- 1School of Civil Engineering, Xi’an University of Architecture and Technology, Xi’an, China
- 2Shaanxi Jianke Construction Special Engineering Co., Ltd., Xi’an, China
- 3Shaanxi Key Laboratory of Geotechnical and Underground Space Engineering (XAUAT), Xi’an, China
- 4UniSA STEM, ScaRCE, University of South Australia, Adelaide, SA, Australia
Intensive agricultural activities could cause lead (Pb) bioaccumulation, threatening human health. Although the enzyme-induced carbonate precipitation (EICP) technology has been applied to tackle the aforesaid problem, the urease may denature or even lose its activity when subjected to a significant Pb2+ toxicity effect. To this end, the nano-hydroxyapatite (nHAP)-assisted EICP was proposed to reduce the mobility of Pb2+. Results indicated that a below 30% immobilization efficiency at 60 mM Pb2+ was attained under EICP. nHAP adsorbed the majority of Pb2+, preventing Pb2+ attachment to urease. Further, hydroxylphosphohedyphane or hydroxylpyromorphite was formed at 60 mM Pb2+, followed by the formation of cerussite, allowing hydroxylphosphohedyphane or hydroxylpyromorphite to be wrapped by cerussite. By contrast, carbonate-bearing hydroxylpyromorphite of higher stability (Pb10(PO4)6CO3) was developed at 20 mM Pb2+ as CO32− substituted the hydroxyl group in hydroxylpyromorphite. Moreover, nHAP helped EICP to form nucleated minerals. As a result, the EICP-nHAP technology raised the immobilization efficiency at 60 mM Pb2+ up to 70%. The findings highlight the potential of applying the EICP-nHAP technology to Pb-containing water bodies remediation.
1 Introduction
Although intensive agricultural activities lead to a good crop, heavy metal could build up in the body during a chronic exposure (Karaouzas et al., 2020; Chen et al., 2024a; Chen et al., 2024b). Lead (Pb) poisoning, also known as plumbism and saturnism, is identified as blood lead level surpassing the upper limit of 10 μg/100 g set by the United States Centers for Disease Control and Prevention. It has symptoms predominantly in the central nervous system. The symptoms like loss of appetite, memory loss, and kidney failure develop over weeks to months, but acute symptoms from intensive exposures also occur (Bai et al., 2021; Xu et al., 2023; Liu et al., 2024; Xue et al., 2024a; Wang et al., 2024a). In severe instances, seizures, coma, or death may take place (Costa de Almeida et al., 2007; Chen et al., 2021). To tackle the said issue, many remediation technologies applied for Pb immobilization have been developed (Bai et al., 2022; Xie et al., 2022; Xue et al., 2022; Xue et al., 2023). However, they are often criticized due to poor performance and are accompanied by a high risk of secondary contamination (Yang et al., 2020; Meng et al., 2022; Hu et al., 2023a; Hu et al., 2023b; Wang et al., 2023; Bai et al., 2024; Xue et al., 2024b).
Enzyme-induced carbonate precipitation (EICP) has recently drawn particular attention as it featured high manoeuvrability and low risk of secondary pollution. Urease catalyzes the hydrolysis of urea resulting in the production of carbonate ions and the rise of surrounding pH. Then precipitation of CaCO3 in between soil particles enhances shear strength and erosion resistance of soil. The urease of a nanometer in size allows for catalyzing urea hydrolysis in deep grounds (Ashkan et al., 2019; Gowthaman et al., 2021; Gowthaman et al., 2023). Previous studies concentrate on problems of low pollution concentration (<5 mM Pb2+ in water bodies or < 400 mg/kg Pb2+ in soils) (Qian et al., 2017; Peng et al., 2020). However, the reality is far worse than people imagined (Chen et al., 2023). For instance, lead-containing wastewater with a Pb2+ concentration of 147 mM was found close to a lead-zinc smelting plant in Southeast China (Duan et al., 2016).
Fang et al. (2021) reported that Ca2+ addition enhanced bacterial tolerance to Cd2+ through competitive adsorption. The minimum inhibition concentration may affect Pb immobilization, although the initial urease activity (UA) was positively correlated to bacterial tolerance (Jiang et al., 2019; Duarte-Nass et al., 2020). Nano-hydroxyapatite (nHAP), with a chemical formula Ca10(PO4)6(OH)2, has a strong potential to work with EICP as it features a large specific surface area and high solubility (Xie et al., 2024; Wang et al., 2024). Its combination with heavy metal ions is as expected (Javadinejad et al., 2017). However, a large body of research does not pay attention to the pathways applied to Pb immobilization, how the nHAP application changes the nucleation and crystallization of bioprecipitates, and the interplay with Ca2+ application. The above accentuates several research gaps and shortcomings that remain to be addressed. The main objectives of this study are to: (1) investigate the role of nHAP in preventing Pb2+ attachment; and (2) explore the inherent mechanism that affects Pb immobilization.
2 Materials and methods
2.1 Urease extraction and nano-hydroxyapatite
This study tested the tolerance of urease to 20 mM–60 mM Pb2+ (Duan et al., 2016; Jiang et al., 2019). Then the results were compared to those that neglected the nHAP application to highlight the relative merits of the nHAP application. Apart from that, the enhancement of Pb immobilization would not have been achieved if the inherence mechanism had not been explored. To this end, a series of samples taken from the bioprecipitates were characterized through SEM, SEM-EDS, FTIR, and XRD. These provide details about the design of experiments and the logic behind this study.
This study first extracted crude urease from jack beans (Canavalia ensiformis L.). After crushing and sieving the dried jack beans, the bean powder was yielded. It helped prepare a stock solution stored at minus four degrees Celsius. Then the stock solution was centrifuged allowing the supernatant to be extracted as the urease solution. Pb2+ attachment could cause the urease to lose its activity, leading to an inability to secure the degree of urea hydrolysis and immobilization efficiency. This study attempted to tackle the said problem by introducing nHAP. nHAP is originally a natural apatite mineral. It could also be synthetic nowadays as a result of the strong demand in bone tissue engineering (Qiu et al., 2021). Its large specific surface area creates an ideal environment for combination with heavy metal ions. nHAP, acquired from Shanghai Macklin Biochemical Co., Ltd, consisted of hydroxyapatite nanoparticles of 60–80 nm in average size and assisted EICP in reducing the mobility of Pb2+, which is referred to also as ‘EICP-nHAP’.
2.2 Characteristics of urease
UA corresponds to the rate of urea hydrolysis. In the present work, UA was measured via a method suggested by Whiffin et al. (2007), in which 1 mL urease solution is added to 9 mL urea solution at 1.11 M, and the resultant change in electric conductivity (EC) over a 5-min period at 20°C ± 2°C was applied to determine UA by Eq. 1.
where EC0 and EC5 are identical to EC at 0 and 5 min, respectively.
2.3 Pb immobilization
EICP as a basis for comparison was applied to the present work when subjected to 20–60 mM Pb2+. Pb-containing solutions were prepared using reagents consisting of 100 mM urea and lead nitrate. Then a 5% urease solution was inoculated into a 100 mLPb-containing solution to catalyze urea hydrolysis toward achieving Pb immobilization. This process remained for a 48-h period. Once the remaining Pb2+ concentration was measured, the immobilization efficiency can be evaluated through Eq. 2:
where C0 and C1 represent the initial and remaining Pb2+ concentrations, respectively. To highlight the relative merits of EICP-nHAP, 100 mg nHAP was appended to the Pb-contaminated solution at the beginning. The remaining steps applied to catalyze urea hydrolysis were the same as in EICP. Then a comparison in the immobilization efficiency between EICP and EICP-nHAP not only enhanced our understanding of the relative merits of EICP-nHAP but also explored the potential of applying EICP-nHAP to Pb-containing water bodies remediation. Apart from that, NH4+ concentration, surrounding pH, and Pb2+ concentration were recorded per the given frequencies. A Nessler spectrophotometer, a pH meter (HI2003; Hanna Instruments Inc., Italy), and an atomic adsorption spectrophotometer (TAS-990; Beijing Purkinje General Instrument Co., Ltd., China) helped to accomplish the measurements.
On the other hand, one-dimensional soil column tests were conducted to see how Pb species affected Pb immobilization. To this end, a series of block loess samples were taken from northwest China. The loess contained 14.2% sand and 85.8% fines content. Its liquid limit (LL) and plasticity index (PI) were 24.4%, and 10.2%, respectively. It was classified as low plasticity clay (CL) using the Unified Soil Classification System (USCS) (ASTM D2487-17, 2010). A mixture of Pb-contaminated loess and nHAP was loaded into the one-dimensional columnar container of 180 mm in height and 61.8 mm in diameter applied to the one-dimensional soil column tests. Three Pb2+ concentrations, namely, 4,000 mg/kg, 8,000 mg/kg, and 16,000 mg/kg, were chosen. After that, the urea solution was injected at 5 mL/min into the Pb-contaminated loess. A control group (CG) that neglected both EICP and EICP-nHAP was set up for comparison sake. The samples taken after 24 h were analyzed based on Tessier sequential extraction procedure.
2.4 Sample characterization
The bioprecipitates were extracted following the EICP (or EICP-nHAP) process, preparing samples using the freeze-drying method. The frozen temperature was set at −5°C. The frozen samples were then stood still at −50°C. SEM, SEM-EDS, FTIR, and XRD were applied in their characterization. The SEM and SEM-EDS images were acquired from a scanning electron microscope (Sigma 300; ZEISS, Germany). The chemical bonds and surface functional groups (e.g., hydroxyl and carboxyl groups) involved in Pb immobilization were analyzed via a Fourier transform infrared spectrometer (Nicolet iS50; Thermo Scientific, United States of America). While the mineralogical compositions of the samples were identified using an X-ray diffractometer (D8 Advance; Bruker AXS, Germany) where the 2θ varied within a range of 5°–85°, with a step width of 0.02° and a scanning rate of 2°/min. MDI Jade software (version 9.5) was responsible for XRD pattern processing.
Five Pb species in Tessier sequential extraction procedure were measured by an atomic absorption spectrometer (TAS-990; Persee Inc., China) where EXC denoted Exchangeable state-Pb, CAR denoted Carbonate combination state-Pb, OX denoted Fe–Mn oxides state-Pb, ORG denoted Organic state-Pb, and RES denoted Residue state-Pb. Three replicates were applied to each of the tests to verify the significance of the results, and the error bars on a graph were used to help see the margins of error and standard deviations at a glance.
3 Results and discussion
3.1 Efficiency deterioration
The temporal relationships of pH and electric conductivity (EC) under EICP and EICP-nHAP are shown in Figure 1A. Under EICP, pH, when subjected to 20 mM Pb2+, increased sharply and then reached approximately 8.5 at 48 h after the hydrolysis of urea. However, pH increased at a slower pace and then reached 6.9 at 48 h when subjected to 40 mM Pb2+, indicating the effect of Pb2+ toxicity. The most significant effect of Pb2+ toxicity was present at 60 mM Pb2+. This led to an inability for the urease to catalyze urea hydrolysis, leading to a pH of below 6.5 at 48 h. The temporal relationships of EC are shown in Figure 1B. Similarly, the value of EC went down when Pb2+ concentration went up. The difference in EC between 20 mM Pb2+ and 60 mM Pb2+ reached 4.5 m. The variations of NH4+ concentration against Pb2+ concentration are depicted in Figure 2A. The more significant the effect of Pb2+ toxicity, the lower the NH4+ concentration, and the lower the degree of urea hydrolysis. These results led us to summarize that the urease lost its activity when Pb2+ concentration exceeded 20 mL/L and that the immobilization efficiency dropped to a value as low as about 25% as a result of a reduction in the degree of urea hydrolysis (see Figure 2B).
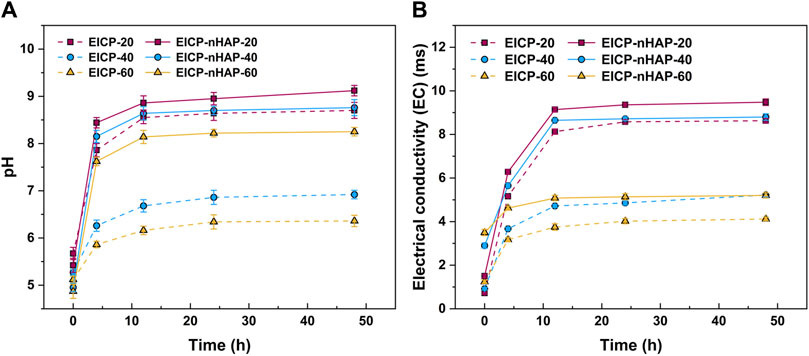
Figure 1. Temporal relationships of (A) pH and (B) EC applied to EICP and EICP-nHAP when subjected to 20 mM, 40 mM, and 60 mM Pb2+.
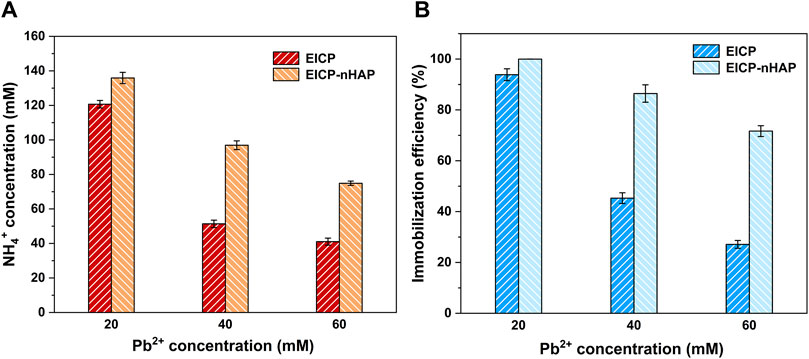
Figure 2. (A) Relationships of NH4+ concentration versus Pb2+ concentration applied to EICP and EICP-nHAP and (B) relationships of immobilization efficiency versus Pb2+ concentration applied to EICP and EICP-nHAP.
3.2 Pb immobilization enhancement
By contrast, under EICP-nHAP, pH reached 9 and 8.3 at 48 h when subjected to 20 mM Pb2+ and 60 mM Pb2+, respectively (see Figure 1A). They were higher than those under EICP. EC was also higher under EICP-nHAP (see Figure 1B). The difference in EC between EICP and EICP-nHAP increased with increasing Pb2+ concentration. The difference in NH4+ between EICP and EICP-nHAP increased from 15 mM at 20 mM Pb2+ to 35 mM at 60 mM Pb2+ (see Figure 2A). The variations of immobilization efficiency against Pb2+ concentration are depicted in Figure 2B. The difference in immobilization efficiency between EICP and EICP-nHAP increased from 5% at 20 mM Pb2+ to 45% at 60 mM Pb2+. These results indicated that nHAP application prevented Pb2+ attachment and allowed UA and the degree of urea hydrolysis to remain high enough. As a result, the immobilization efficiency reached above 70% when even subjected to 60 mM Pb2+.
3.3 Sample characterization
Results from the SEM images showed that under EICP, non-nucleated, flaky minerals were formed as a result of the inability of urease to provide nucleation sites (Figures 3A, B). By contrast, nucleated, chubby rod-shaped minerals were precipitated under EICP-nHAP. Compared to the non-nucleated minerals, the nucleated minerals featured higher stability since nHAP addressed the inability to provide nucleation sites by the urease (Heidarzadeh et al., 2019). This also explained that the nucleated minerals were not precipitated arbitrarily but directionally. On the other hand, C and O elements were distributed uniformly in the SEM-EDS images, meaning that under EICP, carbonate minerals were considered the main contributor to Pb immobilization (Figures 3C, D). Ca and P elements were present in the SEM-EDS images, implying that under EICP-nHAP, hydroxyapatite ruled Pb immobilization.
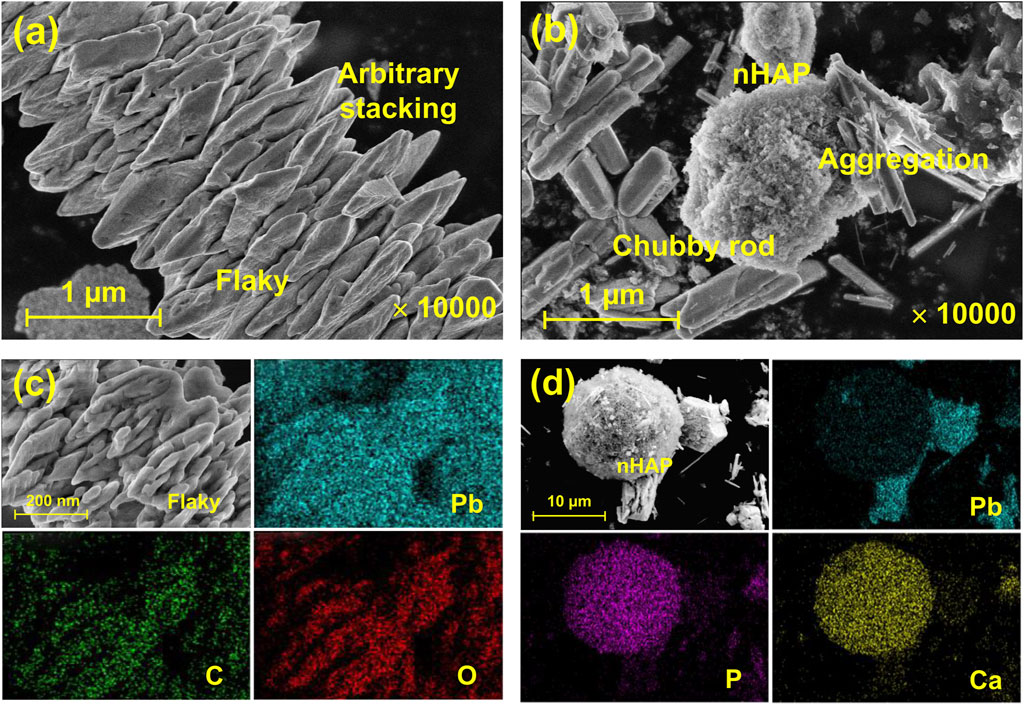
Figure 3. SEM test results applied to (A) EICP (x 10,000) and (B) EICP-nHAP (x 10,000); SEM-EDS test results applied to (C) EICP (x 2000) and (D) EICP-nHAP (x 1,000).
Under EICP, the diffraction peak at 3.554 Å was ascribed to forming cerussite (PbCO3), although its formation was also related to other diffraction peaks of relatively weak intensity at 3.054 Å (Figure 4). Hydroxylphosphohedyphane (Ca10-xPbx(PO4)6(OH)2) and hydroxylpyromorphite (Pb10(PO4)6(OH)2) were present under EICP-nHAP. On the other hand, the adsorption bands were specified at 1730 cm−1 and 1,050 cm−1, which were conformable to C = O and C-O stretching vibrations, respectively (Figure 5) (Bao et al., 2008; Timilsena et al., 2015; Ren et al., 2022). Carbonates were characterized by strong bands at 1,400 cm−1 (C-O bond antisymmetric stretch) and in the 678–838 cm−1 (CO32- deformation) region. These results provided eloquent testimony to support the above claim that carbonate minerals were the main contributor to Pb immobilization under EICP. Under EICP-nHAP, the nHAP application caused the band to shift to 1,035 cm−1 from 1,050 cm−1. Phosphates were also specified by strong adsorption bands within the 567–603 cm−1 (P-O stretch) region. These results were ascribed to the presence of PO43−, indicating that hydroxyapatite primarily governed Pb immobilization under EICP-nHAP (Gao et al., 2019).
CAR-Pb was the major species (approximately 50%) under EICP, while OX-Pb was the major species (about 46.7%) under EICP-nHAP (see Figure 6). In addition to transforming CAR-Pb into OX-Pb, EICP-nHAP reduced the fraction of EXC-Pb further to 2.9% at 4,000 mg/kg Pb2+ and to 5.2% at 8,000 mg/kg Pb2+ as well as to 6.5% at 16,000 mg/kg Pb2+.
3.4 Enhancement mechanism
Compared to the immobilization efficiency higher than 90% at 20 mM Pb2+, it dropped dramatically to way below 30% at 60 mM Pb2+, meaning that the effect of Pb2+ toxicity at 60 mM Pb2+ was not as less significant as 20 mM Pb2+. The urease lost its activity, leading to an inability to catalyze urea hydrolysis and also to secure Pb immobilization. In addition to addressing the inability to provide nucleation sites by the urease, the nHAP application also prevented Pb2+ attachment to the urease. The immobilization efficiency, therefore, remained above 70% when even subjected to 60 mM Pb2+. C and O elements over a broad range of the SEM-EDS images indicated that under EICP, carbonate minerals contributed primarily to Pb immobilization. Carbonates characterized by strong bands at 1,400 cm−1 and in the 678–838 cm−1 region provided a strong piece of evidence to support the above claim. On the other hand, Ca and P elements over a broad range of the SEM-EDS images told that under EICP-nHAP, hydroxyapatite was crucial in Pb immobilization. Phosphates specified by strong bands in the 567–603 cm−1 region verified the presence of hydroxyapatite (Gao et al., 2019). As discussed above, the nHAP application also adsorbed a part of Pb2+ by competing with urease, preventing Pb2+ attachment to the urease. This further explained that Pb element distribution under EICP-nHAP was not as even as EICP. Furthermore, the nHAP application was accompanied by Ca2+/Pb2+ ion exchange (Hopwood et al., 2016). Hydroxylphosphohedyphane or hydroxylpyromorphite at 4.096 Å gave eloquent testimony to support the presence of Ca2+/Pb2+ ion exchange. Noted that the former was developed when Ca2+ was partially replaced with Pb2+. Apart from that, the hydroxyl (-OH) in hydroxylpyromorphite was replaced by CO32− at 20 mM Pb2+, forming carbonate-bearing hydroxylpyromorphite at typically 3.045 Å (Kwasniak-Kominek et al., 2017). It featured Ksp = 3.98 × 10−85 lower than Ksp = 7.4 × 10−14 of the cerussite (often seen under EICP), indicating higher chemical stability. This was not the case when subjected to 60 mM Pb2+. Hydroxylphosphohedyphane or hydroxylpyromorphite, when subjected to 60 mM Pb2+, was formed, followed by cerussite. This allowed hydroxylphosphohedyphane or hydroxylpyromorphite to be wrapped by cerussite, reducing the mobility of Pb2+. This explained why cerussite appeared more frequently than hydroxylphosphohedyphane or hydroxylpyromorphite.
On the other hand, the function of nHAP was verified through the stretching vibrations of its functional groups. Although carbonates characterized by strong bands at 1,400 cm−1 and in the 678–838 cm−1 region were also present under EICP-nHAP, they were not as significant as EICP. Phosphates specified in the 567–603 cm−1 region appeared under EICP-nHAP. The nHAP application also caused a shift from 1,050 cm−1 to 1,035 cm−1. These results gave testimony supporting the claim that the nHAP application prevented Pb2+ attachment to the urease and enhanced Pb immobilization. Last but not the least, the good compatibility of nHAP encouraged forming aggregates with the urease, as indicated by the SEM images. The aggregates were applied for biomineralization as nucleation sites. This explained why the nucleated minerals featured stability higher than non-nucleated minerals. The advantages attained under EICP-nHAP elevated the immobilization efficiency to fall within a range of 70%–100%. The horizon of application of this approach is proven rather broad despite the neglection of other influencing factors (e.g., salt pollution).
4 Conclusion
The present work compared the performance in Pb immobilization between EICP and EICP-nHAP, and the results accentuated the relative merits of EICP-nHAP. Based on the results and discussion, some main conclusions can be drawn as follows: (ASTM D2487-17, 2010)
(1) The urease lost its activity when Pb2+ concentration was in excess of 20 mL/L and therefore, the immobilization efficiency dropped to a value as low as about 25%. nHAP application prevented the urease from being threatened by the effect of Pb2+ toxicity, thereby securing the degree of urea hydrolysis. As a result, the immobilization efficiency remained at above 70% when even subjected to 60 mM Pb2+.
(2) In addition to addressing the inability to provide nucleation sites by the urease, the nHAP application also prevented Pb2+ attachment to the urease. Hydroxylphosphohedyphane and hydroxylpyromorphite provided a strong piece of evidence to support the presence of Ca2+/Pb2+ ion exchange. The carbonate-bearing minerals were formed at 20 mM Pb2+ and featured with stability higher than cerussite (Ksp = 3.98 × 10−85 vs. Ksp = 7.4 × 10−14). Hydroxylphosphohedyphane or hydroxylpyromorphite +was wrapped by cerussite at 60 mM Pb2+, reducing the mobility of Pb2+.
(3) The good compatibility of nHAP encouraged forming aggregates with the urease. The aggregates were applied for biomineralization as nucleation sites. This explained why the nucleated minerals featured higher stability. As a result, the advantages attained under EICP-nHAP elevated the immobilization efficiency to fall within a range of 70%–100%.
(4) CAR-Pb was the major species under EICP, while OX-Pb was the major species under EICP-nHAP. Both EICP and EICP-nHAP reduced the fraction of EXC-Pb. OX-Pb was featured with higher stability and lower ecotoxicity than CAR-Pb.
Data availability statement
The original contributions presented in the study are included in the article/Supplementary Material, further inquiries can be directed to the corresponding author.
Author contributions
Z-WB: Data curation, Formal Analysis, Software, Validation, Writing–original draft. W-CC: Conceptualization, Funding acquisition, Methodology, Supervision, Writing–review and editing. Y-XX: Data curation, Formal Analysis, Software, Validation, Writing–original draft. MR: Supervision, Writing–review and editing. WH: Data curation, Software, Writing–original draft.
Funding
The author(s) declare that financial support was received for the research, authorship, and/or publication of this article. This paper is based upon work supported by the Shaanxi Educational Department (2020TD-005) and the Shaanxi Housing and Urban-Rural Development Office (2018-K15).
Conflict of interest
Author Z-WB was employed by Shaanxi Jianke Construction Special Engineering Co., Ltd.
The remaining authors declare that the research was conducted in the absence of any commercial or financial relationships that could be construed as a potential conflict of interest.
The author(s) declared that they were an editorial board member of Frontiers, at the time of submission. This had no impact on the peer review process and the final decision.
Publisher’s note
All claims expressed in this article are solely those of the authors and do not necessarily represent those of their affiliated organizations, or those of the publisher, the editors and the reviewers. Any product that may be evaluated in this article, or claim that may be made by its manufacturer, is not guaranteed or endorsed by the publisher.
References
Ashkan, N., Shahin, S., and Brina, M. (2019). Influence of microbe and enzyme-induced treatments on cemented sand shear response. J. Geotechnical and Geoenvironmental Eng. 145, 06019008. doi:10.1061/(asce)gt.1943-5606.0002111
ASTM D2487-17 (2010) “Standard practice for classification of soils for engineering purposes (Unified Soil Classification System),” in Annual book of ASTM standards. International West Conshohocken, PA: ASTM.
Bai, B., Jiang, S., Liu, L., Li, X., and Wu, H. (2021). The transport of silica powders and lead ions under unsteady flow and variable injection concentrations. Powder Technol. 387, 22–30. doi:10.1016/j.powtec.2021.04.014
Bai, B., Bai, F., Li, X., Nie, Q., and Jia, X. (2022). The remediation efficiency of heavy metal pollutants in water by industrial red mud particle waste. Environ. Technol. Innov. 28, 102944. doi:10.1016/j.eti.2022.102944
Bai, B., Chen, J., Bai, F., Nie, Q., and Jia, X. (2024). Corrosion effect of acid/alkali on cementitious red mud-fly ash materials containing heavy metal residues. Environ. Technol. Innov. 33, 103485. doi:10.1016/j.eti.2023.103485
Bao, X. L., Lv, Y., Yang, B. C., Ren, C. G., and Guo, S. T. (2008). A study of the soluble complexes formed during calcium binding by soybean protein hydrolysates. J. Food Sci. and Technol. 73, C117–C121. doi:10.1111/j.1750-3841.2008.00673.x
Chen, X., Cao, X. F., Liu, B. B., Nie, X. K., Liang, T. B., Suhr, J., et al. (2021). Effects of functional carbon nanodots on water hyacinth response to Cd/Pb stress: implication for phytoremediation. J. Environ. Manag. 299, 113624. doi:10.1016/j.jenvman.2021.113624
Chen, L., Wang, F., Zhang, Z., Chao, H., He, H., Hu, H., et al. (2023). Influences of arbuscular mycorrhizal fungi on crop growth and potentially toxic element accumulation in contaminated soils: a meta-analysis. Crit. Rev. Environ. Sci. Technol. doi:10.1080/10643389.2023.2183700
Chen, L., Chang, N., Qiu, T., Wang, N., Cui, Q., Zhao, S., et al. (2024a). Meta-analysis of impacts of microplastics on plant heavy metal(loid) accumulation. Environ. Pollut. 348, 123787. doi:10.1016/j.envpol.2024.123787
Chen, L., Fang, L., Yang, X., Luo, X., Qiu, T., Zeng, Y., et al. (2024b). Sources and human health risks associated with potentially toxic elements (PTEs) in urban dust: a global perspective. Environ. Int. 187, 108708. doi:10.1016/j.envint.2024.108708
Costa de Almeida, G. R., Costa de Almeida, M. D. C., Barbosa, F. J., Krug, F. J., Cury, J. A., Rosário de Sousa, M. D. L., et al. (2007). Lead contents in the surface enamel of deciduous teeth sampled in vivo from children in uncontaminated and in lead contaminated areas. Environ. Res. 104, 337–345. doi:10.1016/j.envres.2007.03.007
Duan, Q. N., Lee, J. C., Liu, Y. S., Chen, H., and Hu, H. Y. (2016). Distribution of heavy metal pollution in surface soil samples in China: a graphical review. Bull. Environ. Contam. and Toxicol. 97, 303–309. doi:10.1007/s00128-016-1857-9
Duarte-Nass, C., Rebolledo, K., Valenzuela, T., Kopp, M., Jeison, D., Rivas, M., et al. (2020). Application of microbe-induced carbonate precipitation for copper removal from copper-enriched waters: challenges to future industrial application. J. Environ. Manag. 256, 109938. doi:10.1016/j.jenvman.2019.109938
Fang, L. Y., Niu, Q. J., Cheng, L., Jiang, J. X., Yu, Y. Y., Chu, J., et al. (2021). Ca-mediated alleviation of Cd2+ induced toxicity and improved Cd2+ biomineralization by Sporosarcina pasteurii. Sci. Total Environ. 787, 147627. doi:10.1016/j.scitotenv.2021.147627
Gao, M., Wang, W., Yang, H. B., and Ye, B. C. (2019). Hydrothermal synthesis of hierarchical hollow hydroxyapatite microspheres with excellent fluoride adsorption property. Microporous and Mesoporous Mater. 289, 109620. doi:10.1016/j.micromeso.2019.109620
Gowthaman, S., Yamamoto, M., Chen, M., Nakashima, K., and Kawasaki, S. (2023). Baseline investigation on enzyme induced calcium phosphate precipitation for solidification of sand. Front. Built Environ. 9, 1307650. doi:10.3389/fbuil.2023.1307650
Gowthaman, S., Yamamoto, M., Nakashima, K., Ivanov, V., and Kawasaki, S. (2021). Calcium phosphate biocement using bone meal and acid urease: an eco-friendly approach for soil improvement. J. Clean. Prod. 319, 128782. doi:10.1016/j.jclepro.2021.128782
Heidarzadeh, N., Rafizadeh, M., Taromi, F. A., Puiggalí, J., and del Valle, L. J. (2019). Nucleating and retarding effects of nanohydroxyapatite on the crystallization of poly(butylene terephthalate-co-alkylene dicarboxylate)s with different lengths. J. Therm. Analysis and Calorim. 137 (2), 421–435. doi:10.1007/s10973-018-7953-9
Hopwood, J. D., Derrick, G. R., Brown, D. R., Newman, C. D., Haley, J., Kershaw, R., et al. (2016). The identification and synthesis of lead apatite minerals formed in lead water pipes. J. Chem. 2016, 1–11. doi:10.1155/2016/9074062
Hu, W. L., Cheng, W. C., Wang, Y. H., and Wen, S. J. (2023a). Feasibility study of applying a graphene oxide-alginate composite hydrogel to electrokinetic remediation of Cu (II)- contaminated loess as electrodes. Sep. Purif. Technol. 322, 124361. doi:10.1016/j.seppur.2023.124361
Hu, W. L., Cheng, W. C., Wang, Y. H., Wen, S. J., and Xue, Z. F. (2023b). Applying a nanocomposite hydrogel electrode to mitigate electrochemical polarization and focusing effect in electrokinetic remediation of a Cu- and Pb-contaminated loess. Environ. Pollut. 333, 122039. doi:10.1016/j.envpol.2023.122039
Javadinejad, H. R., Rizi, M. S., Mobarakeh, E. A., and Ebrahimian, M. (2017). Thermal stability of nano-hydroxyapatite synthesized via mechanochemical treatment. Arabian J. Sci. and Eng. 42, 4401–4408. doi:10.1007/s13369-017-2498-y
Jiang, N. J., Liu, R., Du, Y. J., and Bi, Y. Z. (2019). Microbial induced carbonate precipitation for immobilizing Pb contaminants: toxic effects on bacterial activity and immobilization efficiency. Sci. Total Environ. 672, 722–731. doi:10.1016/j.scitotenv.2019.03.294
Karaouzas, I., Kapetanaki, N., Mentzafou, A., Kanellopoulos, T. D., and Skoulikidis, N. (2020). Heavy metal contamination status in Greek surface waters: a review with application and evaluation of pollution indices. Chemosphere 263, 128192. doi:10.1016/j.chemosphere.2020.128192
Kwasniak-Kominek, M., Manecki, M., Matusik, J., and Lempart, M. (2017). Carbonate substitution in lead hydroxyapatite Pb5(PO4)3OH. J. Mol. Struct. 1147, 594–602. doi:10.1016/j.molstruc.2017.06.111
Liu, Y., Xu, L., Su, J. F., Ali, A., Huang, T. L., Wang, Y., et al. (2024). Microbially driven Fe-N cycle: intrinsic mechanisms, enhancement, and perspectives. Sci. Total Environ. 908, 168084. doi:10.1016/j.scitotenv.2023.168084
Meng, Z. W., Huang, S., Xu, T., Lin, Z. B., and Wu, J. W. (2022). Competitive adsorption, immobilization, and desorption risks of Cd, Ni, and Cu in saturated-unsaturated soils by biochar under combined aging. J. Hazard. Mater. 434, 128903. doi:10.1016/j.jhazmat.2022.128903
Peng, D. H., Qiao, S. Y., Luo, Y., Ma, H., Zhang, L., Hou, S. Y., et al. (2020). Performance of microbial induced carbonate precipitation for immobilizing Cd in water and soil. J. Hazard. Mater. 400, 123116. doi:10.1016/j.jhazmat.2020.123116
Qian, X. Y., Fang, C. L., Huang, M. S., and Achal, V. (2017). Characterization of fungal-mediated carbonate precipitation in the biomineralization of chromate and lead from an aqueous solution and soil. J. Clean. Prod. 164, 198–208. doi:10.1016/j.jclepro.2017.06.195
Qiu, X. T., Rao, C. Y., Li, T., and Zhou, R. H. (2021). Research progress in biomimetic synthesis of nano-hydroxyapatite in bone tissue engineering. J. Sichuan Univ. 52, 740–746. doi:10.12182/20210560201
Ren, B., Jin, Y., Zhao, L., Cui, C., and Song, X. (2022). Enhanced Cr(VI) adsorption using chemically modified dormant Aspergillus Niger spores: process and mechanisms. J. Environ. Chem. Eng. 10, 106955. doi:10.1016/j.jece.2021.106955
Timilsena, Y. P., Adhikari, R., Kasapis, S., and Adhikari, B. (2015). Rheological and microstructural properties of the chia seed polysaccharide. Int. J. Biol. Macromol. 81, 991–999. doi:10.1016/j.ijbiomac.2015.09.040
Wang, L., Cheng, W. C., Xue, Z. F., Xie, Y. X., and Lv, X. J. (2023). Study on Cu- and Pb-contaminated loess remediation using electrokinetic technology coupled with biological permeable reactive barrier. J. Environ. Manage. 348, 119348. doi:10.1016/j.jenvman.2023.119348
Wang, L., Cheng, W. C., Xue, Z. F., Rahman, M. M., and Xie, Y. X. (2024a). Struvite and ethylenediaminedisuccinic acid (EDDS) enhance electrokinetic-biological permeable reactive barrier removal of copper and lead from contaminated loess. J. Environ. Manage. 360, 121100. doi:10.1016/j.jenvman.2024.121100
Wang, Z., Su, J. F., Zhao, T. B., Li, J. W., and Zhang, L. F. (2024b). Enhanced removal of fluoride from groundwater using biosynthetic hydroxyapatite modified by bimetallic (La–Fe or La–Al) hydroxides. J. Clean. Prod. 436, 140649. doi:10.1016/j.jclepro.2024.140649
Whiffin, V. S., van Paassen, L. A., and Harkes, M. P. (2007). Microbial carbonate precipitation as a soil improvement technique. Geomicrobiol. J. 24, 417–423. doi:10.1080/01490450701436505
Xie, Y. X., Cheng, W. C., Wang, L., Xue, Z. F., Rahman, M. M., and Hu, W. L. (2022). Immobilizing copper in loess soil using microbial-induced carbonate precipitation: Insights from test tube experiments and one-dimensional soil columns. J. Hazard. Mater. 444, 130417. doi:10.1016/j.jhazmat.2022.130417
Xie, Y. X., Cheng, W. C., Xue, Z. F., Rahman, M. M., and Wang, L. (2024). Deterioration phenomenon of Pb-contaminated aqueous solution remediation and enhancement mechanism of nano-hydroxyapatite-assisted biomineralization. J. Hazard. Mater. 470, 134210. doi:10.1016/j.jhazmat.2024.134210
Xu, L., Yang, Y. Z., Su, J. F., He, C., Shi, J., Yan, H., et al. (2023). Simultaneous removal of nitrate, lead, and tetracycline by a fixed-biofilm reactor assembled with kapok fiber and sponge iron: comparative analysis of operating conditions and biotic community. Environ. Res. 219, 115163. doi:10.1016/j.envres.2022.115163
Xue, Z. F., Cheng, W. C., Wang, L., and Hu, W. L. (2022). Effects of bacterial inoculation and calcium source on microbial-induced carbonate precipitation for lead remediation. J. Hazard. Mater. 426, 128090. doi:10.1016/j.jhazmat.2021.128090
Xue, Z. F., Cheng, W. C., Wang, L., Qin, P., Xie, Y. X., Hu, W. L., et al. (2023). Applying the first microcapsule-based self-healing microbial-induced calcium carbonate materials to prevent the migration of Pb ions. Environ. Res. 239, 117423. doi:10.1016/j.envres.2023.117423
Xue, Z. F., Cheng, W. C., Wang, L., Xie, Y. X., and Qin, P. (2024a). Effect of a harsh circular environment on self-healing microbial-induced calcium carbonate materials for preventing Pb2+ migration. Environ. Technol. Inno. 32, 103380. doi:10.1016/j.eti.2023.103380
Xue, Z. F., Cheng, W. C., Rahman, M. M., Wang, L., and Xie, Y. X. (2024b). Immobilization of Pb(II) by Bacillus megaterium-based microbial-induced phosphate precipitation (MIPP) considering bacterial phosphorolysis ability and Ca-mediated alleviation of lead toxicity. Environ Pollut. 355, 124229. doi:10.1016/j.envpol.2024.124229
Keywords: urea hydrolysis, lead, nano-hydroxyapatite, cerussite, carbonate-bearing hydroxylpyromorphite
Citation: Bian Z-W, Cheng W-C, Xie Y-X, Rahman MM and He W (2024) Nano-hydroxyapatite-assisted enzyme-induced carbonate precipitation enhances Pb-contaminated aqueous solution and loess remediation. Front. Bioeng. Biotechnol. 12:1410203. doi: 10.3389/fbioe.2024.1410203
Received: 31 March 2024; Accepted: 07 June 2024;
Published: 27 June 2024.
Edited by:
Eduardo Jacob-Lopes, Federal University of Santa Maria, BrazilReviewed by:
Shijun Wu, Chinese Academy of Sciences (CAS), ChinaSivakumar Gowthaman, University of Jaffna, Sri Lanka
Copyright © 2024 Bian, Cheng, Xie, Rahman and He. This is an open-access article distributed under the terms of the Creative Commons Attribution License (CC BY). The use, distribution or reproduction in other forums is permitted, provided the original author(s) and the copyright owner(s) are credited and that the original publication in this journal is cited, in accordance with accepted academic practice. No use, distribution or reproduction is permitted which does not comply with these terms.
*Correspondence: Wen-Chieh Cheng, dy1jLmNoZW5nQHhhdWF0LmVkdS5jbg==