- School of Grain Science and Technology, Jiangsu University of Science and Technology, Zhenjiang, China
Valorization of food processing effluent (FPE) by microalgae cultivation for astaxanthin production is regarded as a potential strategy to solve the environmental pollution of food processing industry and promote the development of eco-friendly agriculture. In this review paper, microalgal species which have the potential to be employed for astaxanthin in FPE were identified. Additionally, in terms of CO2 emission, the performances of microalgae cultivation and traditional methods for FPE remediation were compared. Thirdly, an in-depth discussion of some innovative technologies, which may be employed to lower the total cost, improve the nutrient profile of FPE, and enhance the astaxanthin synthesis, was provided. Finally, specific effects of dietary supplementation of algal astaxanthin on the growth rate, immune response, and pigmentation of animals were discussed. Based on the discussion of this work, the cultivation of microalgae in FPE for astaxanthin production is a value-adding process which can bring environmental benefits and ecological benefits to the food processing industry and agriculture. Particularly, technological innovations in recent years are promoting the shift of this new idea from academic research to practical application. In the coming future, with the reduction of the total cost of algal astaxanthin, policy support from the governments, and further improvement of the innovative technologies, the concept of growing microalgae in FPE for astaxanthin will be more applicable in the industry.
1 Introduction
As a ketocarotenoid with superior antioxidative activity, astaxanthin (3,3′-dihydroxy-β, β-carotene-4,4′-dione), has been widely used in cosmetics, animal feed, and healthcare food (Wang et al., 2013; Zhao et al., 2019b; Lu et al., 2021). In recent years, some microalgal strains, such as Chlorella zofingiensis, Haematococcus lacustris (former name Haematococcus pluvialis), and Chlorella sorokiniana, have been employed for the production of natural astaxanthin (Lu et al., 2021). The research interests cover the artificial mutagenesis of algal strains, optimization of environmental factors, extraction of astaxanthin from algae biomass, antioxidative functions of algal astaxanthin and so on (Xie et al., 2018; Fernando et al., 2021; Szczepanik et al., 2022). In a real-world application, however, high cost of natural astaxanthin production by microalgae cultivation has not been fully addressed. In the market, high sale price (US $2,000–2,500 per kilogram) of natural astaxanthin is hindering the wide application of this valuable bio-product in modern agriculture (Liu et al., 2013; Lu and Lu, 2022).
The fast growths of global population and food production are accompanied with the generation of a high volume of food processing effluent (FPE) enriched with organics (Yang K. et al., 2015; Haque et al., 2017). For example, the production of 1 ton palm oil could generate 2.5–3.0 m3 FPE and the processing of 1 ton soybean for bean curd production can yield 7–10 tons of wastewater (Li S. et al., 2019; Chong et al., 2021). Traditionally, FPE is treated by physical filtration, aeration, anaerobic digestion, and/or chemical oxidation, yielding a large amount of sludge and greenhouse gases (CO2 and CH4) (Leifeld et al., 2018; Aziz et al., 2019; Asgharnejad et al., 2021). Compared to the traditional methods, algae-based FPE treatment, which could convert the wastewater-borne nutrients to algal biomass, has much less carbon footprint and more economic benefits. In fact, due to the excellent performance of microalgae in wastewater treatment and water recycling, microalgae cultivation has been regarded as a promising strategy to address the water pollution in agriculture, aquaculture, and industry, achieving the United Nations Sustainable Development Goal 6-“Ensure access to water and sanitation for all” (Oliveira et al., 2022; Olabi et al., 2023). Therefore, to promote the eco-friendly development of food industry, valorization of the FPE by microalgae cultivation for high-value components production is emerging into the limelight (Nishshanka G. K. S. H. et al., 2021).
To support the wide use of astaxanthin in agriculture, the safety of the harvested algal biomass is an important concern. Compared to other wastewater, such as mining effluent, chemical wastewater, and municipal wastewater, FPE contains no or much less toxic components (Lu et al., 2015; Lu et al., 2016). Accordingly, astaxanthin-rich microalgae cultivated in FPE would not be contaminated by heavy metals or chemical toxins. On one hand, in the FPE, due to the absence of toxic components, the growth of microalgae would not be seriously limited. On the other hand, biomass containing no toxic components could be widely used in agriculture. Otherwise, the toxic components may threaten human’s health through the accumulation in food chain. Microalgae have been successfully used to recover nutrients from food wastes or digested food wastes. According to previous studies, high removal efficiency of nutrient (nitrogen, phosphorus, ammonia, organic carbon, etc.) in different types of FPEs, such as starch processing wastewater, raw cheese whey, and household food waste, was achieved by microalgae cultivation (Schanes et al., 2018; Chuka-ogwude et al., 2020). Hence, it has been fully verified that it is a practical way to cultivate microalgae for nutrient recovery from FPE and high-value ingredient production.
In addition to the microalgae-based astaxanthin production, the application of astaxanthin-rich biomass to feed livestock, fish, shrimp, and poultry in agricultural is becoming increasingly popular. Recently, more and more beneficial effects of algal astaxanthin on animals’ health have been reported (Xie et al., 2018; Xie et al., 2020). For example, it was discovered that daily supplementation of astaxanthin-rich microalgae in diet could enhance the growth of poultry, livestock, and fish (Kumar et al., 2019; Xie et al., 2020; Awadh and Zangana, 2021). Besides, the immune response of animals can be improved by the addition of astaxanthin in diet (Xie et al., 2018). In this case, the health problems caused by the overuse of antibiotics and medicines in animal feeds can be solved. Algal astaxanthin could also enhance the pigmentation, which one of the most important quality criteria dictating the market value, of some animals, particularly poultry and fish (Vissio et al., 2021). Hence, the practical application of microalgal astaxanthin is of importance to the development of eco-friendly and value-added agriculture.
The optimization of environmental factors to induce the astaxanthin biosynthesis of algae grown in wastewater has been summarized in many previous review papers (Lu et al., 2021; Basiony et al., 2022). In terms of microalgae-based FPE treatment for astaxanthin production, there are some other interesting and important questions. Firstly, what are the microalgal species that can be employed for simultaneous nutrient recovery and astaxanthin production in FPE? Secondly, what are advantages of microalgae-based FPE treatment over traditional treatment methods in terms of profitability and carbon footprint? Thirdly, are there some technological innovations that can enhance the biomass production and lower the total cost of microalgae-based FPE treatment? Fourthly, what are the downstream applications of astaxanthin-rich microalgae in animals farming? This review paper is to provide answers to the aforementioned questions by summarizing the recent progresses and conducting in-depth analysis. It is expected that this review paper can help readers understand the promising prospects of the algae-based astaxanthin production in FPE and the downstream application of algal astaxanthin in agriculture.
2 Microalgal species for astaxanthin production in food processing effluent
In nature, there are a number of microalgae which could synthesize astaxanthin, but only a few algal species, such as H. lacustris, Chlorococcum sp., C. sorokiniana, and C. zofingiensis, have been intensively studied (Zhang and Lee, 2001; Chen et al., 2009; Raman and Mohamad, 2012; Wang et al., 2013). Some microalgae, such as Oedocladium carolinianum, Monoraphidium sp., Coelastrum sp., Bracteacoccus aggregatus, and Coelastrella rubescens are able to synthesize astaxanthin although they have not been widely commercialized (Table 1). Some of the newly isolated astaxanthin-rich microalgae are found to have high possibility of commercial application. For example, B. aggregatus isolated from the White Sea coastal zone (Russia) has a very high percentage (48%) of astaxanthin in the total carotenoids (Chekanov et al., 2021). In the future, with the technological development, more microalgal strains with the capacity of synthesizing astaxanthin will be found in the nature.
In the industrial application, to select the most appropriate microalgal strain for astaxanthin production in FPE, three factors, including the source of microalgae, astaxanthin content and resistance to harsh environment, should be taken into consideration. Firstly, in the practice, microalgal strains which could be obtained from commercial organizations are more likely to be widely used. Table 1 shows that the some algal strains, including H. lacustris, C. sorokiniana, and C. zofingiensis have been preserved by some international commercial organizations, enabling the wide application of these microalgae for astaxanthin production in the industry. Secondly, to achieve high profitability of microalgae-based astaxanthin production, algal strains with higher content of astaxanthin are preferred. Due to the different metabolic pathways and growth conditions, astaxanthin content in microalgae varies. As shown in Table 1, astaxanthin yield in H. lacustris could reach 30.94 mg L-1 while that in Tetraselmis sp. was only 5.0 mg L-1. Low content of astaxanthin in microalgae not only results in the low productivity, but also increases the cost of astaxanthin extraction. Thirdly, algal strains with high resistance to the harsh environment are more likely to grow well in FPE and convert wastewater-borne nutrients to high-value components. In contrast, the cultivation of unrobust microalgae in FPE may result in the failure of wastewater treatment and astaxanthin production.
Based on the criteria mentioned above, in the view of the present authors, H. lacustris, and C. zofingiensis could be regarded as promising algal strains for FPE treatment and astaxanthin production. Firstly, H. lacustris and C. zofingiensis have been successfully commercialized and they can be easily purchased from some international commercial organizations (Pelah et al., 2004; Wang et al., 2013). Besides, H. lacustris and C. zofingiensis, which have been intensively studied in academic research, could be obtained from the universities or research institutes (Table 1). Since H. lacustris and C. zofingiensis could be obtained from diverse sources of microalgal strains, they have the potential of being cultivated for astaxanthin production in the industry. Secondly, H. lacustris and C. zofingiensis contain high yields of astaxanthin, reaching 30.94 and 36.68 mg L-1, respectively (Liu et al., 2016; Christian et al., 2018). Compared with other microalgal strains (Tetraselmis sp., Chlorococcum sp., C. sorokiniana, etc.), H. lacustris and C. zofingiensis have great advantages in the astaxanthin content and astaxanthin yield (Table 1). Thirdly, H. lacustris and C. zofingiensis could grow well in a variety of waste streams, confirming their high resistance to harsh environment in wastewater (Table 2). For example, it was reported that the tolerance level of Chlorophyceae, to which H. lacustris and C. zofingiensis belong, to ammonia toxicity is 23.758 mM, which is much higher than those of Bacillariophyceae (also known as Diatomophyceae), Dinophyceae, Prymnesiophyceae, and Raphidophyceae (Collos and Harrison, 2014). Therefore, H. lacustris and C. zofingiensis are regarded as promising microalgal strains for simultaneous FPE remediation and astaxanthin production.
In addition to the natural microalgae strains mentioned above, some genetically engineered microalgae are able to produce astaxanthin. Through introducing genes regulating astaxanthin biosynthesis into the microalgal chassis cells, some microalgae, such as Synechocystis sp. and Chlamydomonas reinhardtii, with the capacity of synthesizing astaxanthin were obtained (Liu et al., 2019; Liang et al., 2023). As reported by the previous study, genetically engineered Synechocystis sp. contained high content of astaxanthin, reaching 29.6 mg g-1 (dry cell weight) (Lu et al., 2023b). Hence, the development of genetic modification techniques is bringing new prospects of microalgae-based astaxanthin production. However, it should be noted that due to the potential safety risks of genetic modification, genetically engineered microalgae are studied in lab research at present while have not been widely used in the industrial application.
3 Microalgae-based nutrient recovery in food processing effluent
3.1 Growth of astaxanthin-rich microalgae for FPE treatment
As the effluent of food processing industry, FPE is enriched with a variety of organics, such as carbohydrate, oil, protein hydrolysate, etc. As shown in Table 3, compared with the artificial mixotrophic medium, some FPEs contain much higher concentrations of total organic carbon (TOC), chemical oxygen demand (COD), total nitrogen (TN), total ammonia nitrogen (TAN), and total phosphorus (TP). Therefore, FPE may cause serious environmental pollution if it is not treated properly. With the discharge of FPE into natural waters, the enrichment of nutrients, including organic carbon, nitrogen, and phosphorus and so on, in natural waters will cause the water eutrophication (Preisner et al., 2021). As a consequence, the occurrence of algal bloom followed by eutrophication reduces dissolved oxygen content, releases toxins, and disturbs the ecological balance (Misra et al., 2011; Watson et al., 2016). Also, FPE can be regarded as a nutrient-rich substrate for microalgae cultivation. In this case, microalgae-based FPE remediation could not only attenuate the potential environmental pollution, but also yield high-value biomass, generating both environmental benefit and economic benefit.
Compared with other algal bio-products, such as bio-oil and single-cell protein, algal astaxanthin has higher values in the market. In recent years, therefore, robust algal strains, particularly H. lacustris and C. zofingiensis, have been intensively cultivated in FPE for astaxanthin production. As presented in Table 2, productivity of algal astaxanthin in some FPEs could reach over 20 mg g-1, confirming the feasibility of using FPE as a substrate for microalgae cultivation and astaxanthin production (Pan et al., 2021; Yu et al., 2021).
According to previous studies, high concentration of organic carbon and high ratio of C/N are favorable to the biosynthesis of astaxanthin in algal cells (Lu et al., 2021; Lu and Lu, 2022). Firstly, organic matters, such as sugars and acids, are added in artificial medium to promote the synthesis of algal astaxanthin (Ip and Chen, 2005; Chen et al., 2009). It was discovered that with the increase of glucose content in medium from 5 g L-1–50 g L-1, astaxanthin yield of C. zofingiensis was improved from 0.76 mg L-1–10.29 mg L-1 (Ip and Chen, 2005). It should be noted that in FPE, some organic matters are not in the forms of sugars or acids and some organic matters are even could not be directly assimilated by algal cells (Lu et al., 2015). Hence, conversion of organic matters in FPE to digestible sugars or acids is of importance to the production of algal astaxanthin. Secondly, astaxanthin formation is accompanied with the encystment induced under the high ratio of C/N (Kakizono et al., 1992; Li et al., 2022). Traditionally, to grow microalgae for astaxanthin production, low concentration of nitrate, ammonia, or organic nitrogen was added in artificial medium (Lu and Lu, 2022). As shown in Table 3, however, many FPEs, such as soybean processing effluent and meat processing effluent, contain high concentration of TN. Accordingly, in FPEs with low ratios of C/N, the synthesis of algal astaxanthin may be hindered. To achieve high productivity of microalgae biomass, nutrient profiles of some FPEs should be modified properly.
Based on the discussion above, FPEs contain essential nutrients for microalgae cultivation, but some unfavorable factors, such as the high contents of indigestible nutrients and high ratio of N/C, impact the biomass production, nutrient recovery, and astaxanthin accumulation, hindering the microalgae-based astaxanthin production in FPEs.
3.2 Fate of carbon in FPE treatment
In recent years, to prevent the exacerbation of climatic disasters caused by the greenhouse effect, governments are taking measures to reduce the carbon footprint of industries, including the wastewater treatment industry. Carbon neutrality has become one of the development targets of most countries in the world (Jia and Lin, 2021).
Figure 1 demonstrates the fates of carbon in FPE treated by chemical oxidation or microalgae cultivation. In this work, to intuitively demonstrate the amount of carbon emission during the treatment of FPE, a wastewater treatment plant with a daily capacity of 1,000 m3 FPE is used as an example. Normally, such a wastewater treatment plant could treat the FPE produced by a large-sized food processing factory. Some data in previous studies were used to estimate the fate of carbon in FPE treatment (Lu et al., 2023b; Fajardo-Puerto et al., 2023). Concentration of organic carbon in FPE was set as 2,780 mg L-1. In the Fenton-based FPE treatment, the removal efficiency of organic carbon was set as 85%. In the microalgae-based FPE remediation, removal efficiency of organic carbon, biomass yield, and carbon content in biomass were set as 88.60%, 4.42 g L-1, and 45%, respectively.
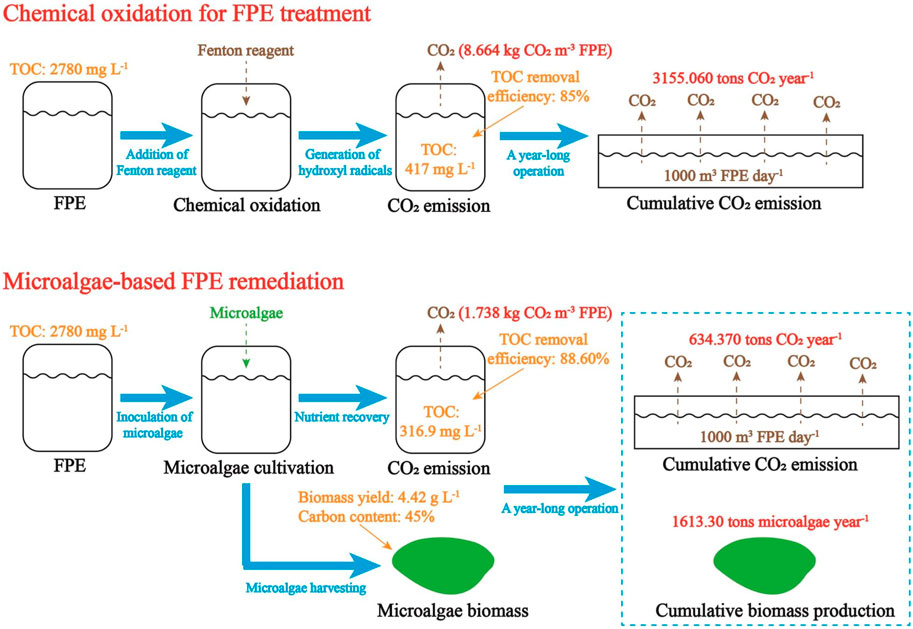
Figure 1. The fates of carbon in wastewater treatment based on chemical oxidation and microalgae cultivation (A wastewater treatment plant with a daily capacity of 1,000 m3 FPE is used as an example for the estimation of carbon emission and two different treatment technologies, traditional chemical oxidation and microalgae-based remediation, are compared).
As shown in Figure 1, in Fenton reaction, a typical chemical oxidation for organics-rich effluent treatment, with the generation of hydroxyl radicals (OH•), organic carbon contained in FPE is converted to CO2 (Fajardo-Puerto et al., 2023). Accordingly, 8.664 kg CO2, which is equal around 4.382 m3 CO2 to at 20°C, could be produced during the treatment of 1 m3 FPE. In a traditional wastewater treatment plant (WWTP) with a treatment capacity of 1,000 m3 FPE day-1, 3155.060 tons CO2 would be produced annually. Therefore, the contribution of CO2 emission in FPE treatment to the greenhouse effect is not neglectable.
According to the parameters mentioned above, in algae-based FPE remediation, a high portion of organic carbon was converted to biomass while CO2 emission was only 1.738 kg m-3. If this eco-friendly strategy is implemented in the WWTP to treat 1,000 m3 FPE daily, annual CO2 emission would be 634.370 tons and 1613.30 tons high-value biomass would be produced each year (Figure 1). In this case, CO2 emission of microalgae-based FPE remediation is 79.89% lower than that of FPE treatment by chemical oxidation. Accordingly, microalgae-based FPE remediation with much less CO2 emission could be regarded as a more eco-friendly and profitable strategy to solve the pollution caused by organics-rich effluent from food industry.
4 Technological innovations for microalgae-based FPE treatment
Microalgae-based astaxanthin production in FPE is an eco-friendly and economic strategy for waste resource recycling and high-value compound production. However, it is challenged by a couple of problems, including low removal efficiency of nutrient, high cost of biomass harvesting, and ammonia toxicity, in the practice. Firstly, due to the enrichment of undissolved organics in wastewater, in some cases, nutrient removal efficiency in microalgae-based FPE remediation is not high. For example, H. lacustris only removed 39.18% COD, 52.15% TN, and 45.46% TP in walnut shell extracts during the 13-day treatment (Yu et al., 2021). Under this situation, FPE after treatment still contains high concentrations of residual nutrients, which not only cause secondary pollution, but also result in underutilization of resource (Hu et al., 2021). Secondly, microalgal cells suspended in culture media or waste stream were mainly harvested by centrifugation, filtration, or Al3+-based flocculation (Matter et al., 2019; Najjar and Abu-Shamleh, 2020). In the practice, high energy consumption of centrifugation would dramatically increase the total cost of algal biomass and addition of Al3+ may limit the use of algal biomass in food and feed industry (Macías-Montes et al., 2021). Thirdly, high concentration of ammonia in some FPEs could negatively impact the growth of microalgae or even cause the failure of microalgae cultivation. To attenuate ammonia toxicity and utilize FPEs as the substrate for algae production, dilution and ammonia stripping were widely applied by previous studies (Fernando et al., 2021; Lu et al., 2023a). Nevertheless, due to the high consumption of freshwater and intensive emission of ammonia, the aforementioned two methods could not be regarded as an eco-friendly or sustainable way for microalgae-based FPE remediation.
Fortunately, in recent years, innovative technologies are developed to enhance the nutrient removal, simplify the biomass harvesting process, and alleviate the ammonia toxicity in wastewater. In this section, co-cultivation of microalgae with heterotrophic microorganisms, immobilization of microalgae by biofilm, and zeolite-based ammonia adsorption, which might promote the practical application of microalgae-based FPE valorization and astaxanthin production, were introduced.
4.1 Co-culture of microalgae with heterotrophic microorganisms
Nutrient removal efficiency is one of the important parameters, which are employed to assess the performance of wastewater remediation. It has been discovered that the co-culture of microalgae with heterotrophic microorganisms, particularly bacteria, yeast, and fungi, could promote the organics decomposition and nutrients removal in wastewater remediation (Liu et al., 2017; Lu et al., 2023b). As shown in Figure 2, the synergistic cooperations between microalgae and heterotrophic microorganisms mainly include the interspecies exchange of O2 and CO2, organics decomposition accelerated by extracellular enzymes, and secretion of growth promoting substances (Lee C. et al., 2019). Firstly, the interspecies exchange of O2 and CO2 creates a “comfortable” environment for the growth of microalgae and heterotrophic microorganisms. In the co-culture system, CO2 produced by heterotrophic microorganisms could be captured by microalgae for photosynthesis while O2 released by microalgae is essential to the metabolisms of heterotrophic microorganisms (Lu et al., 2023a; Lu et al., 2023b). In contrast, without the interspecies exchange of O2 and CO2 between microalgae and heterotrophic microorganisms, algal cells would assimilate the atmospheric CO2, of which the low concentration and dissolution rate may limit the photosynthesis and biomass production. Secondly, some extracellular enzymes, such as protease, amylase, and lipase, secreted by bacteria and fungi can accelerate the organics decomposition, converting high-molecular-weight nutrients to low-molecular-weight nutrients. Accordingly, in the wastewater, an increasing amount of digestible nutrients will be available to microalgae. It should be noted that with the organics decomposition, the presence of some sugars and acids (glucose, fulvic acid, etc.) in wastewater could increase the astaxanthin yield of microalgae (Zhao et al., 2019a; Zhang et al., 2019). Thirdly, some bacteria and fungi could secret growth-promoting substances, which can increase the biomass yield of microalgae. For example, an auxin-producing symbiotic bacterium, Achromobacter sp., with the function of enhancing H. lacustris growth at all growth stages by secreting indole-3-acetic acid was isolated (Lee C. et al., 2019). Therefore, it is a feasible strategy to enhance the astaxanthin production of microalgae grown in FPEs by co-culturing microalgae and bacteria/fungi with growth-promoting functions.
In the academic research, the positive effects of co-culture of microalgae with some heterotrophic microorganisms on nutrient removal and astaxanthin production have been documented (Jiang et al., 2018). The practical application of this innovative strategy, however, is challenged by a couple of potential problems. For example, bacteria with fast reproduction rate may compete with microalgae for nutrients in FPEs, resulting in low yield of algal biomass and astaxanthin. In some cases, the fast growth of bacteria or fungi may even lead to the failure of microalgae cultivation in FPEs. Additionally, in the industry, the traditional method of analyzing bacterial growth trend by quantifying colony-forming unit (CFU) is time-costing while it is expensive to conduct real-time monitoring of the bacterial growth trend in wastewater remediation. Therefore, in the coming future, by realizing the real-time monitoring of bacterial growth at low cost, co-cultivation of microalgae (H. lacustris and C. zofingiensis) in FPEs for astaxanthin production might become more and more applicable in the industry.
4.2 Immobilization of microalgae on biofilm
In the past, most studies treated wastewater by directly inoculating the suspended microalgae cells while rarely focused on the utilization of immobilized microalgae cells for wastewater treatment. However, in the practical application, wastewater treatment by suspended microalgae is challenged by the high cost and high energy consumption of biomass harvesting. It was reported that energy consumption of centrifugation and filtration for microalgae harvesting could reach 1.43 and 1.22 kWh m-3, respectively (Najjar and Abu-Shamleh, 2020). Accordingly, the cost of microalgae harvesting is high, accounting for about 30% of the total cost of microalgae cultivation (Hu et al., 2021).
To use the algal astaxanthin as an ingredient of animal feed, the total cost of microalgae should be maintained at a low level and the safety of the harvested biomass must be improved. Recently, revolving algal biofilm (RAB), which can realize the immobilization of algal cells, is developed as an innovative method for microalgae cultivation and harvesting (Hu et al., 2021). In the RAB, microalgae are attached and grow on the surface of substratum (cotton duct, cotton rag, cotton denim, etc.) and the biomass harvesting can be conducted by using scrappers (Gross et al., 2013; Gross et al., 2015; Hu et al., 2021). Compared to centrifugation and filtration, RAB has much lower energy consumption for biomass harvesting and yields microalgal biomass with low cost. Therefore, RAB, which attracts attentions from researchers in both academia and industry, is being intensively studied and applied in the industry (Gross et al., 2013; Hu et al., 2021).
The formation of microalgal biofilm on substratum is mainly consisted of three steps (Figure 2). Firstly, microalgal cells are attached on the rough surface of substratum when the attractive van der Waals and acid-base interactions overcome the repulsive electrostatic interactions (Hu et al., 2021). In the practice, to enhance the adsorption of microalgal cells, substratum materials with rougher surface and more binding sites are preferred. Hence, many previous studies devoted a lot efforts to selecting the most appropriate substratum materials for microalgae adsorption (Shen et al., 2014; Ji et al., 2023). Secondly, microalgae attached on the surface of substratum could secret extracellular polymeric substances (EPS), which can act as “glue” in the formation of microalgae matrix (Hu et al., 2021). In this process, the presence of EPS could promote the construction of polymer network and improve the physical stability of microbial matrix on the surface substratum. Thirdly, with the gradual colonization of bacteria and filamentous fungi, the interaction between microalgae and substratum will be enhanced and the biofilm thickening process will be accelerated.
Up to now, RAB has been adopted to cultivate microalgae for nutrients recovery in a variety of waste streams, synthetic wastewater and artificial media. For example, in the trough-based RAB, maximum surface biomass productivity and footprint biomass productivity of Chlorella vulgaris reached 5.5 g m-2 day-1 and 46.8 g m-2 day-1, respectively (Gross et al., 2015). In terms of footprint biomass productivity, RAB, which can make full use of vertical space, has much better performance than traditional cultivation systems (Yu et al., 2024). In addition, since microalgae attached on substratum could be harvested easily by using scrappers and the harvested biomass has low moisture content, the cost of biomass harvesting and thickening can be reduced remarkably. Therefore, immobilization of microalgae by RAB to improve the footprint biomass productivity and simplify the biomass harvesting process is regarded as a promising strategy to upgrade microalgae-related industry and promote the commercialization of algal bioproducts.
Previous studies confirmed the feasibility of growing cylindrical or spherical microalgae, such as Tetradesmus obliquus (former name Scenedesmus obliquus) and C. vulgaris, on substratum for the construction of algal biofilm (Gross et al., 2015; Nguyen et al., 2023). The cells of H. lacustris and C. zofingiensis are also spherical, having similar size and structure with the cells of T. obliquus and C. vulgaris. In addition, both H. lacustris and C. zofingiensis have the ability to synthesize and secret EPS, which is one of the key compounds during the formation of algal biofilm (Delattre et al., 2016; Gorgich et al., 2021). Therefore, theoretically, H. lacustris and C. zofingiensis could be cultivated on RAB for astaxanthin production. Since RAB and relevant technologies are recently developed, up to now, few studies have comprehensively evaluated the astaxanthin production in RAB. In the future, experimental studies should be conducted to evaluate the specific effects of microalgae immobilization on astaxanthin synthesis and accumulation in RAB, providing practical guidance to the astaxanthin production.
Although RAB has obvious advantages over conventional microalgae cultivation modes, its drawbacks, such as washout of microalgal cells and poor penetration of light, should not be neglected. Due to the water flow and mechanical vibration, a portion of microalgal cells attached on the surface of biofilm substratum may fall into the wastewater (Zhao et al., 2018). With the discharge of FPE after the microalgae-based treatment, the algal cells suspended in FPE enter the natural waters. Hence, the occurrence of microalgae washout during the continuous operation of RAB not only lowers the final yield of biomass, but also causes potential biological invasion (Hu et al., 2021). In addition, thick layer of microalgae on biofilm substratum limits the penetration of light and the microalgal cells at the inner layer would not receive sufficient light. Accordingly, the insufficient illumination may negatively impact the synthesis of astaxanthin in the microalgal cells at the inner layer. Up to now, few studies have developed applicable methods to address the aforementioned drawbacks of RAB. In the view of the present authors, in the coming future, methods to trigger the secretion of EPS of microalgae could be employed to enhance the stickiness of the algal biofilm and prevent the washout of microalgal cells. Besides, artificial intelligence (AI) technology may be adopted to control the thickness of algal biofilm and promote the penetration of light.
4.3 Zeolite-based ammonium adsorption and desorption
It has been widely documented that high C/N ratio in culture media or waste stream is of importance to the induction of astaxanthin synthesis in microalgal cells (Río et al., 2005; Lu and Lu, 2022). Accordingly, FPEs with high concentration of TN, particularly TAN, were rarely utilized for microalgae-based astaxanthin production. In addition, during the algae growth process, with the exhaustion of ammonia in FPEs, extremely low concentration of nitrogen in the later stage may be unfavorable to algal cells. Therefore, to widely apply FPEs as an alternative substrate for microalgae cultivation and astaxanthin production, the initial concentration of TN and the ratio of C/N in FPEs should be regulated properly.
In the past, to attenuate ammonia toxicity or adjust the ratio of C/N in wastewater, some methods, such as dilution by freshwater and ammonia stripping, have been developed (Li J. et al., 2019; Lu et al., 2023b). However, in the practical application, these traditional methods are hindered by a couple of problems. Firstly, concentrations of TN and TAN in waste stream or culture media can be effectively reduced via dilution. However, dilution by freshwater not only reduce the concentrations of TAN and TN, but also lower the concentration of TOC in waste stream or culture media. As a result, the ratio of C/N in FPEs may not be improved obviously and the astaxanthin synthesis in microalgae will not be positively impacted. Besides, dilution, which consumes a large volume of freshwater, can not be regarded as an eco-friendly and sustainable method for the pretreatment of FPEs (Lu et al., 2023a). Secondly, as a process releasing dissolved ammonia into atmosphere, ammonia stripping can effectively reduce the concentrations of TN and TAN in FPEs while have no obvious effect on the concentration of TOC. This method, hence, can be employed to increase the ratio of C/N in FPEs, creating a suitable environment for microalgae-based astaxanthin production. However, ammonia stripping is accompanied with intensive emission of ammonia, not only reducing the utilization efficiency of TN in FPE, but also cause air pollution (Lu et al., 2023b). Besides, due to the low concentrations of TN and TAN in FPE after ammonia stripping, microalgae may suffer from the nitrogen deficiency in the later stage of exponential phase. Because of the disadvantages mentioned above, technological innovation is needed to realize the microalgae-based astaxanthin production in FPE.
Zeolite is a porous material which can effectively adsorb the ionized ammonium (NH4+) when the concentration of ammonium in water is high while slowly release NH4+ when ammonium is exhausted. Firstly, at the initial stage, with the addition of zeolite in FPE, NH4+ is adsorbed and the concentrations of TAN and TN would be dramatically reduced. As reported by previous study, when zeolite was added in wastewater (960–1,080 mg L-1 TAN) at a density of 125 g L-1, after 15-h, 71.27% of TAN was transferred into zeolite, resulting in a low concentration of TAN (285 mg L-1). Accordingly, the ratio of TOC to TAN in wastewater was remarkably increased from 4.83 to 16.77 (Lu et al., 2023a). Hence, with the addition of zeolite, ammonia toxicity could be attenuated and FPE with high C/N ratio is more favorable to the astaxanthin synthesis in algal cells. Secondly, at the later stage, with the gradual consumption of TAN and further increase of C/N ratio, NH4+ is released from zeolite to maintain the C/N ratio in FPE stable. In our previous study, due to the slow-release of NH4+ by zeolite, TAN concentration in media was maintained stably in a range of 40–50 mg L-1 at the later stage (Day 8 to Day 18) (Lu et al., 2019). Accordingly, the limiting effect of ammonia exhaustion on algal cells can be alleviated, resulting high yields of algal biomass and astaxanthin. Based on the mechanisms and experimental data mentioned above, zeolite-based ammonium adsorption and desorption is regarded as a promising strategy to promote the practical application of FPE for microalgae cultivation and astaxanthin production.
Up to now, dynamics of zeolite-based ammonium adsorption and desorption have been intensively studied and this novel strategy has been successfully implemented to regulate the C/N ratio for Arthrospira platensis, Amphidinium carterae, and C. vulgaris cultivation in waste streams (Markou et al., 2014; López-Rosales et al., 2022; Lu et al., 2023a). It was reported that due to the improvement of C/N ratio in wastewater, oil yield and oil content of C. vulgaris were improved to 1.24 g L-1 and 34.6%, respectively (Lu et al., 2023a). These results fully confirmed the practical feasibility of enhancing the biosynthesis of certain algal compounds by zeolite-based ammonium adsorption and C/N adjustment.
Due to the close relation between C/N ratio and astaxanthin synthesis, zeolite-based ammonium adsorption and desorption can play a key role in the microalgae-based FPE remediation for astaxanthin production. Theoretical procedures are shown in Figure 2. Firstly, based on the optimal C/N ratio for astaxanthin in microalgae, the adding amount and adsorption time of zeolite in FPE should be identified. At this step, physical structure of zeolite, pH of wastewater, temperature, and concentrations of ions in wastewater can impact the dynamic equilibrium and adsorption rate of ammonium. Secondly, by the end of ammonium adsorption, microalgae are inoculated in FPE for nutrient assimilation and astaxanthin production. With the gradual consumption of TAN by microalgae, adsorbed ammonium is released from zeolite into FPE, continuously maintaining the TAN concentration and C/N ratio in wastewater. Thirdly, by the end of FPE remediation, algal biomass enriched with astaxanthin is harvested for downstream application.
One of the major drawbacks of this technology is that the management of zeolite in wastewater treatment is a labor-intensive process. Firstly, according to the data in previous study, total weight of zeolite used for ammonia adsorption is high (Zhao et al., 2018). For example, if the adding amount of zeolite in wastewater is set as 125 g L-1, to treat 1,000 m3 wastewater simultaneously, 125 tons zeolite will be used. Undeniably, it is a labor-intensive process to transport and manage such a huge amount of zeolite. Secondly, to reduce the total cost of zeolite-based wastewater treatment, the used zeolite should be collected and then washed for reuse (Lu et al., 2019). Such a process can be time-consuming and labor-intensive, limiting the wide application of zeolite in FPE remediation. In the view of the present authors, in the further research, efforts could be devoted to find out the zeolite with lower density and higher adsorption capacity for FPE treatment. Additionally, synthetic materials with better performance in ammonia adsorption and desorption could be developed to replace zeolite for FPE treatment.
5 Downstream application of microalgal astaxanthin in agriculture
5.1 Selection of the target market
Based on the discussion above, astaxanthin obtained from the microalgae cultivation in FPE has the advantages of low cost and no toxicity. To truly promote the long-term development of microalgae cultivation in FPE for astaxanthin production, it is of necessity to select the appropriate target market.
At present, the industries, which have high demand on astaxanthin, mainly include cosmetics, food, and animal feed (Lu et al., 2021; Lu and Lu, 2022). Firstly, astaxanthin with antioxidative properties could be added in cosmetics to attenuate the cellular senescence. Low cost of the raw material, however, is rarely a crucial concern of the cosmetics manufacturers due to the high profitability of cosmetics products. Thus, low-cost microalgal astaxanthin obtained from FPE might not be attractive to the manufacturers. Secondly, although microalgal astaxanthin obtained from FPE treatment has no toxicity, it may not be widely accepted by consumers. In the traditional mindset, the sources of food ingredients should never be the FPE. Thirdly, the increase of global population has stimulated the fast development of livestock and poultry farming and aquaculture. Accordingly, market demand on animal feed is increasing continuously, providing numerous opportunities to the commercialization of microalgal astaxanthin. Besides, microalgal astaxanthin could enhance the immune response of animals and prevent the overuse of antibiotics or medicines (Xie et al., 2018; Xie et al., 2020). The addition of microalgal astaxanthin in animal feed, hence, would be supported by the government, industry, and consumers. Last but not the least, due to the low cost, microalgal astaxanthin obtained from FPE is affordable to the manufacturers of animal feeds.
In recent years, owing to the promising application potential of microalgal astaxanthin in animal feed, the industry of animal feed production has become one of the major target markets. In addition, feed recipe research and animal experiment have been intensively conducted to promote the commercialization of microalgal astaxanthin. Hence, in the market, exploitation of algal astaxanthin as an ingredient of animal feed is emerging into the limelight.
5.2 Supplementation of microalgal astaxanthin in animal feed
Up to now, microalgal astaxanthin has been successfully applied in livestock feeding, fish and shrimp feeding, and poultry feeding. Dietary supplementation of astaxanthin in animals feeding could positive impact the meat quality, immune response, and growth rate (Table 4). Accordingly, the overuses of antibiotics and medicines in animal farming industry could be prevented. This is of importance to the development of eco-friendly agriculture and production of high-quality meat products.
5.2.1 Enrichment of astaxanthin
Dietary supplementation of microalgal astaxanthin could directly enrich astaxanthin in animal meat, improving the anti-oxidative properties and carcass traits of animal products. When Chlorella was added in fish diet for rainbow trout (Oncorhynchus mykiss) culture, accumulation of carotenoids (11.9 mg kg-1 dry muscle) was detected in the muscle of fish after 9 weeks (Gouveia et al., 1997). Similar phenomena were discovered in rotifer (Brachionus plicatilis) fed defatted H. lacustris meal and yolk colorness of layer hens fed algal astaxanthin (Magnuson et al., 2018; Li and Liu, 2019; Heng et al., 2021). With the enrichment of astaxanthin, anti-oxidative properties and carcass traits of animal products were improved. Firstly, since algal astaxanthin is beneficial to the health of humans, with the daily intake of astaxanthin-rich meat or egg, the health of consumers will be positively impacted. Secondly, pigmentation and brightness of in meat can be improved by the enrichment of astaxanthin, positively impacting the carcass traits and attractiveness of animal products (Yang et al., 2006). Therefore, dietary supplementation of microalgal astaxanthin can improve the qualities and market values of some animal products.
Deterioration is one of the main factors resulting in the short shelf life of meat and egg products. Besides, deterioration may cause the formation of oxidation compounds, such as aldehydes and ketones, with unfavorable flavors and unhealthy effects. As a consequence, the quality of meat and egg would be negatively impacted and the health of consumers would be threatened. At present, cold-chain logistics is adopted to reduce the deterioration rate and prolong the shelf life of meat and egg products but its cost is extremely high. In previous studies, it was discovered that the enrichment of algal astaxanthin in meat and egg could effectively attenuate deterioration and extend the shelf life of products (Heng et al., 2020; Szczepanik et al., 2022). For example, with the addition of algal astaxanthin in chicken diet, the decrease in yolk index and yolk color was delayed during the storage at 4°C and 25 °C (Heng et al., 2020). Besides, the improvement of shelf life of pork fat by dietary supplementation of algal astaxanthin in pig diet was reported (Szczepanik et al., 2022). The main mechanism for this phenomenon is that astaxanthin with super anti-oxidative capacity would react with oxidative substances in priority, thus protecting the meat and egg products from deterioration (Yuan et al., 2011).
5.2.2 Reduction of oxidative stress
Reactive oxygen species (ROS) are accumulated in cells and oxidative stress is improved when livestock, fish, shrimp and poultry are exposed to harsh environment. As reported by previous study, malonaldehyde (MDA) content of the whole shrimp body increased from 0.12 to 0.23 nmol mg-1 prot when post-larval white shrimps were exposed to salty stress (Xie et al., 2018). To improve the health status and survival of animals in the harsh environment, ROS should be scavanged properly.
Astaxanthin is a superantioxidant with the capacity of scavenging ROS in animals suffered from unfavorable environment. For example, with the dietary supplementation of defatted H. lacustris meal, MDA contents in the serum and hepatopancreas of adult Chinese mitten crab Eriocheir sinensis were reduced to 3.92 nmol mL-1 and 1.64 nmol mg-1 protein, respectively (Ma et al., 2019). In addition, after the acute salinity stress, compared with the control group (0.23 nmol MDA mg-1 protein), white shrimp (Litopenaeus vannamei) fed H. lacustris had much lower MDA content (0.16 nmol mg-1 protein) (Xie et al., 2018). Particularly, via scavenging ROS and reducing oxidative stress, survival of white shrimp was remarkably improved (Xie et al., 2018). Similarly, positive effects of dietary supplementation of algal astaxanthin on immune characteristics of broilers were reported (Awadh and Zangana, 2021). For example, the increase of algal astaxanthin content in chicken diet even caused the drop of MDA content in egg yolk (Magnuson et al., 2018).
It should be noted that the adding amount of algal astaxanthin in diet should be optimized according to the experimental results. In the culture of post-larval L. vannamei, before the acute salinity stress, the increase of H. lacustris content in diet from 3.3 to 13.3 g kg-1 did not cause the significant drop of MDA content in whole shrimp body (Xie et al., 2018). Hence, in terms of the reduction of oxidative stress, excessive addition of algal astaxanthin in animal diet may cause the waste of astaxanthin ingredient.
5.2.3 Promotion of animal growth
In addition to enhancing pigmentation and reducing oxidative stress, in some studies, dietary supplementation of astaxanthin-rich microalgae could effectively promote animal growth. It was discovered that feed intake and body weight gain of heifers increased with the addition of astaxanthin in diet and feed conversion ratio (FCR) was reduced accordingly (Kumar et al., 2019). Positive effects of dietary supplementation of algal astaxanthin on the weight gain and specific growth rate of golden pompano (Trachinotus ovatus) were also reported (Xie et al., 2020). According to the aforementioned results, microalgal astaxanthin can be employed as a growth-inducing agent in animal feed.
Nevertheless, it is noteworthy that some studies did not find the positive relation between dietary supplementation of algal astaxanthin and animal growth. In the animal experiment, when the addition of algal astaxanthin in chicken diet was 0 and 160 mg kg-1, feed consumption reached 136.30 and 137.40 g day-1, respectively (Yang et al., 2006). This result demonstrates that microalgal astaxanthin could not significantly improve the feed consumption of laying hens. In the culture of weaned pig, supplementation of algal astaxanthin at a concentration of 0.025 g kg-1 in diet did not significantly improve the average daily weight gains or reduce the FCR (Szczepanik et al., 2022). In the view of the present authors, in some cases, negative effect of astaxanthin-rich microalgae on animal growth is partially attributed to the poor digestability of algal biomass. Cellulose, which is one of the major components in the cell wall of microalgae, could not be efficiently digested by some carnivorous fish, thus resulting in the low weight gain and survival efficiency of Micropterus salmoides fed the diet with high content of microalgae (Deng et al., 2021).
Up to now, the mechanisms for the positive effects of algal astaxanthin on animal growth have not been fully revealed. Due to the contradictory opinions in previous studies, in the practice, the specific effects of algal astaxanthin on animals should be assessed on a case by case basis.
Based on the discussion above, in some cases, the addition of algal astaxanthin in diet could promote the enrichment of astaxanthin in meat and egg products, enhance the immune response, and improve the growth rate of animals. Compared to antibiotics and artificial hormone, algal astaxanthin, a type of natural pigment with anti-oxidative and growth-enhancing properties, has much less side effects.
6 Problems and prospects
The practical application of FPE for microalgae cultivation and astaxanthin production and the use of astaxanthin-rich microalgae as a feedstock for animal feed production are challenged by a couple of potential problems, including the potential safety risks of FPE in logistics, lack the pilot-scale experiments, and the poor degistibility and palatability of algal biomass. In the view of the present authors, to improve the contribution of algal astaxanthin to eco-friendly agriculture, it is of necessity to reduce the total cost of algal astaxanthin, make policy to support the use of FPE as medium alternative, and promote the technological innovations.
6.1 Potential problems in the practical application
6.1.1 Potential safety risks of FPE during logistics
To prevent the accumulation of unhealthy or toxic compounds in food chain, FPE, which is employed for microalgae cultivation, should not contain unhealthy or toxic compounds. Although most FPEs are regarded as nutrient-rich waste stream containing no toxic compounds, some FPEs might be contaminated by toxic bacteria. For example, in the lab research, researchers obtain low volume of FPE from factory directly and store FPE in refrigerator before the experiment. By contrast, in the industrial application, various bacteria in FPE can have much higher growth rates during the long-distance transportation. With the growth of toxic bacteria or the secretion of toxic compounds by bacteria in FPE, microalgae cultivated in FPE will be contaminated, challenging the safety of microalgae-based diet. According to the previous studies, toxic or unhealthy bacteria discovered in the algal-bacteria consortia include and so on. To our knowledge, due to the potential safety risks, few countries or regions have made policies or regulations to support the wide use of microalgae cultivated in wastewater as the feedstock for animal feed production.
Although cold-chain logistics can effectively prevent the fast growth of bacteria in FPE during the transportation and storage, it can remarkably increase the total cost of microalgae cultivation. Additionally, due to the large volume of FPE in the practice, in most cases, it is too hard to employ the cold-chain logistics to attenuate the deterioration of FPE in the transportation and storage. Therefore, in terms of the safety risks of FPE, there is a huge gap between lab research and industrial application. Based on the present technologies, with the fast growth of bacteria in FPE during transportation and storage, FPE may not be suitable to be used for microalgae cultivation.
6.1.2 Lack of pilot-scale experiments
Technological innovations, such as algal-bacterial co-culture, immobilization of microalgae, and zeolite-based ammonium adsorption, mentioned above have been intensively studied in the lab, however, they are rarely applied in the industry. For example, mechanisms associated with the microalgae immobilization on biofilm for wastewater remediation have been revealed in the lab research, but only a few studied conducted the pilot-scale experiments to evaluate the practical feasibility of RAB for algal astaxanthin production. Also, the concept of zeolite-based ammonium adsorption and desorption was mainly studied in the lab research while pilot-scale experiments were rarely conducted to use this innovative method for astaxanthin-rich microalgae cultivation (Lu et al., 2019; Lu et al., 2023a). Due to the lack of pilot-scale experiments, it is too hard to find out the weaknesses of these innovative methods developed in lab research and making them to be more applicable in the industry.
6.1.3 Poor digestibility and palatability of microalgal biomass
The cell wall of microalgae is consisted of cellulose and hemicellulose, which can not be digested efficiently by some carnivorous fish. In the aquaculture practice, poor digestibility of microalgal biomass results in the high FCR, low growth rate, and high cost of aquaculture production. Under this situation, even if microalgal biomass is enriched with a variety of high-value compounds, including natural astaxanthin, the nutrients contained in microalgae may not be fully assimilated by animals. In addition, fishy smell, which can increase the feed attraction and improve the feed intake, is an important factor impacting the quality of fish diet. In terms of the palatability, microalgae without fishy smell can not be regarded as a promising ingredient in fish diet. According to the previous studies, higher inclusion ratio of microalgal biomass in fish diet is always accompanied with lower feed intake (Deng et al., 2021). Due to the poor digestibility and palatability of microalgae, the application of microalgal biomass as a major ingredient in fish diet is hindered.
The straightforward strategies to improve the digestability of astaxanthin-rich microalgae include breaking the structure of cell wall and extracting astaxanthin from algal biomass. For example, cellulases can be employed to accelerate the decomposition of cell wall of microalgae and promote the release of astaxanthin contained in algal cells. Besides, since astaxanthin is a fat-soluble pigment, oil extraction method can be adopted to separate astaxanthin from algal biomass. Then, the astaxanthin-containing oil is added in diet to provide algal astaxanthin to animals (Namekawa et al., 2010). However, the aforementioned methods have high requirements on the technologies and increase the total cost of astaxanthin and astaxanthin-containing feed.
6.2 Prospects of the application of algal astaxanthin
6.2.1 Reduction of the total cost of algal astaxanthin
High price in the market is one of the major factors that limit the wide application of algal astaxanthin in agriculture. In many developing countries, since agricultural products are sold at low price, farmers or agricultural companies are not willing to use algal astaxanthin in animal diet to develop the eco-friendly agriculture even if they realize the ecological and environmental benefits of algal astaxanthin. In the coming future, to improve the market acceptance of algal astaxanthin, measures must be taken to further reduce the total cost of algal astaxanthin.
In the view of the present authors, in addition to the replacement of artificial medium by FPE, other methods, such as the use of solar energy for illumination and the increase of astaxanthin content in microalgae by genetic modification, could be applied to further lower the total cost of algal astaxanthin. It is expected that with the drop of production cost of algal astaxanthin, the acceptance of astaxanthin-based products in the market will be remarkably improved.
6.2.2 Policy support from the governments
In most developing countries, due to the lack of policies associated with the volarization of waste streams, most farms and food processing industries are not willing to recycle FPE for microalgae cultivation. The main reasons for this phenomenon include the high investment of microalgae cultivation systems, uncertainty in the market of algal bio-products, and lack of advanced technologies for astaxanthin exploitation. In addition, compared with antibiotics, medicines, and artificial hormones, which have been widely used in animal farming for a long time, algal astaxanthin is much less popular in the market. Accordingly, the concept of employing FPE for astaxanthin-rich microalgae cultivation is not attractive to the managers of food processing factories and farms.
In the foreseeable future, to promote the fast development of FPE valorization, astaxanthin-based products, and eco-friendly agriculture, governments should make appropriate policies. For example, the abuses of antibiotics, medicines, and artificial hormones in animals farmings should be forbidden by laws. Only in this way could the managers of farms start to focus on the use of eco-friendly bio-products for animals farming. In addition, policies should be made to require food processing industry to monitor the quality of FPE during transportation and storage, ensuring that microalgae cultivated in FPE would not be contaminated by toxic compounds or unfavorable bacteria. In this way, the quality of astaxanthin produced by FPE-based microalgae cultivation can be improved and the astaxanthin-based products can be more attractive to the consumers in the downstream industry.
6.2.3 Further improvement of the innovative technologies
Specific methods to apply the innovative technologies for microalgae-based astaxanthin production in FPE should be developed based on the pilot-scale experiments. Firstly, research interests should be shifted from the exploration of mechanisms to pilot-scale system design and operation gradually. Secondly, factors, such as complexity of technologies, total cost, availability of raw materials, and so on, which are important to the practical production and application of algal astaxanthin should be taken into consideration by researchers. For example, in previous studies, zeolite-based ammonium adsorption and desorption were conducted in flasks for microalgae cultivation in waste streams while the effectiveness of this innovative technology in pilot-scale system was remained unclear (Lu et al., 2019; Lu et al., 2023a). Besides, techno-economic analysis has not been conducted to comprehensively assess the cost and profitability of employing zeolite for microalgae cultivation in ammonium-rich waste streams. In other words, current research results could not fully present the advantages of zeolite-based ammonium adsorption and desorption for microalgae cultivation and astaxanthin production in FPE.
7 Conclusion
It is concluded that 1) H. lacustris and C. zofingiensis, which are enriched with astaxanthin, easily obtained, highly tolerant to harsh environment in wastewater, are regarded as promising microalgal strains for astaxanthin production in FPE; 2) FPEs, which contain essential nutrients for microalgae cultivation, can be regarded as a promising alternative to artificial medium; 3) based on the theoretical estimation, CO2 emission of microalgae-based FPE remediation is 79.89% lower than that of FPE treatment by chemical oxidation; 4) innovative technologies, including algal-bacterial co-culture, immobilization of microalgae, and zeolite-based ammonium adsorption, have been developed to promote the microalgae-based wastewater valorization; 5) Dietary supplementation of algal astaxanthin is beneficial to the weight gain, immune response, and pigmentation of animals, thus preventing the abuses of antibiotics, artificial hormones, and medicines in animals farming.
It is undeniable that in the practical application, the use of FPE for microalgae cultivation and astaxanthin is challenged by a couple of problems, such as the potential safety risks of FPE during logistics, lack of pilot-scale experiments, and poor digestibility and palatability of microalgal biomass. In the view of the present authors, efforts should be make to lower the total cost of algal astaxanthin, provide policy support, and further improve the innovative technologies, promoting the industrialization of FPE valorization by microalgae-based astaxanthin production.
Author contributions
XZ: Writing–original draft. QL: Writing–review and editing, Funding acquisition.
Funding
The author(s) declare that financial support was received for the research, authorship, and/or publication of this article. This work was supported by Basic Research Program Natural Science Foundation of Jiangsu Province (BK20230665).
Conflict of interest
The authors declare that the research was conducted in the absence of any commercial or financial relationships that could be construed as a potential conflict of interest.
Publisher’s note
All claims expressed in this article are solely those of the authors and do not necessarily represent those of their affiliated organizations, or those of the publisher, the editors and the reviewers. Any product that may be evaluated in this article, or claim that may be made by its manufacturer, is not guaranteed or endorsed by the publisher.
References
Asgharnejad, H., Khorshidi Nazloo, E., Madani Larijani, M., Hajinajaf, N., and Rashidi, H. (2021). Comprehensive review of water management and wastewater treatment in food processing industries in the framework of water-food-environment nexus. Compr. Rev. Food Sci. Food Saf. 20 (5), 4779–4815. doi:10.1111/1541-4337.12782
Avcioglu, S. G., Ozgur, E., Eroglu, I., Yucel, M., and Gunduz, U. (2011). Biohydrogen production in an outdoor panel photobioreactor on dark fermentation effluent of molasses. Int. J. Hydrogen Energy 36 (17), 11360–11368. doi:10.1016/j.ijhydene.2010.12.046
Awadh, I. A., and Zangana, B. S. (2021). Effect of adding different levels of astaxanthin extracted from an algae haematococcus Pluvialis to the diet on some immunological characteristics of broilers reared under natural and elevated environmental conditions. IOP Conf. Ser. Earth Environ. Sci. 910, 012003. doi:10.1088/1755-1315/910/1/012003
Aziz, A., Basheer, F., Sengar, A., Irfanullah, , Khan, S. U., and Farooqi, I. H. (2019). Biological wastewater treatment (anaerobic-aerobic) technologies for safe discharge of treated slaughterhouse and meat processing wastewater. Sci. Total Environ. 686, 681–708. doi:10.1016/j.scitotenv.2019.05.295
Basiony, M., Ouyang, L., Wang, D., Yu, J., Zhou, L., Zhu, M., et al. (2022). Optimization of microbial cell factories for astaxanthin production: biosynthesis and regulations, engineering strategies and fermentation optimization strategies. Synth. Syst. Biotechnol. 7 (2), 689–704. doi:10.1016/j.synbio.2022.01.002
Chekanov, K., Litvinov, D., Fedorenko, T., Chivkunova, O., and Lobakova, E. (2021). Combined production of astaxanthin and β-carotene in a new strain of the microalga Bracteacoccus aggregatus BM5/15 (IPPAS C-2045) cultivated in photobioreactor. Biology 10 (7), 643. doi:10.3390/biology10070643
Chen, T., Wei, D., Chen, G., Wang, Y., and Chen, F. (2009). Employment of organic acids to enhance astaxanthin formation in heterotrophic Chlorella zofingiensis. J. Food Process Preserv 33 (2), 271–284. doi:10.1111/j.1745-4549.2009.00391.x
Chong, J. W. R., Khoo, K. S., Yew, G. Y., Leong, W. H., Lim, J. W., Lam, M. K., et al. (2021). Advances in production of bioplastics by microalgae using food waste hydrolysate and wastewater: a review. Bioresour. Technol. 342, 125947. doi:10.1016/j.biortech.2021.125947
Christian, D., Zhang, J., Sawdon, A. J., and Peng, C. A. (2018). Enhanced astaxanthin accumulation in Haematococcus pluvialis using high carbon dioxide concentration and light illumination. Bioresour. Technol. 256, 548–551. doi:10.1016/j.biortech.2018.02.074
Chuka-ogwude, D., Ogbonna, J., and Moheimani, N. R. (2020). A review on microalgal culture to treat anaerobic digestate food waste effluent. Algal Res. 47, 101841. doi:10.1016/j.algal.2020.101841
Collos, Y., and Harrison, P. J. (2014). Acclimation and toxicity of high ammonium concentrations to unicellular algae. Mar. Pollut. Bull. 80 (1-2), 8–23. doi:10.1016/j.marpolbul.2014.01.006
Delattre, C., Pierre, G., Laroche, C., and Michaud, P. (2016). Production, extraction and characterization of microalgal and cyanobacterial exopolysaccharides. Biotechnol. Adv. 34 (7), 1159–1179. doi:10.1016/j.biotechadv.2016.08.001
Deng, Y., Chen, F., Liao, K., Xiao, Y., Chen, S., Lu, Q., et al. (2021). Microalgae for nutrient recycling from food waste to aquaculture as feed substitute: a promising pathway to eco-friendly development. J. Chem. Technol. Biotechnol. 96 (9), 2496–2508. doi:10.1002/jctb.6786
Diao, J., Song, X., Zhang, L., Cui, J., Chen, L., and Zhang, W. (2020). Tailoring cyanobacteria as a new platform for highly efficient synthesis of astaxanthin. Metab. Eng. 61, 275–287. doi:10.1016/j.ymben.2020.07.003
Fajardo-Puerto, E., Elmouwahidi, A., Bailón-García, E., Pérez-Cadenas, A. F., and Carrasco-Marín, F. (2023). From Fenton and ORR 2e−-Type Catalysts to Bifunctional Electrodes for Environmental Remediation Using the Electro-Fenton Process. Catalysts 13 (4), 674. doi:10.3390/catal13040674
Fernando, J. S. R., Premaratne, M., Dinalankara, D. M. S. D., Perera, G. L. N. J., and Ariyadasa, T. U. (2021). Cultivation of microalgae in palm oil mill effluent (POME) for astaxanthin production and simultaneous phycoremediation. J. Environ. Chem. Eng. 9 (4), 105375. doi:10.1016/j.jece.2021.105375
Gorgich, M., Martins, A. A., Mata, T. M., and Caetano, N. S. (2021). Composition, cultivation and potential applications of Chlorella zofingiensis–A comprehensive review. Algal Res. 60, 102508. doi:10.1016/j.algal.2021.102508
Gouveia, L., Gomes, E., and Empis, J. (1997). Use of Chlorella vulgaris in rainbow trout, Oncorhynchus mykiss, diets to enhance muscle pigmentation. J. Appl. Aquacult 7 (2), 61–70. doi:10.1300/j028v07n02_07
Gross, M., Henry, W., Michael, C., and Wen, Z. (2013). Development of a rotating algal biofilm growth system for attached microalgae growth with in situ biomass harvest. Bioresour. Technol. 150, 195–201. doi:10.1016/j.biortech.2013.10.016
Gross, M., Mascarenhas, V., and Wen, Z. (2015). Evaluating algal growth performance and water use efficiency of pilot-scale revolving algal biofilm (RAB) culture systems. Biotechnol. Bioeng. 112 (10), 2040–2050. doi:10.1002/bit.25618
Gupta, S., and Pawar, S. B. (2018). An integrated approach for microalgae cultivation using raw and anaerobic digested wastewaters from food processing industry. Bioresour. Technol. 269, 571–576. doi:10.1016/j.biortech.2018.08.113
Haque, F., Dutta, A., Thimmanagari, M., and Chiang, Y. W. (2017). Integrated Haematococcus pluvialis biomass production and nutrient removal using bioethanol plant waste effluent. Process Saf. Environ. Prot. 111, 128–137. doi:10.1016/j.psep.2017.06.013
Heng, N., Gao, S., Chen, Y., Wang, L., Li, Z., Guo, Y., et al. (2021). Dietary supplementation with natural astaxanthin from Haematococcus pluvialis improves antioxidant enzyme activity, free radical scavenging ability, and gene expression of antioxidant enzymes in laying hens. Poult. Sci. 100 (5), 101045. doi:10.1016/j.psj.2021.101045
Heng, N., Gao, S., Guo, Y., Chen, Y., Wang, L., Sheng, X., et al. (2020). Effects of supplementing natural astaxanthin from Haematococcus pluvialis to laying hens on egg quality during storage at 4°C and 25°C. Poult. Sci. 99 (12), 6877–6883. doi:10.1016/j.psj.2020.09.010
Hongyang, S., Yalei, Z., Chunmin, Z., Xuefei, Z., and Jinpeng, L. (2011). Cultivation of Chlorella pyrenoidosa in soybean processing wastewater. Bioresour. Technol. 102 (21), 9884–9890. doi:10.1016/j.biortech.2011.08.016
Hu, X., Meneses, Y. E., Stratton, J., and Wang, B. (2019). Acclimation of consortium of micro-algae help removal of organic pollutants from meat processing wastewater. J. Clean. Prod. 214, 95–102. doi:10.1016/j.jclepro.2018.12.255
Hu, Y., Xiao, Y., Liao, K., Leng, Y., and Lu, Q. (2021). Development of microalgal biofilm for wastewater remediation: from mechanism to practical application. J. Chem. Technol. Biotechnol. 96 (11), 2993–3008. doi:10.1002/jctb.6850
Ip, P.-F., and Chen, F. (2005). Production of astaxanthin by the green microalga Chlorella zofingiensis in the dark. Process Biochem. 40 (2), 733–738. doi:10.1016/j.procbio.2004.01.039
Ji, C., Wang, H., Cui, H., Zhang, C., Li, R., and Liu, T. (2023). Characterization and evaluation of substratum material selection for microalgal biofilm cultivation. Appl. Microbiol. Biotechnol. 107 (7-8), 2707–2721. doi:10.1007/s00253-023-12475-7
Jia, Z., and Lin, B. (2021). How to achieve the first step of the carbon-neutrality 2060 target in China: the coal substitution perspective. Energy 233, 121179. doi:10.1016/j.energy.2021.121179
Jiang, X., Liu, L., Chen, J., and Wei, D. (2018). Effects of Xanthophyllomyces dendrorhous on cell growth, lipid, and astaxanthin production of Chromochloris zofingiensis by mixed culture strategy. J. Appl. Phycol. 30, 3009–3015. doi:10.1007/s10811-018-1553-8
Kaha, M., Iwamoto, K., Yahya, N. A., Suhaimi, N., Sugiura, N., Hara, H., et al. (2021). Enhancement of astaxanthin accumulation using black light in Coelastrum and Monoraphidium isolated from Malaysia. Sci. Rep. 11 (1), 11708. doi:10.1038/s41598-021-91128-z
Kakizono, T., Kobayashi, M., and Nagai, S. (1992). Effect of carbon/nitrogen ratio on encystment accompanied with astaxanthin formation in a green alga, Haematococcus pluvialis. J. Ferment Bioeng. 74 (6), 403–405. doi:10.1016/0922-338x(92)90041-r
Khalili, Z., Jalili, H., Noroozi, M., and Amrane, A. (2019). Effect of linoleic acid and methyl jasmonate on astaxanthin content of Scenedesmus acutus and Chlorella sorokiniana under heterotrophic cultivation and salt shock conditions. J. Appl. Phycol. 31, 2811–2822. doi:10.1007/s10811-019-01782-0
Kumar, S., Singh, S. V., and Bhan, S. C. (2019). Effect of dietary supplementation of astaxanthin (potent antioxidant) on growth rate, DMI, FCR and metabolic changes in Karan Fries heifers during heat stress. J. Agrometeorol. 21 (1), 80–88. doi:10.54386/jam.v21i1.210
Lee, C., Jeon, M. S., Kim, J. Y., Lee, S. H., Kim, D. G., Roh, S. W., et al. (2019a). Effects of an auxin-producing symbiotic bacterium on cell growth of the microalga Haematococcus pluvialis: elevation of cell density and prolongation of exponential stage. Algal Res. 41, 101547. doi:10.1016/j.algal.2019.101547
Lee, Z. S., Chin, S. Y., Lim, J. W., Witoon, T., and Cheng, C. K. (2019b). Treatment technologies of palm oil mill effluent (POME) and olive mill wastewater (OMW): a brief review. Environ. Technol. Innov. 15, 100377. doi:10.1016/j.eti.2019.100377
Leifeld, V., dos Santos, T. P. M., Zelinski, D. W., and Igarashi-Mafra, L. (2018). Ferrous ions reused as catalysts in Fenton-like reactions for remediation of agro-food industrial wastewater. J. Environ. Manage 222, 284–292. doi:10.1016/j.jenvman.2018.05.087
Li, F., Cai, M., Wu, Y., Lian, Q., Qian, Z., Luo, J., et al. (2022). Effects of nitrogen and light intensity on the astaxanthin accumulation in motile cells of Haematococcus pluvialis. Front. Mar. Sci. 9, 909237. doi:10.3389/fmars.2022.909237
Li, H., and Liu, J. (2019). Effects of defatted Haematococcus pluvialis meal (DHPM) supplementation on the growth performance, and the carotenoid content and composition in the rotifer (Brachionus plicatilis). Aquaculture 505, 34–40. doi:10.1016/j.aquaculture.2019.02.027
Li, J., Wang, L., Lu, Q., and Zhou, W. (2019a). Toxicity alleviation for microalgae cultivation by cationic starch addition and ammonia stripping and study on the cost assessment. RSC Adv. 9 (65), 38235–38245. doi:10.1039/c9ra03454d
Li, S., Zhao, S., Yan, S., Qiu, Y., Song, C., Li, Y., et al. (2019b). Food processing wastewater purification by microalgae cultivation associated with high value-added compounds production—a review. Chin. J. Chem. Eng. 27 (12), 2845–2856. doi:10.1016/j.cjche.2019.03.028
Liang, H., Chen, H., Liu, X., Wang, Z., Li, P., and Lu, S. (2023). Heterologous production in the Synechocystis chassis suggests the biosynthetic pathway of astaxanthin in cyanobacteria. Antioxidants 12 (10), 1826. doi:10.3390/antiox12101826
Liu, H., Lu, Q., Wang, Q., Liu, W., Wei, Q., Ren, H., et al. (2017). Isolation of a bacterial strain, Acinetobacter sp. from centrate wastewater and study of its cooperation with algae in nutrients removal. Bioresour. Technol. 235, 59–69. doi:10.1016/j.biortech.2017.03.111
Liu, J., Mao, X., Zhou, W., and Guarnieri, M. T. (2016). Simultaneous production of triacylglycerol and high-value carotenoids by the astaxanthin-producing oleaginous green microalga Chlorella zofingiensis. Bioresour. Technol. 214, 319–327. doi:10.1016/j.biortech.2016.04.112
Liu, J., Sun, Z., Zhong, Y., Gerken, H., Huang, J., and Chen, F. (2013). Utilization of cane molasses towards cost-saving astaxanthin production by a Chlorella zofingiensis mutant. J. Appl. Phycol. 25, 1447–1456. doi:10.1007/s10811-013-9974-x
Liu, Y., Cui, Y., Chen, J., Qin, S., and Chen, G. (2019). Metabolic engineering of Synechocystis sp. PCC6803 to produce astaxanthin. Algal Res. 44, 101679. doi:10.1016/j.algal.2019.101679
López-Rosales, L., López-García, P., Benyachou, M., Molina-Miras, A., Gallardo-Rodríguez, J., Cerón-García, M., et al. (2022). Treatment of secondary urban wastewater with a low ammonium-tolerant marine microalga using zeolite-based adsorption. Bioresour. Technol. 359, 127490. doi:10.1016/j.biortech.2022.127490
Lu, Q., Han, P., Chen, F., Liu, T., Li, J., Leng, L., et al. (2019). A novel approach of using zeolite for ammonium toxicity mitigation and value-added Spirulina cultivation in wastewater. Bioresour. Technol. 280, 127–135. doi:10.1016/j.biortech.2019.02.042
Lu, Q., Li, H., Zou, Y., Liu, H., and Yang, L. (2021). Astaxanthin as a microalgal metabolite for aquaculture: a review on the synthetic mechanisms, production techniques, and practical application. Algal Res. 54, 102178. doi:10.1016/j.algal.2020.102178
Lu, Q., Liu, H., Sun, Y., and Li, H. (2023a). Combined zeolite-based ammonia slow-release and algae-yeast consortia to treat piggery wastewater: improved nitrogen and carbon migration. Bioresour. Technol. 387, 129671. doi:10.1016/j.biortech.2023.129671
Lu, Q., and Lu, Y. (2022). Microalga-and yeast-based astaxanthin production via nutrient recovery from wastewater for aquaculture practice: an emerging technology for sustainable development. J. Chem. Technol. Biotechnol. 97 (11), 3035–3048. doi:10.1002/jctb.7164
Lu, Q., Ma, C., Guo, L., Lu, Y., and Li, H. (2023b). Co-fermentation of Chlorella vulgaris with oleaginous yeast in starch processing effluent as a carbon-reducing strategy for wastewater treatment and biofuel feedstock production. Fermentation 9 (5), 476. doi:10.3390/fermentation9050476
Lu, Q., Zhou, W., Min, M., Ma, X., Chandra, C., Doan, Y. T., et al. (2015). Growing Chlorella sp. on meat processing wastewater for nutrient removal and biomass production. Bioresour. Technol. 198, 189–197. doi:10.1016/j.biortech.2015.08.133
Lu, Q., Zhou, W., Min, M., Ma, X., Ma, Y., Chen, P., et al. (2016). Mitigating ammonia nitrogen deficiency in dairy wastewaters for algae cultivation. Bioresour. Technol. 201, 33–40. doi:10.1016/j.biortech.2015.11.029
Ma, N., Long, X., Liu, J., Chang, G., Deng, D., Cheng, Y., et al. (2019). Defatted Haematococcus pluvialis meal can enhance the coloration of adult Chinese mitten crab Eriocheir sinensis. Aquaculture 510, 371–379. doi:10.1016/j.aquaculture.2019.05.063
Macías-Montes, A., Zumbado, M., Luzardo, O., Rodríguez-Hernández, Á., Acosta-Dacal, A., Rial-Berriel, C., et al. (2021). Nutritional evaluation and risk assessment of the exposure to essential and toxic elements in dogs and cats through the consumption of pelleted dry food: how important is the quality of the feed? Toxics 9 (6), 133. doi:10.3390/toxics9060133
Magnuson, A., Sun, T., Yin, R., Liu, G., Tolba, S., Shinde, S., et al. (2018). Supplemental microalgal astaxanthin produced coordinated changes in intrinsic antioxidant systems of layer hens exposed to heat stress. Algal Res. 33, 84–90. doi:10.1016/j.algal.2018.04.031
Markou, G., Vandamme, D., and Muylaert, K. (2014). Using natural zeolite for ammonia sorption from wastewater and as nitrogen releaser for the cultivation of Arthrospira platensis. Bioresour. Technol. 155, 373–378. doi:10.1016/j.biortech.2013.12.122
Matter, I. A., Bui, V. K. H., Jung, M., Seo, J. Y., Kim, Y. E., Lee, Y. C., et al. (2019). Flocculation harvesting techniques for microalgae: a review. Appl. Sci. 9 (15), 3069. doi:10.3390/app9153069
Milan, F. S., Maleki, B. R. S., Moosavy, M. H., Mousavi, S., Sheikhzadeh, N., and Khatibi, S. A. (2021). Ameliorating effects of dietary Haematococcus pluvialis on arsenic-induced oxidative stress in rainbow trout (Oncorhynchus mykiss) fillet. Ecotoxicol. Environ. Saf. 207, 111559. doi:10.1016/j.ecoenv.2020.111559
Minyuk, G., Chelebieva, E., Chubchikova, I., Dantsyuk, N., Drobetskaya, I., Sakhon, E., et al. (2017). Stress-induced secondary carotenogenesis in Coelastrella rubescens (Scenedesmaceae, Chlorophyta), a producer of value-added keto-carotenoids. Algae 32 (3), 245–259. doi:10.4490/algae.2017.32.8.6
Misra, A., Chandra, P., and Raghavendra, V. (2011). Modeling the depletion of dissolved oxygen in a lake due to algal bloom: effect of time delay. Adv. Water Resour. 34 (10), 1232–1238. doi:10.1016/j.advwatres.2011.05.010
Najjar, Y. S., and Abu-Shamleh, A. (2020). Harvesting of microalgae by centrifugation for biodiesel production: a review. Algal Res. 51, 102046. doi:10.1016/j.algal.2020.102046
Namekawa, T., Ikeda, S., Sugimoto, M., and Kume, S. (2010). Effects of astaxanthin-containing oil on development and stress-related gene expression of bovine embryos exposed to heat stress. Reprod. Domest. Anim. 45 (6), e387–e391. doi:10.1111/j.1439-0531.2010.01584.x
Nguyen, V.-T., Le, V. A., Do, Q. H., and Vo, T. D. H. (2023). Emerging revolving algae biofilm system for algal biomass production and nutrient recovery from wastewater. Sci. Total Environ. 912, 168911. doi:10.1016/j.scitotenv.2023.168911
Nishshanka, G. K. S. H., Liyanaarachchi, V. C., Premaratne, M., Nimarshana, P., Ariyadasa, T. U., and Kornaros, M. (2021a). Wastewater-based microalgal biorefineries for the production of astaxanthin and co-products: current status, challenges and future perspectives. Bioresour. Technol. 342, 126018. doi:10.1016/j.biortech.2021.126018
Nishshanka, S. H., Liyanaarachchi, V. C., Premaratne, M., Ariyadasa, T. U., and Nimarshana, V. (2021b). “Sustainable cultivation of haematococcus pluvialis for the production of natural astaxanthin,” in 2021 Moratuwa Engineering Research Conference (MERCon), Moratuwa, Sri Lanka, 27-29 July 2021 (IEEE), 297–302.
Olabi, A., Shehata, N., Sayed, E. T., Rodriguez, C., Anyanwu, R. C., Russell, C., et al. (2023). Role of microalgae in achieving sustainable development goals and circular economy. Sci. Total Environ. 854, 158689. doi:10.1016/j.scitotenv.2022.158689
Oliveira, C. Y. B., Jacob, A., Nader, C., Matos, Â. P., Araújo, E. S., Shabnam, N., et al. (2022). An overview on microalgae as renewable resources for meeting sustainable development goals. J. Environ. Manage 320, 115897. doi:10.1016/j.jenvman.2022.115897
Pan, M., Zhu, X., Pan, G., and Angelidak, I. (2021). Integrated valorization system for simultaneous high strength organic wastewater treatment and astaxanthin production from Haematococcus pluvialis. Bioresour. Technol. 326, 124761. doi:10.1016/j.biortech.2021.124761
Pelah, D., Sintov, A., and Cohen, E. (2004). The effect of salt stress on the production of canthaxanthin and astaxanthin by Chlorella zofingiensis grown under limited light intensity. World J. Microbiol. Biotechnol. 20, 483–486. doi:10.1023/b:wibi.0000040398.93103.21
Perozeni, F., Cazzaniga, S., Baier, T., Zanoni, F., Zoccatelli, G., Lauersen, K. J., et al. (2020). Turning a green alga red: engineering astaxanthin biosynthesis by intragenic pseudogene revival in Chlamydomonas reinhardtii. Plant Biotechnol. J. 18 (10), 2053–2067. doi:10.1111/pbi.13364
Preisner, M., Neverova-Dziopak, E., and Kowalewski, Z. (2021). Mitigation of eutrophication caused by wastewater discharge: a simulation-based approach. Ambio 50 (2), 413–424. doi:10.1007/s13280-020-01346-4
Raman, R., and Mohamad, S. E. (2012). Astaxanthin production by freshwater microalgae Chlorella sorokiniana and marine microalgae Tetraselmis sp. Pak J. Biol. Sci. 15 (24), 1182–1186. doi:10.3923/pjbs.2012.1182.1186
Río, E. D., Acién, F. G., García-Malea, M. C., Rivas, J., Molina-Grima, E., and Guerrero, M. G. (2005). Efficient one-step production of astaxanthin by the microalga Haematococcus pluvialis in continuous culture. Biotechnol. Bioeng. 91 (7), 808–815. doi:10.1002/bit.20547
Schanes, K., Dobernig, K., and Gözet, B. (2018). Food waste matters-A systematic review of household food waste practices and their policy implications. J. Clean. Prod. 182, 978–991. doi:10.1016/j.jclepro.2018.02.030
Shen, Y., Xu, X., Zhao, Y., and Lin, X. (2014). Influence of algae species, substrata and culture conditions on attached microalgal culture. Bioprocess Biosyst. Eng. 37, 441–450. doi:10.1007/s00449-013-1011-6
Su, K., Li, X., Lu, T., Mou, Y., Liu, N., Song, M., et al. (2022). Screening of the heterotrophic microalgae strain for the reclamation of acid producing wastewater. Chemosphere 307, 136047. doi:10.1016/j.chemosphere.2022.136047
Szczepanik, K., Furgał-Dierżuk, I., Gala, Ł., and Świątkiewicz, M. (2022). Effects of hermetia illucens larvae meal and astaxanthin as feed additives on health and production indices in weaned pigs. Animals 13 (1), 163. doi:10.3390/ani13010163
Tsioptsias, C., Petridis, D., Athanasakis, N., Lemonidis, I., Deligiannis, A., and Samaras, P. (2015). Post-treatment of molasses wastewater by electrocoagulation and process optimization through response surface analysis. J. Environ. Manage 164, 104–113. doi:10.1016/j.jenvman.2015.09.007
Vasistha, S., Balakrishnan, D., Manivannan, A., and Rai, M. P. (2023). Microalgae on distillery wastewater treatment for improved biodiesel production and cellulose nanofiber synthesis: a sustainable biorefinery approach. Chemosphere 315, 137666. doi:10.1016/j.chemosphere.2022.137666
Vissio, P. G., Darias, M. J., Di Yorio, M. P., Pérez Sirkin, D. I., and Delgadin, T. H. (2021). Fish skin pigmentation in aquaculture: the influence of rearing conditions and its neuroendocrine regulation. Gen. Comp. Endocrinol. 301, 113662. doi:10.1016/j.ygcen.2020.113662
Waldenstedt, L., Inborr, J., Hansson, I., and Elwinger, K. (2003). Effects of astaxanthin-rich algal meal (Haematococcus pluvalis) on growth performance, caecal campylobacter and clostridial counts and tissue astaxanthin concentration of broiler chickens. Anim. Feed Sci. Technol. 108 (1-4), 119–132. doi:10.1016/s0377-8401(03)00164-0
Wang, J., Sommerfeld, M. R., Lu, C., and Hu, Q. (2013). Effect of initial biomass density on growth and astaxanthin production of Haematococcus pluvialis in an outdoor photobioreactor. J. Appl. Phycol. 25, 253–260. doi:10.1007/s10811-012-9859-4
Wang, Y., Jia, J., Chi, Q., Li, Y., Wang, H., Gong, Y., et al. (2022). Critical assessment of the filamentous green microalga Oedocladium carolinianum for astaxanthin and oil production. Algal Res. 61, 102599. doi:10.1016/j.algal.2021.102599
Watson, S. B., Miller, C., Arhonditsis, G., Boyer, G. L., Carmichael, W., Charlton, M. N., et al. (2016). The re-eutrophication of Lake Erie: harmful algal blooms and hypoxia. Harmful Algae 56, 44–66. doi:10.1016/j.hal.2016.04.010
Xie, J., Fang, H., He, X., Liao, S., Liu, Y., Tian, L., et al. (2020). Study on mechanism of synthetic astaxanthin and Haematococcus pluvialis improving the growth performance and antioxidant capacity under acute hypoxia stress of golden pompano (Trachinotus ovatus) and enhancing anti-inflammatory by activating Nrf2-ARE pathway to antagonize the NF-κB pathway. Aquaculture 518, 734657. doi:10.1016/j.aquaculture.2019.734657
Xie, S., Fang, W., Wei, D., Liu, Y., Yin, P., Niu, J., et al. (2018). Dietary supplementation of Haematococcus pluvialis improved the immune capacity and low salinity tolerance ability of post-larval white shrimp, Litopenaeus vannamei. Fish. Shellfish Immunol. 80, 452–457. doi:10.1016/j.fsi.2018.06.039
Yang, K., Qin, L., Wang, Z., Feng, W., Feng, P., Zhu, S., et al. (2015a). Water-saving analysis on an effective water reuse system in biodiesel feedstock production based on Chlorella zofingiensis fed-batch cultivation. Water Sci. Technol. 71 (10), 1562–1568. doi:10.2166/wst.2015.139
Yang, L., Tan, X., Li, D., Chu, H., Zhou, X., Zhang, Y., et al. (2015b). Nutrients removal and lipids production by Chlorella pyrenoidosa cultivation using anaerobic digested starch wastewater and alcohol wastewater. Bioresour. Technol. 181, 54–61. doi:10.1016/j.biortech.2015.01.043
Yang, Y., Kim, Y. J., Jin, Z., Lohakare, J. D., Kim, C. H., Ohh, S. H., et al. (2006). Effects of dietary supplementation of astaxanthin on production performance, egg quality in layers and meat quality in finishing pigs. Asian-Australas J. Anim. Sci. 19 (7), 1019–1025. doi:10.5713/ajas.2006.1019
Yu, C., Li, X., Han, B., Zhao, Y., Geng, S., Ning, D., et al. (2021). Simultaneous improvement of astaxanthin and lipid production of Haematococcus pluvialis by using walnut shell extracts. Algal Res. 54, 102171. doi:10.1016/j.algal.2020.102171
Yu, M., Wang, L., Feng, P., Wang, Z., and Zhu, S. (2024). Treatment of mixed wastewater by vertical rotating microalgae-bacteria symbiotic biofilm reactor. Bioresour. Technol. 393, 130057. doi:10.1016/j.biortech.2023.130057
Yuan, J.-P., Chen, F., Liu, X., and Li, X. Z. (2002). Carotenoid composition in the green microalga Chlorococcum. Food Chem. 76 (3), 319–325. doi:10.1016/s0308-8146(01)00279-5
Yuan, J. P., Peng, J., Yin, K., and Wang, J. (2011). Potential health-promoting effects of astaxanthin: a high-value carotenoid mostly from microalgae. Mol. Nutr. Food Res. 55 (1), 150–165. doi:10.1002/mnfr.201000414
Zhang, D.-H., and Lee, Y.-K. (2001). Two-step process for ketocarotenoid production by a green alga, Chlorococcum sp. strain MA-1. Appl. Microbiol. Biotechnol. 55, 537–540. doi:10.1007/s002530000526
Zhang, Z., Sun, D., Zhang, Y., and Chen, F. (2019). Glucose triggers cell structure changes and regulates astaxanthin biosynthesis in Chromochloris zofingiensis. Algal Res. 39, 101455. doi:10.1016/j.algal.2019.101455
Zhao, X., Kumar, K., Gross, M. A., Kunetz, T. E., and Wen, Z. (2018). Evaluation of revolving algae biofilm reactors for nutrients and metals removal from sludge thickening supernatant in a municipal wastewater treatment facility. Water Res. 143, 467–478. doi:10.1016/j.watres.2018.07.001
Zhao, Y., Xing, H., Li, X., Geng, S., Ning, D., Ma, T., et al. (2019a). Physiological and metabolomics analyses reveal the roles of fulvic acid in enhancing the production of astaxanthin and lipids in Haematococcus pluvialis under abiotic stress conditions. J. Agric. Food Chem. 67 (45), 12599–12609. doi:10.1021/acs.jafc.9b04964
Keywords: microalgae, astaxanthin, food processing effluent, bioproduct, valorization
Citation: Zhang X and Lu Q (2024) Cultivation of microalgae in food processing effluent for pollution attenuation and astaxanthin production: a review of technological innovation and downstream application. Front. Bioeng. Biotechnol. 12:1365514. doi: 10.3389/fbioe.2024.1365514
Received: 04 January 2024; Accepted: 06 March 2024;
Published: 20 March 2024.
Edited by:
Gassan Hodaifa, Pablo de Olavide University, SpainReviewed by:
Hongli Cui, Chinese Academy of Sciences (CAS), ChinaCarlos Yure B. Oliveira, Federal University of Santa Catarina, Brazil
Copyright © 2024 Zhang and Lu. This is an open-access article distributed under the terms of the Creative Commons Attribution License (CC BY). The use, distribution or reproduction in other forums is permitted, provided the original author(s) and the copyright owner(s) are credited and that the original publication in this journal is cited, in accordance with accepted academic practice. No use, distribution or reproduction is permitted which does not comply with these terms.
*Correspondence: Xiaowei Zhang, eGlhb3dlaUBqdXN0LmVkdS5jbg==; Qian Lu, bHVxaWFuQGp1c3QuZWR1LmNu