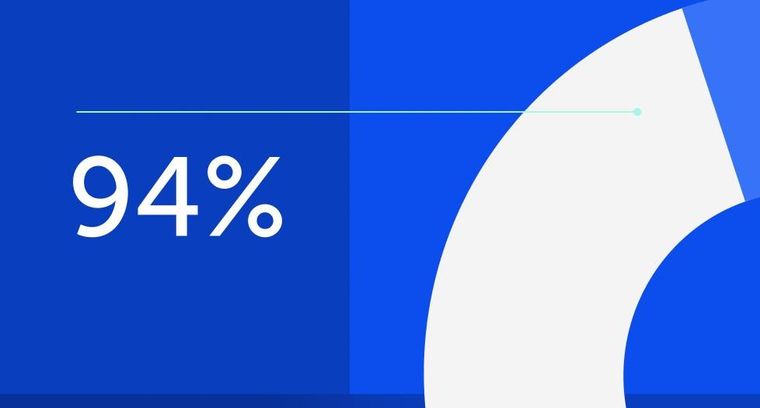
94% of researchers rate our articles as excellent or good
Learn more about the work of our research integrity team to safeguard the quality of each article we publish.
Find out more
REVIEW article
Front. Bioeng. Biotechnol., 28 May 2024
Sec. Tissue Engineering and Regenerative Medicine
Volume 12 - 2024 | https://doi.org/10.3389/fbioe.2024.1389733
This article is part of the Research TopicAdvances in Biotechnological Approaches for Reproductive Tissue EngineeringView all 9 articles
The repair of irregular bone tissue suffers severe clinical problems due to the scarcity of an appropriate therapeutic carrier that can match dynamic and complex bone damage. Fortunately, stimuli-responsive in situ hydrogel systems that are triggered by a special microenvironment could be an ideal method of regenerating bone tissue because of the injectability, in situ gelatin, and spatiotemporally tunable drug release. Herein, we introduce the two main stimulus-response approaches, exogenous and endogenous, to forming in situ hydrogels in bone tissue engineering. First, we summarize specific and distinct responses to an extensive range of external stimuli (e.g., ultraviolet, near-infrared, ultrasound, etc.) to form in situ hydrogels created from biocompatible materials modified by various functional groups or hybrid functional nanoparticles. Furthermore, “smart” hydrogels, which respond to endogenous physiological or environmental stimuli (e.g., temperature, pH, enzyme, etc.), can achieve in situ gelation by one injection in vivo without additional intervention. Moreover, the mild chemistry response-mediated in situ hydrogel systems also offer fascinating prospects in bone tissue engineering, such as a Diels–Alder, Michael addition, thiol-Michael addition, and Schiff reactions, etc. The recent developments and challenges of various smart in situ hydrogels and their application to drug administration and bone tissue engineering are discussed in this review. It is anticipated that advanced strategies and innovative ideas of in situ hydrogels will be exploited in the clinical field and increase the quality of life for patients with bone damage.
As the load-bearing structure of the human body, the bone skeleton plays crucial functions, such as constituting a motion system and protecting nerves and organs (Harada and Rodan, 2003; Wu and Chang, 2014). However, the bone tissue easily suffers damage due to crashing, falling, infection, and age (Stolzing et al., 2008). Although bone tissue possesses a strong self-repair capacity, it fails to self-heal when the defect exceeds a certain boundary. Therefore, the reconstruction of bone tissue is an extremely significant challenge for clinical surgeons. Several approaches have been exploited for treating bone damage, relying on various therapies (e.g., autografts, xenografts, and allografts) ((Harada and Rodan, 2003; Wu and Chang, 2014). However, these approaches still have challenges, such as donor site breakage, large segmental bone loss, morphology structure mismatch, etc. (Stolzing et al., 2008). To deal with those challenges, many therapeutic strategies using biomaterial-based systems (e.g., bone tissue engineering, bone organoids, etc.) have been exploited and utilized in vivo and in vitro and have demonstrated a satisfactory therapeutic effect (Liu S. et al., 2023).
The extracellular matrix (ECM) plays a vital role in signaling transfer and the exchange of nutrition and oxygen with emergent tissue (Shang et al., 2021). When designing novel biomaterial systems, it is necessary to match the properties of ECM to mimic the microenvironment of cell proliferative differentiation and improve bone tissue regeneration (Li J. et al., 2023). Hydrogels, an excellent biocompatible replacement material for the ECM, are considered to be the most promising applicants in bone tissue engineering because of their unique 3D-network structure, water-swellable, and adjustable physical and chemical properties (Cui et al., 2019). The hydrogel can also function as a carrier and depot for the delivery of drugs, bioactive peptides, and filling agents to adjust the tissue regeneration processes and boost the development of novel natural tissue (Zheng et al., 2023; Goh et al., 2024; Kalairaj et al., 2024; Park et al., 2024). Various hydrogel-based biomaterials have been extensively utilized in bone tissue regeneration, such as chitosan, gelatin, hyaluronic acid (HA), etc. (Neves et al., 2020; Zhuang and Cui, 2021). In addition, hydrogels have the potential to enhance the proliferation, adhesion, and differentiation properties of bone marrow mesenchymal stromal cells (BMSCs) during bone tissue regeneration (Chaudhuri et al., 2016). Thus, hydrogels are attractive biomaterials in bone tissue regeneration.
The synthesis and application of hydrogels have developed rapidly based on advanced technology, which paves a significant way for bone tissue regeneration (Choi et al., 2021; Yan et al., 2021; Zhang et al., 2022). Smart hydrogels are becoming more attractive in the biomaterial fields for promising applications (Balakrishnan and Banerjee, 2011; Chen et al., 2023). These strategies present several novel possibilities to overcome the shortcomings of traditional materials in bone tissue regeneration, including weak bioactivity and lack of spatial-temporal regulation (Xue et al., 2022a; Kurian et al., 2022). Injectable in situ hydrogels are usually modified to mimic the cell environment in terms of having similar properties to the ECM; they can match any defect shape (Liu et al., 2017; Hernández-González et al., 2020). Furthermore, the in situ hydrogel system used as a filler and carrier can be loaded with growth factors or bioactive molecules to enhance bone tissue regeneration and avoid surgical intervention in a nidus (Saravanan et al., 2019).
ISmart in situ hydrogels can be transformed from fluid to gel under external stimuli and can be used to fill and treat various irregularly shaped bone tissue defects. However, injectable hydrogels (which were in a gel state prior to injection) do not achieve this function. Although both can be stimulus-responsive, the stimulus response described in this article is mainly to realize the transformation of the gel precursor from a liquid state to a gel state in vivo. Traditional transplant hydrogels are manufactured before implantation and cannot be dynamically adjusted in an externally controlled and user-defined manner. Compared with traditional prefabricated surgical implant scaffolds, the most significant properties of these hydrogels is that they can be easily injected into the default site in situ and form a solid hydrogel, which helps to resist geometric deformation (Fan and Wang, 2017). However, the in situ hydrogel systems are subjected to the trigger approaches in the process of converting the precursor into a solid gel structure. To date, the trigger approaches of smart responsive in situ hydrogel systems are achieved by exogenous or endogenous stimuli. The exogenous stimuli strategies require an extra-activation instrument in the process of forming a solid 3D network hydrogel, such as ultraviolet (UV), near-infrared (NIR), or ultrasound (US) (Li et al., 2017). The endogenous stimuli strategies mainly depend on the interaction of both physical microenvironments and precursors (e.g., temperature, pH, enzyme, etc.) (Kumar and Mohammad, 2011; Cheng et al., 2016; Gong et al., 2017). To achieve the various smart responses, the various natural or synthetic hydrogels are usually modified by functional groups (e.g., unsaturated bond) or compounded with functional nanoparticles (e.g., MoS2, Cu2O, F3O4, etc.) (Faustino et al., 2023; Wihadmadyatami et al., 2023; Hong et al., 2024).
This review provides a brief overview of the various triggers of in situ hydrogels in smart crosslinking hydrogel administration and bone tissue regeneration (Figure 1). The various conceptions of trigger approaches for in situ hydrogels in bone tissue engineering are highlighted and commented on. In this work, we first introduce the bone tissue structure and microenvironment. Then, we summarize specific and distinct responses to various external stimuli (e.g., UV, NIR, US, etc.) by in situ formed hydrogels, which modified biocompatible materials by various functional groups or hybrid functional nanoparticles. Furthermore, we summarize smart hydrogels that respond to physiological environmental stimuli (e.g., temperature, enzyme, etc.) to form an in situ delivery system in bone tissue. Lastly, we devote this review to describing the various injectable in situ hydrogel systems and their applications in bone tissue. We hope that this review can provide a basic guide for further development of smart responsive in situ hydrogels in bone tissue engineering and bone organoid culturing.
Figure 1. Different stimulus-responsive in situ hydrogel systems based on biomaterials were explored to obtain excellent treatment in bone tissue engineering. Exogenous stimulus-responsive systems form in situ hydrogels in response to triggers such as UV, NIR, US, etc. Endogenous stimulus-responsive systems form in situ hydrogels in response to internal triggers such as temperature, enzyme, etc.
Bone is a hierarchical hard tissue that is mineralized and neurovascularized, supports the skeleton, and has many functions, including immunoregulation, growth, movement, hematopoiesis, and organ protection (Sun W. et al., 2023; Wang P. et al., 2024). The homeostasis regulation of bone tissue is primarily relayed in the microenvironment of the ECM. The bone microenvironment is a complex dynastic system composed of a specific ECM, integrins, growth factors, and functional cells (e.g., hematopoietic lineage cells, mesenchymal lineage cells, etc.) (Li Y. Y. et al., 2023; Shi et al., 2023). By gaining a deep understanding of this special biological and physical environment of bone tissue, we can draw inspiration to design more appropriate bone tissue regeneration approaches and promote the bone-healing process.
Bone tissue is a highly dynamic and complex structure, which is constantly updated and iterated via the balance of the osteoblasts and osteoclasts (Rodolfi et al., 2023). The structure of bone primarily includes the periosteum, sclerotin, and bone marrow (Abdalla and Pendegrass, 2023). The periosteum is made up of fibrous connective tissue, which is rich in nerves and blood vessels and plays a vital role in bone nutrition, regeneration, and sensation (Caffarelli et al., 2023). Sclerotin is a hierarchical and anisotropic structure, which is mainly composed of dense cortical bone and spongy cancellous bone (Delawan et al., 2023; Tang et al., 2023). The dense cortical bone possesses high mechanical strength and encompasses the cancellous bone on the periphery, which possesses an excellent stabilizing and supporting function. The sponge-like cancellous bone consists of countless flaky trabeculated bones, which are laid out inside the bone. Bone marrow is present in the spongy cancellous bone space and the bone marrow cavity of long bones and is composed of various types of cells and reticulated connective tissue (Caffarelli et al., 2023; Cao et al., 2024). Thus, the bone tissue can be precisely divided into multiple levels for investigation. For example, at the micron level, the cortical bone is arranged along the long axis of the bone. An osteon is the functional basic unit of the long bone, consisting of lamellar bones arranged in concentric circles. Nerve fibers and blood vessels pass through the bones to form the Havers system. The cancellous bone, an anisotropic arrangement of rod-like trabecular bones, forms a honeycomb-like network. Observing the microscopic structure, the main component of ECM is collagen fibers, which have a diameter of approximately 35–60 nm. The collagen fibers are formed by the self-assembly of unique triple-helix biomolecules (Yin and Li, 2006; Wegst et al., 2015; Zhu et al., 2021). Hydroxyapatite crystals with superior anisotropic mechanical properties play a vital role during controlled bio-mineralization (Figure 2A).
Figure 2. Schematic diagram of bone tissue structure. (A) Bone hierarchical structure (Zhu et al., 2021). HAP, hydroxyapatite; GAGs, glycosaminoglycans; NCPs, non-collagenous proteins. Copyright 2021, Elsevier. (B) Schematic diagram of cartilage and subchondral bone.
Cartilage is a powerful gliding tissue that covers the moving extremities of the bone skeleton. However, cartilage regeneration is challenging due to its limited ability to self-heal, owing to complex structures and components and a lack of blood vessels (Zhang et al., 2023). Cartilage primarily consists of chondrocytes and ECM (mainly composed of water and type II collagen fibers) (Sun L. et al., 2023). Furthermore, the cartilage defects may initiate the development of osteoarthritis (OA). One work demonstrated bottom-up primary degeneration from subchondral bone during cartilage erosion in OA with aging (Wang H. et al., 2024). The special relationship between subchondral bone and cartilage via various molecular signaling has been widely reported (Wu Z. et al., 2023; Ouyang et al., 2023). For example, the subchondral bone builds a bridge between cartilage and bone to provide both mechanical and nutritional support for cartilage and maintain the stability of the cartilage microenvironment, which is the bony layer below the hyaline cartilage and cement line (Luo et al., 2024). Anatomically, the subchondral bone consists of the subchondral plate and the subchondral bone trabeculae. The subchondral plate is a dense and crisscrossed poly-porous calcified plate. The subchondral bone trabeculae possess a spongy-like cancellous structure behind the subchondral plate, which contributes to continuous bone regeneration and remodeling and maintaining the physiological homeostasis microenvironment of the cartilage (Su et al., 2023; Yu et al., 2023; Zhao et al., 2024) (Figure 2B).
The ideal candidate biomaterials can bridge tissue damage and improve regeneration by enhancing new natural tissue regeneration (Xue et al., 2021). Simultaneously, they are also required to demonstrate excellent biocompatible, tunable mechanical properties and biodegradation as they degrade gradually during tissue repair (Wu D. et al., 2024; Zhou H. et al., 2024; Vaidya et al., 2024). To date, a variety of natural and synthetic biomaterials have been applied in an attempt to simulate ECM of the natural bone tissue (Xu et al., 2023; Sung et al., 2024; Yoon et al., 2024). Hydrogels are regarded as the leader in biomedical and tissue regeneration engineering applications, owing to their 3D structure and characters (Xue et al., 2022b). However, the extensive clinical application of hydrogels faces significant challenges in matching the complex functional and pathological alterations of the human body. Therefore, novel hydrogel biomaterials with smart properties to promote clinical practices in biomedicine and tissue regeneration engineering are needed. Smart responsive in situ hydrogels are extensively applied in bone tissue engineering (Ali et al., 2024; Asadi et al., 2024; Zhou H. et al., 2024; Garg et al., 2024). This strategy can guide hydrogels to adapt to diverse shapes and depths of bone defects, which will promote integration with bone tissue. Among the various fabrication strategies, an exogenous stimulus is usually selected to fabricate in situ hydrogels for bone repair because of easy control and manipulation via adjusting the input energy flow density. This section mainly reviews the various exogenous stimulus phase transition strategies used for in situ hydrogel applications in bone tissue engineering (e.g., UV, NIR, etc.), as shown in Figure 3.
Figure 3. Scheme of various exogenous stimulus strategies for in situ hydrogel triggering in bone tissue engineering.
Photo-sensitive hydrogels have the ability to achieve a liquid-gel transition when exposed to special wavelengths of light stimuli (Patel et al., 2022). They usually include polymeric systems and functional photoreceptive parts, in which the physicochemical properties are altered (liquid-gel) after light exposure. They have multiple biomedical applications and mainly utilize visible light. Photo-responsiveness is an attractive stimulus method because of its advantages (e.g., light is non-invasive and allows remote control of materials without byproducts). In addition, the light could be precisely modulated by irradiation parameters (e.g., light intensity, irradiation time, input power) to regulate the physical properties of the gels.
However, most natural biomaterials fail to respond to the trigger condition due to the absence of photo-responsive functional groups (Ding et al., 2015). Fortunately, these in situ response systems are achieved by introducing functional groups through photochemical addition reactions or activation of functional groups via the release of photo-caging groups and photoisomerization processes. Some bioactive materials are modified via methacryloyl (MA) or unsaturated bond groups (C=C or clickable C≡C) to respond to light triggers (e.g., gelatin methacryloyl (GelMA) (Zhang et al., 2016; Byambaa et al., 2017; Sun X. M. et al., 2018), hyaluronic acid- butyramide (HA-NB) (Zhang et al., 2016), polyethylene glycol (PEG) (Burke et al., 2019), etc.). For example, Qiao et al. (2020) designed a novel injectable in situ osteogenic polypeptide hydrogel system (GelMA-c-OGP) via ultraviolet radiation co-crosslinking with photo-cross-linkable osteogenic growth peptides (OGP). The in situ hydrogel system accelerates the bone regeneration process of osteogenic precursor cells by reinforcing the expression of osteogenic-related genes and increasing the precipitation of calcium in osteoblasts (Qiao et al., 2020) (Figure 4A). The injectable hydrogel precursor converts to solid hydrogel with UV in situ irradiation, which also can reinforce the mechanical properties (e.g., stress-strain, shear force.) of the defective bone, avoid burst release osteogenic drugs, and achieve timed release throughout the bone-healing term. Similar work was reported by Montazerian, H. to enhance the toughness, stretchability, and adhesive properties of GelMA hydrogel. They conjugated the polydopamine to the GelMA to improve the cell migration capacity and enhance bone regeneration (Montazerian et al., 2021). Furthermore, researchers have recently focused on RNA treatment due to its high effectiveness. For example, Gan et al. (2021) utilized the cholesterol-modified non-coding microRNA chol-miR-26a to promote the osteogenic differentiation of human mesenchymal stem cells (hMSCs). Chol-miR-26a was conjugated to an injectable poly(ethylene glycol) (PEG) hydrogel by an ultraviolet (UV)-cleavable ester bond. The injectable PEG hydrogel was formed by a copper-free click reaction between the terminal azide groups of 8-armed PEG and dibenzocyclooctyne-biofunctionalized PEG, while UV-cleavable chol-miR-26a was simultaneously conjugated via a Michael addition reaction. Upon UV irradiation, Gel-c-miR-26a (MLCaged) not only released chol-c-miR-26a selectively but also exhibited a significantly improved efficacy in bone regeneration compared to the hydrogel without UV irradiation and UV-uncleavable MLControl (Gan et al., 2021) (Figure 4B).
Figure 4. Photo-crosslinking in situ hydrogel applied to bone tissue engineering. (A) Schematic representation of GelMA-c-OGP hydrogel construction and its mechanical properties (Qiao et al., 2020). Copyright 2020, Wiley-VCH. (B) Schematic illustration of bone regeneration in vivo at the time of injection of this hydrogel modeled with cholesterol-modified miR-26a at the site of bone malformation (Gan et al., 2021). Copyright 2021, Elsevier. (C) Schematic diagram of the IGF-1bsn functioning as activators for promoting cell proliferation and inhibiting apoptosis (Wu H. et al., 2023). Copyright 2023, Elsevier. (D) Schematic illustration of therapeutic sEVs released from GelMA/nanoclay hydrogel for cartilage regeneration (Hu et al., 2020). Copyright 2020, Taylor & Francis Group.
In addition to traditional bone regeneration, the UV-mediated in situ hydrogel is combined with an autologous bone grafting technique to improve osseointegration and bone fusion. For example, Wu et al. (Wu H. et al., 2023) initiated a new method to mend the gaps between osteochondral plugs after mosaicplasty. This approach employs an injectable photo-sensitive hydrogel (BSN-GelMA), which is fabricated by GelMA loaded with bioactive supramolecular nanofibers to mimic IGF-1(IGF-1bsn) that can bind to IGF-1 receptors and improve the bioavailability, due to their high stability and tissue retention. The result demonstrated that the BSN-GelMA could realize seamless osteochondral integration in the gap region between plugs of osteochondral defects after mosaicplasty because they can prevent cartilage degeneration and promote graft survival. These bio-interactive materials offer a way to bridge the gap and enhance osteochondral integration after mosaicplasty (Figure 4C). Recently, UV-mediated in situ hydrogel became an ideal strategy for improving cartilage regeneration (Huang et al., 2018; Xue et al., 2022a). Articular cartilage has limited self-regenerative capacity, and the therapeutic methods for cartilage defects are still dissatisfactory. To overcome this problem, Hu et al. (2020) fabricated a GelMA/nanoclay hydrogel medium for sustaining the release of extracellular vesicles that come from human umbilical cord mesenchymal stem cell-derived small extracellular vesicles (hUC-MSCs-sEVs) to improve the cartilage regeneration, which exhibited outstanding mechanical property. The results revealed that the GelMA/nanoclay hydrogel containing hUC-MSCs-sEVs stimulated chondrogenesis and healed cartilage damage by improving the expression of collagen II and glycosaminoglycan (GAG) (Figure 4D).
Although photo-sensitive hydrogels possess high sensitivity and are widely applied in research, there are several shortcomings that need to be considered in clinical application. Due to the limited ability of ultraviolet light to penetrate tissue, photoactivation must be directed in situ after gel injection, which is difficult in clinical practice. Moreover, there is a need for additional chemical reactions to graft special functional groups due to the most natural biomaterials do not have triggers for photoactivation. This complicates the preparation of photo-sensitive hydrogels and makes industrial production more difficult. Therefore, the production and preparation of photo-responsive gel precursors with high safety can improve the clinical practice of UV-stimulated in situ hydrogels.
Near-infrared (NIR) is an electromagnetic wave between visible light (VI.S) and mid-infrared light (MIR) and has a wavelength in the range of 780–2,526 nm as defined by the American Association for Testing and Materials Testing (ASTM) (Zhang et al., 2024c). The NIR spectrum belongs to the frequency multiplier and main frequency absorption spectrum of the molecular vibration spectrum due to the non-resonance of the molecular vibration (the molecular vibration is generated when the molecular vibration transitions from the ground state to the high energy level). The NIR possesses a strong penetration ability because of its special physical properties (Wang K. et al., 2024). In addition, NIR, without being strongly absorbed by body fluid, presents better tissue transparency than other wavelengths and is an ideal candidate for designing light-responsive in situ hydrogels (Raza et al., 2019). Thus, many injectable hydrogel systems have been explored to construct an in situ hydrogel in niduses via an NIR light trigger due to its noninvasive nature, high spatial resolution, temporal control, and convenience (Abueva et al., 2020) (Figure 5).
Figure 5. NIR-responsive in situ hydrogel in tissue engineering. (A) In situ gelation in vitro and in vivo of PNAM–MoS2 (Lee et al., 2021). Copyright 2021, Wiley-VCH. (B) The controllable in situ synthesis of a CuS/131I -PEGDA hydrogel (Meng et al., 2018). Copyright 2018, American Chemical Society. (C) Schematic illustration of NIR light-induced angiogenesis in a photoactivatable hydrogel with embedded UCNP-PMAOs (Zheng et al., 2020). Copyright 2021, RSC.
The photothermal effect is the primary mechanism of NIR-responsive in situ hydrogels. Precisely, it refers to the release of vibrational energy (heat) while the nanoparticle (NP) photosensitizer is excited by a specific band of light in the precursor (the conversion of light to heat) and then forms a hydrogel via the tune temperature (Kim and Lee, 2018). The NIR light was applied as an “on/off” trigger to remotely heat and activate thermosensitive in situ hydrogels. Similar systems are used for on-demand drug delivery and synergistic photothermal chemotherapy. For example, Liu et al. (2019) designed an injectable, thermosensitive photothermal-network hydrogel (PNT-gel) through host-guest self-assembly of photothermal conjugated polymers and ɑ-cyclodextrin. The conjugated polymer backbones can directly convert incident light into heat, endowing the PNT-gel with high photothermal conversion efficiency (g = 52.6%) and enhancing photothermal stability. Meanwhile, the mild host-guest assembly enables shear-thinning injectability and the photothermally driven and reversible gel-sol conversion of the hydrogel.
Many nanoparticles with photothermal conversion properties (including nanostructures of Au, Ag, Pt, Pd, graphene oxide, carbon nanotubes, CuS, MoS2, and PDA) have been developed to work as multifunctional nanoplatforms to realize photothermal therapy in tissue engineering (Jiang et al., 2019). Lee et al. (2021) designed a novel type of NIR-triggered in situ gelation system based on the defect-rich 2D MoS2 nano-assemblies and thiol-functionalized thermos-responsive poly (N-isopropyl acrylamide-co-acrylamide-co-2-mercaptoethylacrylamide) (PNAM). In this process, the dynamic polymer–nanomaterial interactions activate under the NIR radiation without a photoinitiator due to the photothermal characteristics of MoS2 and the intrinsic phase transition ability of the PNAM thermos-responsive polymer. Specifically, this phase transition behavior of PNAM stems from dynamic changes in the hydrophilic properties of the lateral radicals. Thus, as the temperature increases, the hydrophobic interaction of MoS2 nanoparticles increases with the PNAM chain. In addition, the MoS2 nanoparticles generate heat under near-infrared irradiation to attract vulcanized PNAM chains to wrap themselves, while the defects of MoS2 nanoparticles react rapidly with the thiol side groups to form covalent bonds (Figure 5A). Consequently, the remotely controlled on-demand release of drugs is achieved via a photothermal-induced gel-sol transition (Wu et al., 2017; Meng et al., 2018; Wang et al., 2020) (Figure 5B).
The photothermal effect of the incorporated photothermal agents not only induced the on-demand drug release through the gel-sol phase transition of thermosensitive hydrogel but also induced proliferative differentiation of cells via hyperthermia (Shen et al., 2021). Here, Zheng et al. (2020) described an NIR strategy for controlling activated cell processes (3D cell diffusion and angiogenesis) by embedding up-conversion nanoparticles (UCNPs) into the hydrogel, which is modified with a photo-activated cell adhesion motif. The UCNPs can convert NIR light (974 nm) into localized UV emission and activate photochemical reactions on demand. This light regulation is spatially controllable and dose-dependent and can be performed at different time points in cell culture without causing significant photodamage to the cells. Human umbilical vein endothelial cells (HUVEC) cells embedded in this hydrogel can form a network of blood vessels at predefined geometries determined by the irradiation pattern (Figure 6C). There are two main mechanisms of in situ hydrogel gel formation induced by NIR. The first is to realize photothermal conversion through a photothermal factor, and the other is to convert near-infrared light into ultraviolet light by UCNPs and then induce in situ gelling. However, the NIR utilization rate of both types of gelatinization is very low.
Figure 6. Schematic illustration of the design of in situ nanocomposite hydrogels with a controlled release of KGN and ultrasonic stimuli ROS production for irregular cartilage repair (Wu S. et al., 2023). Copyright 2023, RSC.
Ultrasound is a potentially valuable trigger for hydrogels because of the mechanical pressure waves that oscillate at high frequencies (≥20 kHz) and produce a range of thermal and nonthermal effects (Wiklund et al., 2012). The thermal effects refer to the transition from acoustic energy to thermal energy, inducing a rise in temperature in tissues (Gao et al., 2005). The nonthermal effects, also named cavitation effects, refer to the tiny gas bubbles generated by ultrasound vibration (Manouras and Vamvakaki, 2017).
Ultrasound can trigger reactions in polymers, which has been reported since the 1930s (Price, 2003). This system enables noninvasive, spatiotemporally controlled modulation and morphological properties using focused ultrasound in the body. For example, Wu S. et al. (2023) designed and prepared a novel ROS-responsive controlled-release in situ drug nanohydrogel for articular cartilage repair (Figure 6). Ultrasonic-cleavable ketothiol (TK)-based nanoliposomes are loaded into cartilage drugs (KGNs), thrombin, and sonics (PpIX). Partial rupture of liposomes under ultrasound stimulation is accompanied by enzymatic reactions of fibrinogen and thrombin to achieve in situ gelation for filling tissue defects. In addition, the controllable release of KGN drugs and the appropriate amount of ROS production were achieved by regulating the ultrasound conditions. More importantly, the appropriate amount of ROS and the continuous release of KGN in the in situ nanocomposite hydrogel microenvironment effectively promoted the differentiation of BMSCs in the direction of cartilage formation by stimulating the Smad5/mTOR signaling pathway, thereby accelerating the repair of articular cartilage defects. Current research also focuses on inducing in situ gelatinization through cascade reactions. The development of ultrasound in situ hydrogels is slow due to the lack of ultrasound responsiveness of natural gel materials. There is still a long distance to clinical application.
Magnetic hydrogels (MHGs) have extensive tissue engineering and biomedical applications and possess the advantages of quick response, biocompatibility, tunable mechanical properties, porosity, and internal morphology (Asadi et al., 2024; Liu et al., 2024). MHGs can be fabricated by blending exogenous additives (e.g., paramagnetic, ferromagnetic) in the polymeric matrix and placing the matrix in a magnetic field (static or oscillating) (Zhou K. et al., 2024; Zhang K. et al., 2024).
The most common and straightforward method for making magnetic composite hydrogels is blending with magnetically responsive nanoparticles. For example, Fe3O4 is one of the most common magnetic nanoparticles (MNPs) mixed with a polymer for the preparation of magnetic hydrogels. The Fe3O4 magnetic nanocomposite gel can be adjusted by applying a magnetic field (Yan et al., 2022). In addition, the type of hydrogel networks and MNPs (the content, size, distribution), as well as the interactions between MNPs and polymer networks, can make a significant impact on the physicochemical properties and magnetic responsiveness of magnetic hydrogels (Thoniyot et al., 2015). Magnetite and maghemite have been prepared and loaded into dextran hydrogel to produce a magneto-sensitive composite hydrogel system by photopolymerization (Brunsen et al., 2012). The in situ synthesis of MNP-based composite hydrogels is more accurate in terms of particle size and hydrogel architecture (Farzaneh et al., 2021). Gao et al. (2014) developed a magnetic hydrogel composed of anion networks of poly (2-acrylamide-2-methylpropane sulfonic acid) (PAMPS) and anisotropic nanocrystals of Fe3O4. The Fe3O4 nanocrystals were prepared in situ in the PAMPS networks, where negatively loaded SO3 facilitated the adsorption and uniform distribution of iron ions in the hydrogel network. They found that the size and shape of Fe3O4 nanocrystals can be adjusted by modifying the crosslinking density of hydrogel without applying stringent experimental conditions (Gao et al., 2014). Although the magnetic response in situ hydrogel has achieved excellent results, it still faces great challenges in the clinical transformation stage due to the complex composition and the need to introduce magnetic response factors. Therefore, simplifying the composition of magnetic response in situ hydrogels and increasing their usability will be conducive to their use in tissue engineering.
Endogenous stimulus-responsive in situ hydrogels with no additional stimulus approaches and easy control have been extensively applied in tissue engineering (e.g., temperature, enzyme, etc.) under physiological and pathologic environment conditions (Wu P. et al., 2024; Gang et al., 2024). In particular, these stimuli-sensitive hydrogels can exhibit significant sol-to-gel transitions after certain stimuli. Hydrogels with appropriate reactivity are synthesized for different practical applications, such as modulation of cargo release and/or replication of dynamic interactions/evolutions in the in situ microenvironment (Yoon et al., 2012).
Amongst several stimuli-responsive in situ hydrogel systems, the thermal stimulation in situ hydrogel is one of the most widely investigated classes because they have a lower critical solution temperature (LCST) and can flexibly modify their physical characteristic (e.g., changes in volume) in response to alters in various temperatures (Ma et al., 2019; Li X. et al., 2021; Wang J. et al., 2021; Nguyen et al., 2021). Thermo-gel behavior combines different thermosensitive mechanisms, such as the precipitation of water-insoluble polymers above LCST and then the aggregation of thermo-gelling (Zhang K. et al., 2021). Precisely, the hydrogel precursor is liquid when kept at temperatures lower than the critical solution temperature and transforms into solid-phase gel at body physiological temperature (Zhang et al., 2019). This smart strategy can form an in situ hydrogel and offer the possibility of injecting those systems and conforming to any irregular defects (El-Husseiny et al., 2022).
Many kinds of thermo-responsive hydrogels have been reported to improve the regeneration of bone tissue. Temperature-responsive hydrogels usually possess distinctive thermoplastic characteristics owing to hydrophobic moieties (e.g., propyl, ethyl, methyl group, etc.) (Sood et al., 2016). For example, poly (N-isopropyl acrylamide) (PNIPAm) gel has an obvious coil–globule transition at 32 °C (LCST); the compound is hydrophilic below this temperature and hydrophobic above it. Thus, these injectable precursors show a solution state at ambient temperature; the gel form is produced in situ at physiologic temperature (Karimi et al., 2016). Many thermo-responsive hydrogels based on PNIPAms have been designed and applied in tissue engineering, including N-isopropyl acrylamide hydrogel crosslinked with di(ethylene glycol) divinyl ether poly (NIPAAm-co-DEGDVE) (Werzer et al., 2019) and polymer poly [(propylene sulfide)-block-(N,N-dimethyl acrylamide)-block-(N-isopropyl acrylamide)] (PPS-b-PDMA-b-PNIPAAM) (Gupta et al., 2014).
Poloxamers are another biodegradable biomaterial that has been widely applied to thermo-responsive in situ hydrogel systems in tissue engineering due to the suitable sol-gel transition temperature (Liu et al., 2020; Zhang T. et al., 2021). For example, Madry et al. (2020) designed an injectable and thermosensitive hydrogel based on poly(ethylene oxide) (PEO)–poly(propylene oxide)(PPO)–PEO poloxamers, capable of controlling the release of a therapeutic recombinant adeno-associated virus (rAAV) vector overexpressing the chondrogenic Sox9 transcription factor in full-thickness chondral defects. This work indicated that the rAAV-FLAG-hsox9/PEO–PPO–PEO hydrogel significantly improves cartilage repair with a collagen fiber orientation similar to the normal cartilage and protects the subchondral bone plate from early bone loss in a minipig model (Madry et al., 2020) (Figure 7A).
Figure 7. Temperature stimulus-responsive in situ hydrogel applied in bone tissue engineering. (A) Graphical representation of the injectable in situ hydrogel experimental strategy in vivo or in vitro (Muscolino et al., 2021). Copyright 2021, Elsevier. (B) Schematic representation of PF127-based copolymer temperature stimulus-responsive in situ hydrogel in chondral regeneration (Madry et al., 2020). Copyright 2020, Wiley-VCH. (C) Schematic diagram of one-time injection of the TePN hydrogel system for long-term osteoarthritis treatment (Seo et al., 2022). Copyright 2022, Elsevier. (D) Preparation of DFO-GMs hydrogel complex for repairing a critical-sized femoral defect (Zeng et al., 2022). Copyright 2022, Elsevier.
Several natural biomaterials (e.g., cellulose and gelatin) and artificial synthetic polymers (e.g., poly (N-isopropyl acrylamide) (PNIPAAm), poloxamer, and polyfluorene 127, etc.) are exploited to fabricate thermosensitive hydrogels which suggest promising applications for bone tissue engineering (Chatterjee et al., 2018). For example, Ren et al. (2015) developed a thermosensitive hydrogel by gelatin grafted with PNIPAAm (Gel–PNIPAAm). These injectable hydrogel precursors exhibit an excellent biocompatible, sol-to-gel transformation property at physiological temperature and an excellent fill-ability for complex and irregular bone defects. The result demonstrated that this gel–PNIPAAm thermosensitive in situ hydrogel can induce bone differentiation and improve bone regeneration compared to the control in a cranial damage model.
As recently reported, several natural polysaccharides (e.g., agarose, amylose, carrageenan, etc.) possess thermo-gelling properties, but they failed to form gels at body temperature (Zhang B. et al., 2024; Elizalde-Cárdenas et al., 2024; Riviello et al., 2024). Fortunately, when xyloglucan is partially degalactosylated (Deg-XG), it will achieve sol-to-gel at a physiological temperature of concentration over 2 wt% (Chandramouli et al., 2012). For example, E.M and colleagues presented a simple strategy based on the synergic combination of forming in situ hydrogels and spheroids of adipose stem cells (SASCs) with great potential for minimally invasive regenerative interventions aimed to treat bone and cartilage defects. They loaded SASCs in aqueous dispersions of partially Deg-XG and either a chondroinductive or an osteoinductive medium to induce bone and cartilage regeneration. The dispersions rapidly set into hydrogels when the temperature was brought to 37°C. The physicochemical and mechanical properties of the hydrogels are controlled by polymer concentration (Muscolino et al., 2021) (Figure 7B). Similar results were reported by Dispenza et al. (2017). They designed the same injectable thermosensitive hydrogel (Deg-XG loading with the growth factor FGF-18) to promote cartilage reconstruction at gelation at 37°C.
Thermosensitive hydrogels were also extensively applied in the effective treatment of osteoarthritis by local injection to replace oral administration. For example, Seo et al. (2022) designed an injectable polymeric nanoparticle hydrogel system with poly(organophosphazenes), which is loaded with triamcinolone acetate (TCA, nonsteroidal anti-inflammatory drugs) for achieving a long-term anti-inflammatory effect and treat osteoarthritis. The TePN precursor turned into a solid hydrogel after intra-articular injection, and the formed 3D TePN hydrogel released TePNs for months in an in vivo microenvironment. Long-term release of TCA treats OA by inhibiting matrix proteinase (MMP) expressions in the cartilages via decreased pro-inflammatory and increased anti-inflammatory cytokine expressions (Figure 7C).
Insufficient vascularization is still a great challenge in the regeneration of critical-sized bone tissue defects (Takaoka et al., 2024; Yuan et al., 2024). The formation of the H-type vessel plays a key role in the progress. To address this problem, Zeng et al. (2022) designed an injectable thermosensitive demethoxyamine (DFO) gelatin microsphere (GMs) hydrogel complex to stimulate the production of functional H-type blood vessels. The results showed that the DFO-GMs thermosensitive hydrogel complex could stimulate the production of functional H-type blood vessels and effectively promote the proliferation, formation, and migration of HUVECs in vitro. Moreover, this thermosensitive hydrogel can expand the distribution range of H-type blood vessels in the defect area and increase the native bone tissue, which was confirmed via fluorescence immunostaining and radioactivity examination in vivo (Figure 7D).
The thermo-responsive hydrogels possess great potential to adjust the threshold response levels and allow on-demand triggers due to excellent biocompatibility, mild trigger conditions, no toxicity, etc. More studies should examine the integration of thermo-responsive hydrogels with multi-gradient temperature triggers. In addition, thermo-responsive hydrogel systems are generally regarded as crucial triggers in designing advanced external stimuli (e.g., NIR, magnetic, US, etc.) for smart, responsive platforms.
Biocatalytic reactions of enzyme complexes are especially important in naturally occurring multicellular organisms (Bleier et al., 2015). Furthermore, the enzymes catalyze the recognition of the 3D substrate structures that are adapted to the enzymes for binding. The “substrate specificity” closely regulates enzymatic activity without adverse side effects. In recent years, the enzyme medium stimulus-responsive in situ hydrogels have played an increasing role in tissue repair due to the mild reaction mechanism. Most enzymes involved in crosslinking also catalyze natural reactions in our body (Jin et al., 2011) (Figure 8). Furthermore, the enzymatic reactions are catalyzed with a neutral-pH microenvironment in an aqueous medium at moderate temperatures, which means that they can also be utilized to develop in situ hydrogels. Smart enzymatic reaction systems can be designed not only to create native extracellular matrices (ECM) but also to construct degradable biomaterials (Hubbell, 2003). These events capture, in substance, one of the most significant biological features of the ECM, which is remodeling. In addition to degradability, it is essential to adapt the gelation rate to applications such as drug administration and tissue regeneration strategies. A controlled gelation rate is essential to prevent diffusion of the precursors, ensure localized drug delivery, obtain a suitable cell distribution, and properly integrate the gel with the surrounding tissues (mainly for irregular-shape filling applications) (Teixeira et al., 2012). The enzyme stimulus-responsive in situ hydrogel has raised much attention in bone tissue engineering (e.g., OA, bone defects, fracture, etc.) (Zhou S. et al., 2024; Vidal et al., 2024). In this contribution, the enzymatically crosslinked gels are reviewed in the context of regenerative strategy applications.
Figure 8. Various enzyme stimulus-responsive in situ hydrogel schemes. Enzyme response mechanisms of horseradish peroxidase (A), transglutaminase (B), and thrombin (C).
Peroxidases are a broad family of enzymes that usually catalyze the following reaction: ROOR′ + electron donor (2e−) + 2H+—ROH + R′OH. Horseradish peroxidase (HRP) is the most common peroxide used in the formation of hydrogel (Krainer and Glieder, 2015; Khanmohammadi et al., 2018). HRP is widely distributed in the plant kingdom. It is a colorless enzyme protein comprising brown iron porphyrin combined with glycoprotein. In addition, HRP is combined with hydrogen peroxide (H2O2) to obtain an [HRP-H2O2] complex that can oxidize a variety of hydrogel donor substrates (Ryan et al., 2006).
In crosslinking, the basic physical properties (mechanisms) and biologically inducible properties mainly rely on the precursor concentration. Saghati and colleagues (Saghati et al., 2021) detect the effect of several parameters, including H2O2 and HRP concentrations, on the hydrogel properties of Alg-based hydrogels via an enzymatic cross-linked procedure. They found that the physical properties of 1.2% (v/w) Alg-Ph, 5 U HRP, and 100 mM H2O2 at the ratio of 1:0.54:0.54 are more appropriate for the cartilage-like structure, which has a great potential to induce cartilage regeneration. Several studies have been reported to describe enzymatic crosslinking in situ hydrogels (Arora et al., 2017; Qi et al., 2018; Behrendt et al., 2020; Li Q. et al., 2021). For example, Arora et al. (2017) developed a novel enzymatically cross-linked injectable hydrogel composed of carboxymethyl cellulose (CMC), sulfated carboxymethyl cellulose (sCMC), and gelatin for the delivery of infrapatellar fat pad-derived MSCs and articular chondrocytes to a cartilage defect site while enabling TGF-β1-mediated chondrogenesis (Figure 9A). Similarly, Zhang et al. (2020) fabricated an in situ hydrogel consisting of collagen-type I-tyramine (Col-TA) and hyaluronic acid-tyramine (HA-TA) that also loaded the BMSC for cartilage regeneration. Further, the proliferation and differentiation of BMSCs within the Col-HA hydrogel were evaluated, and the ability of in vivo cartilage repair was also examined in the presence of the TGF-β1. These results illustrated that this hydrogel could provide an excellent microenvironment for BMSC growth and cartilage differentiation in vitro and in vivo (Zhang et al., 2020) (Figure 9B).
Figure 9. Peroxidase enzymatically crosslinking injectable in situ hydrogels. (A) Overall scheme of enzymatically cross-linked injectable hydrogels for delivery of cells and TGF-β1 for cartilage tissue engineering (Arora et al., 2017). Copyright 2017, Europe PMC. (B) Preparation of the BMSC-laden injectable Col-HA hydrogel for cartilage regeneration (Zhang et al., 2020). Copyright 2020, RSC.
Transglutaminase, a type of biological glue, is found in plasma, tissues, keratinocytes, and epidermal cells in the human body. Transglutaminase catalyzes the development of an isopeptide binding of the amide group of a glutamine residue to the primary amine group of a lysine residue (Greenberg et al., 1991). The amide portion of the glutamine residue attacks the enzyme as the donor acrylic substrate (an intermediary thioester form), resulting in an acrylic-enzyme form. In the presence of an acyl-acceptor substrate, such as a lysine residue, the imidazole group in the active site was deprotonated by the amine group, while the acyl-enzyme and lysine residues form a tetrahedral group (Lai et al., 2017; Savoca et al., 2018). Finally, this group decomposes into an isopeptide group to form a reticulated system78. In addition, transglutaminase was applied to hydrogel as an enzymatic crosslinking agent to achieve in situ gelling, due to the safe and highly effective catalytic effect. For example, Chen X. et al. (2021) manufactured a novel gelatin-based hydrogel that was crosslinked by transglutaminase (TG) and tannic acid (TA). This hydrogel, when incorporated into the MPs-His6-T4L-BMP2, demonstrated excellent in situ injectability, heat sensitivity, adhesion, and mechanical properties. The efficient charging mode resulted in a controllable and sustainable release of His6-T4L-BMP2 to improve bone regeneration in a critical bone defect (Figure 10). The injection and tunable hyaluronan-transglutaminase hydrogel were obtained by adding coagulation factor XIII. The specific amino acid residues from substrate FXIIIa contribute to reducing toxicity during crosslinking (Anjum et al., 2016).
Figure 10. Fabrication of hydrogel Gel/TG/TA-MPs-His6-T4L-BMP2 (Hydrogel/MPs-His6-T4L-BMP2), which was applied to bone defects (Chen X. et al., 2021). Copyright 2021, Elsevier.
Thrombin, a serum protease found in the bloodstream, is specific to arginine-glycine bonds in fibrinogenic peptides. Thus, thrombin and fibrin are generally applied to tissue engineering as a field administration system (Noori et al., 2017). Injectable fibrin-based hydrogels have been extensively investigated for applications in bone tissue regeneration due to their minimally invasive implant procedure and shortened healing time, which reduces patient discomfort and complications and decreases healthcare costs (Kim et al., 2014). Furthermore, they can easily bridge faults of irregular shapes and provide the necessary mechanical support (van der Stok et al., 2015). During the final step of the biological polymerization process, soluble fibrinogen is cleaved by thrombin to produce insoluble fibrin, which binds to form fibers and a network. Meanwhile, a large number of red blood cells, platelets, and other entities are encapsulated in the network into a firm hydrogel system (Hickman et al., 2018). The in situ injection strategy offers the benefits of simple surgical procedures and the prevention of patient discomfort. Non-surgical strategies involving cellular or in situ hydrogels have been reported (Lyu et al., 2020). For example, Chen et al. (2022) developed a double-function injectable fibrin hydrogel with semi-synthetic chitosan sulfate nanoparticles that dramatically improves the level of recombinant human bone morphogenetic protein-2 (BMP-2) to induce osteogenesis and reduces the inflammatory response at the damage site. This fibrin-based hydrogel system effectively manages the release of BMP-2 and induces osteogenetic differentiation. Furthermore, the hydrogel system regulates the polarization of macrophages from the M1 to M2 stage with a significant decrease in inflammatory cytokines (Figure 11A). However, most injectables were composed of biomaterials that have not yet been clinically approved. In addition, they were also subjected to low mechanical stresses to reduce the load-bearing stresses applied to the meniscus. Therefore, mechanically stable, highly bioactive biomaterials must be developed for translational research using biomaterials approved by the FDA. For example, An et al. (2021) developed a clinically applicable and injectable semi-interpenetrated network (semi-IPN) hydrogel system based on fibrin, reinforced with F127 and polymethyl methacrylate (PMMA) to improve the intrinsic weak mechanical properties. Through the dual-syringe device system, the hydrogel could form a gel state within approximately 50 s, and the increment of compressive modulus of fibrin hydrogels was achieved by adding F127 from 3.0% (72.0 ± 4.3 kPa) to 10.0% (156.0 ± 9.8 kPa) (Figure 11B). Similar work has been reported by Kim et al. (2021), who fabricated a semi-IPN hydrogel system consisting of fibrin and polyethylene oxide (PEO) to improve the treating a segmental defect of the meniscus in a rabbit meniscal defect model (Figure 11C).
Figure 11. Fibrin-thrombin-based in situ hydrogels were applied in bone tissue disease. (A) Schematic illustration of rhBMP-2@SCS NPs to induce therapeutic osteogenesis (Chen et al., 2022). Copyright 2022, Elsevier. (B) Scheme of the gelation process of Fb/F127/PMMA hydrogel through a dual-syringe device while filling the meniscal defect region (An et al., 2021). Copyright 2021, SAGE Publications Ltd. (C) The schematic representation describes the overall procedure during the injection. The fibrinogen and thrombin (Tb) solutions were injected, and the semi-interpenetrated polymer network was subsequently formed (Kim et al., 2021). Copyright 2021, American Orthopaedic Society for Sports Medicine.
Glucose oxidase (GOD) is an important industrial enzyme in the food industry, textile bleaching and dyeing, biofuels, glucose biosensors, and other emerging fields. It is widely distributed in animals, plants, and microorganisms. Importantly, GOD can specifically catalyze the β-D-glucose to form gluconic acid and hydrogen peroxide under aerobic conditions. It offers the possibility of inducing in situ hydrogels by the cascade reaction from oxidation products. However, it has been applied to a special pathological model (diabetes) to achieve in situ hydrogel because it requires much glucose in this in vivo process. Thus, several works focus on GOD to induce an in situ hydrogel to treat wounds and bone tissue damage in patients with diabetes (Figures 12A,B) (Zhao et al., 2017; Chen Y. et al., 2021). For example, Zhang Q. et al. (2021) proposed a cascading enzyme polymerization process triggered by tissue fluids catalyzed by glucose oxide and ferrous glycinate, which improves the ultra-fast gelification rate of acryloylate and acrylamide chondroitin sulfates. The highly efficient production of carbon radicals and macromolecules helps the gel to rapidly polymerize, promoting soft tissue growth in bone defects. In addition, this copolymer hydrogel demonstrated potential for cartilage regeneration in vivo and in vitro. As a first example of using artificial enzyme complexes for in situ polymerization, this work provides a biomimetic approach to the design of force-adjustable hydrogels for bio-implantation and bio-printing applications (Figure 12C).
Figure 12. Glucose oxidase-based in situ hydrogels were applied in bone tissue engineering. (A) Scheme of pH and glucose dual-responsive injectable hydrogels with insulin and fibroblasts as bioactive dressings for diabetic wound healing (Zhao et al., 2017). Copyright 2017, American Chemical Society. (B) Schematic illustrations of gelation mechanisms by O2 oxidation, direct addition of H2O2 solution, and the indirect H2O2 supplement method via GOD-catalyzed glucose oxidation and glucose-triggered in situ forming keratin hydrogel as a drug depot for the treatment of diabetic wounds (Chen Y. et al., 2021). Copyright 2021, Elsevier. (C) Fabrication of the CS-DMAA hydrogel. The molecular structures of the acryloylated-CS and the poly (DMAA) are given. This hydrogel is used as a tissue filler via in situ injection and glucose-responsive hydrogenation (Zhang Q. et al., 2021). Copyright 2021, Wiley-VCH.
An enzyme is an active substance with high catalytic efficiency and specificity. Because of this, enzyme-catalyzed in situ hydrogels have a singular nature. Human enzyme catalysis is affected by the concentration of enzymes in the body. In addition, it is easy to lose enzyme activity when specific enzymes are introduced in vitro due to the special storage conditions of enzymes. These shortcomings limit the clinical application of enzyme-catalyzed in situ gels. In the future, vigorously developing synthetic nanozymes to replace natural enzymes may become a novel strategy to promote the development of enzyme-catalyzed in situ hydrogels.
Compared with physical crosslinking, the in situ hydrogels produced by chemical crosslinking possess higher stability in the physiological state and showed excellent mechanical properties, gel stiffness, and slower degradation rates due to the covalent linking of the interpolymer chain (Chai et al., 2024). In addition, they exhibit flexible biodegradation and mechanical properties by adjusting hydrogel precursors and providing longer-lasting spatial support for slow regeneration processes (Pranantyo et al., 2024). Usually, those chemical reactions promote covalent linkage in hydrogels through chemical crosslinking, such as click chemistry (e.g., Diels–Alder reaction, Michael addition reaction, or thiol–Michael addition reaction). In the assembly of hydrophilic polymers, those deterministic groups, such as COOH-, OH-, and NH2-, are utilized to construct a hydrogel system via a covalent bond between amine-carboxylate and a Schiff alkali or isocyanate-OH/NH2 (Liu H. et al., 2023; Wang X. et al., 2024; Qu et al., 2024).
Recently, the development of click chemistry has presented a possibility to further enhance the specificity of in situ hydrogel systems. Click reactions are fast, spontaneous, versatile, and highly selective chemical conjugation reactions that can be generated under mild reaction conditions when two molecular substances or components are mixed or reacted together (Albada et al., 2021). Examples include strain-promoted azide-alkyne cycloaddition (SPAAC) (Liu et al., 2021) and inverse electron-demand Diels–Alder reactions, etc. (Vu et al., 2022). There are no potentially toxic catalysts in the progress of hydrogel crosslinking. Thus, click chemistry has expanded the research fields of tissue engineering and regenerative medicine (Jewett and Bertozzi, 2010).
Due to the mild, safe, and effective reaction conditions, click chemistry offers the possibility of applying artificial chemical reactions in vivo. The wide application of click-through responses in vivo to increase the link between nanoparticles and target cells for drug administration has paved a new path in bone tissue engineering (Vu et al., 2022). For example, to obtain a quick gelling, Hermann et al. (2014) prepared a multi-arm PEG polymer modified by azide (a linear crosshair PEG-DBCO) via a SPAAC reaction between the azides and the BOD to apply in bone regeneration. Both polymers could be crosslinked in less than 30 s at ambient temperature and form a stable hydrogel structure. The hydrogel was developed to inhibit craniosynostosis via the release of the rmGremlin1 BMP inhibitor. After injection into the calvarial defect in a mouse model, this rmGremlin1 hydrogel successfully delayed synostosis in micro-CT images, demonstrating the potential of the SPAAC-based hydrogel. Similar work has been reported by Liu et al. (2021), who reported a new oligomeric polyhedral oligomeric SPAAC system of organic-inorganic nanohybrids (click-ON) that can be crosslinked without toxic initiators or catalysts. Click-ON scaffolding can also support high adhesion, proliferation, and osteogenesis of stem cells. In vivo evaluation results revealed exceptional bone formation with minimum cytotoxicity via a rat head malformation model. A high expression of alkaline osteogenic phosphatase and vascular marker CD31 was found at the defect site, which indicates an excellent regeneration capability for in vivo osteogenesis and vascularization (Figure 13A).
Figure 13. Chemical reaction crosslinking stimulus-responsive in situ hydrogel. (A) Oligomeric polyhedral oligomeric alkyne-azide cycloaddition (SPAAC) system (Liu et al., 2021). Copyright 2021, Elsevier. (B) Schematic illustration of the in situ hydrogel formed by a Diels–Alder click-crosslinking reaction. (Park et al., 2020). Copyright 2020, Elsevier. (C) Thiol-Michael addition reaction (Gilchrist et al., 2021). Copyright 2021, Elsevier. (D) Schematic representation of the formation of injectable hydrogels chemically cross-linked by a Schiff’s base reaction between aqueous solutions of GC and poly(EO-co-Gly)-CHO (Cao et al., 2015). Copyright 2015, RSC.
In addition, countless biomaterials (e.g., collagen, HA, alginate, dextran, chitosan, gelatin, silk, etc.) are modified by chemical bonds to achieve in situ click chemical crosslinking in physical conditions (Lao et al., 2023; Morrison and Gramlich, 2023; Rizwan et al., 2023; Tournier et al., 2023). For example, to prolong the degradation time of HA in vivo, Park et al. (2020). exploited tetrazine-modified HA and trans-cyclooctene-modified HA monomer to prepare an injectable in situ hydrogel system by Diels–Alder click-crosslinking reaction, which loaded a BMP2 mimetic peptide for improving bone tissue regeneration. After the in situ construction of hydrogel in vivo, this hydrogel system provided an excellent mimic ECM microenvironment for the human dental pulp stem cells (hDPSCs). The bone morphogenetic protein-2 (BMP-2) mimetic peptide is similar to BMP-2, which possesses an excellent ability to induce the osteogenic differentiation of hDPSCs. This HA-based in situ hydrogel quickly achieved sol-gel and formed a scaffold in vivo, which retained the BMP-2 for over a month. Thus, the HA-based in situ hydrogel is advantageous in inducing osteogenic differentiation of loaded hDPSCs (Figure 13B).
Furthermore, smart hydrogel systems, such as those triggered by UV (Sun S. et al., 2018; Jansen et al., 2018), NIR (Anugrah et al., 2019), and enzymes (Nguyen et al., 2019; Criado-Gonzalez et al., 2020), have been developed to induce the occurrence of click chemistry. For example, Ding et al. (2021) constructed a pH-responsive UV-cross-linkable chitosan hydrogel for actively modulating drug release behavior. The C6-OH selectively modified chitosan via a protection/deprotection strategy for the amino groups. In addition, the allyl groups on the C6 site and the amino groups on the C2 site endowed chitosan with UV crosslinking capability and pH responsiveness, respectively. A rapid gelling by UV crosslinking (30 s) with low-dose UV irradiation (4 mW/cm2) by thiol-ene was demonstrated for in situ in vivo microgels and hydrogels.
The Michael addition reactions have been broadly applied in organic synthesis, such as material applications, surface modifications, polymer modifications, and polymer synthesis (Mather et al., 2006; Nair et al., 2014). Herein, we focus on a specific Michael addition reaction: the thiol-Michael addition reaction, which occurs between the thiol and vinyl groups and can be initiated by various catalysts, such as bases, metals, organometallics, and nucleophiles (Xiao et al., 2022). Among those catalysts, bases and nucleophiles are the most effective, with the least propensity to secondary reactions. In the traditional method of a thiol-Michael addition catalyzed by a base, the base extracts a thiol proton to produce a thiolate anion, which subsequently undergoes the addition of a Michael thiol. In the nucleophilic-mediated pathway, the nucleophile reacts with the electron-deficient double bond to produce an intermediate carbanion, which, in turn, deprotonates the thiol to produce a thiolate anion. The versatility provided by the weak sulfuric-hydrogen bond enables the thiol-Michael addition reaction to be initiated using a wide range of precursor materials.
The thiol-Michael addition reaction can be carried out under physiological conditions, which results in irreversible reactions in the bioengineering toolkit to fabricate a 3D hydrogel scaffold (Yu and Chau, 2012). For example, Gilchrist et al. (2021) described a functional gelatin hydrogel with maleimide, which can be crosslinked by a thiol-maleimide addition reaction for encapsulation of susceptible stem cell populations. This hydrogel system has tunable mechanics to match the bone marrow microenvironment for culturing hematopoietic stem cells in vivo without generating free radicals (Figure 13C). Liu et al. (2015) created a novel thiol-Michael addition reaction for in situ hydrogels that crosslinks dextran derived from glycidyl methacrylate and dithiothreitol. The encapsulation capacity of 3D cells is demonstrated by encapsulation of rat mesenchymal BSCs and NIH/3T3 fibroblasts in in situ hydrogels. The encapsulated cells maintain high cell viability.
Schiff base reactions are extensively applied to synthesize in situ hydrogels for bone tissue regeneration applications due to the mild reaction conditions and high reaction rate (Amirian et al., 2021). The Schiff reaction takes place between the amino (R-NH2) or hydrazide (R-NHNH2) and aldehyde (R-CHO) functional groups of imine or acrylic hydrazone. It has grown into one of the most popular strategies for developing biocompatible hydrogels in tissue engineering (Wang B. et al., 2021; Hu et al., 2021). Recently, various biomaterials have been explored for utilization in Schiff base in situ hydrogels (Lee, 2018; Hernández-González et al., 2021). Chitosan is an excellent biomaterial for the preparation of in situ hydrogel systems based on Schiff reactions because of the abundance of amino groups on its spinal column (Li Z. et al., 2021). For example, Cheng et al. (2014) reported in situ chitosan polysaccharide hydrogel for both cellular and protein administration. The chitosan polysaccharide is crosslinked via an imine bond resulting from the Schiff base reaction between the amino functionalities of chitosan and the aldehyde groups of the dextran aldehyde precursors. This approach eliminated the potential cytotoxicity and improved the biocompatibility of polymers without additional crosslinking agents. In addition, this work demonstrated the versatility of the gel in terms of manufacturing and the ability to change the mechanical properties by adjusting the extent of crosslinking. Cao et al. (2015) successfully explored a functionalized multi-benzaldehyde analog PEG, poly (ethylene-co-glycidol ether)-CHO (poly (EO-co-Gly)-CHO), and glycol chitosan to develop an in situ hydrogel system for cartilage tissue repair. This multi-functionalized hydrogel system has been chemically crosslinked by a basic Schiff reaction between the chitosan glycol amino groups and polyaldehydes (EO-co-Gly)-CHO groups under physiological conditions (Figure 13D). The results indicated that the in situ hydrogel system not only possesses excellent adjustable physical properties (e.g., water uptake, degradation, mechanical properties, and network morphology) but also has the excellent ability to induce chondrogenesis. Furthermore, other in situ hydrogels based on biomaterials coupled with the crosslinking of the Schiff base have been extensively studied in tissue engineering (Antony et al., 2019; Chen J. et al., 2021).
Chemical reaction crosslinking stimulus-responsive in situ hydrogels were formed by special functional groups between molecules in precise crosslinking and stable. However, the functional groups or reaction products of these chemical grafts can potentially be toxic. This will be detrimental to the application and registration of gel products. Therefore, it is necessary to improve and optimize the safe chemical grafting preparation process in order to promote the industrialization development of the chemical reaction crosslinking stimulus-responsive in situ hydrogels.
Metal ions are an indispensable microelement in regulating whole-body homeostasis (Zhang et al., 2024b). They can be elaborately designed by linking with polymer chains to transform in situ functional hydrogels to improve bone tissue regeneration (Zhang P. et al., 2024). Ions can recognize biomaterial monomers, promote crosslinking between molecules, and enhance the stability of the structure. Therefore, the ion-triggered biomaterials that form in situ hydrogels have received extensive research attention (Ramírez et al., 2024). Alginate, a naturally derived polysaccharide, has been regarded as an ideal hydrogel biomaterial for bone tissue engineering because of its biocompatibility, tunable mechanism properties, low immunogenicity, and ease of gelation by metal ions (Li et al., 2024). It is generally believed that this crosslinking mechanism is the interaction of two carboxyl groups on the adjacent polymer chain with divalent cations to form an ion bridge or chelate with ions via the hydroxyl and carboxyl groups on polymer chains. Thus, alginate-based hydrogel systems can be flexibly manufactured to achieve metal ions response (e.g., Ca2+, Si2+, etc.) (Chen et al., 2024; Li et al., 2024; Zhong et al., 2024). Usually, alginate-based hydrogels are prepared by contacting alginate precursors with CaCl2 aqueous solutions. However, the gelling rate is too fast and difficult to control when using CaCl2 as a crosslinker. Thus, various crosslinking retardation agents have been applied to tune the gelation rate to form an injectable in situ alginate-based hydrogel (Nandi et al., 2008). For instance, Jung et al. (2018) prepared in situ gel alginate (ALG)/HA hydrogel system with a controlled gelatin rate by blending CaSO4 and Na2HPO4 as crosslinking retardation agents. The ALG/HA hydrogels possess a controlled gelation rate in vivo. It is possible to stabilize and control the release of bioactive molecules (BMP-2 immobilized in the hydrogel for 5 weeks) for bone regeneration. The results of cell culture and animal studies on micropigs revealed that osteogenesis differentiation of hBMSCs improved with the increase of HA components in BMP-2-stimulated ALG hydrogels. In addition, hBMSCs/BMP-2 loaded in ALG/HA hydrogels can extensively enhance bone regeneration by synergistic action compared to single ALG/HA hydrogels.
Metal-chelating hydrogels are physical hydrogels that are completely crosslinked by complexes between ligand-modified polymers and metal ions. The mechanical properties of these hydrogels depend on the density and kinetics of the metal coordination interaction (Raymond et al., 2015). For example, HA and the catechol compound gallic acid (GA) have iron coordination activity. The conjugates of HA and GA (HA-GA) immediately form the hydrogel in the presence of the Fe3+ ion. After subcutaneous injection into mice, HA-GA and Fe3+ ions form an in situ hydrogel and remain at the injection site for at least 8 days (Ko et al., 2019). The ions in the ion crosslinking can replenish the trace elements of the human body. However, the amount of ions in the gel is much higher than the normal level of ions in the human body. Therefore, the safety of the tissues surrounding the gel must be thoroughly evaluated and studied.
Stimuli-responsive in situ hydrogel delivery platforms have demonstrated great potential in repairing various kinds of bone damage (e.g., accidents, cancer, or age). Recent years have witnessed rapid progress in the development of in situ hydrogel systems based on different functional biomaterials and stimulus-response approaches to treat bone-related diseases. This review summarized the various strategies of the smart responsive in situ hydrogel systems in bone tissue engineering. The two strategies of exogenous and endogenous stimulus response are detailed and introduced. The exogenous stimulus-responsive in situ hydrogel systems usually require exogenous trigger apparatus, such as ultraviolet light, near-infrared light, and ultrasonic apparatus, to form gels. In this process, those nature or synthesis biomaterials are usually modified by functional groups (e.g., unsaturated bonds) or combined with functional nanoparticles (e.g., MoS2, Cu2O, F3O4, etc.) to match special trigger approaches. However, the endogenous stimulus-responsive in situ hydrogel systems, which are free of exogenous trigger apparatuses, relay on the physical microenvironment (e.g., temperature, enzyme) or chemical reactions (click chemistry, thiol-Michael addition reaction, Schiff base reaction) to form gels in vivo. Moreover, we analyzed the research status and advantages and disadvantages of different kinds of stimulus-responsive in situ hydrogels. It appears that temperature-sensitive hydrogels will be most promising for clinical application. The specific reasons are: 1) Temperature-sensitive hydrogels can form in situ hydrogels at human body temperature without the need for manipulation and specific functional modifications. 2) The simple composition of temperature-sensitive hydrogels both reduces cytotoxicity and opens the possibility for industrial production. 3) Temperature-sensitive hydrogels only need to be injected once to achieve gelatinization therapy in vivo, which can greatly improve patient compliance and is more suitable for clinical applications.
Numerous studies have confirmed that smart responsive in situ hydrogel technology plays a significant role in bone tissue engineering, which provides a theoretical foundation for future clinical applications. Although excellent regeneration effects have been achieved, research is still in its infancy. Clinically, there are many limits to the development of a reliable, efficient, smart, responsive in situ hydrogel system. First, the systems are subjected to advanced trigger conditions, so it is necessary to develop novel stimulation methods. Second, the rapid rate of degradation of the hydrogel platform must be adjusted to be consistent with the rate of regeneration of the bone defects. In addition, the poor mechanical stability of the hydrogel system fails to match bone tissue, including rapid degradation and burst release, poor integration with native cells, low mechanical stability, and immunogenicity.
Despite the significant challenges, the development of smart responsive in situ hydrogel-based bone regeneration is extremely promising for the future treatment of bone diseases and damage. With a better understanding of hydrogels, bone abnormalities, ECM, and how they interact, hydrogels will undoubtedly become a powerful tool for the clinical treatment of bone abnormalities.
SW: investigation, writing–original draft, and writing–review and editing. TG: investigation and writing–review and editing. JC: supervision and writing–review and editing. XC: funding acquisition, supervision, validation, writing–review and editing, and writing–original draft. WC: funding acquisition, project administration, supervision, and writing–review and editing.
The author(s) declare that financial support was received for the research, authorship, and/or publication of this article. This work was supported by the National Natural Science Foundation of China (NSFC, U22A20588), the Sanya Science and Technology Project (2022KJCX57), the Qingdao National Laboratory for Marine Science and Technology (12-04), and the Wenzhou Association for Science and Technology Science Service and Technology Innovation Project (nlts045).
Author SW was employed by Hangzhou Singclean Medical Products Co., Ltd.
The remaining authors declare that the research was conducted in the absence of any commercial or financial relationships that could be construed as a potential conflict of interest.
All claims expressed in this article are solely those of the authors and do not necessarily represent those of their affiliated organizations, or those of the publisher, the editors, and the reviewers. Any product that may be evaluated in this article, or claim that may be made by its manufacturer, is not guaranteed or endorsed by the publisher.
Abdalla, A. A., and Pendegrass, C. J. (2023). Biological approaches to the repair and regeneration of the rotator cuff tendon-bone enthesis: a literature review. Biomater. Transl. 4 (2), 85–103. doi:10.12336/biomatertransl.2023.02.004
Abueva, C. D. G., Chung, P.-S., Ryu, H.-S., Park, S.-Y., and Woo, S. H. (2020). Photoresponsive hydrogels as drug delivery systems. Med. Lasers 9 (1), 6–11. doi:10.25289/ML.2020.9.1.6
Albada, B., Keijzer, J. F., Zuilhof, H., and van Delft, F. (2021). Oxidation-induced "One-Pot" click chemistry. Chem. Rev. 121 (12), 7032–7058. doi:10.1021/acs.chemrev.0c01180
Ali, I., Rizwan, A., Vu, T. T., Jo, S. H., Oh, C. W., Kim, Y. H., et al. (2024). NIR-responsive carboxymethyl-cellulose hydrogels containing thioketal-linkages for on-demand drug delivery system. Int. J. Biol. Macromol. 260 (Pt 2), 129549. doi:10.1016/j.ijbiomac.2024.129549
Amirian, J., Zeng, Y., Shekh, M. I., Sharma, G., Stadler, F. J., Song, J., et al. (2021). In-situ crosslinked hydrogel based on amidated pectin/oxidized chitosan as potential wound dressing for skin repairing. Carbohydr. Polym. 251, 117005. doi:10.1016/j.carbpol.2020.117005
An, Y. H., Kim, J. A., Yim, H. G., Han, W. J., Park, Y. B., Jin Park, H., et al. (2021). Meniscus regeneration with injectable Pluronic/PMMA-reinforced fibrin hydrogels in a rabbit segmental meniscectomy model. J. Tissue Eng. 12, 204173142110501. doi:10.1177/20417314211050141
Anjum, F., Lienemann, P. S., Metzger, S., Biernaskie, J., Kallos, M. S., and Ehrbar, M. (2016). Enzyme responsive GAG-based natural-synthetic hybrid hydrogel for tunable growth factor delivery and stem cell differentiation. Biomaterials 87, 104–117. doi:10.1016/j.biomaterials.2016.01.050
Antony, R., Arun, T., and Manickam, S. T. D. (2019). A review on applications of chitosan-based Schiff bases. Int. J. Biol. Macromol. 129, 615–633. doi:10.1016/j.ijbiomac.2019.02.047
Anugrah, D. S. B., Ramesh, K., Kim, M., Hyun, K., and Lim, K. T. (2019). Near-infrared light-responsive alginate hydrogels based on diselenide-containing cross-linkage for on demand degradation and drug release. Carbohydr. Polym. 223, 115070. doi:10.1016/j.carbpol.2019.115070
Arora, A., Mahajan, A., and Katti, D. S. (2017). TGF-β1 presenting enzymatically cross-linked injectable hydrogels for improved chondrogenesis. Colloids Surf. B Biointerfaces 159, 838–848. doi:10.1016/j.colsurfb.2017.08.035
Asadi, K., Samiraninezhad, N., Akbarizadeh, A. R., Amini, A., and Gholami, A. (2024). Stimuli-responsive hydrogel based on natural polymers for breast cancer. Front. Chem. 12, 1325204. doi:10.3389/fchem.2024.1325204
Balakrishnan, B., and Banerjee, R. (2011). Biopolymer-based hydrogels for cartilage tissue engineering. Chem. Rev. 111 (8), 4453–4474. doi:10.1021/cr100123h
Behrendt, P., Ladner, Y., Stoddart, M. J., Lippross, S., Alini, M., Eglin, D., et al. (2020). Articular joint-simulating mechanical load activates endogenous TGF-β in a highly cellularized bioadhesive hydrogel for cartilage repair. Am. J. Sports Med. 48 (1), 210–221. doi:10.1177/0363546519887909
Bleier, L., Wittig, I., Heide, H., Steger, M., Brandt, U., and Dröse, S. (2015). Generator-specific targets of mitochondrial reactive oxygen species. Free Radic. Biol. Med. 78, 1–10. doi:10.1016/j.freeradbiomed.2014.10.511
Brunsen, A., Utech, S., Maskos, M., Knoll, W., and Jonas, U. (2012). Magnetic composite thin films of FexOy nanoparticles and photocrosslinked dextran hydrogels. J. Magnetism Magnetic Mater. 324 (8), 1488–1497. doi:10.1016/j.jmmm.2011.11.039
Burke, G., Barron, V., Geever, T., Geever, L., Devine, D. M., and Higginbotham, C. L. (2019). Evaluation of the materials properties, stability and cell response of a range of PEGDMA hydrogels for tissue engineering applications. J. Mech. Behav. Biomed. Mater 99, 1–10. doi:10.1016/j.jmbbm.2019.07.003
Byambaa, B., Annabi, N., Yue, K., Trujillo-de Santiago, G., Alvarez, M. M., Jia, W., et al. (2017). Bioprinted osteogenic and vasculogenic patterns for engineering 3D bone tissue. Adv. Healthc. Mater 6 (16). doi:10.1002/adhm.201700015
Caffarelli, C., Al Refaie, A., Mondillo, C., De Vita, M., Baldassini, L., Valacchi, G., et al. (2023). Bone fracture in rett syndrome: mechanisms and prevention strategies. Child. (Basel) 10 (12), 1861. doi:10.3390/children10121861
Cao, L., Cao, B., Lu, C., Wang, G., Yu, L., and Ding, J. (2015). An injectable hydrogel formed by in situ cross-linking of glycol chitosan and multi-benzaldehyde functionalized PEG analogues for cartilage tissue engineering. J. Mater Chem. B 3 (7), 1268–1280. doi:10.1039/c4tb01705f
Cao, Z., Qin, Z., Duns, G. J., Huang, Z., Chen, Y., Wang, S., et al. (2024). Repair of infected bone defects with hydrogel materials. Polym. (Basel) 16 (2), 281. doi:10.3390/polym16020281
Chai, W., Chen, X., Liu, J., Zhang, L., Liu, C., Li, L., et al. (2024). Recent progress in functional metal-organic frameworks for bio-medical application. Regen. Biomater. 11, rbad115. doi:10.1093/rb/rbad115
Chandramouli, Y., Firoz, S., Vikram, A., Mahitha, B., Rubia, B., and Hemanthpavankumar, K. (2012). Tamarind seed polysaccharide (TSP) - an adaptable excipient for novel drug delivery systems. Int J. Pharm. Pract. Drug Res. 2, 57-63.
Chatterjee, S., Hui, P. C., and Kan, C. W. (2018). Thermoresponsive hydrogels and their biomedical applications: special insight into their applications in textile based transdermal therapy. Polym. (Basel) 10 (5), 480. doi:10.3390/polym10050480
Chaudhuri, O., Gu, L., Klumpers, D., Darnell, M., Bencherif, S. A., Weaver, J. C., et al. (2016). Hydrogels with tunable stress relaxation regulate stem cell fate and activity. Nat. Mater 15 (3), 326–334. doi:10.1038/nmat4489
Chen, J., Yang, J., Wang, L., Zhang, X., Heng, B. C., Wang, D. A., et al. (2021a). Modified hyaluronic acid hydrogels with chemical groups that facilitate adhesion to host tissues enhance cartilage regeneration. Bioact. Mater 6 (6), 1689–1698. doi:10.1016/j.bioactmat.2020.11.020
Chen, K., Gu, L., Zhang, Q., Luo, Q., Guo, S., Wang, B., et al. (2024). Injectable alginate hydrogel promotes antitumor immunity through glucose oxidase and Fe(3+) amplified RSL3-induced ferroptosis. Carbohydr. Polym. 326, 121643. doi:10.1016/j.carbpol.2023.121643
Chen, W., Zhang, H., Zhou, Q., Zhou, F., Zhang, Q., and Su, J. (2023). Smart hydrogels for bone reconstruction via modulating the microenvironment. Res. (Wash D C) 6, 0089. doi:10.34133/research.0089
Chen, X., Tan, B., Bao, Z., Wang, S., Tang, R., Wang, Z., et al. (2021b). Enhanced bone regeneration via spatiotemporal and controlled delivery of a genetically engineered BMP-2 in a composite Hydrogel. Biomaterials 277, 121117. doi:10.1016/j.biomaterials.2021.121117
Chen, X., Wang, S., Zhang, X., Yu, Y., Wang, J., and Liu, C. (2022). Dual-function injectable fibrin gel incorporated with sulfated chitosan nanoparticles for rhBMP-2-induced bone regeneration. Appl. Mater. Today 26, 101347. doi:10.1016/j.apmt.2021.101347
Chen, Y., Li, Y., Yang, X., Cao, Z., Nie, H., Bian, Y., et al. (2021c). Glucose-triggered in situ forming keratin hydrogel for the treatment of diabetic wounds. Acta Biomater. 125, 208–218. doi:10.1016/j.actbio.2021.02.035
Cheng, X., Jin, Y., Sun, T., Qi, R., Li, H., and Fan, W. (2016). An injectable, dual pH and oxidation-responsive supramolecular hydrogel for controlled dual drug delivery. Colloids Surf. B Biointerfaces 141, 44–52. doi:10.1016/j.colsurfb.2016.01.034
Cheng, Y., Nada, A. A., Valmikinathan, C. M., Lee, P., Liang, D., Yu, X., et al. (2014). In situ gelling polysaccharide-based hydrogel for cell and drug delivery in tissue engineering. drug Deliv. tissue Eng. 131 (4). doi:10.1002/app.39934
Choi, D. H., Lee, K. E., Oh, S. Y., Lee, S. M., Jo, B. S., Lee, J. Y., et al. (2021). Tonsil-derived mesenchymal stem cells incorporated in reactive oxygen species-releasing hydrogel promote bone formation by increasing the translocation of cell surface GRP78. Biomaterials 278, 121156. doi:10.1016/j.biomaterials.2021.121156
Criado-Gonzalez, M., Loftin, B., Rodon Fores, J., Vautier, D., Kocgozlu, L., Jierry, L., et al. (2020). Enzyme assisted peptide self-assemblies trigger cell adhesion in high density oxime based host gels. J. Mater Chem. B 8 (20), 4419–4427. doi:10.1039/d0tb00456a
Cui, Z. K., Kim, S., Baljon, J. J., Wu, B. M., Aghaloo, T., and Lee, M. (2019). Microporous methacrylated glycol chitosan-montmorillonite nanocomposite hydrogel for bone tissue engineering. Nat. Commun. 10 (1), 3523. doi:10.1038/s41467-019-11511-3
Delawan, M., Sharma, M., Ismail, M., Algabri, M. H., Abdalridha, R. H., Alawadi, M. N., et al. (2023). Methods of hemostasis in cranial neurosurgery: an anatomy-based stepwise review. World Neurosurg. 178, 241–259.e3. doi:10.1016/j.wneu.2023.08.030
Ding, D., Guerette, P. A., Fu, J., Zhang, L., Irvine, S. A., and Miserez, A. (2015). From soft self-healing gels to stiff films in suckerin-based materials through modulation of crosslink density and β-sheet content. Adv. Mater 27 (26), 3953–3961. doi:10.1002/adma.201500280
Ding, H., Li, B., Jiang, Y., Liu, G., Pu, S., Feng, Y., et al. (2021). pH-responsive UV crosslinkable chitosan hydrogel via "thiol-ene" click chemistry for active modulating opposite drug release behaviors. Carbohydr. Polym. 251, 117101. doi:10.1016/j.carbpol.2020.117101
Dispenza, C., Todaro, S., Bulone, D., Sabatino, M. A., Ghersi, G., San Biagio, P. L., et al. (2017). Physico-chemical and mechanical characterization of in-situ forming xyloglucan gels incorporating a growth factor to promote cartilage reconstruction. Mater Sci. Eng. C Mater Biol. Appl. 70 (Pt 1), 745–752. doi:10.1016/j.msec.2016.09.045
El-Husseiny, H. M., Mady, E. A., Hamabe, L., Abugomaa, A., Shimada, K., Yoshida, T., et al. (2022). Smart/stimuli-responsive hydrogels: cutting-edge platforms for tissue engineering and other biomedical applications. Mater Today Bio 13, 100186. doi:10.1016/j.mtbio.2021.100186
Elizalde-Cárdenas, A., Ribas-Aparicio, R. M., Rodríguez-Martínez, A., Leyva-Gómez, G., Ríos-Castañeda, C., and González-Torres, M. (2024). Advances in chitosan and chitosan derivatives for biomedical applications in tissue engineering: an updated review. Int. J. Biol. Macromol. 262 (Pt 1), 129999. doi:10.1016/j.ijbiomac.2024.129999
Fan, C., and Wang, D. A. (2017). Macroporous hydrogel scaffolds for three-dimensional cell culture and tissue engineering. Tissue Eng. Part B Rev. 23 (5), 451–461. doi:10.1089/ten.TEB.2016.0465
Farzaneh, S., Hosseinzadeh, S., Samanipour, R., Hatamie, S., Ranjbari, J., and Khojasteh, A. (2021). Fabrication and characterization of cobalt ferrite magnetic hydrogel combined with static magnetic field as a potential bio-composite for bone tissue engineering. J. Drug Deliv. Sci. Technol. 64, 102525. doi:10.1016/j.jddst.2021.102525
Faustino, C., Duarte, N., and Pinheiro, L. (2023). Triterpenes drug delivery systems, a modern approach for arthritis targeted therapy. Pharm. (Basel) 17 (1), 54. doi:10.3390/ph17010054
Gan, M., Zhou, Q., Ge, J., Zhao, J., Wang, Y., Yan, Q., et al. (2021). Precise in-situ release of microRNA from an injectable hydrogel induces bone regeneration. Acta Biomater. 135, 289–303. doi:10.1016/j.actbio.2021.08.041
Gang, F., Xu, M., Zhang, S., Zhang, C., He, J., Xiao, Y., et al. (2024). Biodegradable active composite hydrogel packaging for postharvest climacteric bananas preservation. Food Chem. 442, 138494. doi:10.1016/j.foodchem.2024.138494
Gao, Y., Wei, Z., Li, F., Yang, Z. M., Chen, Y. M., Zrinyi, M., et al. (2014). Synthesis of a morphology controllable Fe3O4 nanoparticle/hydrogel magnetic nanocomposite inspired by magnetotactic bacteria and its application in H2O2 detection. Green Chem. 16 (3), 1255–1261. doi:10.1039/C3GC41535J
Gao, Z. G., Fain, H. D., and Rapoport, N. (2005). Controlled and targeted tumor chemotherapy by micellar-encapsulated drug and ultrasound. J. Control Release 102 (1), 203–222. doi:10.1016/j.jconrel.2004.09.021
Garg, A., Agrawal, R., Singh Chauhan, C., and Deshmukh, R. (2024). In-situ gel: a smart carrier for drug delivery. Int. J. Pharm. 652, 123819. doi:10.1016/j.ijpharm.2024.123819
Gilchrist, A. E., Serrano, J. F., Ngo, M. T., Hrnjak, Z., Kim, S., and Harley, B. A. C. (2021). Encapsulation of murine hematopoietic stem and progenitor cells in a thiol-crosslinked maleimide-functionalized gelatin hydrogel. Acta Biomater. 131, 138–148. doi:10.1016/j.actbio.2021.06.028
Goh, M., Du, M., Peng, W. R., Saw, P. E., and Chen, Z. (2024). Advancing burn wound treatment: exploring hydrogel as a transdermal drug delivery system. Drug Deliv. 31 (1), 2300945. doi:10.1080/10717544.2023.2300945
Gong, C., Shan, M., Li, B., and Wu, G. (2017). Injectable dual redox responsive diselenide-containing poly(ethylene glycol) hydrogel. J. Biomed. Mater Res. A 105 (9), 2451–2460. doi:10.1002/jbm.a.36103
Greenberg, C. S., Birckbichler, P. J., and Rice, R. H. (1991). Transglutaminases: multifunctional cross-linking enzymes that stabilize tissues. Faseb J. 5 (15), 3071–3077. doi:10.1096/fasebj.5.15.1683845
Gupta, M. K., Martin, J. R., Werfel, T. A., Shen, T., Page, J. M., and Duvall, C. L. (2014). Cell protective, ABC triblock polymer-based thermoresponsive hydrogels with ROS-triggered degradation and drug release. J. Am. Chem. Soc. 136 (42), 14896–14902. doi:10.1021/ja507626y
Harada, S., and Rodan, G. A. (2003). Control of osteoblast function and regulation of bone mass. Nature 423 (6937), 349–355. doi:10.1038/nature01660
Hermann, C. D., Wilson, D. S., Lawrence, K. A., Ning, X., Olivares-Navarrete, R., Williams, J. K., et al. (2014). Rapidly polymerizing injectable click hydrogel therapy to delay bone growth in a murine re-synostosis model. Biomaterials 35 (36), 9698–9708. doi:10.1016/j.biomaterials.2014.07.065
Hernández-González, A. C., Téllez-Jurado, L., and Rodríguez-Lorenzo, L. M. (2020). Alginate hydrogels for bone tissue engineering, from injectables to bioprinting: a review. Carbohydr. Polym. 229, 115514. doi:10.1016/j.carbpol.2019.115514
Hernández-González, A. C., Téllez-Jurado, L., and Rodríguez-Lorenzo, L. M. (2021). Preparation of covalently bonded silica-alginate hybrid hydrogels by SCHIFF base and sol-gel reactions. Carbohydr. Polym. 267, 118186. doi:10.1016/j.carbpol.2021.118186
Hickman, D. A., Pawlowski, C. L., Sekhon, U. D. S., Marks, J., and Gupta, A. S. (2018). Biomaterials and advanced technologies for hemostatic management of bleeding. Adv. Mater 30 (4). doi:10.1002/adma.201700859
Hong, X., Tian, G., Zhu, Y., and Ren, T. (2024). Exogeneous metal ions as therapeutic agents in cardiovascular disease and their delivery strategies. Regen. Biomater. 11, rbad103. doi:10.1093/rb/rbad103
Hu, B., Gao, M., Boakye-Yiadom, K. O., Ho, W., Yu, W., Xu, X., et al. (2021). An intrinsically bioactive hydrogel with on-demand drug release behaviors for diabetic wound healing. Bioact. Mater 6 (12), 4592–4606. doi:10.1016/j.bioactmat.2021.04.040
Hu, H., Dong, L., Bu, Z., Shen, Y., Luo, J., Zhang, H., et al. (2020). miR-23a-3p-abundant small extracellular vesicles released from Gelma/nanoclay hydrogel for cartilage regeneration. J. Extracell. Vesicles 9 (1), 1778883. doi:10.1080/20013078.2020.1778883
Huang, H., Tan, Y., Ayers, D. C., and Song, J. (2018). Anionic and zwitterionic residues modulate stiffness of photo-cross-linked hydrogels and cellular behavior of encapsulated chondrocytes. ACS Biomater. Sci. Eng. 4 (5), 1843–1851. doi:10.1021/acsbiomaterials.8b00124
Hubbell, J. A. (2003). Materials as morphogenetic guides in tissue engineering. Curr. Opin. Biotechnol. 14 (5), 551–558. doi:10.1016/j.copbio.2003.09.004
Jansen, L. E., Negrón-Piñeiro, L. J., Galarza, S., and Peyton, S. R. (2018). Control of thiol-maleimide reaction kinetics in PEG hydrogel networks. Acta Biomater. 70, 120–128. doi:10.1016/j.actbio.2018.01.043
Jewett, J. C., and Bertozzi, C. R. (2010). Cu-free click cycloaddition reactions in chemical biology. Chem. Soc. Rev. 39 (4), 1272–1279. doi:10.1039/b901970g
Jiang, B. P., Zhou, B., Lin, Z., Liang, H., and Shen, X. C. (2019). Recent advances in carbon nanomaterials for cancer phototherapy. Chemistry 25 (16), 3993–4004. doi:10.1002/chem.201804383
Jin, R., Moreira Teixeira, L. S., Dijkstra, P. J., van Blitterswijk, C. A., Karperien, M., and Feijen, J. (2011). Chondrogenesis in injectable enzymatically crosslinked heparin/dextran hydrogels. J. Control Release 152 (1), 186–195. doi:10.1016/j.jconrel.2011.01.031
Jung, S. W., Byun, J. H., Oh, S. H., Kim, T. H., Park, J. S., Rho, G. J., et al. (2018). Multivalent ion-based in situ gelling polysaccharide hydrogel as an injectable bone graft. Carbohydr. Polym. 180, 216–225. doi:10.1016/j.carbpol.2017.10.029
Kalairaj, M. S., Pradhan, R., Saleem, W., Smith, M. M., and Gaharwar, A. K. (2024). Intra-articular injectable biomaterials: therapeutic delivery for cartilage repair and regeneration. Adv. Healthc. Mater, e2303794. doi:10.1002/adhm.202303794
Karimi, M., Sahandi Zangabad, P., Ghasemi, A., Amiri, M., Bahrami, M., Malekzad, H., et al. (2016). Temperature-responsive smart nanocarriers for delivery of therapeutic agents: applications and recent advances. ACS Appl. Mater Interfaces 8 (33), 21107–21133. doi:10.1021/acsami.6b00371
Khanmohammadi, M., Dastjerdi, M. B., Ai, A., Ahmadi, A., Godarzi, A., Rahimi, A., et al. (2018). Horseradish peroxidase-catalyzed hydrogelation for biomedical applications. Biomater. Sci. 6 (6), 1286–1298. doi:10.1039/c8bm00056e
Kim, B. S., Sung, H. M., You, H. K., and Lee, J. (2014). Effects of fibrinogen concentration on fibrin glue and bone powder scaffolds in bone regeneration. J. Biosci. Bioeng. 118 (4), 469–475. doi:10.1016/j.jbiosc.2014.03.014
Kim, H. S., and Lee, D. Y. (2018). Near-infrared-responsive cancer photothermal and photodynamic therapy using gold nanoparticles. Polym. (Basel) 10 (9), 961. doi:10.3390/polym10090961
Kim, J. A., An, Y. H., Yim, H. G., Han, W. J., Park, Y. B., Park, H. J., et al. (2021). Injectable fibrin/polyethylene oxide semi-IPN hydrogel for a segmental meniscal defect regeneration. Am. J. Sports Med. 49 (6), 1538–1550. doi:10.1177/0363546521998021
Ko, S., Park, J. Y., and Oh, Y. K. (2019). A microbial siderophore-inspired self-gelling hydrogel for noninvasive anticancer phototherapy. Cancer Res. 79 (24), 6178–6189. doi:10.1158/0008-5472.Can-19-0975
Krainer, F. W., and Glieder, A. (2015). An updated view on horseradish peroxidases: recombinant production and biotechnological applications. Appl. Microbiol. Biotechnol. 99 (4), 1611–1625. doi:10.1007/s00253-014-6346-7
Kumar, C. S., and Mohammad, F. (2011). Magnetic nanomaterials for hyperthermia-based therapy and controlled drug delivery. Adv. Drug Deliv. Rev. 63 (9), 789–808. doi:10.1016/j.addr.2011.03.008
Kurian, A. G., Singh, R. K., Patel, K. D., Lee, J. H., and Kim, H. W. (2022). Multifunctional GelMA platforms with nanomaterials for advanced tissue therapeutics. Bioact. Mater 8, 267–295. doi:10.1016/j.bioactmat.2021.06.027
Lai, T. S., Lin, C. J., and Greenberg, C. S. (2017). Role of tissue transglutaminase-2 (TG2)-mediated aminylation in biological processes. Amino Acids 49 (3), 501–515. doi:10.1007/s00726-016-2270-8
Lao, W., Fan, L., Zhang, Q., Lou, C., Li, H., Li, Y., et al. (2023). Click-based injectable bioactive PEG-hydrogels guide rapid craniomaxillofacial bone regeneration by the spatiotemporal delivery of rhBMP-2. J. Mater Chem. B 11 (14), 3136–3150. doi:10.1039/d2tb02703h
Lee, H. P., Lokhande, G., Singh, K. A., Jaiswal, M. K., Rajput, S., and Gaharwar, A. K. (2021). Light-triggered in situ gelation of hydrogels using 2D molybdenum disulfide (MoS(2)) nanoassemblies as crosslink epicenter. Adv. Mater 33 (23), e2101238. doi:10.1002/adma.202101238
Lee, J. H. (2018). Injectable hydrogels delivering therapeutic agents for disease treatment and tissue engineering. Biomater. Res. 22, 27. doi:10.1186/s40824-018-0138-6
Li, D., Li, M., Wang, L., Zhang, J., Wang, X., Nie, J., et al. (2024). The synergetic effect of alginate-derived hydrogels and metal-phenolic nanospheres for chronic wound therapy. J. Mater Chem. B 12, 2571–2586. doi:10.1039/d3tb02685j
Li, J., Ma, J., Feng, Q., Xie, E., Meng, Q., Shu, W., et al. (2023a). Building osteogenic microenvironments with a double-network composite hydrogel for bone repair. Res. (Wash D C) 6, 0021. doi:10.34133/research.0021
Li, P., Zhang, J. C., and Dong, C. M. (2017). Photosensitive poly(o-nitrobenzyloxycarbonyl-L-lysine)-b-PEO polypeptide copolymers: synthesis, multiple self-assembly behaviors, and the photo/pH-thermo-sensitive hydrogels. Polym. Chem. 8 (45), 7033–7043. doi:10.1039/c7py01574g
Li, Q., Xu, S., Feng, Q., Dai, Q., Yao, L., Zhang, Y., et al. (2021a). 3D printed silk-gelatin hydrogel scaffold with different porous structure and cell seeding strategy for cartilage regeneration. Bioact. Mater 6 (10), 3396–3410. doi:10.1016/j.bioactmat.2021.03.013
Li, X., Lu, Y., Wang, Y., Zhou, S., Li, L., and Zhao, F. (2021b). Thermo-responsive injectable naringin-loaded hydrogel polymerised sodium alginate/bioglass delivery for articular cartilage. Drug Deliv. 28 (1), 1290–1300. doi:10.1080/10717544.2021.1938752
Li, Y. Y., Zhang, L. Y., Xiang, Y. H., Li, D., and Zhang, J. (2023b). Matrix metalloproteinases and tissue inhibitors in multiple myeloma: promote or inhibit? Front. Oncol. 13, 1127407. doi:10.3389/fonc.2023.1127407
Li, Z., Li, B., Li, X., Lin, Z., Chen, L., Chen, H., et al. (2021c). Ultrafast in-situ forming halloysite nanotube-doped chitosan/oxidized dextran hydrogels for hemostasis and wound repair. Carbohydr. Polym. 267, 118155. doi:10.1016/j.carbpol.2021.118155
Liu, C., Guo, X., Ruan, C., Hu, H., Jiang, B.-P., Liang, H., et al. (2019). An injectable thermosensitive photothermal-network hydrogel for near-infrared-triggered drug delivery and synergistic photothermal-chemotherapy. Acta Biomater. 96, 281–294. doi:10.1016/j.actbio.2019.07.024
Liu, H., Hu, Y., Liu, Y., Hu, R., Wu, X., and Li, B. (2023a). A review of recent advances in biomedical applications of smart cellulose-based hydrogels. Int. J. Biol. Macromol. 253 (Pt 6), 127149. doi:10.1016/j.ijbiomac.2023.127149
Liu, J., Fang, Q., Lin, H., Yu, X., Zheng, H., and Wan, Y. (2020). Alginate-poloxamer/silk fibroin hydrogels with covalently and physically cross-linked networks for cartilage tissue engineering. Carbohydr. Polym. 247, 116593. doi:10.1016/j.carbpol.2020.116593
Liu, M., Zeng, X., Ma, C., Yi, H., Ali, Z., Mou, X., et al. (2017). Injectable hydrogels for cartilage and bone tissue engineering. Bone Res. 5, 17014. doi:10.1038/boneres.2017.14
Liu, M., Zhang, W., Han, S., Zhang, D., Zhou, X., Guo, X., et al. (2024). Multifunctional conductive and electrogenic hydrogel repaired spinal cord injury via immunoregulation and enhancement of neuronal differentiation. Adv. Mater, e2313672. doi:10.1002/adma.202313672
Liu, S., Yu, J. M., Gan, Y. C., Qiu, X. Z., Gao, Z. C., Wang, H., et al. (2023b). Biomimetic natural biomaterials for tissue engineering and regenerative medicine: new biosynthesis methods, recent advances, and emerging applications. Mil. Med. Res. 10 (1), 16. doi:10.1186/s40779-023-00448-w
Liu, X., Camilleri, E. T., Li, L., Gaihre, B., Rezaei, A., Park, S., et al. (2021). Injectable catalyst-free "click" organic-inorganic nanohybrid (click-ON) cement for minimally invasive in vivo bone repair. Biomaterials 276, 121014. doi:10.1016/j.biomaterials.2021.121014
Liu, Z. Q., Wei, Z., Zhu, X. L., Huang, G. Y., Xu, F., Yang, J. H., et al. (2015). Dextran-based hydrogel formed by thiol-Michael addition reaction for 3D cell encapsulation. Colloids Surfaces B Biointerfaces 128, 140–148. doi:10.1016/j.colsurfb.2015.02.005
Luo, D., Zhu, H., Li, S., Wang, Z., and Xiao, J. (2024). Mesenchymal stem cell-derived exosomes as a promising cell-free therapy for knee osteoarthritis. Front. Bioeng. Biotechnol. 12, 1309946. doi:10.3389/fbioe.2024.1309946
Lyu, Y., Xie, J., Liu, Y., Xiao, M., Li, Y., Yang, J., et al. (2020). Injectable hyaluronic acid hydrogel loaded with functionalized human mesenchymal stem cell aggregates for repairing infarcted myocardium. ACS Biomater. Sci. Eng. 6 (12), 6926–6937. doi:10.1021/acsbiomaterials.0c01344
Ma, F., Ge, Y., Liu, N., Pang, X., Shen, X., and Tang, B. (2019). In situ fabrication of a composite hydrogel with tunable mechanical properties for cartilage tissue engineering. J. Mater Chem. B 7 (15), 2463–2473. doi:10.1039/c8tb01331d
Madry, H., Gao, L., Rey-Rico, A., Venkatesan, J. K., Müller-Brandt, K., Cai, X., et al. (2020). Thermosensitive hydrogel based on PEO-PPO-PEO poloxamers for a controlled in situ release of recombinant adeno-associated viral vectors for effective gene therapy of cartilage defects. Adv. Mater 32 (2), e1906508. doi:10.1002/adma.201906508
Manouras, T., and Vamvakaki, M. (2017). Field responsive materials: photo-electro-magnetic- and ultrasound-sensitive polymers. Polym. Chem. 8 (1), 74–96. doi:10.1039/C6PY01455K
Mather, B. D., Viswanathan, K., Miller, K. M., and Long, T. E. (2006). Michael addition reactions in macromolecular design for emerging technologies. Prog. Polym. Sci. 31 (5), 487–531. doi:10.1016/j.progpolymsci.2006.03.001
Meng, Z., Chao, Y., Zhou, X., Liang, C., Liu, J., Zhang, R., et al. (2018). Near-infrared-triggered in situ gelation system for repeatedly enhanced photothermal brachytherapy with a single dose. ACS Nano 12 (9), 9412–9422. doi:10.1021/acsnano.8b04544
Montazerian, H., Baidya, A., Haghniaz, R., Davoodi, E., Ahadian, S., Annabi, N., et al. (2021). Stretchable and bioadhesive gelatin methacryloyl-based hydrogels enabled by in situ dopamine polymerization. ACS Appl. Mater. Interfaces 13 (34), 40290–40301. doi:10.1021/acsami.1c10048
Morrison, T. X., and Gramlich, W. M. (2023). Tunable, thiol-ene, interpenetrating network hydrogels of norbornene-modified carboxymethyl cellulose and cellulose nanofibrils. Carbohydr. Polym. 319, 121173. doi:10.1016/j.carbpol.2023.121173
Muscolino, E., Di Stefano, A. B., Trapani, M., Sabatino, M. A., Giacomazza, D., Moschella, F., et al. (2021). Injectable xyloglucan hydrogels incorporating spheroids of adipose stem cells for bone and cartilage regeneration. Mater. Sci. Eng. C 131, 112545. doi:10.1016/j.msec.2021.112545
Nair, D. P., Podgórski, M., Chatani, S., Gong, T., Xi, W., Fenoli, C. R., et al. (2014). The thiol-michael addition click reaction: a powerful and widely used tool in materials chemistry. Chem. Mater. 26 (1), 724–744. doi:10.1021/cm402180t
Nandi, S. K., Kundu, B., Ghosh, S. K., De, D. K., and Basu, D. (2008). Efficacy of nano-hydroxyapatite prepared by an aqueous solution combustion technique in healing bone defects of goat. J. Vet. Sci. 9 (2), 183–191. doi:10.4142/jvs.2008.9.2.183
Neves, S. C., Moroni, L., Barrias, C. C., and Granja, P. L. (2020). Leveling up hydrogels: hybrid systems in tissue engineering. Trends Biotechnol. 38 (3), 292–315. doi:10.1016/j.tibtech.2019.09.004
Nguyen, H. D., Liu, H. Y., Hudson, B. N., and Lin, C. C. (2019). Enzymatic cross-linking of dynamic thiol-norbornene click hydrogels. ACS Biomater. Sci. Eng. 5 (3), 1247–1256. doi:10.1021/acsbiomaterials.8b01607
Nguyen, T. P. T., Li, F., Shrestha, S., Tuan, R. S., Thissen, H., Forsythe, J. S., et al. (2021). Cell-laden injectable microgels: current status and future prospects for cartilage regeneration. Biomaterials 279, 121214. doi:10.1016/j.biomaterials.2021.121214
Noori, A., Ashrafi, S. J., Vaez-Ghaemi, R., Hatamian-Zaremi, A., and Webster, T. J. (2017). A review of fibrin and fibrin composites for bone tissue engineering. Int. J. Nanomedicine 12, 4937–4961. doi:10.2147/ijn.S124671
Ouyang, Z., Dong, L., Yao, F., Wang, K., Chen, Y., Li, S., et al. (2023). Cartilage-related collagens in osteoarthritis and rheumatoid arthritis: from pathogenesis to therapeutics. Int. J. Mol. Sci. 24 (12), 9841. doi:10.3390/ijms24129841
Park, J., Ghanim, R., Rahematpura, A., Gerage, C., and Abramson, A. (2024). Electromechanical convective drug delivery devices for overcoming diffusion barriers. J. Control Release 366, 650–667. doi:10.1016/j.jconrel.2024.01.008
Park, S. H., Park, J. Y., Ji, Y. B., Ju, H. J., Min, B. H., and Kim, M. S. (2020). An injectable click-crosslinked hyaluronic acid hydrogel modified with a BMP-2 mimetic peptide as a bone tissue engineering scaffold. Acta Biomater. 117, 108–120. doi:10.1016/j.actbio.2020.09.013
Patel, H., Chen, J., Hu, Y., and Erturk, A. (2022). Photo-responsive hydrogel-based re-programmable metamaterials. Sci. Rep. 12 (1), 13033. doi:10.1038/s41598-022-15453-7
Pranantyo, D., Yeo, C. K., Wu, Y., Fan, C., Xu, X., Yip, Y. S., et al. (2024). Hydrogel dressings with intrinsic antibiofilm and antioxidative dual functionalities accelerate infected diabetic wound healing. Nat. Commun. 15 (1), 954. doi:10.1038/s41467-024-44968-y
Price, G. J. (2003). Recent developments in sonochemical polymerisation. Ultrason. Sonochem 10 (4-5), 277–283. doi:10.1016/s1350-4177(02)00156-6
Qi, C., Liu, J., Jin, Y., Xu, L., Wang, G., Wang, Z., et al. (2018). Photo-crosslinkable, injectable sericin hydrogel as 3D biomimetic extracellular matrix for minimally invasive repairing cartilage. Biomaterials 163, 89–104. doi:10.1016/j.biomaterials.2018.02.016
Qiao, Y., Liu, X., Zhou, X., Zhang, H., Zhang, W., Xiao, W., et al. (2020). Gelatin templated polypeptide Co-Cross-Linked hydrogel for bone regeneration. Adv. Healthc. Mater. 9 (1), 1901239. doi:10.1002/adhm.201901239
Qu, S., Tang, Y., Ning, Z., Zhou, Y., and Wu, H. (2024). Desired properties of polymeric hydrogel vitreous substitute. Biomed. Pharmacother. 172, 116154. doi:10.1016/j.biopha.2024.116154
Ramírez, O., Bonardd, S., Saldías, C., Leiva, A., and Díaz Díaz, D. (2024). Highly efficient and reusable CuAu nanoparticles supported on crosslinked chitosan hydrogels as a plasmonic catalyst for nitroarene reduction. Environ. Res. 247, 118204. doi:10.1016/j.envres.2024.118204
Raymond, K. N., Allred, B. E., and Sia, A. K. (2015). Coordination chemistry of microbial iron transport. Acc. Chem. Res. 48 (9), 2496–2505. doi:10.1021/acs.accounts.5b00301
Raza, A., Hayat, U., Rasheed, T., Bilal, M., and Iqbal, H. M. N. (2019). “Smart” materials-based near-infrared light-responsive drug delivery systems for cancer treatment: a review. J. Mater. Res. Technol. 8 (1), 1497–1509. doi:10.1016/j.jmrt.2018.03.007
Ren, Z., Wang, Y., Ma, S., Duan, S., Yang, X., Gao, P., et al. (2015). Effective bone regeneration using thermosensitive poly(N-isopropylacrylamide) grafted gelatin as injectable carrier for bone mesenchymal stem cells. ACS Appl. Mater Interfaces 7 (34), 19006–19015. doi:10.1021/acsami.5b02821
Riviello, G., Connor, B., McBrearty, J., Rodriguez, G., and Hu, X. (2024). Protein and polysaccharide-based optical materials for biomedical applications. Int. J. Mol. Sci. 25 (3), 1861. doi:10.3390/ijms25031861
Rizwan, A., Ali, I., Jo, S. H., Vu, T. T., Gal, Y. S., Kim, Y. H., et al. (2023). Facile fabrication of NIR-responsive alginate/CMC hydrogels derived through IEDDA click chemistry for photothermal-photodynamic anti-tumor therapy. Gels 9 (12), 961. doi:10.3390/gels9120961
Rodolfi, S., Davidson, C., and Vecellio, M. (2023). Regulatory T cells in spondyloarthropathies: genetic evidence, functional role, and therapeutic possibilities. Front. Immunol. 14, 1303640. doi:10.3389/fimmu.2023.1303640
Ryan, B. J., Carolan, N., and O’Fágáin, C. (2006). Horseradish and soybean peroxidases: comparable tools for alternative niches? Trends Biotechnol. 24 (8), 355–363. doi:10.1016/j.tibtech.2006.06.007
Saghati, S., Khoshfetrat, A. B., Tayefi Nasrabadi, H., Roshangar, L., and Rahbarghazi, R. (2021). Fabrication of alginate-based hydrogel cross-linked via horseradish peroxidase for articular cartilage engineering. BMC Res. Notes 14 (1), 384. doi:10.1186/s13104-021-05795-2
Saravanan, S., Vimalraj, S., Thanikaivelan, P., Banudevi, S., and Manivasagam, G. (2019). A review on injectable chitosan/beta glycerophosphate hydrogels for bone tissue regeneration. Int. J. Biol. Macromol. 121, 38–54. doi:10.1016/j.ijbiomac.2018.10.014
Savoca, M. P., Tonoli, E., Atobatele, A. G., and Verderio, E. A. M. (2018). Biocatalysis by transglutaminases: a review of biotechnological applications. Micromachines (Basel) 9 (11), 562. doi:10.3390/mi9110562
Seo, B. B., Kwon, Y., Kim, J., Hong, K. H., Kim, S. E., Song, H. R., et al. (2022). Injectable polymeric nanoparticle hydrogel system for long-term anti-inflammatory effect to treat osteoarthritis. Bioact. Mater 7, 14–25. doi:10.1016/j.bioactmat.2021.05.028
Shang, F., Yu, Y., Liu, S., Ming, L., Zhang, Y., Zhou, Z., et al. (2021). Advancing application of mesenchymal stem cell-based bone tissue regeneration. Bioact. Mater 6 (3), 666–683. doi:10.1016/j.bioactmat.2020.08.014
Shen, K. H., Lu, C. H., Kuo, C. Y., Li, B. Y., and Yeh, Y. C. (2021). Smart near infrared-responsive nanocomposite hydrogels for therapeutics and diagnostics. J. Mater Chem. B 9 (35), 7100–7116. doi:10.1039/d1tb00980j
Shi, D., Mei, Y., Hao, W., Li, J., Liu, S., and Lin, X. (2023). Biological functions and applications of LncRNAs in the regulation of the extracellular matrix in osteoarthritis. Front. Cell Dev. Biol. 11, 1330624. doi:10.3389/fcell.2023.1330624
Sood, N., Bhardwaj, A., Mehta, S., and Mehta, A. (2016). Stimuli-responsive hydrogels in drug delivery and tissue engineering. Drug Deliv. 23 (3), 748–770. doi:10.3109/10717544.2014.940091
Stolzing, A., Jones, E., McGonagle, D., and Scutt, A. (2008). Age-related changes in human bone marrow-derived mesenchymal stem cells: consequences for cell therapies. Mech. Ageing Dev. 129 (3), 163–173. doi:10.1016/j.mad.2007.12.002
Su, Z., Tan, P., Zhang, J., Wang, P., Zhu, S., and Jiang, N. (2023). Understanding the mechanics of the temporomandibular joint osteochondral interface from micro- and nanoscopic perspectives. Nano Lett. 23 (24), 11702–11709. doi:10.1021/acs.nanolett.3c03564
Sun, L., Xu, Y., Han, Y., Cui, J., Jing, Z., Li, D., et al. (2023a). Collagen-based hydrogels for cartilage regeneration. Orthop. Surg. 15 (12), 3026–3045. doi:10.1111/os.13884
Sun, S., Oliveira, B. L., Jiménez-Osés, G., and Bernardes, G. J. L. (2018a). Radical-mediated thiol-ene strategy: photoactivation of thiol-containing drugs in cancer cells. Angew. Chem. Int. Ed. Engl. 57 (48), 15832–15835. doi:10.1002/anie.201811338
Sun, W., Ye, B., Chen, S., Zeng, L., Lu, H., Wan, Y., et al. (2023b). Neuro-bone tissue engineering: emerging mechanisms, potential strategies, and current challenges. Bone Res. 11 (1), 65. doi:10.1038/s41413-023-00302-8
Sun, X. M., Zhang, H. B., He, J. L., Cheng, R. Y., Cao, Y. W., Che, K. M., et al. (2018b). Adjustable hardness of hydrogel for promoting vascularization and maintaining stemness of stem cells in skin flap regeneration. Appl. Mater. TODAY 13, 54–63. doi:10.1016/j.apmt.2018.08.007
Sung, T. C., Chen, Y. H., Wang, T., Qian, L., Chao, W. H., Liu, J., et al. (2024). Design of dual peptide-conjugated hydrogels for proliferation and differentiation of human pluripotent stem cells. Mater Today Bio 25, 100969. doi:10.1016/j.mtbio.2024.100969
Takaoka, Y., Fujibayashi, S., Onoe, H., Goto, K., Otsuki, B., Kawai, T., et al. (2024). Bone ingrowth into a porous structure is achieved by preceding fibrogenesis and vascularization. Acta Biomater. 177, 243–252. doi:10.1016/j.actbio.2024.02.016
Tang, Y., Wu, B., Huang, T., Wang, H., Shi, R., Lai, W., et al. (2023). Collision of commonality and personalization: better understanding of the periosteum. Tissue Eng. Part B Rev. 29 (2), 91–102. doi:10.1089/ten.TEB.2022.0076
Teixeira, L. S., Feijen, J., van Blitterswijk, C. A., Dijkstra, P. J., and Karperien, M. (2012). Enzyme-catalyzed crosslinkable hydrogels: emerging strategies for tissue engineering. Biomaterials 33 (5), 1281–1290. doi:10.1016/j.biomaterials.2011.10.067
Thoniyot, P., Tan, M. J., Karim, A. A., Young, D. J., and Loh, X. J. (2015). Nanoparticle-hydrogel composites: concept, design, and applications of these promising, multi-functional materials. Adv. Sci. (Weinh) 2 (1-2), 1400010. doi:10.1002/advs.201400010
Tournier, P., Saint-Pé, G., Lagneau, N., Loll, F., Halgand, B., Tessier, A., et al. (2023). Clickable dynamic bioinks enable post-printing modifications of construct composition and mechanical properties controlled over time and space. Adv. Sci. (Weinh) 10 (30), e2300055. doi:10.1002/advs.202300055
Vaidya, G., Pramanik, S., Kadi, A., Rayshan, A. R., Abualsoud, B. M., Ansari, M. J., et al. (2024). Injecting hope: chitosan hydrogels as bone regeneration innovators. J. Biomater. Sci. Polym. Ed. 35, 756–797. doi:10.1080/09205063.2024.2304952
van der Stok, J., Koolen, M. K., de Maat, M. P., Yavari, S. A., Alblas, J., Patka, P., et al. (2015). Full regeneration of segmental bone defects using porous titanium implants loaded with BMP-2 containing fibrin gels. Eur. Cell Mater 29, 141–154. doi:10.22203/ecm.v029a11
Vidal, L., Lopez-Garzon, M., Venegas, V., Vila, I., Domínguez, D., Rodas, G., et al. (2024). A novel tendon injury model, induced by collagenase administration combined with a thermo-responsive hydrogel in rats, reproduces the pathogenesis of human degenerative tendinopathy. Int. J. Mol. Sci. 25 (3), 1868. doi:10.3390/ijms25031868
Vu, T. T., Gulfam, M., Jo, S. H., Park, S. H., and Lim, K. T. (2022). Injectable and biocompatible alginate-derived porous hydrogels cross-linked by IEDDA click chemistry for reduction-responsive drug release application. Carbohydr. Polym. 278, 118964. doi:10.1016/j.carbpol.2021.118964
Wang, B., Liu, J., Niu, D., Wu, N., Yun, W., Wang, W., et al. (2021a). Mussel-Inspired bisphosphonated injectable nanocomposite hydrogels with adhesive, self-healing, and osteogenic properties for bone regeneration. ACS Appl. Mater Interfaces 13 (28), 32673–32689. doi:10.1021/acsami.1c06058
Wang, C., Zhao, N., and Yuan, W. (2020). NIR/Thermoresponsive injectable self-healing hydrogels containing polydopamine nanoparticles for efficient synergistic cancer thermochemotherapy. ACS Appl. Mater Interfaces 12 (8), 9118–9131. doi:10.1021/acsami.9b23536
Wang, H., Yuan, T., Wang, Y., Liu, C., Li, D., Li, Z., et al. (2024a). Osteoclasts and osteoarthritis: novel intervention targets and therapeutic potentials during aging. Aging Cell, e14092. doi:10.1111/acel.14092
Wang, J., Huang, L., Huang, Y., Jiang, Y., Zhang, L., Feng, G., et al. (2021b). Therapeutic effect of the injectable thermosensitive hydrogel loaded with SHP099 on intervertebral disc degeneration. Life Sci. 266, 118891. doi:10.1016/j.lfs.2020.118891
Wang, K., Liu, X., Liang, X., Jiang, Y., Wen, C. Y., and Zeng, J. (2024b). Near-infrared responsive Ag@Au nanoplates with exceptional stability for highly sensitive colorimetric and photothermal dual-mode lateral flow immunoassay. Anal. Chem. doi:10.1021/acs.analchem.3c05787
Wang, P., Shao, W., Li, Z., Wang, B., Lv, X., Huang, Y., et al. (2024c). Non-bone-derived exosomes: a new perspective on regulators of bone homeostasis. Cell Commun. Signal 22 (1), 70. doi:10.1186/s12964-023-01431-7
Wang, X., Wei, W., Guo, Z., Liu, X., Liu, J., Bing, T., et al. (2024d). Organic-inorganic composite hydrogels: compositions, properties, and applications in regenerative medicine. Biomater. Sci. 12, 1079–1114. doi:10.1039/d3bm01766d
Wegst, U. G., Bai, H., Saiz, E., Tomsia, A. P., and Ritchie, R. O. (2015). Bioinspired structural materials. Nat. Mater 14 (1), 23–36. doi:10.1038/nmat4089
Werzer, O., Tumphart, S., Keimel, R., Christian, P., and Coclite, A. M. (2019). Drug release from thin films encapsulated by a temperature-responsive hydrogel. Soft Matter 15 (8), 1853–1859. doi:10.1039/c8sm02529k
Wihadmadyatami, H., Zulfikar, M. A., Herawati, H., Pratama, D., Saragih, G. R., Kustiati, U., et al. (2023). Chitosan hydrogel nanoparticle enhance therapeutic effect of bovine umbilical mesenchymal stem cell conditioned medium on canine cognitive dysfunction or canine Alzheimer’s like mediated by inhibition of neuronal apoptotic. Open Vet. J. 13 (12), 1504–1516. doi:10.5455/OVJ.2023.v13.i12.1
Wiklund, M., Green, R., and Ohlin, M. (2012). Acoustofluidics 14: applications of acoustic streaming in microfluidic devices. Lab. Chip 12 (14), 2438–2451. doi:10.1039/c2lc40203c
Wu, C., and Chang, J. (2014). Multifunctional mesoporous bioactive glasses for effective delivery of therapeutic ions and drug/growth factors. J. Control Release 193, 282–295. doi:10.1016/j.jconrel.2014.04.026
Wu, D., Tao, S., Zhu, L., Zhao, C., and Xu, N. (2024a). Chitosan hydrogel dressing loaded with adipose mesenchymal stem cell-derived exosomes promotes skin full-thickness wound repair. ACS Appl. Bio Mater 7, 1125–1134. doi:10.1021/acsabm.3c01039
Wu, H., Shang, Y., Sun, W., Ouyang, X., Zhou, W., Lu, J., et al. (2023a). Seamless and early gap healing of osteochondral defects by autologous mosaicplasty combined with bioactive supramolecular nanofiber-enabled gelatin methacryloyl (BSN-GelMA) hydrogel. Bioact. Mater. 19, 88–102. doi:10.1016/j.bioactmat.2022.03.038
Wu, P., Xu, C., Zou, X., Yang, K., Xu, Y., Li, X., et al. (2024b). Capacitive-coupling-responsive hydrogel scaffolds offering wireless in situ electrical stimulation promotes nerve regeneration. Adv. Mater 36, e2310483. doi:10.1002/adma.202310483
Wu, S., Zhang, H., Wang, S., Sun, J., Hu, Y., Liu, H., et al. (2023b). Ultrasound-triggered in situ gelation with ROS-controlled drug release for cartilage repair. Mater. Horizons 10 (9), 3507–3522. doi:10.1039/D3MH00042G
Wu, Y., Wang, K., Huang, S., Yang, C., and Wang, M. (2017). Near-infrared light-responsive semiconductor polymer composite hydrogels: spatial/temporal-controlled release via a photothermal "sponge" effect. ACS Appl. Mater Interfaces 9 (15), 13602–13610. doi:10.1021/acsami.7b01016
Wu, Z., Yang, Z., Liu, L., and Xiao, Y. (2023c). Natural compounds protect against the pathogenesis of osteoarthritis by mediating the NRF2/ARE signaling. Front. Pharmacol. 14, 1188215. doi:10.3389/fphar.2023.1188215
Xiao, Q., Tong, Q. X., and Zhong, J. J. (2022). Recent advances in visible-light photoredox catalysis for the thiol-ene/yne reactions. Molecules 27 (3), 619. doi:10.3390/molecules27030619
Xu, C., Lin, T., Zhao, X., Gan, Y., Huang, J., Zhang, J., et al. (2023). Microenvironment responsive hypoxia-mimetic DFO composite hydrogel for on-demand neovascularization to promote tendon-to-bone healing. Compos. Part B Eng. 259, 110726. doi:10.1016/j.compositesb.2023.110726
Xue, X., Hu, Y., Deng, Y., and Su, J. (2021). Recent advances in design of functional biocompatible hydrogels for bone tissue engineering. Bone Tissue Eng. 31 (19), 2009432. doi:10.1002/adfm.202009432
Xue, X., Hu, Y., Wang, S., Chen, X., Jiang, Y., and Su, J. (2022a). Fabrication of physical and chemical crosslinked hydrogels for bone tissue engineering. Bioact. Mater 12, 327–339. doi:10.1016/j.bioactmat.2021.10.029
Xue, X., Zhang, H., Liu, H., Wang, S., Li, J., Zhou, Q., et al. (2022b). Rational design of multifunctional CuS nanoparticle-PEG composite soft hydrogel-coated 3D hard polycaprolactone scaffolds for efficient bone regeneration. Adv. Funct. Mater. 32 (33), 2202470. doi:10.1002/adfm.202202470
Yan, T., Rao, D., Chen, Y., Wang, Y., Zhang, Q., and Wu, S. (2022). Magnetic nanocomposite hydrogel with tunable stiffness for probing cellular responses to matrix stiffening. Acta Biomater. 138, 112–123. doi:10.1016/j.actbio.2021.11.001
Yan, X., Yang, B., Chen, Y., Song, Y., Ye, J., Pan, Y., et al. (2021). Anti-friction MSCs delivery system improves the therapy for severe osteoarthritis. Adv. Mater 33 (52), e2104758. doi:10.1002/adma.202104758
Yin, T., and Li, L. (2006). The stem cell niches in bone. J. Clin. Invest. 116 (5), 1195–1201. doi:10.1172/jci28568
Yoon, H., Kang, J. H., Cho, S. W., Park, C. G., Kim, D. W., and Park, T. E. (2024). Brain-decellularized ECM-based 3d myeloid sarcoma platform: mimicking adaptive phenotypic alterations in the brain. Adv. Healthc. Mater, e2304371. doi:10.1002/adhm.202304371
Yoon, J., Bian, P., Kim, J., McCarthy, T. J., and Hayward, R. C. (2012). Local switching of chemical patterns through light-triggered unfolding of creased hydrogel surfaces. Angew. Chem. Int. Ed. Engl. 51 (29), 7146–7149. doi:10.1002/anie.201202692
Yu, X., Deng, Z., Li, H., Ma, Y., and Zheng, Q. (2023). In situ fabrication of an anisotropic double-layer hydrogel as a bio-scaffold for repairing articular cartilage and subchondral bone injuries. RSC Adv. 13 (50), 34958–34971. doi:10.1039/d3ra06222h
Yu, Y., and Chau, Y. (2012). One-step "click" method for generating vinyl sulfone groups on hydroxyl-containing water-soluble polymers. Biomacromolecules 13 (3), 937–942. doi:10.1021/bm2014476
Yuan, K., Deng, C., Tan, L., Wang, X., Yan, W., Dai, X., et al. (2024). Structural and temporal dynamics analysis of zinc-based biomaterials: history, research hotspots and emerging trends. Bioact. Mater 35, 306–329. doi:10.1016/j.bioactmat.2024.01.017
Zeng, Y., Huang, C., Duan, D., Lou, A., Guo, Y., Xiao, T., et al. (2022). Injectable temperature-sensitive hydrogel system incorporating deferoxamine-loaded microspheres promotes H-type blood vessel-related bone repair of a critical size femoral defect. Acta Biomater. 153, 108–123. doi:10.1016/j.actbio.2022.09.018
Zhang, B., Zhang, M., Tian, J., Zhang, X., Zhang, D., Li, J., et al. (2024a). Advances in the regulation of radiation-induced apoptosis by polysaccharides: a review. Int. J. Biol. Macromol. 263, 130173. doi:10.1016/j.ijbiomac.2024.130173
Zhang, H., Gan, X., Yan, Y., and Zhou, J. (2024b). A sustainable dual cross-linked cellulose hydrogel electrolyte for high-performance zinc-metal batteries. Nanomicro Lett. 16 (1), 106. doi:10.1007/s40820-024-01329-0
Zhang, H., Mao, R., Yuan, L., Wang, Y., Liu, W., Wang, J., et al. (2024c). Near-infrared organic photodetectors with spectral response over 1200 nm adopting a thieno[3,4-c]thiadiazole-based acceptor. ACS Appl. Mater Interfaces 16, 9088–9097. doi:10.1021/acsami.3c15902
Zhang, J., Yang, Y., Chen, Y., Liu, X., Guo, S., Zhu, L., et al. (2016). An in situ phototriggered-imine-crosslink composite hydrogel for bone defect repair. J. Mater Chem. B 4 (5), 973–981. doi:10.1039/c5tb02377g
Zhang, K., Liu, Y., Zhao, Z., Shi, X., Zhang, R., He, Y., et al. (2024d). Magnesium-Doped nano-hydroxyapatite/polyvinyl alcohol/chitosan composite hydrogel: preparation and characterization. Int. J. Nanomedicine 19, 651–671. doi:10.2147/ijn.S434060
Zhang, K., Xue, K., and Loh, X. J. (2021a). Thermo-responsive hydrogels: from recent progress to biomedical applications. Gels 7 (3), 77. doi:10.3390/gels7030077
Zhang, P., Raza, S., Cheng, Y., Claudine, U., Hayat, A., Bashir, T., et al. (2024e). Fabrication of maleic anhydride-acrylamide copolymer based sodium alginate hydrogel for elimination of metals ions and dyes contaminants from polluted water. Int. J. Biol. Macromol. 261 (Pt 1), 129146. doi:10.1016/j.ijbiomac.2023.129146
Zhang, Q., Xu, H., Wu, C., Shang, Y., Wu, Q., Wei, Q., et al. (2021b). Tissue fluid triggered enzyme polymerization for ultrafast gelation and cartilage repair. Angew. Chem. Int. Ed. Engl. 60 (36), 19982–19987. doi:10.1002/anie.202107789
Zhang, S., Liang, R., Xu, K., Zheng, S., Mukherjee, S., Liu, P., et al. (2022). Construction of multifunctional micro-patterned PALNMA/PDADMAC/PEGDA hydrogel and intelligently responsive antibacterial coating HA/BBR on Mg alloy surface for orthopedic application. Mater Sci. Eng. C Mater Biol. Appl. 132, 112636. doi:10.1016/j.msec.2021.112636
Zhang, T., Chen, S., Dou, H., Liu, Q., Shu, G., Lin, J., et al. (2021c). Novel glucosamine-loaded thermosensitive hydrogels based on poloxamers for osteoarthritis therapy by intra-articular injection. Mater Sci. Eng. C Mater Biol. Appl. 118, 111352. doi:10.1016/j.msec.2020.111352
Zhang, Y., Cao, Y., Zhao, H., Zhang, L., Ni, T., Liu, Y., et al. (2020). An injectable BMSC-laden enzyme-catalyzed crosslinking collagen-hyaluronic acid hydrogel for cartilage repair and regeneration. J. Mater Chem. B 8 (19), 4237–4244. doi:10.1039/d0tb00291g
Zhang, Y., Li, G., Wang, J., Zhou, F., Ren, X., and Su, J. (2023). Small joint organoids 3D bioprinting: construction strategy and application. Small 20, e2302506. doi:10.1002/smll.202302506
Zhang, Z., Li, H., Kasmi, S., Van Herck, S., Deswarte, K., Lambrecht, B. N., et al. (2019). A synthetic, transiently thermoresponsive homopolymer with UCST behaviour within a physiologically relevant window. Angew. Chem. Int. Ed. Engl. 58 (23), 7866–7872. doi:10.1002/anie.201900224
Zhao, L., Niu, L., Liang, H., Tan, H., Liu, C., and Zhu, F. (2017). pH and glucose dual-responsive injectable hydrogels with insulin and fibroblasts as bioactive dressings for diabetic wound healing. ACS Appl. Mater. Interfaces 9 (43), 37563–37574. doi:10.1021/acsami.7b09395
Zhao, Z., Xia, X., Liu, J., Hou, M., Liu, Y., Zhou, Z., et al. (2024). Cartilage-inspired self-assembly glycopeptide hydrogels for cartilage regeneration via ROS scavenging. Bioact. Mater 32, 319–332. doi:10.1016/j.bioactmat.2023.10.013
Zheng, H., Xie, X., Ling, H., You, X., Liang, S., Lin, R., et al. (2023). Transdermal drug delivery via microneedles for musculoskeletal systems. J. Mater Chem. B 11 (35), 8327–8346. doi:10.1039/d3tb01441j
Zheng, Y., Chen, Z., Jiang, Q., Feng, J., Wu, S., and del Campo, A. (2020). Near-infrared-light regulated angiogenesis in a 4D hydrogel. Nanoscale 12 (25), 13654–13661. doi:10.1039/D0NR02552F
Zhong, Y., Ning, S., Wu, K., Li, Z., Wang, X., He, C., et al. (2024). Novel phosphate functionalized sodium alginate hydrogel for efficient adsorption and separation of Nd and Dy from Co. J. Environ. Manage 353, 120283. doi:10.1016/j.jenvman.2024.120283
Zhou, H., Zhu, Y., Yang, B., Huo, Y., Yin, Y., Jiang, X., et al. (2024a). Stimuli-responsive peptide hydrogels for biomedical applications. J. Mater Chem. B 12, 1748–1774. doi:10.1039/d3tb02610h
Zhou, K., Sun, R., Wojciechowski, J. P., Wang, R., Yeow, J., Zuo, Y., et al. (2024b). 4D multi-material printing of soft actuators with spatial and temporal control. Adv. Mater, e2312135. doi:10.1002/adma.202312135
Zhou, S., Xiao, C., Fan, L., Yang, J., Ge, R., Cai, M., et al. (2024c). Injectable ultrasound-powered bone-adhesive nanocomposite hydrogel for electrically accelerated irregular bone defect healing. J. Nanobiotechnology 22 (1), 54. doi:10.1186/s12951-024-02320-y
Zhu, G., Zhang, T., Chen, M., Yao, K., Huang, X., Zhang, B., et al. (2021). Bone physiological microenvironment and healing mechanism: basis for future bone-tissue engineering scaffolds. Bioact. Mater 6 (11), 4110–4140. doi:10.1016/j.bioactmat.2021.03.043
Keywords: exogenous stimulus, endogenous stimulus, in situ hydrogels, smart hydrogels, bone tissue engineering
Citation: Wu S, Gai T, Chen J, Chen X and Chen W (2024) Smart responsive in situ hydrogel systems applied in bone tissue engineering. Front. Bioeng. Biotechnol. 12:1389733. doi: 10.3389/fbioe.2024.1389733
Received: 22 February 2024; Accepted: 15 April 2024;
Published: 28 May 2024.
Edited by:
Ana Claudia Oliveira Carreira, Federal University of ABC, BrazilCopyright © 2024 Wu, Gai, Chen, Chen and Chen. This is an open-access article distributed under the terms of the Creative Commons Attribution License (CC BY). The use, distribution or reproduction in other forums is permitted, provided the original author(s) and the copyright owner(s) are credited and that the original publication in this journal is cited, in accordance with accepted academic practice. No use, distribution or reproduction is permitted which does not comply with these terms.
*Correspondence: Jie Chen, MTc4MTY1MDYwODhAMTYzLmNvbQ==; Xiguang Chen, eGdjaGVuQG91Yy5jZHUuY24=; Weikai Chen, d2Vpa2FpY2hlbkBzaHUuZWR1LmNu
Disclaimer: All claims expressed in this article are solely those of the authors and do not necessarily represent those of their affiliated organizations, or those of the publisher, the editors and the reviewers. Any product that may be evaluated in this article or claim that may be made by its manufacturer is not guaranteed or endorsed by the publisher.
Research integrity at Frontiers
Learn more about the work of our research integrity team to safeguard the quality of each article we publish.