; Hu et al., 2018), highlighting the demand for efficient and safe periodontal regeneration methods.
Strategies for periodontal regeneration are categorized into GTR and periodontal tissue engineering. The concept of GTR was introduced by Nyman et al. in 1982 (Gottlow et al., 1990), emphasizing the use of membranes to prevent gingival epithelium from expanding toward the tooth root, thereby encouraging osteoblasts and periodontal ligament stem cells (PDLSCs) to form new attachments (Gottlow et al., 1990). The selection and application of barrier membranes are crucial for the effectiveness of GTR technology, which offers both absorbable and non-absorbable varieties (Alqahtani, 2023; Zhu et al., 2023). Non-absorbable membranes, such as titanium and polytetrafluoroethylene, are known for their excellent mechanical and biocompatibility properties, suitable for independent usage in clinical settings (Mizraji et al., 2023). However, their non-resorbable nature necessitates a second surgical procedure for removal, increasing the risk of infection and potentially damaging newly formed periodontal tissue. In contrast, absorbable membranes, made from synthetic or natural polymers like polylactic acid, eliminate the need for a second surgery as they are absorbed by the body during the healing process. These membranes are typically cell-affine, facilitating cell attachment and proliferation, and are easy to handle. However, absorbable membranes possess relatively low mechanical strength and a degradation rate that is difficult to control precisely, potentially limiting their effectiveness in periodontal regeneration (Sasaki et al., 2021). The success of GTR technology in periodontal regeneration, despite its widespread application, depends on several factors including the specific tooth anatomy, the precision of the surgical procedure, postoperative care, and proper indication selection (Villar and Cochran, 2010). Therefore, for enhanced effectiveness and therapeutic outcomes of periodontal regeneration, physicians implementing GTR treatments must consider these variables.
Tissue engineering for periodontal regeneration is an interdisciplinary field that merges clinical medicine, biology, and materials science (Liu et al., 2018; Liang et al., 2020), aiming to rebuild damaged periodontal tissues through the use of stem cells, growth factors, and scaffold materials (Han et al., 2014). A typical approach involves isolating and culturing specific cells in vitro, seeding them onto a scaffold that mimics the target tissue’s natural extracellular matrix (ECM), and then implanting the assembly into the patient’s target site. Stem cells are crucial for periodontal tissue engineering due to their self-renewal capability and potential to differentiate into various cell types (Iwasaki et al., 2022). Stem cells for periodontal tissue are often derived from dental pulp, bone marrow mesenchymal stem cells, and PDLSCs (Gao et al., 2024), which can differentiate into specific periodontal cells such as osteoblasts and fibroblasts, aiding in tissue regeneration. Growth factors play a vital role in regulating cell behavior and promoting cell migration, proliferation, differentiation, and neovascularization in periodontal tissue regeneration (Kale et al., 2022). Scaffold materials serve as the foundation for tissue engineering, offering a structure for cell attachment, growth, and differentiation. Current periodontal scaffolds are made from synthetic materials like polycaprolactone (PCL) and polylactic acid-glycolic acid copolymer (PLGA), as well as natural materials like collagen (Daghrery et al., 2023; Ostrovidov et al., 2023). Despite significant progress, periodontal tissue engineering faces challenges, including the need to improve the success rate of tissue engineering repairs, as outcomes are often unpredictable, and ensuring the stability of in vivo applications (Galli et al., 2021). The complexity of periodontal tissue, comprising intricate tissue architectures and diverse types, means current treatments cannot fully restore the tissue’s original structure and function. Thus, periodontal regeneration remains a complex and challenging domain, requiring ongoing scientific research and technological advancements to address existing challenges (de Jong et al., 2017).
Hydrogels have shown considerable promise in clinical periodontal tissue regeneration, offering a biomimetic environment similar to natural biological tissues and the ECM (Sánchez-Cid et al., 2022). These polymeric materials can absorb and retain large amounts of water while maintaining a solid structure, thanks to a three-dimensional network of polymer chains interconnected through physical or chemical cross-links. Their high water content endows hydrogels with softness and biocompatibility, fostering an ideal setting for cell adhesion, proliferation, differentiation, and growth (Radulescu et al., 2022). The physicochemical properties of hydrogels can be customized by adjusting their functional elements, cross-linking methods, and synthetic ingredients, allowing them to meet the specific mechanical and biological requirements of different tissues. Hydrogels have found applications in drug delivery, regenerative medicine (Dimatteo et al., 2018; Mantha et al., 2019; Tavakoli and Klar, 2020) and more, thanks to their ability to be tailored for specific medical uses (Jacob et al., 2021). The primary ingredients of natural hydrogels are polysaccharides and proteins, such as gelatin, hyaluronic acid, and chitosan (CS). These substances are favored for their natural origins. However, their low mechanical strength and limited stability render them less suitable for clinical applications, despite their proven biosafety and biodegradability in both animal and clinical trials (Catoira et al., 2019). By contrast, synthetic hydrogels are formed through physical or chemical crosslinking and include polymers like polyvinyl alcohol. These hydrogels offer superior mechanical properties and consistent mass stability, but the presence of unreacted monomers, initiators, or crosslinkers can cause inflammation or cytotoxicity (Branco et al., 2022). To enhance the qualities of composites, hydrogel can also be used in combination with other materials (Wang Y. et al., 2020; Patel and Koh, 2020).
This study describes the use of hydrogels in periodontal tissue engineering, focusing on their roles as GTR barrier membranes, scaffolds, carriers for cell transplantation and drug delivery systems (Scheme 1). It highlights the research progress of hydrogels in different application directions, and the potential for further development of hydrogel materials to aid in the regeneration of periodontal tissues.
2 Hydrogels as guided tissue regeneration/guided bone regeneration materials
In GTR and GBR, the membrane plays a crucial role in the regeneration of periodontal tissue. An ideal barrier membrane should possess several characteristics (Gao et al., 2022; Mirzaeei et al., 2022): First, it must be biocompatible with the surrounding periodontal tissue to minimize immune responses and inflammation. Second, it should have adequate mechanical strength and flexibility to maintain its shape and provide necessary space for new tissue growth during surgery. Furthermore, the membrane should feature moderate porosity to prevent epithelial cell invasion into the bone defect area while allowing the exchange of growth stimuli and nutrients. Biodegradability is another important factor, requiring the membrane to degrade at a rate compatible with periodontal tissue regeneration. Lastly, the material should be easy to handle clinically, including cutting, placing, and securing. Recent research has focused on improving the biomechanical properties of GTR/GBR membranes and introducing new therapeutic functionalities, such as osteoinduction or antibacterial activity (Gao et al., 2022; Mirzaeei et al., 2022). Due to their excellent biosafety and adjustable properties, hydrogels are considered as potential materials for GTR/GBR membranes (Alauddin et al., 2022). For instance, a study by Humber et al. (2010) demonstrated the effectiveness of an in situ formed polyethylene glycol hydrogel membrane as a GBR barrier in supporting bone tissue repair. However, the use of hydrogels as GTR/GBR membranes faces challenges, particularly in mechanical performance, often failing to meet clinical requirements. Therefore, ongoing research aims to enhance the biomechanical properties of hydrogels through formula optimization, cross-linking density adjustment, and the inclusion of functional additives to broaden their therapeutic applications.
Enhancing the biomechanical characteristics of hydrogels can be achieved through the incorporation of inorganic fillers (Bee and Hamid, 2022). Commonly used inorganic materials in periodontal tissue engineering include inorganic phosphate ceramics such as hydroxyapatite (HA) and β-tricalcium phosphate. Akansha Kishen et al. (2023) introduced a novel hydroxyapatite-reinforced polymer hydrogel membrane by combining hydroxyapatite with alginate, a naturally occurring polymer known for its excellent biological compatibility and biodegradable properties, capable of forming a gel structure in the body (Zhang et al., 2021). Given hydroxyapatite’s osteoinductive qualities, it enhanced the hydrogel membrane’s mechanical strength and accelerated periodontal tissue regeneration, suggesting its potential in periodontal regeneration therapy as a GTR membrane. The asymmetric barrier membrane developed by He et al. (2021) consisted of agarose, hydroxyapatite carbonate (CHA), and ε-polylysine. CHA is derived from HA through chemical modification, maintaining HA’s high mechanical strength and biocompatibility while enhancing bone conductivity. This enhancement supports bone tissue development and regeneration (Wang et al., 2024). Increasing the CHA concentration in the hydrogel significantly raised the storage modulus of the barrier membrane. Specifically, a CHA content of 10% resulted in a 30 kPa increase in storage modulus compared to pure agarose gel, indicating that adjusting the CHA content can enhance the membrane’s mechanical properties and its barrier effectiveness. Moreover, membranes with higher CHA concentrations showed increased mineralization capacity and osteogenesis-promoting effects. The incorporation of ε-polylysine provided antimicrobial properties, useful in preventing or reducing periodontitis recurrence. Thus, this barrier membrane offers improved mechanical properties, bone tissue regeneration promotion, and antimicrobial defense, contributing significantly to GTR for periodontal tissue regeneration and rebuilding.
The addition of graphite and its derivatives into hydrogels notably enhances their mechanical properties. Graphene, the primary conductive carbon-based substance, is recognized for its excellent biological activity and bone repair effects (Sattar, 2019). It is one of the strongest materials known, exhibiting remarkable toughness, biocompatibility, stability, thermal conductivity, and a large specific surface area. These features make graphene widely used in biosensing, bioimaging, and tissue regeneration. The structural and mechanical properties of hydrogels, such as toughness, tear strength, and elastic modulus, can be influenced by graphene through mechanisms such as hydrogen bonding, electrostatic interactions, and hydrophobicity (Zare et al., 2024). Lu et al. (2016) described a graphene hydrogel (MGH) membrane consisting of multilayered chemically modified graphene sheets, notable for its strength and flexibility. The membrane’s average tensile modulus was comparable to that of rat skull tissue, ensuring strong adherence to defects in rat skull models and stability post-transplantation. Microcomputed tomography (CT) images verified new bone tissue development beneath the MGH membrane after 8 weeks, indicating its role in physical support and osteoblast growth promotion, thereby facilitating bone tissue regeneration. Consequently, MGH membranes present a novel approach for the clinical management of bone defects as GBR membranes.
Fibers are materials made up of continuous or discontinuous filaments that possess significant stiffness. However, they face challenges in forming three-dimensional (3D) structures. To overcome this, numerous studies have focused on incorporating them into hydrogels to create composite materials that leverage the strengths of both components. Fiber-reinforced hydrogels, for example, are enhanced by the addition of fibers, making their structure more akin to the ECM, thereby promoting better cellular functions (Khorshidi and Karkhaneh, 2018). ε-caprolactone is converted into PCL, a polymer with a low melting point, through ring-opening polymerization (Hernandez et al., 2017). This material is biocompatible and versatile, thanks to 3D printing technologies. Dubey et al. (2020) developed a highly porous PCL network via melt electrowriting, which was then integrated into a gelatin methacryloyl (GelMA) hydrogel supported by amorphous magnesium phosphate. This integration significantly improved the hydrogel’s mechanical properties, enabling it to withstand nearly 70 kPa of stress under 20% deformation. This enhancement not only helped the hydrogel maintain its structure under stress but also acted as an effective barrier against epithelial cell invasion. The fibrous network structure of the hydrogel facilitated a faster rate of mineralization when used as a GBR membrane, supporting bone tissue formation more effectively. This enhancement was attributed to the rigid support of the PCL network, which also slowed down degradation, thereby extending its lifespan in vivo and providing sustained support for bone tissue regeneration. Overall, this bioactive, fiber-reinforced hydrogel membrane presents significant potential for GBR applications.
A double network (DN) hydrogel comprises two interpenetrating polymer networks, characterized by a loose network structure and a polyelectrolyte network structure with a high cross-linking density. This configuration imparts unique structural characteristics to the DN hydrogel (Zhou et al., 2020; Li S. et al., 2021; Hanyková et al., 2023), including a stable support from the cross-linked neutral network, which also absorbs external stress through the polyelectrolyte network structure. Besides maintaining the physical properties of conventional hydrogels, the dual network significantly improves toughness and mechanical properties (Ning et al., 2022), offering a synergistic performance that enhances mechanical strength and inhibits phase separation (Huang et al., 2023). Chichiricco et al. (2018) developed a photochemically cross-linked hydrogel membrane by combining a silylated hydroxypropyl methylcellulose network with a methacrylated carboxymethyl CS network, achieving rapid gelation. The presence of the second polymer reduced gelation time significantly, forming a hydrogel membrane within 2 min of irradiation. This rapid curing process, combined with the membrane’s adaptability to wound shapes, enhances the cell barrier effect, making it easier to use during surgery. Furthermore, the double network structure provided a synergistic increase in mechanical strength, evidenced by a higher Young’s modulus compared to single-network hydrogels. This enhancement in mechanical properties, attributed to the supportive interaction between the two networks, makes the hydrogel membrane particularly valuable for applications requiring robust barrier membranes, such as in periodontal regeneration therapy.
Bacterial infections are a prevalent complication with medical implants. Additionally, microbes cause periodontitis, a serious gum disease. The site of periodontal tissue healing often becomes contaminated, which hampers effective periodontal regeneration. Therefore, ensuring the antibacterial efficacy of GTR/GBR barrier membranes is crucial. Incorporating antibacterial substances into these membranes helps control bacterial colonization and reduces infection risk during the initial healing stages. The most common antibacterial agents used in GTR/GBR are antibiotics, such as minocycline, doxycycline, metronidazole, and vancomycin (Toledano-Osorio et al., 2022). A study introduced the broad-spectrum antibiotic chloramphenicol and the local anesthetic lidocaine into a bioresorbable hydrogel membrane, creating a drug-eluting system with potential for periodontal GTR (Vasconcelos et al., 2022). However, excessive reliance on antibiotics can lead to increased multidrug resistance and reduced treatment effectiveness. To overcome these challenges, alternative strategies are necessary to complement or replace traditional antibiotic approaches. Natural materials have been used since ancient times in the antibacterial field to enhance therapeutic efficacy and reduce bacterial resistance. These can be utilized either alone or alongside conventional antibiotics (Malczak and Gajda, 2023). Hinokitiol, a natural compound from monoterpenoids with antibacterial properties, inhibits bacterial cell respiration, DNA, and protein synthesis (Chang et al., 2019). By using N-(3-dimethylaminopropyl)-N′-ethylcarbodiimide hydrochloride to cross-link gelatin and hyaluronic acid, and incorporating hinokitiol, Chang et al. (2021) developed a hydrogel-regenerated membrane. This membrane, laden with hinokitiol, showed potent antibacterial activity against both Staphylococcus aureus and Escherichia coli. Its antibacterial strength significantly increased with higher hinokitiol concentrations. Additionally, the hydrophobic properties of juniper mercaptan slowed the hydrogel membrane’s degradation, prolonging the antibacterial agent’s release. This sustained release mechanism enables the membrane to maintain an effective antimicrobial effect for at least 2 weeks, offering a promising antimicrobial treatment option. Chitin, known for its biocompatibility, biodegradability, non-toxicity, and antibacterial properties, can be transformed into CS, a polysaccharide widely used in biomedicine (Elizalde-Cárdenas et al., 2024). Carboxymethyl chitosan, a chemically modified version of CS, can be grafted with a photosensitive methacrylic acid group to create a novel polymer. This polymer quickly formed a 3D network architecture when exposed to blue light, making it suitable as a barrier membrane due to its biocompatible, degradable, and antibacterial characteristics (Xing et al., 2022).
Metals and metal oxides serve as broad-spectrum, long-acting antimicrobials, killing bacteria through the dissolution of metal ions (Abo-Zeid and Williams, 2020)and the generation of reactive oxygen species (ROS), which oxidize biological macromolecules (Kannan et al., 2020). Xu et al. (2020) developed a polydopamine-modified titania nanoparticle and cuprous oxide-doped injectable sodium alginate hydrogel (TiO2@PDA), which exhibited peroxidase-like activity under blue light, generating ROS to eliminate bacteria effectively and support early debridement. The ROS generated by TiO2@PDA also transformed Cu+ into Cu2+, enhancing the growth and osteogenic differentiation of bone mesenchymal stem cells (BMSCs). Moreover, polydopamine granted the titania nanoparticles a photothermal effect, producing thermal energy under near-infrared light to further promote bone regeneration. The unique light-sensitive properties of dual-photoresponsive composite hydrogels enableed them to alter their structure or chemistry under varying light conditions, seamlessly transitioning between antimicrobial and osteogenic functions (Figure 1). This adaptability allows the material to meet the specific needs of the treatment phase. For instance, the composite hydrogels can release ROS at targeted light wavelengths for strong antimicrobial action, or adjust light conditions to promote bone tissue growth. Additionally, non-metallic photosensitizers like temoporfin can be incorporated into the barrier membrane, offering a photodynamic antibacterial effect under appropriate light exposure.
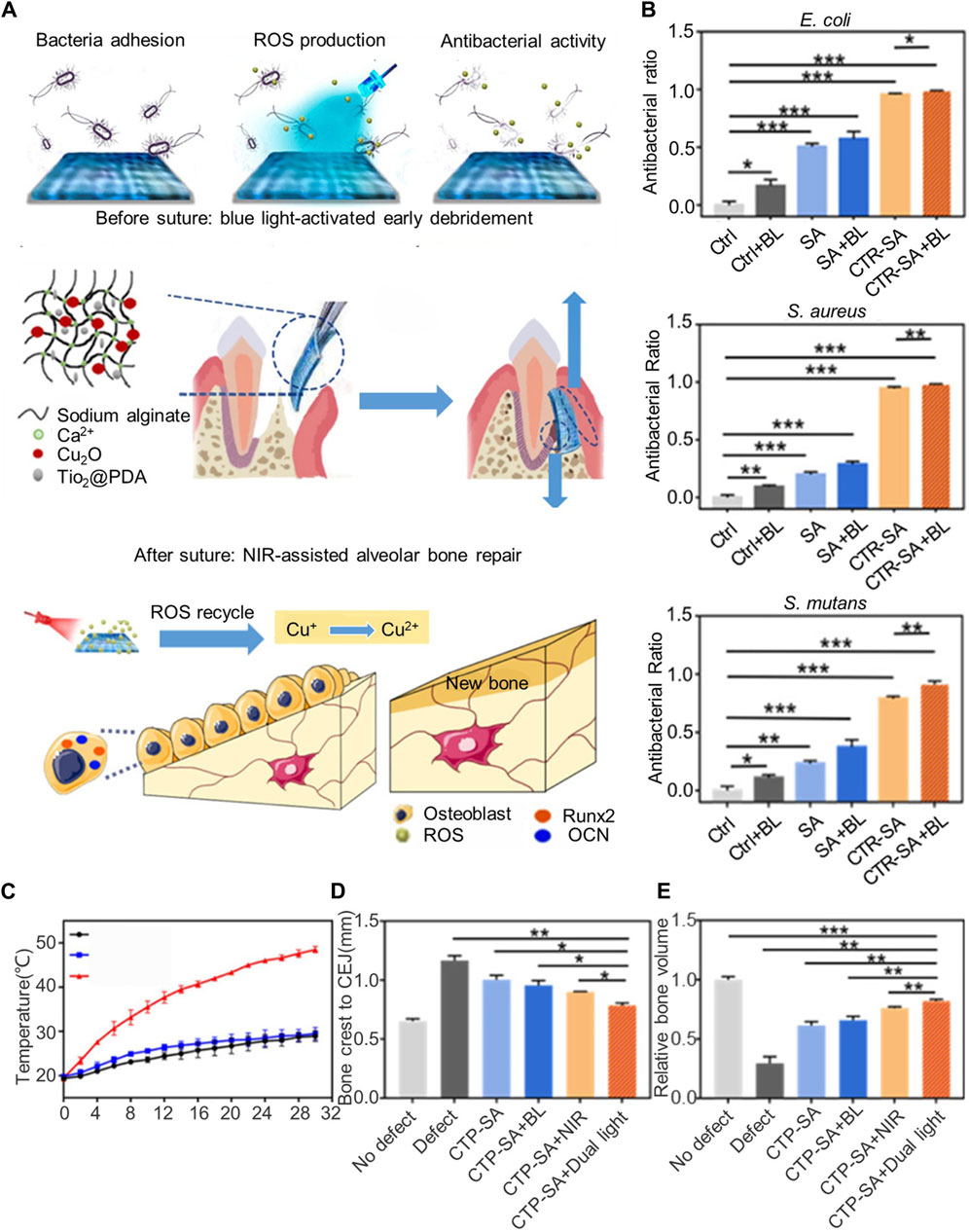
Figure 1. Dual-light-tunable sodium alginate hydrogel used as a barrier membrane for GTR with antibacterial/osteogenic mode switch properties. (A) Schematic illustration of the material composition, experimental procedure, and therapeutic mechanism of CTP-SA. (B) In vitro antibacterial effects of blue light irradiation on Escherichia coli, Staphylococcus aureus, and Streptococcus mutans. (C) Photothermal curves under NIR irradiation. (D,E) Evaluation of the effect of dual light-modulated hydrogels on osteogenesis in vivo. (D) Quantitative statistics of the distance from the bone crest to CEJ. (E) Relative bone volume. Reprinted with permission from Xu et al. (2020). Copyright (2020) American Chemical Society.
Growth factors play a crucial role in regenerative medicine by enhancing the repair and regeneration of damaged tissues. Incorporating growth factors into GBR/GTR membranes results in their sustained release in the target area, thus more effectively stimulating the differentiation and proliferation of osteoprogenitor cells or similar cells, which speeds up the tissue regeneration process (Mirzaeei et al., 2022). Consequently, hydrogel bio-barrier membranes serve as effective carriers of growth factors, like bone morphogenetic proteins, to boost tissue regeneration efficiency (He et al., 2021). Considering the tendency of implants to displace and the frequent compression of periodontal tissues by external forces leading to suboptimal regeneration, recent research has focused on enhancing the adhesion capabilities of barrier membranes to resist external stimuli. Li et al. (2023) introduced a dual-layer adhesive barrier membrane combining a collagen membrane with a polyacrylamide/polydopamine hydrogel. The dopamine’s catechol group chemically bonded to the bone surface, creating a strong adhesive effect. In tests, this membrane demonstrated high adhesive strength, exceeding 90 kPa, ensuring a robust bond to the bone surface. When used with bone graft material, it effectively retained nearly all the grafts, in contrast to the minimal retention observed with traditional collagen membranes. This indicated its superior fixation and bone repair capabilities, with significant alveolar bone regeneration observed 25 weeks post-transplantation.
Advances in materials science have significantly propelled the regeneration of periodontal and bone tissues. The evolution from early non-absorbable membranes to later absorbable ones, and now to contemporary multifunctional bio-barrier membranes that combine the advantages of both, has markedly advanced regenerative medicine technology. Hydrogel bio-barrier membranes have demonstrated promising clinical application potential in both cell and animal models, promoting cell adhesion and proliferation and providing an ideal microenvironment for tissue regeneration. Nonetheless, further research into the precise biological mechanisms of these hydrogel materials in clinical settings is necessary to fully optimize their efficacy.
3 Hydrogels for periodontal tissue engineering
3.1 Hydrogels as scaffolds
Scaffolds play a pivotal role in tissue engineering, aiming to replicate the composition of the ECM by providing 3D structural support and guidance for both exogenous and endogenous cells (Yamada et al., 2022). Hydrogels are particularly valued in this field for their unique 3D network structure and high water content, resembling the natural ECM closely (El-Sherbiny and Yacoub, 2013). To enhance tissue regeneration, numerous innovative hydrogel scaffolds have been developed to improve the interaction between target tissue cells and scaffold materials.
When designing hydrogels to serve as scaffolds for periodontal regeneration, it is crucial to consider the material, structure, and functionalization techniques to ensure they meet specific design requirements (Gorriz et al., 2015; Naahidi et al., 2017). These include the following: (a) biocompatibility: It is essential that the hydrogel scaffolds are compatible with the target tissue. After their synthesis, any remaining monomers, initiators, or other components not involved in the reaction could harm the tissue. Therefore, careful selection of materials and synthesis processes, followed by thorough purification, is necessary to minimize any potential adverse effects; (b) biodegradability: Hydrogel scaffolds should degrade and resorb in a controlled manner in vivo; (c) porosity: A key property of hydrogel scaffolds is their porosity (Chen et al., 2018), which allows nutrients and oxygen to reach cells and waste products from metabolism to be expelled. Suitable, interconnected pores will facilitate cell growth into the scaffold, and uniformly distributed cells will aid in tissue regeneration. Pore characteristics, including size, shape, and volume distribution, are crucial factors affecting a scaffold’s porosity. These characteristics influence cell penetration distance within the scaffold. Mechanical strength is essential for the scaffold to integrate into the damaged area and support weight. Additionally, the hydrogel scaffold’s surface properties are vital for influencing cell adhesion and proliferation by interacting with surrounding tissue. Through comprehensive consideration and optimization of these characteristics, hydrogel scaffolds can provide an ideal 3D microenvironment that significantly promotes the repair of periodontal tissue. Pan et al. (2020) developed an injectable hydrogel scaffold containing nano-hydroxyapatite based on polysaccharides. This scaffold showcased excellent microstructure and mechanical properties, along with a porous structure that supports the migration of endogenous stem cells. After implantation in a rat model of alveolar bone defects for 4 weeks, the scaffold completely degraded, leading to substantial alveolar bone regeneration without any significant inflammatory reactions. Additionally, incorporating nano-hydroxyapatite into porous hydrogel scaffolds made from polyvinyl alcohol and alginate (Bahrami et al., 2019) could mitigate the scaffolds’ mechanical weaknesses, increasing their compressive strength to approximately 3.51 MPa. These alginate/polyvinyl alcohol scaffolds with hydroxyapatite nanoparticles also exhibited superior cell attachment and promoted growth and differentiation of PDLSCs into osteoblasts, thus creating an optimal environment for periodontal tissue repair.
In tissue engineering, selecting and using the right scaffold materials is crucial. Scaffolds provide the necessary 3D structure for cell adhesion and growth but often lack the intrinsic activity to stimulate cell proliferation and division. To enhance scaffold functionality, researchers frequently incorporate growth factors or other bioactive molecules. Incorporating growth factors into hydrogel scaffolds enables localized, controlled release of specific active ingredients, delivering vital bioactive signals at crucial tissue regeneration stages, fostering cellular responses, and guiding tissue repair and regeneration. He et al. (2019) developed high-stiffness transglutaminase gels enriched with interleukin-4 and/or stromal cell-derived factor-1α (SDF-1α) as scaffolds, which demonstrated that interleukin-4 could induce macrophages to adopt an anti-inflammatory M2 phenotype, promoting osteogenic development of BMSCs in vitro. Additionally, SDF-1α consistently enhanced the recruitment of BMSCs, resulting in effective bone regeneration. In another study, Momose et al. (2016) achieved alveolar bone reconstruction using a collagen hydrogel scaffold loaded with fibroblast growth factor-2 in a beagle dog model of a class II bifurcation deficit, without any abnormal healing. Similarly, collagen hydrogel scaffolds combined with bone morphogenetic protein-2 (BMP-2) have been shown to induce effective periodontal repair (Kato et al., 2015). Tan et al. (2019) encapsulated bioactive components in supramolecular hydrogels to promote alveolar bone reconstruction, co-assembling the biocompatible hydrogel agent Nap-Phe-Phe-Tyr-OH (NapFFY) with SDF-1 and BMP-2. Their findings indicated that these bioactive substances could be synchronously and continuously released, significantly enhancing BMSC recruitment, osteogenic differentiation, and alveolar bone regeneration. Rats treated with the SDF-1/BMP-2/NapFFY hydrogel for 8 weeks exhibited a significantly higher bone regeneration rate (56.7% bone volume fraction) compared with controls. The results of the study revealed that two bioactive compounds could be synchronously and continuously released from a hydrogel, effectively enhancing the recruitment of BMSCs, osteogenic differentiation, and the regeneration of alveolar bone. Rats with bone defects, treated with the SDF-1/BMP-2/NapFFY hydrogel for 8 weeks, experienced significantly improved bone regeneration, with a bone volume fraction of 56.7%, compared to the control group.
During periodontal regeneration, the hydrogel scaffold acts as carriers for stem cell transplantation. The differentiation of these stem cells can be further promoted by altering the hydrogel scaffold, thereby impacting the regeneration of periodontal tissue. Utilizing a 4-amino-DL-phenylalanine (Phe-NH) dynamic acyl hydrazone crosslinker, Yang et al. (2021) developed an injectable chitin hydrogel system. This method encapsulated rat bone marrow-derived stem cells (rMSCs) within the hydrogel. After 7 days, the rMSCs were evenly distributed throughout the hydrogel, with over 95% cell survival. The hydrogel was observed to boost the differentiation of rMSCs, as indicated by a marked increase in marker expression. For periodontal tissue regeneration, scaffolds, external stem cells, and growth factors can be used in combination. For periodontal tissue regeneration, scaffolds, external stem cells, and growth factors can be used in combination. Isaac et al. (2019) created polyethylene glycol hydrogel microparticles using immersion electrospray, then formed microporous annealed granular hydrogels (TzMAP hydrogels) by linking norbornene-containing polyethylene glycol hydrogel microspheres through tetrazine click chemistry. Integrating PDLSCs during annealing showed that cell viability after 24 h showed that the survival rate of PDLSCs was approximately 87% ± 5%. The incorporation of PDLSCs and platelet-derived growth factor-BB (PDGF-BB) into TzMAP resulted in increased cell proliferation and migration, foundational for periodontal tissue regeneration (Figure 2). Ammar et al. (2018) mixed platelet concentrates into thermosensitive CS/β-glycerophosphate hydrogels, demonstrating that TGF-β1 and PDGF-BB could be continuously released for up to 2 weeks, thereby sustaining the viability of encapsulated PDLSCs for 7 days and promoting periodontal regeneration.
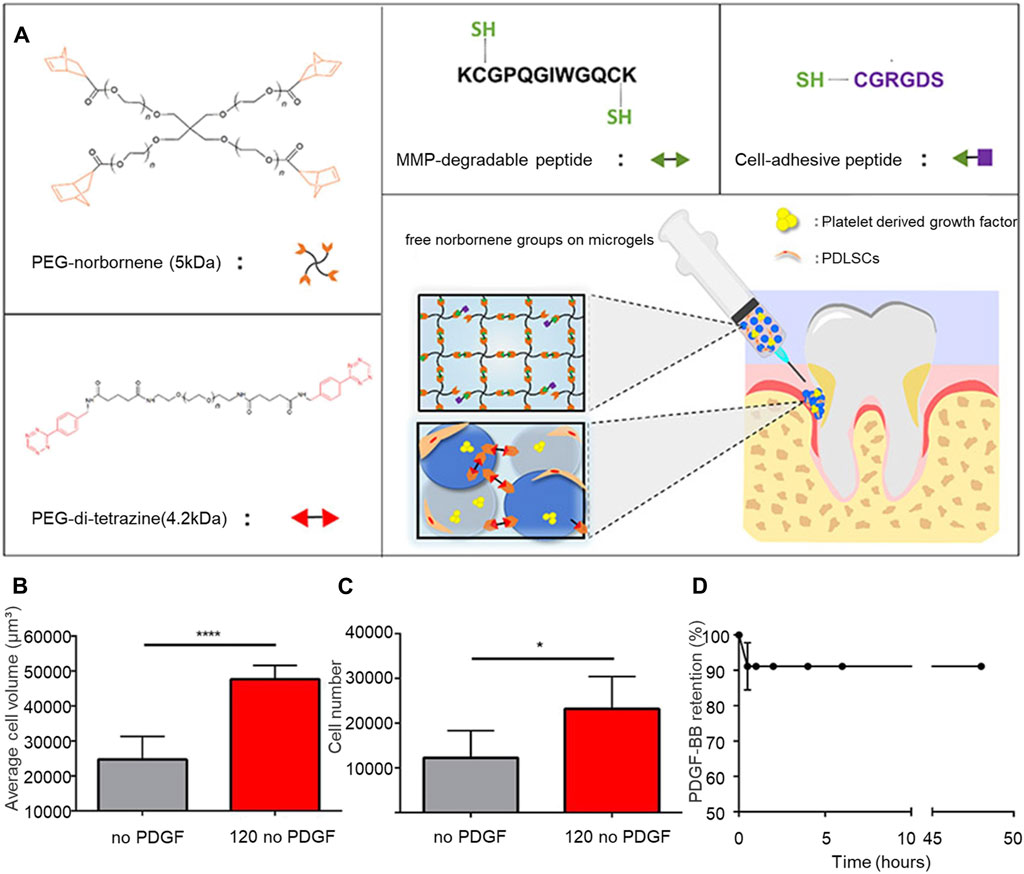
Figure 2. TzMAP hydrogel for periodontal regeneration. (A) Overview of hydrogel materials and use. (B) Average cell volume of PDLSCs at 5 days (C) Number of cells per 5 μL of hydrogel observed at 50 days. (D) Changes in the content of PDGF-BB in the TzMAP hydrogel over time. Reprinted with permission from Isaac et al. (2019). Copyright (2019) American Chemical Society.
Furthermore, functionally graded scaffolds have been developed for the regeneration of multiple tissues, acknowledging that periodontal regeneration involves complex tissues and structures. Sowmya et al. (2017) introduced a three-layer scaffold system for periodontal tissue regeneration that includes a layer for cementum regeneration using CS/poly (lactic-co-glycolic acid)/nanobioactive glass-ceramic/cementum protein 1, a CS/PLGA/fibroblast growth factor-2 layer for PDL regeneration, and a CS/PLGA/nano-sized bioactive glass/PRP layer for bone regeneration. This system achieved simultaneous regeneration of cementum, PDL, and alveolar bone.
Although various hydrogels exhibit promising effects in periodontal restoration and are prepared using advanced methods, commercial options remain limited. This scarcity stems from the complex manufacturing processes of composite hydrogels, which often require specialized formulations and equipment, leading to longer preparation times and significantly higher costs compared to simpler hydrogel products. Additionally, the impact of different structural and physicochemical characteristics on periodontal restoration is not well-understood, compounded by numerous confounding factors. These challenges considerably hinder the clinical application of hydrogels with complex structures.
3.2 Hydrogels as carriers for cell transplantation
Stem cells, known for their self-replication capability and versatility in developing into various functional cell types, present a significant potential in cell repair, regenerative medicine, and pharmacology (Li B. et al., 2021; Bartold and Ivanovski, 2022). This potential is largely due to their underdifferentiated state. Research has extensively examined stem cells’ ability to regenerate periodontal tissue, identifying two main categories: odontogenic and non-odontogenic stem cells. Odontogenic stem cells include dental pulp stem cells, stem cells from human exfoliated deciduous teeth, PDLSCs, apical papilla stem cells, and gingival mesenchymal stem cells (GMSCs). By contrast, non-odontogenic stem cells encompass BMSCs, adipose-derived stromal cells, embryonic stem cells, and induced pluripotent stem cells. A wealth of animal studies and clinical reports supports the effectiveness of stem cell therapy in addressing periodontal tissue defects (Vandana et al., 2017; Kandalam et al., 2021; Fonticoli et al., 2022; Iwasaki et al., 2022; Shaikh et al., 2022). For successful tissue regeneration, stem cells must efficiently reach and function within the lesion, making cell transplantation essential. However, the approach faces challenges such as low survival rates and difficulty in cell engraftment, underscoring the need for better cell delivery mechanisms. Carrier materials for periodontal regenerative stem cells should support cell differentiation and growth (Ding et al., 2021), with specific requirements for optimal performance: appropriate pore size and porosity for physiological functions, biological strength, biocompatibility, tissue adhesion, controlled degradation with non-toxic by-products, and sterilization compatibility. Hydrogels, known for enabling metabolite, oxygen, and nutrient diffusion (Li et al., 2018), have emerged as promising stem cell carriers. They can be tailored to specific needs by adjusting their composition, properties, and fabrication methods, offering a versatile platform for cell delivery.
One advantage of hydrogels is their ability to be directly injected into the lesion area through minimally invasive procedures. A research team, led by Kandalam et al. (2021), successfully encapsulated pre-differentiated GMSCs in a self-assembled hydrogel known as PuraMatrix™. The GMSCs, encapsulated with 0.5% PuraMatrix, exhibited remarkable adhesion and proliferation rates. Earlier discussions in this review highlighted that DN hydrogels possess superior mechanical properties compared to single hydrogels, enhancing their utility in cellular delivery. Choi et al. (2019) developed DN hydrogels by combining gellan gum, a natural polysaccharide, with poloxamer-heparin. These hydrogels, referred to as PoH/GG DNH, were used to culture rabbit BMSCs. The results indicated that PoH/GG DNH not only served as an effective cell culture medium but also significantly improved osteogenic differentiation and cell proliferation in both lab and animal studies. Ding et al. (2021) employed the Knoevenagel condensation process to create a novel hydrogel by gelling dextran, functionalized with benzaldehyde and cyanoacetate groups. This was followed by photocrosslinking 4-arm acrylated polyethylene glycol to form a secondary, enhanced network within the hydrogel. This composite hydrogel demonstrated exceptional injectability, self-healing properties, and the ability to protect cells from mechanical damage during injection. Additionally, the second network exhibited remarkable stability, acting as a scaffold post-transplantation, while the first network rapidly hydrolyzed, facilitating cell proliferation and diffusion.
The lack of a microporous structure in conventional hydrogels often hinders cell spreading and proliferation. While increasing the porosity of the hydrogel by reducing polymer concentration is a possible adjustment, it may compromise the hydrogel’s stability. A viable solution is the development of microporous hydrogels, which enhance both host and encapsulated cell motility. A previous study introduced gelatin and GelMA-based fast-curing microporous hydrogels (Edwards et al., 2022). These hydrogels, which set within 2.5 min of injection through photopolymerization and enzymatic cross-linking, allowed for uniform cell distribution and significant cell spreading and proliferation within a week. Additionally, these hydrogels could transport hMSCs primed with interferon-gamma, boosting the release of anti-inflammatory agents such as prostaglandin E2 and interleukin-6. Hence, these hydrogels present a promising cell delivery mechanism. Hydrogel particles (HMPs) offer another protective means for cell transplantation. Consisting of biopolymer-made, tiny 3D network structures, HMPs protect cells from shear stresses during transplantation, thus preserving cell viability (Blaeser et al., 2016; Chen et al., 2017; Mealy et al., 2018). For instance, a capillary microfluidic device was employed by Zhao et al. (2016) to produce GelMA HMPs encapsulating BMSCs. Over 4 weeks, these BMSC/GelMA cultures showed a significant increase in DNA content, indicating effective cell migration and viability. Further, Teng et al. (2022) demonstrated that GelMA hydrogel microspheres could act as carriers for rat BMSCs without compromising cell survival rate of almost 99%. Following implantation in a rat bone defect model, CT scans revealed significant bone regeneration at the defect site after 8 weeks.
Hydrogels can be precisely engineered for controlled cell distribution and tissue structure formation using self-assembly or 3D printing techniques. A bioprinting approach utilized injectable composite hydrogels composed of GelMA and polyethylene glycol dimethacrylate to encapsulate PDLSCs (Ma et al., 2017). The addition of polyethylene glycol enhanced droplet management. Hydrogels containing PDLSCs showed increased bone growth in rat alveolar bone defects compared to hydrogels without cells, as evidenced by in vivo experiments.
While hydrogels have shown promise as carriers for stem cell transplantation, challenges remain. Issues such as cell death during implantation, low cell retention post-transplantation, difficult long-term cell survival, and inadequate structural support significantly impact the effectiveness of periodontal regeneration therapy. Currently, there is no ideal hydrogel cell carrier that addresses these concerns.
3.3 Hydrogels as drug delivery systems
Over the last decade, studies have shown that various medications and growth factors can promote periodontal tissue regeneration (Liang et al., 2020), as detailed in Table 1. However, the limited application of these bioactive substances is often due to their short half-lives and rapid degradation rates. To address this issue, researchers have developed strategies to mitigate the drug’s adverse effects and extend its duration of action (Onaciu et al., 2019; Pushpamalar et al., 2021). One popular method involves using hydrogels for drug delivery. Due to their unique physical properties, hydrogels are ideal for this purpose, allowing for sustained drug release. Drugs can be encapsulated within hydrogels, integrated into their structures, or adsorbed on their surfaces. Various factors influence drug release from hydrogels, including the hydrogel’s composition, shape, manufacturing method, external conditions, and the interaction between the hydrogel and the bioactive substance (Zięba et al., 2020). By fine-tuning these factors, the rate and duration of drug release can be precisely controlled. The ideal hydrogel drug carrier should exhibit several biophysical and chemical characteristics. It must be biocompatible, not eliciting adverse reactions or immune rejection, and should degrade gradually in the body. The degradation byproducts should be non-toxic and non-inflammatory, with a degradation rate that matches the drug release, ensuring the drug’s efficacy for the required duration. Furthermore, the hydrogel must adhere securely to periodontal tissue to prevent displacement during treatment or daily activities.
In recent years, there has been growing interest in the development of stimulus-responsive or “smart” hydrogels (Huang et al., 2020). These hydrogels can change shape or phase in response to environmental stimuli, such as temperature changes, facilitating drug release through swelling-shrinking actions or gel-sol transitions. Thermosensitive hydrogels, for example, are in liquid form at low temperatures but gelate at higher temperatures, a property crucial for effective drug delivery. Wang et al. (2023) developed a berberine heat-sensitive hydrogel that gels rapidly at body temperature (37°C) within approximately 3 min, demonstrating efficacy in treating periodontal inflammatory bone conditions by modulating the PI3K/AKT signaling pathway and offering anti-inflammatory and osteogenic benefits. Xu et al. (2019) created an injectable thermosensitive hydrogel that continuously released aspirin and erythropoietin, facilitating the healing of inflammation and alveolar bone repair. Aspirin and erythropoietin released 86.6% and 69.4% of their doses, respectively, within the first 3 days of the drug release trial, with aspirin’s faster release rate aiding in the early stages of periodontitis treatment where controlling inflammation quickly is vital. The thermosensitive hydrogel developed by Liu et al. (2022) useed polyethylene glycol diacrylate as a basal scaffold and imparted excess ROS–scavenging effects to the hydrogel and oxidative stress–reducing effected to cells by adding the reducing sugar alcohol dithiothreitol, thereby protecting periodontal cells from inflammatory damage. The inclusion of SDF-1α increased the hydrogel’s biological activity, promoting cell proliferation and differentiation by activating the Wnt/β-catenin signaling pathway, essential for alveolar bone regeneration. They also introduced a new functional peptide module responsive to arginine protease secreted by Porphyromonas gingivalis, creating a smart gingival protease-reactive hydrogel (Liu et al., 2021). This hydrogel generated short antimicrobial peptides activated by arginine protease, inhibiting the growth of P. gingivalis. Under the action of different concentrations of gingival protease R1 protein, the release rate of SDF-1 in hydrogels increased with the increase of protease concentration, because protease would cut off the functional peptide module in the hydrogel and destroy the structure of the hydrogel, which was conducive to the antibacterial function of antimicrobial peptides and the function of SDF-1 to recruit host stem cells and promote osteogenesis, as illustrated in Figure 3.
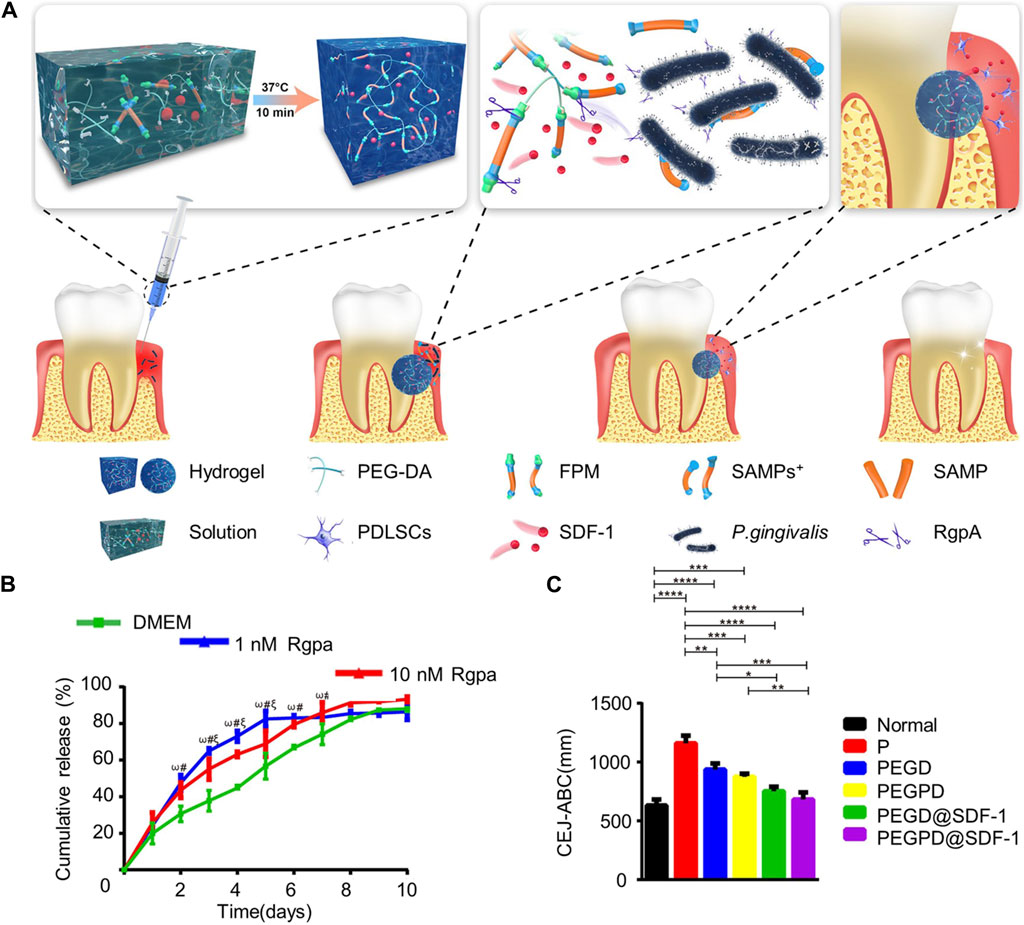
Figure 3. SDF-1-loaded gingival protease-reactive thermothermal hydrogel promotes periodontal regeneration. (A) Schematic representation of the hydrogelmaterial composition and processing. (B) Release curves of SDF-1 from the hydrogel. (C) Distance between CEJ and ABC in vivo model 4 weeks after treatment. Reprinted with permission from Liu et al. (2021). Copyright (2021) American Chemical Society.
Inflammation in periodontal tissues leads to an increase in ROS levels, prompting the development of ROS-reactive materials for periodontal disease treatment. The hydrogel system designed by Zhao et al. (2022) used the presence of ROS to initiate drug release. This system, made from oxidized dextran and phenylboronic acid-functionalized polyethylenimine, enhances the efficiency of doxycycline and metformin delivery through B-N ligands. It achieves localized drug release in response to ROS, boosting the combined therapeutic benefits of doxycycline’s antimicrobial action and metformin’s bone regeneration promotion. Additionally, the alteration of pH within the periodontal pocket during disease progression offers a chance to develop pH-sensitive hydrogels that respond to this change, aiding in periodontal tissue regeneration. pH-sensitive hydrogels, which are protonation polymers containing acidic or basic groups, change their volume in response to pH alterations, either releasing or absorbing protons. Yu et al. (2016) developed a pH-responsive hydrogel containing anti-glycation agents that slowed the progression of periodontitis and promoted recovery. Beyond environmentally sensitive hydrogels, there are also stimulus-responsive hydrogels and multisensitive hydrogels that offer combined advantages for drug delivery mechanisms, showing significant potential for future applications (Lin et al., 2020; Hsia et al., 2024).
In addition to stimuli-responsive hydrogels, the structure of hydrogels formed by a dual dynamic network can be tuned to manage the controlled release of drugs effectively (Bertsch et al., 2022). The innovative injectable and self-healing hydrogel developed by Guo et al. (2021) is aimed at facilitating periodontal regeneration. This hydrogel, synthesized from aldehyde-modified hyaluronic acid (HA-CHO) and ethylene glycol chitosan (GC), formed a dual dynamic network through the dynamic Schiff base bonds between the -CHO group in HA-CHO and the -NH2 group in GC, alongside dynamically oriented bonds between the COO- group and Fe3+ ions in HA-CHO. Owing to its unique structure, the hydrogel exhibited robust self-healing capabilities, enabling it to swiftly regain its mechanical properties even after being subjected to high strain (e.g., 500%) and return to its original state under low strain (e.g., 1%). Moreover, by adjusting the biodynamic bonds, the hydrogel’s drug release rate could be customized to meet specific therapeutic needs. In their study, the researchers demonstrated this capability by loading the hydrogel with ginsenoside Rg1, known for its anti-inflammatory properties, and enamel for its regeneration-promoting effects, under varying concentrations of FeCl3 to control the release duration. The results indicated the potential for precise control over drug release timing through the adjustment of biodynamic linkages in hydrogels. Significant periodontal regeneration in rats with periodontal defects showcased the hydrogel’s potential in promoting tissue regeneration, offering a novel solution for periodontitis management.
While in situ hydrogels offer a degree of local pharmaceutical storage, achieving sustained local delivery of hydrophilic small molecules remains a challenge. Microspheres, however, are recognized for their effective long-acting sustained-release property (Zhang et al., 2018). The introduction of microspheres into hydrogels introduces two physical barriers that the drug must overcome, enabling prolonged therapeutic effects. A research group designed a hydrogel system for the sequential release of basic fibroblast growth factor and transforming growth factor-beta 3 (Yu et al., 2022). In this system, transforming growth factor-beta 3 was encapsulated in CS microspheres before being co-loaded with basic fibroblast growth factor into CS hydrogels, facilitating a staggered release that aligns with different healing phases. Notably, the system demonstrated significant alveolar bone repair 12 weeks after being injected into an alveolar bone defect model. Wang B. et al. (2020) developed an innovative topical drug delivery system for periodontal applications by utilizing electrospraying to encapsulate two medications into PLGA microspheres within a thermoreversible polyisocyanate hydrogel. This method offers key advantages, including biosafety, injectability, and enhanced structural stability over time. By adjusting the load-to-mass ratio of acid-terminated PLGA, the release of the drug could be regulated. An adjustable and injectable topical drug delivery system was developed by incorporating two drugs into PLGA microspheres within a thermoreversible polyisocyanate hydrogel through electrospray. This technology offers biosafety, injectability, and long-term structural stability. Adjusting the load-to-mass ratio of acid-terminated PLGA allows for precise manipulation of drug release.
The precise spatial and temporal control of bioactive signals is essential for their anti-inflammatory and regenerative effects. Proper timing is critical in controlling inflammation, a necessary step for tissue regeneration, as is the timely administration of growth factors for the regeneration of various periodontal tissues. Despite the development of numerous hydrogel drug delivery systems, achieving exact control over the delivery of bioactive molecules for periodontal tissue regeneration remains a challenge.
4 Conclusion and perspectives
Hydrogels have significantly advanced periodontal regeneration therapy due to their excellent biocompatibility and structural resemblance to the ECM, allowing for their widespread use in this field. They serve various roles, including as GTR membranes, tissue engineering scaffolds and drug delivery systems, contributing to periodontal regeneration. However, despite promising experimental results, hydrogels have not yet achieved successful clinical outcomes in periodontal regeneration. The challenges in utilizing hydrogels for functional periodontal tissue regeneration are as follows:
Periodontal tissue regeneration is complex, involving multiple cell and tissue types with differing structures, compositions, and functions. This complexity makes it challenging for a single hydrogel to effectively mimic these characteristics. Current strategies often focus on regenerating a single tissue type, such as alveolar bone, without adequately addressing the regeneration of the PDL diverse fiber bundles. The natural regenerative capacity of periodontal tissue is limited, particularly in the presence of infection and inflammation. Enhancing regeneration often requires external interventions, such as cell transplantation or recruiting stem cells from surrounding tissues. For effective periodontitis treatment, the drug delivery system must precisely control the release timing and location of medications, transitioning from anti-inflammatory to tissue-regenerating agents. While hydrogels can provide controlled release in response to stimuli, they fall short in precisely regulating periodontal tissue regeneration. This limitation stems from an incomplete understanding of the regeneration and healing process and the limited efficacy of bioactive molecules in promoting regeneration. The mechanical strength of hydrogels is another limiting factor for their application in periodontal tissue engineering. Achieving a balance between mechanical strength and injectability is challenging, as enhancing mechanical properties often reduces injectability. Moreover, rapid degradation of hydrogels in vivo, influenced by factors such as the inflammatory microenvironment, differs from in vitro results and requires further research. Despite these obstacles, hydrogels remain a promising area of research for periodontal tissue regeneration. Ongoing development of hydrogel systems capable of repairing periodontal tissue offers hope for improved treatment outcomes and innovative solutions for periodontal patients in the future.
Author contributions
HS: Writing–original draft. JL: Writing–review and editing. SD: Writing–review and editing.
Funding
The author(s) declare that financial support was received for the research, authorship, and/or publication of this article. This study was supported by the Jilin Provincial Department of Finance Backbone Talent Project, China (Grant No. jcsz2023481-10).
Conflict of interest
The authors declare that the research was conducted in the absence of any commercial or financial relationships that could be construed as a potential conflict of interest.
Publisher’s note
All claims expressed in this article are solely those of the authors and do not necessarily represent those of their affiliated organizations, or those of the publisher, the editors and the reviewers. Any product that may be evaluated in this article, or claim that may be made by its manufacturer, is not guaranteed or endorsed by the publisher.
References
Abo-Zeid, Y., and Williams, G. R. (2020). The potential anti-infective applications of metal oxide nanoparticles: a systematic review. Wiley Interdiscip. Rev. Nanomed. Nanobiotechnol 12, e1592. doi:10.1002/wnan.1592
Alauddin, M. S., Abdul Hayei, N. A., Sabarudin, M. A., and Mat Baharin, N. H. (2022). Barrier membrane in regenerative therapy: a narrative review. Membranes 12, 444. doi:10.3390/membranes12050444
Alqahtani, A. M. (2023). Guided tissue and bone regeneration membranes: a review of biomaterials and techniques for periodontal treatments. Polymers 15, 3355. doi:10.3390/polym15163355
Ammar, M. M., Waly, G. H., Saniour, S. H., and Moussa, T. A. (2018). Growth factor release and enhanced encapsulated periodontal stem cells viability by freeze-dried platelet concentrate loaded thermo-sensitive hydrogel for periodontal regeneration. Saudi Dent. J. 30, 355–364. doi:10.1016/j.sdentj.2018.06.002
Bahrambeigi, S., Yousefi, B., Rahimi, M., and Shafiei-Irannejad, V. (2019). Metformin; an old antidiabetic drug with new potentials in bone disorders. Biomed. Pharmacother. 109, 1593–1601. doi:10.1016/j.biopha.2018.11.032
Bahrami, N., Bayat, M., Farzin, A., Hajseyedjavadi, M. S., Goodarzi, A., Salehi, M., et al. (2019). The ability of 3D alginate/polyvinyl alcohol cross-linked hybrid hydrogel to differentiate periodontal ligament stem cells into osteoblasts. Arch. Neurosci. 6, e85118. doi:10.5812/ans.85118
Bartold, M., and Ivanovski, S. (2022). Stem cell applications in periodontal regeneration. Dent. Clin. N. Am. 66, 53–74. doi:10.1016/j.cden.2021.06.002
Bee, S.-L., and Hamid, Z. A. A. (2022). Asymmetric resorbable-based dental barrier membrane for periodontal guided tissue regeneration and guided bone regeneration: a review. J. Biomed. Mater. Res. Part B Appl. Biomaterials 110, 2157–2182. doi:10.1002/jbm.b.35060
Bertsch, P., Diba, M., Mooney, D. J., and Leeuwenburgh, S. C. G. (2022). Self-healing injectable hydrogels for tissue regeneration. Chem. Rev. 123, 834–873. doi:10.1021/acs.chemrev.2c00179
Blaeser, A., Duarte Campos, D. F., Puster, U., Richtering, W., Stevens, M. M., and Fischer, H. (2016). Controlling shear stress in 3D bioprinting is a key factor to balance printing resolution and stem cell integrity. Adv. Healthc. Mat. 5, 326–333. doi:10.1002/adhm.201500677
Branco, A. C., Oliveira, A. S., Monteiro, I., Nolasco, P., Silva, D. C., Figueiredo-Pina, C. G., et al. (2022). PVA-based hydrogels loaded with diclofenac for cartilage replacement. Gels 8, 143. doi:10.3390/gels8030143
Catoira, M. C., Fusaro, L., Di Francesco, D., Ramella, M., and Boccafoschi, F. (2019). Overview of natural hydrogels for regenerative medicine applications. J. Mater Sci. Mater Med. 30, 115–210. doi:10.1007/s10856-019-6318-7
Chang, K.-C., Chen, W.-C., Chen, C.-H., Ko, C.-L., Liu, S.-M., and Chen, J.-C. (2021). Chemical cross-linking on gelatin-hyaluronan loaded with hinokitiol for the preparation of guided tissue regeneration hydrogel membranes with antibacterial and biocompatible properties. Mater. Sci. Eng. C 119, 111576. doi:10.1016/j.msec.2020.111576
Chang, K.-C., Lin, D.-J., Wu, Y.-R., Chang, C.-W., Chen, C.-H., Ko, C.-L., et al. (2019). Characterization of genipin-crosslinked gelatin/hyaluronic acid-based hydrogel membranes and loaded with hinokitiol: in vitro evaluation of antibacterial activity and biocompatibility. Mater. Sci. Eng. C 105, 110074. doi:10.1016/j.msec.2019.110074
Chen, M. H., Wang, L. L., Chung, J. J., Kim, Y.-H., Atluri, P., and Burdick, J. A. (2017). Methods to assess shear-thinning hydrogels for application as injectable biomaterials. ACS Biomater. Sci. Eng. 3, 3146–3160. doi:10.1021/acsbiomaterials.7b00734
Chen, N., Ren, R., Wei, X., Mukundan, R., Li, G., Xu, X., et al. (2021). Thermoresponsive hydrogel-based local delivery of simvastatin for the treatment of periodontitis. Mol. Pharm. 18, 1992–2003. doi:10.1021/acs.molpharmaceut.0c01196
Chen, X., Fan, H., Deng, X., Wu, L., Yi, T., Gu, L., et al. (2018). Scaffold structural microenvironmental cues to guide tissue regeneration in bone tissue applications. Nanomaterials 8, 960. doi:10.3390/nano8110960
Chichiricco, P. M., Riva, R., Thomassin, J.-M., Lesoeur, J., Struillou, X., Le Visage, C., et al. (2018). In situ photochemical crosslinking of hydrogel membrane for Guided Tissue Regeneration. Dent. Mater. 34, 1769–1782. doi:10.1016/j.dental.2018.09.017
Chien, K.-H., Chang, Y.-L., Wang, M.-L., Chuang, J.-H., Yang, Y.-C., Tai, M.-C., et al. (2018). Promoting induced pluripotent stem cell-driven biomineralization and periodontal regeneration in rats with maxillary-molar defects using injectable BMP-6 hydrogel. Sci. Rep. 8, 114. doi:10.1038/s41598-017-18415-6
Chiu, H.-C., Chiang, C.-Y., Tu, H.-P., Wikesjö, U. M. E., Susin, C., and Fu, E. (2013). Effects of bone morphogenetic protein-6 on periodontal wound healing/regeneration in supraalveolar periodontal defects in dogs. J. Clin. Periodontol. 40, 624–630. doi:10.1111/jcpe.12075
Choi, J. H., Choi, O. K., Lee, J., Noh, J., Lee, S., Park, A., et al. (2019). Evaluation of double network hydrogel of poloxamer-heparin/gellan gum for bone marrow stem cells delivery carrier. Colloids Surfaces B Biointerfaces 181, 879–889. doi:10.1016/j.colsurfb.2019.06.041
Daghrery, A., Ferreira, J. A., Xu, J., Golafshan, N., Kaigler, D., Bhaduri, S. B., et al. (2023). Tissue-specific melt electrowritten polymeric scaffolds for coordinated regeneration of soft and hard periodontal tissues. Bioact. Mater. 19, 268–281. doi:10.1016/j.bioactmat.2022.04.013
Deas, D. E., Moritz, A. J., Sagun, R. S., Gruwell, S. F., and Powell, C. A. (2016). Scaling and root planing vs. conservative surgery in the treatment of chronic periodontitis. Periodontol 71, 128–139. doi:10.1111/prd.12114
de Jong, T., Bakker, A. D., Everts, V., and Smit, T. H. (2017). The intricate anatomy of the periodontal ligament and its development: lessons for periodontal regeneration. J. Periodont. Res. 52, 965–974. doi:10.1111/jre.12477
Dimatteo, R., Darling, N. J., and Segura, T. (2018). In situ forming injectable hydrogels for drug delivery and wound repair. Adv. Drug Deliv. Rev. 127, 167–184. doi:10.1016/j.addr.2018.03.007
Ding, X., Wang, Y., Liu, J., Zhang, P., Li, G., Sun, T., et al. (2021). Injectable in situ forming double-network hydrogel to enhance transplanted cell viability and retention. Chem. Mat. 33, 5885–5895. doi:10.1021/acs.chemmater.1c00635
Dubey, N., Ferreira, J. A., Daghrery, A., Aytac, Z., Malda, J., Bhaduri, S. B., et al. (2020). Highly tunable bioactive fiber-reinforced hydrogel for guided bone regeneration. Acta Biomater. 113, 164–176. doi:10.1016/j.actbio.2020.06.011
Edwards, S. D., Hou, S., Brown, J. M., Boudreau, R. D., Lee, Y., Kim, Y. J., et al. (2022). Fast-curing injectable microporous hydrogel for in situ cell encapsulation. ACS Appl. Bio. Mat. 5, 2786–2794. doi:10.1021/acsabm.2c00214
Elizalde-Cárdenas, A., Ribas-Aparicio, R. M., Rodríguez-Martínez, A., Leyva-Gómez, G., Ríos-Castañeda, C., and González-Torres, M. (2024). Advances in chitosan and chitosan derivatives for biomedical applications in tissue engineering: an updated review. Int. J. Biol. Macromol. 262, 129999. doi:10.1016/j.ijbiomac.2024.129999
El-Sherbiny, I. M., and Yacoub, M. H. (2013). Hydrogel scaffolds for tissue engineering: progress and challenges. Glob. Cardiol. Sci. Pract. 2013, 38. doi:10.5339/gcsp.2013.38
Fonticoli, L., Della Rocca, Y., Rajan, T. S., Murmura, G., Trubiani, O., Oliva, S., et al. (2022). A narrative review: gingival stem cells as a limitless reservoir for regenerative medicine. Int. J. Mol. Sci. 23, 4135. doi:10.3390/ijms23084135
Galli, M., Yao, Y., Giannobile, W. V., and Wang, H.-L. (2021). Current and future trends in periodontal tissue engineering and bone regeneration. Plastic Aesthetic Res. 8, 3. doi:10.20517/2347-9264.2020.176
Gao, P., Kajiya, M., Motoike, S., Ikeya, M., and Yang, J. (2024). Application of mesenchymal stem/stromal cells in periodontal regeneration: opportunities and challenges. Jpn. Dent. Sci. Rev. 60, 95–108. doi:10.1016/j.jdsr.2024.01.001
Gao, Y., Wang, S., Shi, B., Wang, Y., Chen, Y., Wang, X., et al. (2022). Advances in modification methods based on biodegradable membranes in guided bone/tissue regeneration: a review. Polymers 14, 871. doi:10.3390/polym14050871
Giannobile, W. V., and McClain, P. K. (2015). Enhancing periodontal health through regenerative approaches. J. Periodont. 86, S1–S3. doi:10.1902/jop.2015.140525
Gorriz, C., Ribeiro, F., Guedes, J. M., and Fernandes, P. R. (2015). A biomechanical approach for bone regeneration inside scaffolds. Procedia Eng. 110, 82–89. doi:10.1016/j.proeng.2015.07.013
Gottlow, J., Karring, T., and Nyman, S. (1990). Guided tissue regeneration following treatment of recession-type defects in the monkey. J. Periodontology 61, 680–685. doi:10.1902/jop.1990.61.11.680
Guo, H., Huang, S., Yang, X., Wu, J., Kirk, T. B., Xu, J., et al. (2021). Injectable and self-healing hydrogels with double-dynamic bond tunable mechanical, gel–sol transition and drug delivery properties for promoting periodontium regeneration in periodontitis. ACS Appl. Mat. Interfaces 13, 61638–61652. doi:10.1021/acsami.1c18701
Han, J., Menicanin, D., Gronthos, S., and Bartold, P. (2014). Stem cells, tissue engineering and periodontal regeneration. Aust. Dent. J. 59, 117–130. doi:10.1111/adj.12100
Hanyková, L., Krakovský, I., Šťastná, J., Ivaniuzhenkov, V., and Labuta, J. (2023). Thermal response of double network hydrogels with varied composition. e-Polymers 23. doi:10.1515/epoly-2023-0044
He, X.-T., Li, X., Xia, Y., Yin, Y., Wu, R.-X., Sun, H.-H., et al. (2019). Building capacity for macrophage modulation and stem cell recruitment in high-stiffness hydrogels for complex periodontal regeneration: experimental studies in vitro and in rats. Acta Biomater. 88, 162–180. doi:10.1016/j.actbio.2019.02.004
He, Z., Zhou, X., Wang, Y., Lin, J., Huang, S., Hu, R., et al. (2021). Asymmetric barrier membranes based on polysaccharide micro-nanocomposite hydrogel: synthesis, characterization, and their antibacterial and osteogenic activities. Carbohydr. Polym. 273, 118525. doi:10.1016/j.carbpol.2021.118525
Hernandez, I., Kumar, A., and Joddar, B. (2017). A bioactive hydrogel and 3D printed polycaprolactone system for bone tissue engineering. Gels 3, 26. doi:10.3390/gels3030026
Hsia, T., Lin, Z., Xia, Y., Shu, R., and Xie, Y. (2024). A photoresponsive recombinant human amelogenin-loaded hyaluronic acid hydrogel promotes bone regeneration. J Periodontal Res., jre13235. doi:10.1111/jre.13235
Hu, L., Liu, Y., and Wang, S. (2018). Stem cell-based tooth and periodontal regeneration. Oral Dis. 24, 696–705. doi:10.1111/odi.12703
Huang, C.-L., Huang, H.-Y., Lu, Y.-C., Cheng, C.-J., and Lee, T.-M. (2023). Development of a flexible film made of polyvinyl alcohol with chitosan based thermosensitive hydrogel. J. Dent. Sci. 18, 822–832. doi:10.1016/j.jds.2023.01.007
Huang, J., Wang, Z., Krishna, S., Hu, Q., Xuan, M., and Xie, H. (2020). Environment-sensitive hydrogels as potential drug delivery systems for the treatment of periodontitis. Mat. express 10, 975–985. doi:10.1166/mex.2020.1705
Humber, C. C., Sándor, G. K. B., Davis, J. M., Peel, S. A. F., Brkovic, B. M. B., Kim, Y. D., et al. (2010). Bone healing with an in situ–formed bioresorbable polyethylene glycol hydrogel membrane in rabbit calvarial defects. Oral Surg. Oral Med. Oral Pathology, Oral Radiology, Endodontology 109, 372–384. doi:10.1016/j.tripleo.2009.10.008
Isaac, A., Jivan, F., Xin, S., Hardin, J., Luan, X., Pandya, M., et al. (2019). Microporous bio-orthogonally annealed particle hydrogels for tissue engineering and regenerative medicine. ACS Biomater. Sci. Eng. 5, 6395–6404. doi:10.1021/acsbiomaterials.9b01205
Iwasaki, K., Peng, Y., Kanda, R., Umeda, M., and Ishikawa, I. (2022). Stem cell transplantation and cell-free treatment for periodontal regeneration. Int. J. Mol. Sci. 23, 1011. doi:10.3390/ijms23031011
Jacob, S., Nair, A. B., Shah, J., Sreeharsha, N., Gupta, S., and Shinu, P. (2021). Emerging role of hydrogels in drug delivery systems, tissue engineering and wound management. Pharmaceutics 13, 357. doi:10.3390/pharmaceutics13030357
Kale, Dr. P., Mani, Dr. A., and Agrawal, Dr. A. (2022). Growth factors: key biological mediators involved in periodontal regeneration. Int. J. Res. Rev. 9, 21–23. doi:10.52403/ijrr.20221103
Kandalam, U., Kawai, T., Ravindran, G., Brockman, R., Romero, J., Munro, M., et al. (2021). Predifferentiated gingival stem cell-induced bone regeneration in rat alveolar bone defect model. Tissue Eng. Part A 27, 424–436. doi:10.1089/ten.tea.2020.0052
Kannan, K., Radhika, D., Sadasivuni, K. K., Reddy, K. R., and Raghu, A. V. (2020). Nanostructured metal oxides and its hybrids for photocatalytic and biomedical applications. Adv. Colloid Interface Sci. 281, 102178. doi:10.1016/j.cis.2020.102178
Kato, A., Miyaji, H., Ishizuka, R., Tokunaga, K., Inoue, K., Kosen, Y., et al. (2015). Combination of root surface modification with BMP-2 and collagen hydrogel scaffold implantation for periodontal healing in beagle dogs. Open Dent. J. 9, 52–59. doi:10.2174/1874210601509010052
Khorshidi, S., and Karkhaneh, A. (2018). Hydrogel/fiber conductive scaffold for bone tissue engineering. J. Biomed. Mat. Res. Part A 106, 718–724. doi:10.1002/jbm.a.36282
Kishen, A., Cecil, A., and Chitra, S. (2023). Fabrication of hydroxyapatite reinforced polymeric hydrogel membrane for regeneration. Saudi Dent. J. 35, 678–683. doi:10.1016/j.sdentj.2023.05.021
Kononen, E., Gursoy, M., and Gursoy, U. K. (2019). Periodontitis: a multifaceted disease of tooth-supporting tissues. J. Clin. Med. 8, 1135. doi:10.3390/jcm8081135
Kwon, T., Lamster, I. B., and Levin, L. (2021). Current concepts in the management of periodontitis. Int. Dent. J. 71, 462–476. doi:10.1111/idj.12630
Li, B., Ouchi, T., Cao, Y., Zhao, Z., and Men, Y. (2021a). Dental-derived mesenchymal stem cells: state of the art. Front. Cell Dev. Biol. 9, 654559. doi:10.3389/fcell.2021.654559
Li, J., Yin, X., and Luan, Q. (2018). Comparative study of periodontal differentiation propensity of induced pluripotent stem cells from different tissue origins. J. Periodontol. 89, 1230–1240. doi:10.1002/JPER.18-0033
Li, Q., He, W., Li, W., Luo, S., Zhou, M., Wu, D., et al. (2023). Band-aid-like self-fixed barrier membranes enable superior bone augmentation. Adv. Sci. 10, e2206981. doi:10.1002/advs.202206981
Li, S., Wang, X., Zhu, J., Wang, Z., and Wang, L. (2021b). Preparation and characterization of double network hydrogel with high-strength and self-healing. Mat. Today Commun. 27, 102450. doi:10.1016/j.mtcomm.2021.102450
Liang, Y., Luan, X., and Liu, X. (2020). Recent advances in periodontal regeneration: a biomaterial perspective. Bioact. Mater. 5, 297–308. doi:10.1016/j.bioactmat.2020.02.012
Lin, J., He, Z., Liu, F., Feng, J., Huang, C., Sun, X., et al. (2020). Hybrid hydrogels for synergistic periodontal antibacterial treatment with sustained drug release and NIR-responsive photothermal effect. IJN 15, 5377–5387. doi:10.2147/IJN.S248538
Liu, A.-Q., Hu, C.-H., Jin, F., Zhang, L.-S., and Xuan, K. (2018). Contributions of bioactive molecules in stem cell-based periodontal regeneration. Int. J. Mol. Sci. 19, 1016. doi:10.3390/ijms19041016
Liu, S., Wang, Y., Ma, B., Shao, J., Liu, H., and Ge, S. (2021). Gingipain-responsive thermosensitive hydrogel loaded with SDF-1 facilitates in situ periodontal tissue regeneration. ACS Appl. Mat. Interfaces 13, 36880–36893. doi:10.1021/acsami.1c08855
Liu, S., Wang, Y., Yu, L., Li, J., and Ge, S. (2022). Development of a thermosensitive hydrogel loaded with DTT and SDF-1 facilitating in situ periodontal bone regeneration. Chem. Eng. J. 432, 134308. doi:10.1016/j.cej.2021.134308
Lu, J., Cheng, C., He, Y., Lyu, C., Wang, Y., Yu, J., et al. (2016). Multilayered graphene hydrogel membranes for guided bone regeneration. Adv. Mat. 28, 4025–4031. doi:10.1002/adma.201505375
Ma, Y., Ji, Y., Zhong, T., Wan, W., Yang, Q., Li, A., et al. (2017). Bioprinting-based PDLSC-ECM screening for in vivo repair of alveolar bone defect using cell-laden, injectable and photocrosslinkable hydrogels. ACS Biomater. Sci. Eng. 3, 3534–3545. doi:10.1021/acsbiomaterials.7b00601
Malczak, I., and Gajda, A. (2023). Interactions of naturally occurring compounds with antimicrobials. J. Pharm. Analysis 13, 1452–1470. doi:10.1016/j.jpha.2023.09.014
Mantha, S., Pillai, S., Khayambashi, P., Upadhyay, A., Zhang, Y., Tao, O., et al. (2019). Smart hydrogels in tissue engineering and regenerative medicine. Materials 12, 3323. doi:10.3390/ma12203323
Mealy, J. E., Chung, J. J., Jeong, H.-H., Issadore, D., Lee, D., Atluri, P., et al. (2018). Injectable granular hydrogels with multifunctional properties for biomedical applications. Adv. Mater. 30, 1705912. doi:10.1002/adma.201705912
Mirzaeei, S., Ezzati, A., Mehrandish, S., Asare-Addo, K., and Nokhodchi, A. (2022). An overview of guided tissue regeneration (GTR) systems designed and developed as drug carriers for management of periodontitis. J. Drug Deliv. Sci. Technol. 71, 103341. doi:10.1016/j.jddst.2022.103341
Mizraji, G., Davidzohn, A., Gursoy, M., Gursoy, U. K., Shapira, L., and Wilensky, A. (2023). Membrane barriers for guided bone regeneration: an overview of available biomaterials. Periodontol. 2000 93, 56–76. doi:10.1111/prd.12502
Momose, T., Miyaji, H., Kato, A., Ogawa, K., Yoshida, T., Nishida, E., et al. (2016). Collagen hydrogel scaffold and fibroblast growth factor-2 accelerate periodontal healing of class II furcation defects in dog. Open Dent. J. 10, 347–359. doi:10.2174/1874210601610010347
Naahidi, S., Jafari, M., Logan, M., Wang, Y., Yuan, Y., Bae, H., et al. (2017). Biocompatibility of hydrogel-based scaffolds for tissue engineering applications. Biotechnol. Adv. 35, 530–544. doi:10.1016/j.biotechadv.2017.05.006
Ning, X., Huang, J., A, Y., Yuan, N., Chen, C., and Lin, D. (2022). Research advances in mechanical properties and applications of dual network hydrogels. Int. J. Mol. Sci. 23, 15757. doi:10.3390/ijms232415757
Onaciu, M., Munteanu, R. A., Moldovan, A. L., Moldovan, C. S., and Berindan-Neagoe, L. (2019). Hydrogels based drug delivery synthesis, characterization and administration. Pharmaceutics 11, 432. doi:10.3390/pharmaceutics11090432
Ostrovidov, S., Ramalingam, M., Bae, H., Orive, G., Fujie, T., Shi, X., et al. (2023). Bioprinting and biomaterials for dental alveolar tissue regeneration. Front. Bioeng. Biotechnol. 11, 991821. doi:10.3389/fbioe.2023.991821
Pan, J., Deng, J., Luo, Y., Yu, L., Zhang, W., Han, X., et al. (2019). Thermosensitive hydrogel delivery of human periodontal stem cells overexpressing platelet-derived growth factor-BB enhances alveolar bone defect repair. Stem Cells Dev. 28, 1620–1631. doi:10.1089/scd.2019.0184
Pan, Y., Zhao, Y., Kuang, R., Liu, H., Sun, D., Mao, T., et al. (2020). Injectable hydrogel-loaded nano-hydroxyapatite that improves bone regeneration and alveolar ridge promotion. Mater. Sci. Eng. C 116, 111158. doi:10.1016/j.msec.2020.111158
Patel, M., and Koh, W.-G. (2020). Composite hydrogel of methacrylated hyaluronic acid and fragmented polycaprolactone nanofiber for osteogenic differentiation of adipose-derived stem cells. Pharmaceutics 12, 902. doi:10.3390/pharmaceutics12090902
Pushpamalar, J., Meganathan, P., Tan, H. L., Dahlan, N. A., Ooi, L.-T., Neerooa, B. N. H. M., et al. (2021). Development of a polysaccharide-based hydrogel drug delivery system (DDS): an update. Gels 7, 153. doi:10.3390/gels7040153
Radulescu, D.-M., Neacsu, I. A., Grumezescu, A.-M., and Andronescu, E. (2022). New insights of scaffolds based on hydrogels in tissue engineering. Polymers (Basel) 14, 799. doi:10.3390/polym14040799
Sam, G., and Pillai, B. R. M. (2014). Evolution of barrier membranes in periodontal Regeneration-“Are the third generation membranes really here?”. J. Clin. Diagn Res. 8, ZE14–7. doi:10.7860/JCDR/2014/9957.5272
Sánchez-Cid, P., Jiménez-Rosado, M., Romero, A., and Pérez-Puyana, V. (2022). Novel trends in hydrogel development for biomedical applications: a review. Polymers 14, 3023. doi:10.3390/polym14153023
Sasaki, J.-I., Abe, G. L., Li, A., Thongthai, P., Tsuboi, R., Kohno, T., et al. (2021). Barrier membranes for tissue regeneration in dentistry. Biomater. Investig. Dent. 8, 54–63. doi:10.1080/26415275.2021.1925556
Sattar, T. (2019). Current review on synthesis, composites and multifunctional properties of graphene. Top. Curr. Chem. 377, 10. doi:10.1007/s41061-019-0235-6
Shaikh, M. S., Shahzad, Z., Tash, E. A., Janjua, O. S., Khan, M. I., and Zafar, M. S. (2022). Human umbilical cord mesenchymal stem cells: current literature and role in periodontal regeneration. Cells 11, 1168. doi:10.3390/cells11071168
Slots, J. (2017). Periodontitis: facts, fallacies and the future. Periodontol 75, 7–23. doi:10.1111/prd.12221
Sowmya, S., Mony, U., Jayachandran, P., Reshma, S., Kumar, R. A., Arzate, H., et al. (2017). Tri-layered nanocomposite hydrogel scaffold for the concurrent regeneration of cementum, periodontal ligament, and alveolar bone. Adv. Healthc. Mater 6. doi:10.1002/adhm.201601251
Tan, J., Zhang, M., Hai, Z., Wu, C., Lin, J., Kuang, W., et al. (2019). Sustained release of two bioactive factors from supramolecular hydrogel promotes periodontal bone regeneration. ACS Nano 13, 5616–5622. doi:10.1021/acsnano.9b00788
Tavakoli, S., and Klar, A. S. (2020). Advanced hydrogels as wound dressings. Biomolecules 10, 1169. doi:10.3390/biom10081169
Teng, C., Tong, Z., He, Q., Zhu, H., Wang, L., Zhang, X., et al. (2022). Mesenchymal stem cells–hydrogel microspheres system for bone regeneration in calvarial defects. Gels 8, 275. doi:10.3390/gels8050275
Toledano-Osorio, M., Vallecillo, C., Vallecillo-Rivas, M., Manzano-Moreno, F.-J., and Osorio, R. (2022). Antibiotic-loaded polymeric barrier membranes for guided bone/tissue regeneration: a mini-review. Polymers 14, 840. doi:10.3390/polym14040840
Vandana, K. L., Ryana, H., and Dalvi, P. J. (2017). Autologous periodontal stem cell assistance in periodontal regeneration technique (SAI-PRT) in the treatment of periodontal intrabony defects: a case report with one-year follow-up. J. Dent. Res. Dent. Clin. Dent. Prospects 11, 123–126. doi:10.15171/joddd.2017.022
Vasconcelos, M. O., Silva, L. A. D., Sousa-Junior, A. A., Dos Santos, T. R. M., Da Silva, C. A., Valadares, M. C., et al. (2022). Lidocaine- and chloramphenicol-loaded nanoparticles embedded in a chitosan/hyaluronic acid/glycerol matrix: drug-eluting biomembranes with potential for guided tissue regeneration. Front. Nanotechnol. 4, 1049599. doi:10.3389/fnano.2022.1049599
Villar, C. C., and Cochran, D. L. (2010). Regeneration of periodontal tissues: guided tissue regeneration. Dent. Clin. North Am. 54, 73–92. doi:10.1016/j.cden.2009.08.011
Wadia, R. (2021). Treatment guidelines for stage I-III periodontitis. Br. Dent. J. 230, 362. doi:10.1038/s41415-021-2846-x
Wang, B., Wang, J., Shao, J., Kouwer, P. H. J., Bronkhorst, E. M., Jansen, J. A., et al. (2020a). A tunable and injectable local drug delivery system for personalized periodontal application. J. Control. Release 324, 134–145. doi:10.1016/j.jconrel.2020.05.004
Wang, C., Liu, C., Liang, C., Qu, X., Zou, X., Du, S., et al. (2023). Role of berberine thermosensitive hydrogel in periodontitis via PI3K/AKT pathway in vitro. Int. J. Mol. Sci. 24, 6364. doi:10.3390/ijms24076364
Wang, S., Shao, C., Zhao, X., Guo, Y., Song, H., Shen, L., et al. (2024). Application of three-dimension printing nano-carbonated-hydroxylapatite to the repair of defects in rabbit bone. IJN 19, 1667–1681. doi:10.2147/IJN.S439775
Wang, Y., Cao, X., Ma, M., Lu, W., Zhang, B., and Guo, Y. (2020b). A GelMA-PEGDA-nHA composite hydrogel for bone tissue engineering. Materials 13, 3735. doi:10.3390/ma13173735
Wen, Y. F., Chen, M. X., Yin, G., Lin, R., Zhong, Y. J., Dong, Q. Q., et al. (2021). The global, regional, and national burden of cancer among adolescents and young adults in 204 countries and territories, 1990–2019: a population-based study. J. Hematol. Oncol. 14, 89. doi:10.1186/s13045-021-01093-3
Xing, X., Su, J., Liu, Y., Lin, H., Wang, Y., and Cheng, H. (2022). A novel visible light-curing chitosan-based hydrogel membrane for guided tissue regeneration. Colloids Surfaces B Biointerfaces 218, 112760. doi:10.1016/j.colsurfb.2022.112760
Xu, X., Gu, Z., Chen, X., Shi, C., Liu, C., Liu, M., et al. (2019). An injectable and thermosensitive hydrogel: promoting periodontal regeneration by controlled-release of aspirin and erythropoietin. Acta Biomater. 86, 235–246. doi:10.1016/j.actbio.2019.01.001
Xu, Y., Zhao, S., Weng, Z., Zhang, W., Wan, X., Cui, T., et al. (2020). Jelly-inspired injectable guided tissue regeneration strategy with shape auto-matched and dual-light-defined antibacterial/osteogenic pattern switch properties. ACS Appl. Mat. Interfaces 12, 54497–54506. doi:10.1021/acsami.0c18070
Yamada, S., Shanbhag, S., and Mustafa, K. (2022). Scaffolds in periodontal regenerative treatment. Dent. Clin. N. Am. 66, 111–130. doi:10.1016/j.cden.2021.06.004
Yang, X., Yang, H., Jiang, X., Yang, B., Zhu, K., Lai, N. C.-H., et al. (2021). Injectable chitin hydrogels with self-healing property and biodegradability as stem cell carriers. Carbohydr. Polym. 256, 117574. doi:10.1016/j.carbpol.2020.117574
Yu, F., Geng, D., Kuang, Z., Huang, S., Cheng, Y., Chen, Y., et al. (2022). Sequentially releasing self-healing hydrogel fabricated with TGFβ3-microspheres and bFGF to facilitate rat alveolar bone defect repair. Asian J. Pharm. Sci. 17, 425–434. doi:10.1016/j.ajps.2022.03.003
Yu, M.-C., Chang, C.-Y., Chao, Y.-C., Jheng, Y.-H., Yang, C., Lee, N., et al. (2016). pH-responsive hydrogel with an anti-glycation agent for modulating experimental periodontitis. J. Periodontology 87, 742–748. doi:10.1902/jop.2016.150542
Zang, S., Mu, R., Chen, F., Wei, X., Zhu, L., Han, B., et al. (2019). Injectable chitosan/β-glycerophosphate hydrogels with sustained release of BMP-7 and ornidazole in periodontal wound healing of class III furcation defects. Mater. Sci. Eng. C 99, 919–928. doi:10.1016/j.msec.2019.02.024
Zare, I., Mirshafiei, M., Kheilnezhad, B., Far, B. F., Hassanpour, M., Pishbin, E., et al. (2024). Hydrogel-integrated graphene superstructures for tissue engineering: from periodontal to neural regeneration. Carbon 223, 118970. doi:10.1016/j.carbon.2024.118970
Zhang, H., Cheng, J., and Ao, Q. (2021). Preparation of alginate-based biomaterials and their applications in biomedicine. Mar. Drugs 19, 264. doi:10.3390/md19050264
Zhang, W., Xu, W., Ning, C., Li, M., Zhao, G., Jiang, W., et al. (2018). Long-acting hydrogel/microsphere composite sequentially releases dexmedetomidine and bupivacaine for prolonged synergistic analgesia. Biomaterials 181, 378–391. doi:10.1016/j.biomaterials.2018.07.051
Zhang, Y., Ding, N., Zhang, T., Sun, Q., Han, B., and Yu, T. (2019). A tetra-PEG hydrogel based aspirin sustained release system exerts beneficial effects on periodontal ligament stem cells mediated bone regeneration. Front. Chem. 7, 682. doi:10.3389/fchem.2019.00682
Zhang, Y., Dou, X., Zhang, L., Wang, H., Zhang, T., Bai, R., et al. (2022). Facile fabrication of a biocompatible composite gel with sustained release of aspirin for bone regeneration. Bioact. Mater. 11, 130–139. doi:10.1016/j.bioactmat.2021.09.033
Zhao, X., Liu, S., Yildirimer, L., Zhao, H., Ding, R., Wang, H., et al. (2016). Injectable stem cell-laden photocrosslinkable microspheres fabricated using microfl uidics for rapid generation of osteogenic tissue constructs. Adv. Funct. Mat. 26, 2809–2819. doi:10.1002/adfm.201504943
Zhao, X., Yang, Y., Yu, J., Ding, R., Pei, D., Zhang, Y., et al. (2022). Injectable hydrogels with high drug loading through B–N coordination and ROS-triggered drug release for efficient treatment of chronic periodontitis in diabetic rats. Biomaterials 282, 121387. doi:10.1016/j.biomaterials.2022.121387
Zhou, T., Zheng, K., Sui, B., Boccaccini, A. R., and Sun, J. (2020). In vitro evaluation of poly (vinyl alcohol)/collagen blended hydrogels for regulating human periodontal ligament fibroblasts and gingival fibroblasts. Int. J. Biol. Macromol. 163, 1938–1946. doi:10.1016/j.ijbiomac.2020.09.033
Zhu, Y., Zhao, L., and Ngai, T. (2023). Multiphasic membranes/scaffolds for periodontal guided tissue regeneration. Macro Mater. Eng 308, 2300081. doi:10.1002/mame.202300081
Keywords: hydrogel, periodontitis, periodontal regeneration, guide tissue regeneration, cell transplantation, scaffolds, drug delivery systems, tissue engineering
Citation: Sun H, Luan J and Dong S (2024) Hydrogels promote periodontal regeneration. Front. Bioeng. Biotechnol. 12:1411494. doi: 10.3389/fbioe.2024.1411494
Received: 03 April 2024; Accepted: 06 May 2024;
Published: 17 May 2024.
Edited by:
Wenliang Li, Jilin Medical University, ChinaReviewed by:
Qihang Ding, Korea University, Republic of KoreaLesan Yan, Wuhan University of Technology, China
Guihua Cui, Jilin Medical University, China
向阳 李, Songshan Lake Material Laboratory, China
Junchao Wei, Nanchang University, China
Copyright © 2024 Sun, Luan and Dong. This is an open-access article distributed under the terms of the Creative Commons Attribution License (CC BY). The use, distribution or reproduction in other forums is permitted, provided the original author(s) and the copyright owner(s) are credited and that the original publication in this journal is cited, in accordance with accepted academic practice. No use, distribution or reproduction is permitted which does not comply with these terms.
*Correspondence: Shujun Dong, ZHNqQGpsdS5lZHUuY24=