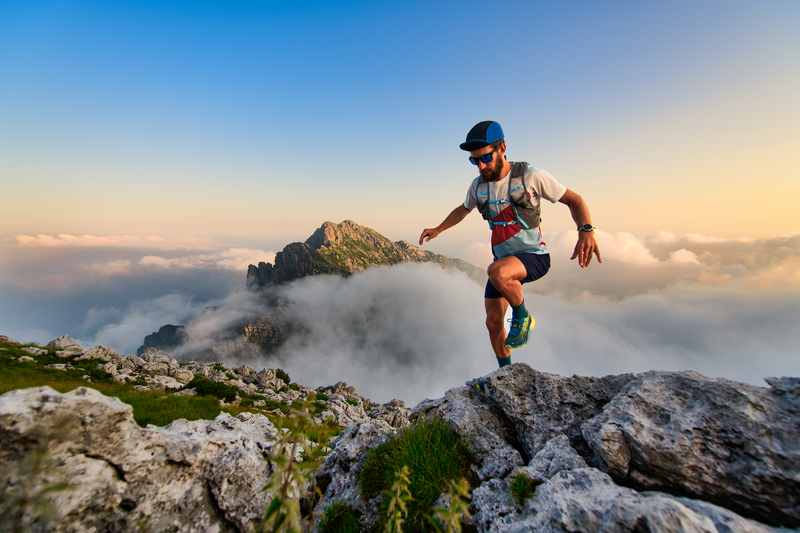
95% of researchers rate our articles as excellent or good
Learn more about the work of our research integrity team to safeguard the quality of each article we publish.
Find out more
ORIGINAL RESEARCH article
Front. Bioeng. Biotechnol. , 12 February 2024
Sec. Nanobiotechnology
Volume 12 - 2024 | https://doi.org/10.3389/fbioe.2024.1361966
This article is part of the Research Topic Research on Nanomaterials in Tumor Diagnosis and Therapy View all 8 articles
The mitochondria act as the main producers of reactive oxygen species (ROS) within cells. Elevated levels of ROS can activate the mitochondrial apoptotic pathway, leading to cell apoptosis. In this study, we devised a molecular prodrug named CTT2P, demonstrating notable efficacy in facilitating mitochondrial apoptosis. To develop nanomedicine, we enveloped CTT2P within bovine serum albumin (BSA), resulting in the formulation known as CTT2P@B. The molecular prodrug CTT2P is achieved by covalently conjugating mitochondrial targeting triphenylphosphine (PPh3), photosensitizer TPPOH2, ROS-sensitive thioketal (TK), and chemotherapeutic drug camptothecin (CPT). The prodrug, which is chemically bonded, prevents the escape of drugs while they circulate throughout the body, guaranteeing the coordinated dispersion of both medications inside the organism. Additionally, the concurrent integration of targeted photodynamic therapy and cascade chemotherapy synergistically enhances the therapeutic efficacy of pharmaceutical agents. Experimental results indicated that the covalently attached prodrug significantly mitigated CPT cytotoxicity under dark conditions. In contrast, TPPOH2, CTT2, CTT2P, and CTT2P@B nanoparticles exhibited increasing tumor cell-killing effects and suppressed tumor growth when exposed to light at 660 nm with an intensity of 280 mW cm−2. Consequently, this laser-triggered, mitochondria-targeted, combined photodynamic therapy and chemotherapy nano drug delivery system, adept at efficiently promoting mitochondrial apoptosis, presents a promising and innovative approach to cancer treatment.
Based on the most recent Global Cancer Statistics 2020 report, cancer has emerged as a primary or secondary factor contributing to mortality among individuals below the age of 70 in 112 nations worldwide (Sung et al., 2021). Chemotherapy, a conventional cancer treatment modality, often induces significant side effects due to its limited therapeutic specificity, bioavailability, and vulnerability to multidrug resistance (Li et al., 2018; Pan et al., 2021; Zou et al., 2021). Prodrugs provide a technique to accomplish targeted drug activation at the site of the tumor, liberating bioactive medications as needed in order to minimize overall toxicity. Prodrug activation mainly relies on utilizing distinct characteristics of the tumor microenvironment, such as reduced pH, glutathione concentrations, hypoxia, and specific enzyme expression. Additionally, external stimuli like light, heat, and ultrasound can also be employed for this purpose (Yin et al., 2019). Red and near-infrared light are attractive stimuli for prodrug activation due to their deep tissue penetration depth and low phototoxicity.
Phototriggered prodrugs have shown great potential in tumor treatment, given their non-invasive and remote spatiotemporal control capabilities, which are increasingly utilized in precision tumor treatment. When exposed to laser radiation, photosensitizers have the ability to produce significant amounts of reactive oxygen species (ROS) (O'Connor et al., 2009; Yi et al., 2018; Agwa et al., 2023; Yang et al., 2023; Yu et al., 2023). These ROS can eliminate cancer cells and break down ROS-sensitive chemical bonds, such as thioketal (TK) (Shi et al., 2018), alkyl thioether or selenide (Luo et al., 2019), aryl boronic acid or ester (Daum et al., 2017). Utilizing ROS-sensitive bonds to covalently link chemotherapeutic drugs and photosensitizers in the design of photo-triggered molecular prodrugs improves the specificity of chemotherapy drugs, reduces side effects, and synchronizes drug distribution in vivo (Liu et al., 2016; Xu et al., 2016; Jiang et al., 2020; Lebepe et al., 2021). However, despite allowing temporal and spatial control of drug release, diverse release sites within the cell can significantly impact drug therapeutic efficacy. Therefore, exploring strategies to optimize therapeutic outcomes is imperative.
Mitochondria, which are versatile organelles that control cellular bioenergy, calcium balance, redox equilibrium, and programmed cell death, are responsible for 90% of intracellular ROS generation (Nunnari and Suomalainen, 2012; Yue et al., 2016a; Xie et al., 2020). Mitochondria possess antioxidant systems to maintain redox homeostasis, and ROS at specific concentrations benefit normal cellular physiological functions. However, excessive ROS disrupts redox equilibrium, inducing mitochondrial death (Porporato et al., 2018; Wang et al., 2023). The mitochondrial genome lacks an effective DNA damage repair mechanism, making it a sensitive target for drugs that act on DNA and reverse chemotherapy resistance (Mallick et al., 2018). The close proximity of the photosensitizer to the target area greatly enhances the effectiveness of photodynamic therapy, as a result of the brief lifespan and restricted spread of ROS in biological systems (<0.1 ms, <20 nm) (Yuan et al., 2014). Delivering chemotherapeutic medicines and photosensitizers to mitochondria maximizes treatment efficacy.
Here, we developed CTT2P, a molecular prodrug targeting mitochondria. CTT2P comprises a triphenylphosphine cation, a porphyrin-type photosensitizer, a ROS-sensitive bond thioketal (TK), and covalently coupled camptothecin (CPT). During cancer treatment, CPT, a typical medicine for the mitochondrial apoptosis pathway, acts as an inhibitor of cellular respiration and DNA topoisomerase I (Liu et al., 2019; Zhang et al., 2019; Chen et al., 2023). CPT was chosen as a chemotherapeutic drug to promote the generation of ROS and induce cell apoptosis in synergism with the photosensitizer laser-induced dual pathway. Positively charged compounds are accumulated in the mitochondrial matrix of cancer cells due to their more negative membrane potential in the mitochondrial inner membrane compared to normal cells. With its excellent lipophilicity and positive charge, triphenylphosphine cation is a commonly utilized mitochondrial targeting group, facilitating rapid substance transport across the membrane to mitochondria (Mehta et al., 2017; Zielonka et al., 2017). Tumor cells, more sensitive to mitochondrial perturbations due to widespread metabolic reprogramming, benefit from prodrugs acting at the mitochondrial site, improving drug efficacy and efficiently promoting mitochondrial apoptosis, subsequently triggering cell apoptosis (Fu et al., 2019).
However, molecular prodrugs exhibit low solubility and are quickly metabolized in blood circulation, resulting in low bioavailability. Therefore, improving drug accumulation in tumor tissue becomes a practical consideration. Utilizing the enhanced permeability and retention (EPR) effect at the tumor site, nanoparticles (NPs) composed of either natural or synthetic substances efficiently administer a precise amount of molecular therapeutics to tumor tissue, proving to be an effective approach for drug delivery (Mayr et al., 2017; Luo et al., 2023).
In this study, we created the molecular drug CTT2P targeting mitochondria for ROS responsiveness (Scheme 1A, B). We encapsulated it through bovine serum albumin (BSA) as CTT2P@BSA NPs to prolong drug circulation, delivering CTT2P to the tumor site for maximal therapeutic efficacy with minimal side effects. First, CTT2 was obtained by covalently linking the porphyrin derivative TPPOH2 to the chemotherapeutic drug CPT using the ROS-sensitive bond TK. Derivating the 20-hydroxy group of CPT can decrease activity, reducing the side effects of chemotherapy drugs. (Hong et al., 2000). Then, triphenylphosphine PPh3 was covalently linked to CTT2 to achieve mitochondrial targeting of CTT2P. Finally, a nano-drug delivery system (CTT2P@B) was constructed by coating CTT2P with bovine serum albumin (BSA). Triphenyl phosphate groups target CTT2P to mitochondria after CTT2P@B releases the prodrug CTT2P in tumor cells. Low levels of ROS in tumor cells are insufficient to cleave TK bonds (Yin et al., 2019; Chu et al., 2020). However, irradiation with a 660 nm laser causes the photosensitizer component to generate ROS, significantly increasing ROS levels and enhancing cell damage.
SCHEME 1. Design of mitochondria-targeted ROS-responsive nanomedicine. (A) Schematic of each part of the CTT2P prodrug-linked compound and its encapsulation into CTT2P@B NPs by BSA. (B) Schematic diagram of CTT2P NPs release and treatment at local tumor sites.
Additionally, it cleaves the TK bond, releasing the photosensitizer and effective CPT in the mitochondria. CPT accumulation in mitochondria leads to ROS production (Zhang et al., 2019), increasing the breakage of the TK link and the cumulative release of CPT. This repeated superposition self-cycling action boosts mitochondrial apoptosis and triggers cell apoptosis with high efficiency and low toxicity.
Camptothecin (CPT), 3-mercaptopropionic acid, p-hydroxybenzaldehyde, benzaldehyde, 4-dimethylaminopyridine (DMAP), and 1,3-diphenylisobenzofuran (DPBF) were purchased from Shanghai Macklin Biochemical Technology Co., Ltd. (Shanghai, China). Pyrrole, 1-(3-dimethylaminopropyl)-3-ethylcarbodiimide hydrochloride (EDCI), (3-carboxypropyl) triphenyl phosphonium bromide (PPh3), and Bovine Serum Albumin (BSA) were purchased from Shanghai Aladdin Biochemical Technology Co., Ltd. (Shanghai, China). Propionic acid was purchased from Anhui Zesheng Technology Co., Ltd. (Shanghai, China). Mito-Tracker Green, Reactive Oxygen Species Assay Kit (DCFH-DA) was purchased from Beyotime Biotechnology Co., Ltd. (Shanghai, China). Annexin-V FITC/PI, Mitochondrial Membrane Potential Assay Kit (with JC-1) was purchased from Elabscience Biotechnology Co., Ltd. (Hubei, China). All other reagents were of analytical grade and used as received.
The synthesis of thioketal (TK) was carried out according to established procedures (Yue et al., 2016b). Anhydrous acetone (5.70 g, 98.20 mmol) and 3-mercaptopropionic acid (5.21 g, 49.10 mmol) were thoroughly mixed, and anhydrous hydrogen chloride gas was introduced. The reaction proceeded for 4 h at room temperature and was terminated. After crystallization in an ice water bath, the resulting product underwent filtration and was alternatively washed with hexane and cold water. Vacuum drying yielded the white powder product TK, with a 27. 3% yield. Confirmation of TK was achieved through 1H NMR spectroscopy (500 MHz, CDCl3): δ 2.90 (t, 4H), 2.68 (t, 4H), 1.59 (s, 6H).
For the synthesis of TPPOH2, p-hydroxybenzaldehyde (45.00 mmol, 5.50 g) and benzaldehyde (45.00 mmol, 4.57 mL) were added to 150 mL of propionic acid and heated to reflux. Within a time frame of 20 min, Pyrrole (90.00 mmol, 6.23 mL) was introduced into a constant pressure funnel. The mixture was then stirred at a temperature of 140°C for a duration of 90 min. Following the reaction, vacuum concentration removed the propionic acid, producing an oily liquid. The liquid was dissolved in a mixture of dichloromethane and methanol (in a ratio of 70:1), then filtered. The resulting solution was concentrated under vacuum to obtain the crude product. Separation and purification through silica gel column chromatography (100–200 mesh) with dichloromethane: methanol ratios of 50:1 and 100:1 yielded the purple product TPPOH2, with a 2.4% yield. The confirmation was done using 1H NMR (600 MHz, DMSO-d6): δ 9.97 (s, 2H), 8.89 (s, 4H), 8.80 (s, 4H), 8.21 (d, J = 6.3 Hz, 4H), 8.01 (d, J = 8.2 Hz, 4H), 7.86–7.79 (m, 6H), 7.21 (d, J = 8.2 Hz, 4H), −2.90 (s, 2H), and ESI-MS: m/z calculated for C44H30N4O2 [M + H]+: 647.2441.
The thioketal (TK) (10.08 mg, 0.04 mmol), 4-dimethylaminopyridine (DMAP) (5.86 mg, 0.048 mmol), and 1-(3-dimethylaminopropyl)-3-ethylcarbodiimide hydrochloride (EDCI) (9.20 mg, 0.048 mmol) were combined and added to 5 mL of dichloromethane in a concise process and stirred in an ice bath for 20 min. Subsequently, dropwise, 3 mL of dichloromethane-dissolved CPT (6.97 mg, 0.02 mmol) was meticulously added and stirred for 24 h at room temperature. Post-reaction, the solution underwent vacuum concentration to yield the resultant mixture. This mixture underwent separation and purification via silica gel column chromatography utilizing dichloromethane:methanol gradient elution ranging from 70:1 to 50:1. Ultimately, the white product TK-CPT was obtained with a yield of 40%. TK-CPT was confirmed using 1H NMR and mass spectrometry (MS). 1H NMR (600 MHz, DMSO-d6): δ 8.70 (s, 1H), 8.13 (q, 2H), 7.87 (t, J = 7.6 Hz, 1H), 7.72 (t, J = 7.5 Hz, 1H), 7.24 (s, 1H), 5.50 (s, 2H), 5.32 (q, 2H), 2.94–2.76 (m, 4H), 2.69 (t, J = 7.2, 3.4 Hz, 2H), 2.38 (t, J = 7.0 Hz, 2H), 2.19–2.11 (m, 2H), 1.57 (s, 3H), 1.50 (s, 3H), 0.93 (t, J = 7.4 Hz, 3H). ESI-MS: m/z calculated for C29H30N2O7S2 [M + H]+: 583.1565.
TK-CPT (11.65 mg, 0.02 mmol), DMAP (2.93 mg, 0.024 mmol), and EDCI (4.60 mg, 0.024 mmol) were included in 5 mL of dichloromethane and mixed in an ice bath for a duration of 20 min. After that, a solution of TPPOH2 (25.87 mg, 0.04 mmol) dissolved in 4 mL of dichloromethane was added once, and the amalgamation reacted at room temperature for 24 h. Subsequently, the solution was subjected to vacuum concentration to obtain the resulting mixture. The resulting combination was isolated and refined using a silica gel column chromatography method, employing a gradient elution of dichloromethane and methanol ranging from 100:1 to 70:1. Ultimately, the purple product CTT2 was obtained with a commendable yield of 86%. CTT2 was confirmed using 1H NMR and mass spectrometry (MS). 1H NMR (600 MHz, DMSO-d6): δ 9.98 (s, 1H), 8.90 (d, J = 12.0 Hz, 2H), 8.80 (d, J = 13.6 Hz, 6H), 8.38 (s, 1H), 8.25–8.19 (m, 6H), 8.06 (d, J = 8.5 Hz, 1H), 8.01 (d, J = 8.3 Hz, 2H), 7.89 (d, J = 8.0 Hz, 1H), 7.86–7.80 (m, 6H), 7.77 (t, 1H), 7.57 (t, J = 7.5 Hz, 1H), 7.49 (d, J = 8.3 Hz, 2H), 7.25 (s, 1H), 7.21 (d, J = 8.4 Hz, 2H), 5.50 (s, 2H), 5.07 (q, 2H), 3.01–2.87 (m, 8H), 2.15 (q, J = 7.3 Hz, 2H), 1.68 (s, 3H), 1.63 (s, 3H), 0.94 (t, J = 7.4 Hz, 3H), −2.96 (s, 2H). ESI-MS: m/z calculated for C73H58N6O8S2 [M + H]+: 1211.3829.
(3-Carboxypropyl) triphenyl phosphonium bromide (PPh3, 4.29 mg, 0.01 mmol), DMAP (1.47 mg, 0.012 mmol), and EDCI (2.30 mg, 0.012 mmol) were meticulously measured and introduced into 2 mL of dichloromethane and mixed in an ice bath for a duration of 20 min. Subsequently, 3 mL of CTT2 (12.11 mg, 0.01 mmol) dissolved in dichloromethane was carefully added to the reaction solution, and the amalgamation underwent stirring for 24 h at room temperature. Post-reaction, the solution underwent vacuum concentration to yield the resultant mixture. This resultant mixture was separated and purified using silica gel column chromatography, employing a gradient elution of dichloromethane: methanol within the range of 70:1 to 20:1. Ultimately, the purple-black product CTT2P was obtained, demonstrating a commendable yield of 66%. The structure of CTT2P was confirmed using 1H NMR and mass spectrometry (MS). 1H NMR (600 MHz, DMSO-d6): δ 8.93–8.71 (m, 8H), 8.38 (s, 1H), 8.27 (d, J = 8.2 Hz, 2H), 8.25–8.19 (m, 6H), 8.07 (d, J = 8.5 Hz, 1H), 7.97–7.89 (m, 10H), 7.87–7.81 (m, 12H), 7.78 (t, 1H), 7.62 (d, J = 8.3 Hz, 2H), 7.58 (t, J = 7.5 Hz, 1H), 7.50 (d, J = 8.3 Hz, 2H), 7.25 (s, 1H), 5.50 (s, 2H), 5.05 (q, 2H), 3.86–3.79 (m, 2H), 3.08–3.04 (m, 2H), 3.02–2.88 (m, 8H), 2.15 (q, J = 7.4 Hz, 2H), 2.09–2.00 (m, 2H), 1.68 (s, 3H), 1.63 (s, 3H), 0.94 (t, J = 7.4 Hz, 3H), −2.98 (s, 2H). ESI-MS: m/z calculated for C95H78N6O9S2P+Br− [M-Br-]+: 1541.4982.
10 mg of CTT2P was dissolved in d6-DMSO and irradiated with a 280 mW cm−2, 660 nm laser for 1 min and 5 min, respectively. The ROS response of CTT2P was observed using 1H NMR.
CTT2P@B NPs were synthesized using a desolvation method. Specifically, 15 mg of Bovine Serum Albumin (BSA) was dissolved in 1 mL of deionized water. After stirring to achieve complete dissolution, the pH was accurately set to 9.0 by adding a 0.1 M NaOH solution. Afterwards, a solution of CTT2P dissolved in acetone, measuring 4 mL, was added to the aqueous phase at a steady rate of 1 mL min−1, while keeping the stirring rate at 500 r·min-1. After stirring for 30 min, 10 μL of 8% glutaraldehyde was incorporated, and the solution underwent a curing process for 12 h. Finally, the solution was centrifuged at 12,000 rpm for 20 min. The resulting precipitate was subjected to two additional resuspension and centrifugation with deionized water. The supernatant was retained, and the precipitate was re-dispersed in 2 mL of deionized water before being stored at 4°C.
The Zetasizer nanoZS (Malvern Instruments) was used to determine the particle size and zeta potential of the nanomedicine CTT2P@B NPs. Furthermore, the shape of CTT2P@B NPs was observed using the TEM (JEM-1400 microscope).
UV-vis spectroscopy was used to measure the absorbance of the supernatant, which was retained after centrifugation, at 415 nm. From the standard curve of CTT2P, the calculation of CTT2P@B NPs’ encapsulation efficiency and drug loading was determined.
The absorbance of TPPOH2, CTT2, CTT2P, and CTT2P@B in DMSO was measured using UV-vis spectroscopy. The fluorescence emission spectra of TPPOH2, CTT2, CTT2P, and CTT2P@B under 420 nm excitation were measured using a multifunctional microplate reader.
In vitro release assays were conducted employing a centrifugation-based methodology. CTT2P@B NPs were evenly distributed in 1.5 mL of PBS (pH 7.4 and 5.0) containing 10% ethanol. Subsequently, they were placed in a constant-temperature shaker and incubated at 37°C. The nanosuspension was subjected to centrifugation at 12,000 r·min−1 for 10 min at specific time intervals (0.25, 0.5, 1, 2, 4, 6, 8, 10, 12, 24, 36, and 48 h), and 1 mL of supernatant was collected and supplemented with 1 mL of fresh buffer. Quantifying the CTT2P drug within the supernatant was executed utilizing UV-vis spectroscopy, facilitating the computation of the release rate at each designated time point.
Solutions of TPPOH2, CTT2, CTT2P, and CTT2P@B NPs, each with a concentration of 5 μM and containing DPBF, were prepared. The DPBF concentration was set at 150 μg mL−1. Subsequently, a 2 mL sample solution was placed in a 24-well plate. In order to commence the experiment, every specimen was exposed to light with a wavelength of 660 nm and an intensity of 280 mW cm−2 for a duration of 16 min. UV-vis spectroscopy at 420 nm was diligently recorded at 1-min intervals throughout the irradiation period. The manifestation of generated singlet oxygen was indicated by the discernible reduction in DPBF absorption at 420 nm.
The murine breast cancer cells (4T1) were procured from Icell Biotech Co., Ltd. (Shanghai, China). The 4T1 cells were cultivated in Dulbecco’s Modified Eagle’s Medium (DMEM) supplemented with 10% (v/v) fetal bovine serum (FBS) and 1% (v/v) penicillin/streptomycin. The cells were cultivated in a cell incubator maintained at a temperature of 37°C with 5% CO2 (v/v).
To conduct experiments, 4T1 cells were placed in 12-well plates with a density of 1.5 × 105 cells per well. After incubating for 24 h, the 4T1 cells were treated with 5 μM CPT, TPPOH2, CTT2, CTT2P, and CTT2P@B NPs individually for a duration of 6 h. Afterwards, the cells were treated with 10 μM DCFH-DA for a duration of 20 min, followed by a PBS wash. Subsequently, the cells were subjected to laser irradiation at a wavelength of 660 nm and intensity of 280 mW cm−2 for a period of 1 min. The intracellular production of ROS was visualized using fluorescence microscopy.
The intracellular localization of different categories of drugs was examined using Confocal Laser Scanning Microscopy (CLSM). In summary, 4T1 cells were grown in confocal dishes with a cell density of 50,000 cells per well. After 24 h of incubation, the existing medium was removed, and TPPOH2, CTT2, CTT2P, and CTT2P@B NPs (with a photosensitizer concentration of 10 μM), diluted in fresh medium, were introduced. Afterwards, Mito-tracker Green (200 nM) was added to all samples and incubated together in fresh medium for 30 min to mark the mitochondria. In the end, the liquid was removed, cells were washed with PBS, and a small amount of new liquid was introduced to each well prior to being placed under CLSM for observation. The excitation wavelength and emission spectra for each fluorescence index were as follows: TPPOH2, CTT2, CTT2P, and CTT2P@B NPs were excited at 405 nm, with fluorescence emission spectra collected at 600–660 nm. Mito-Tracker Green was excited at 488 nm, and fluorescence emission spectra were collected from 510 to 540 nm.
Mitochondrial depolarization in 4T1 cells was assessed employing the JC-1 probe. Briefly, 4T1 cells were placed in 12-well plates with a concentration of 2 × 105 cells per well. After being incubated at 37°C for 24 h, the cells were subjected to 5 μM of CPT, TPPOH2, CTT2, CTT2P, and CTT2P@B NPs for a duration of 6 h. Then, the cells were exposed to a 660 nm, 280 mW cm−2 laser for 1 min, followed by an additional 18-h incubation period. The control group cells were treated with medium or carbonyl cyanide m-chlorophenyl hydrazone (CCCP) to ascertain the status of the membrane potential whether it remained normal or dissipated. Following the incubation period, the cells were rinsed with PBS, trypsinized, and then gathered in centrifuge tubes to be centrifuged. Afterward, 500 μL of JC-1 working solution were introduced and left to incubate at a temperature of 37°C for a duration of 20 min. After centrifugation, the liquid portion was discarded, and the cells were washed and centrifuged once using JC-1 Assay Buffer. Finally, the cells were resuspended to create a cell suspension. Flow cytometry analysis was performed using 525 nm (FITC channel) and 585 nm (PE channel) bandpass filters, with excitation at 488 nm.
4T1 cells were seeded in 96-well plates at a concentration of 5,000 cells per well using 100 μL of culture medium and incubated for 24 h. Afterwards, the cells were treated with different solutions of CPT, TPPOH2, CTT2, CTT2P, and CTT2P@B NPs (drug loading concentrations of 0.5, 1, 2, 5, 10, and 20 μM). For dark toxicity assessment, cells were exposed to drugs for a period of 24 h while being protected from any light exposure. For the assessment of phototoxicity, the cells underwent exposure to a laser with a wavelength of 660 nm and power density of 280 mW·cm−2 for a duration of 1 min, following continuous culturing for 6 h. After the irradiation, the cells were further cultured for 18 h. Then, a combination of CCK-8 and DMEM medium was introduced and incubated with the cells for 2 h, and the absorbance at 450 nm was determined using a microplate reader.
4T1 cells were seeded in 6-well dishes with a density of 5 × 105 cells per well and incubated for 24 h. The cells were treated with CPT, TPPOH2, CTT2, CTT2P, and CTT2P@B NPs solutions (drug concentration of 5 μM) for 6 h. Following that, a laser with a wavelength of 660 nm and an intensity of 280 mW cm−2 was used for 1 min of irradiation. The culture was then allowed to continue for 18 h post-irradiation. After two washes with PBS, the plates were trypsinized without EDTA, and the cells were collected in centrifuge tubes for centrifugation. Binding Buffer was added after resuspending and washing the single-cell suspensions with PBS. After being treated with Annexin-V FITC and PI for a duration of 15 min, the cells underwent evaluation of apoptosis using a flow cytometer (BD Canto II).
Blood was obtained from mice, and erythrocytes were separated by centrifugation. The erythrocytes were subsequently diluted by washing with PBS before use. The red blood cell suspension was mixed with 10 μM solutions of CPT, TPPOH2, CTT2, CTT2P, and CTT2P@B NPs in the experiments. After incubation at 37°C for 2 h, the samples were centrifuged to collect the supernatant, which was then subjected to UV-vis spectroscopy analysis at 540 nm. PBS and Triton X-100 served as the negative and positive controls, respectively. Hemolysis was calculated using the following formula:
All Balb/c mice (4 weeks old, female) were procured from Jinan Pengyue Experimental Animal Breeding Co., Ltd. All animal studies followed the guidelines and protocols approved by the Animal Care Agency of Binzhou Medical University. The 4T1 tumor-bearing mouse model was established by subcutaneously injecting a 4T1 cell suspension (1 × 106 cells) into the right abdomen of the mice. Mice were utilized for biodistribution and NIR fluorescence imaging studies when the tumor volume reached 200 mm3. Antitumor therapy was conducted using mice that had a tumor volume of 100 mm3.
The 4T1 tumor-bearing mice had their skin shaved and were divided into three groups randomly (n = 3 in each group). The mice were intravenously injected with 200 μL of CTT2P and CTT2P@B NPs (both containing 100 μg CTT2P). Mice were anesthetized and imaged using the IVIS Spectrum (Perkin-Elmer, United States) at 2, 4, 8, 10, and 24 h after injection. The imaging involved an excitation wavelength of 640 nm and a fluorescence emission wavelength of 700 nm. After 24 h post-injection, the mice, as mentioned above, were euthanized, and major organs were collected and imaged.
In each group, the mice with 4T1 tumors were randomly divided into six groups (n = 5) and given NS or CPT, TPPOH2, CTT2, CTT2P, and CTT2P@B NPs intravenously. Each group was administered a single dose every other day, which is equal to a CPT dosage of 2 mg kg−1 Cel. After 10 h of injection, the tumors in the laser irradiation groups were subjected to a 660 nm laser for a duration of 1 min, with a power intensity of 280 mW cm−2. Subsequently, the therapeutic efficacy of each group was assessed by measuring tumor size every 2 days. The formula for calculating tumor volume is tumor volume (mm3) = (W2 × L)/2, where L denotes the greater diameter of the tumor and W denotes the lesser diameter of the tumor. After 12 days, the mice were euthanized and their organs (including the heart, liver, spleen, lung, and kidney) along with solid tumor tissues were removed for histological examination. The examination was conducted using the standard H&E staining method. TUNEL immunohistochemical stains were performed to analyze tumor tissue apoptosis.
The mean ± SD was used to express the quantitative data. The statistical analysis was performed utilizing the software GraphPad Prism 8.0. To compare two groups, the Student's t-test was used, while for comparing multiple groups, a one-way analysis of variance (ANOVA) was employed. Statistical significance was determined for p-values below 0. 05 (*p < 0. 05, **p < 0. 01, ***p < 0. 001, ****p < 0. 0001).
The molecular prodrug CTT2P is primarily synthesized through a three-step reaction (Supplementary Scheme S1). To begin with, TPPOH2 and the TK sensitive to ROS were created using the method described in the source. The structures of these compounds were validated through 1H NMR and ESI-MS analyses (Supplementary Figures S1−S3). Subsequently, CTT2, a novel ROS-cleavable compound, was synthesized by covalently coupling CPT with TPPOH2 using TK. Finally, the molecular prodrug CTT2P was synthesized from (3-carboxypropyl) triphenyl phosphonium bromide and CTT2 through an esterification reaction. The structures of the described compounds were confirmed through 1H NMR and ESI-MS analyses (Supplementary Figures S4−S9). We then measured the ROS response release for CTT2P. Supplementary Figure S10 illustrates a significant reduction in the NMR peak of the TK part of CTT2P after 1 min and 5 min of illumination, and a significant shift in the chemical shift of the CPT part of CTT2P. This indicates that there is enough ROS for the compound CTT2P to break TK bonds when exposed to a 280 mW cm 2, 660 nm laser for 5 min.
The prodrug, which is bound covalently, reduces the escape of the drug while it circulates throughout the body, guaranteeing a one-to-one ratio between the target and the administered drug in vivo. Due to its positive charge, the triphenylphosphine group facilitates targeting the mitochondria of cancer cells. Upon exposure to laser light, TK in CTT2P is cleaved, releasing CPT in response to the abundant ROS generated by the photosensitizer and those present in the mitochondria themselves. Hydrophobic CPT was selected as the chemotherapeutic drug not only for its anticancer activity and ease of modification but also for its ability to inhibit cellular respiration, leading to an excessive production of ROS in mitochondria (Sen et al., 2004). Conversely, the excessive ROS produced by photosensitizers and CPT can effectively induce mitochondrial apoptosis, triggering cell apoptosis.
We employed desolvation to encapsulate CTT2P into BSA nanoparticles, yielding CTT2P@B NPs. To validate the successful synthesis of CTT2P@B, blank BSA NPs were synthesized as a control using the same method. The size and distribution of hydrated particles in blank BSA NPs and CTT2P@B NPs were first measured using dynamic light scattering (DLS) (Figures 1A,B). The results revealed that the hydrated particle sizes of blank BSA NPs and CTT2P@B NPs were 64.35 ± 5.09 nm and 92.89 ± 4.06 nm, respectively. Additionally, the potentials of blank BSA NPs and CTT2P@B NPs were −19.37 ± 0.70 mV and −36.13 ± 0.68 mV, respectively. The CTT2P@B NPs exhibited a size approximately 28 nm more prominent than the blank BSA NPs, and the potential had an absolute value of approximately 17 mV higher. These notable changes in particle size and potential indicated the successful encapsulation of CTT2P in BSA.
FIGURE 1. Analysis of CTT2P@B nanoparticles. (A) Particle size distribution of blank BSA NPs and CTT2P@B NPs. (B) Zeta potential of blank BSA NPs and CTT2P@B NPs. (C) Stability of CTT2P@B NPs in deionized aqueous solution. (D) TEM images of blank BSA NPs and CTT2P@B NPs. Scale bar = 200 nm. (E) The release of CTT2P from CTT2P@B NPs in a PBS solution at 37°C was examined using in vitro testing.
Furthermore, as indicated by prior research, the higher the magnitude of the potential, the increased stability of the nanoparticles was observed (Khan et al., 2016). This implies that CTT2P@B NPs exhibited enhanced stability compared to blank BSA NPs. Notably, the negatively charged surface of the NPs enhances their resistance to nonspecific protein adsorption in the bloodstream, ultimately prolonging their circulation time, as observed in other studies (Yuan et al., 2012). The results obtained from transmission electron microscopy (TEM) were consistent with the measured hydrated particle size using dynamic light scattering, confirming that CTT2P@B NPs are indeed more significant than their blank BSA counterparts (Figure 1D). All these indicators strongly suggest the successful encapsulation of CTT2P in BSA. TEM analysis revealed that CTT2P@B NPs exhibited a uniform distribution and good morphology, appearing as spherical particles. Subsequently, we evaluated the stability of CTT2P@B NPs in an aqueous solution. As shown in Figure 1C, The dimensions and polydispersity index (PDI) of CTT2P@B NPs remained stable over the course of 1 week, suggesting exceptional nanoparticle stability.
Utilizing the UV-vis spectroscopy of CTT2P, the standard curve was used to determine the encapsulation efficiency and drug loading of CTT2P@B NPs. The CTT2P@B NPs exhibited an encapsulation efficiency of 43.98% ± 2.38% and a drug loading of 2.75% ± 0.15%. Next, we measured the spectral properties of TPPOH2, CTT2, CTT2P, and CTT2P@B (Supplementary Figures S11, S12). As observed in the UV spectra, the maximum absorbance of TPPOH2, CTT2, and CTT2P around 420 nm gradually decreased with the successive modification of chemical bonds. However, due to BSA encapsulation, CTT2P@B exhibits a BSA UV absorption peak around 280 nm, and the maximum absorbance at 420 nm is smaller than that of CTT2P. Similarly, based on the fluorescence emission patterns, the maximum emission wavelengths of TPPOH2, CTT2, CTT2P, and CTT2P@B gradually decreased. Additionally, we examined the drug release pattern of CTT2P@B NPs in simulated physiological and tumor-acidic conditions. The drug release rate of CTT2P@B NPs in PBS solutions with pH values of 7.4 and 5.0 was evaluated by examining the cumulative release over time (Figure 1E), the results indicated a faster drug release under acidic conditions. This accelerated release is attributed to BSA’s self-degradation and erosion in acidic environments, aligning with our designed system. In the acidic environment of tumor cells, CTT2P@B NPs can more readily release the molecular drug CTT2P into tumor cells, facilitating the effective targeting of mitochondria and the intended therapeutic function.
1,3-Diphenylisobenzofuran (DPBF) can react with singlet oxygen (1O2) generated during the process. The absorption intensity of DPBF at 420 nm decreases with an increase in the concentration of 1O2 (Xu et al., 2018). Hence, DPBF functioned as a tool for assessing the ability of medications to produce singlet oxygen (1O2). Initially, we examined whether there was 1O2 release from the drug under dark conditions. In Figure 2A, based on the data, it is clear that the DPBF, TPPOH2, CTT2, CTT2P, and CTT2P@B NPs did not show any noticeable alterations in absorbance when kept in darkness. This suggests that the photosensitizer created did not generate 1O2 without light. We confirmed the release of 1O2 from the synthesized photosensitizer under laser irradiation by utilizing the absorbance change of the drug in the dark as a control. Figure 2B shows that the absorbance of DPBF also did not undergo significant changes under laser irradiation conditions, indicating that the presence of DPBF did not interfere with the experiment. The slope and degree of absorbance drop represent the rate of 1O2 production. The slopes of TPPOH2, CTT2, and CTT2P are nearly identical, suggesting a consistent rate of 1O2 release and an excellent ability to produce 1O2. However, the slope of absorbance decline for CTT2P@B NPs was slower than TPPOH2, CTT2, and CTT2P. The presence of nanoparticles leads to a decrease in the rate of 1O2 emission, resulting in a reduction of laser irradiation intensity.
FIGURE 2. ROS generation detection in vitro. (A) The release of singlet oxygen from each drug was detected in vitro under dark conditions. Data were presented as the mean ± SD (n = 3). (B) Detection of singlet oxygen release of each drug under 660 nm laser irradiation at a power density of 280 mW cm-2 was performed in vitro. Data were presented as the mean ± SD (n = 3). (C) ROS generation was observed in 4T1 cells treated with each medication while being exposed to laser irradiation at a wavelength of 660 nm and intensity of 280 mW cm−2. Bright: Bright field. DCF: Green fluorescence represents intracellular ROS. Merge: Superimpose the image. Scale Bar = 100 μm.
Additionally, DCFH-DA staining was utilized to analyze the generation of intracellular ROS in 4T1 cells across all drug groups. DCFH-DA, an impermeable indicator of cellular permeability for reactive oxygen species (ROS), is hydrolyzed by esterases within cells and then oxidized by ROS to generate the fluorescent compound DCF. As observed through a fluorescence microscope (Figure 2C), the CPT group did not display noticeable fluorescence. In contrast, the TPPOH2, CTT2, CTT2P, and CTT2P@B NPs groups exhibited significant fluorescence, indicating that drugs modified with a photosensitizer still retained the ability to generate ROS upon irradiation in cells.
To assess the drugs’ capacity to target mitochondria, the drugs were administered to 4T1 cells for a duration of 6 h, and the co-localization of the drug was analyzed using CLSM. Since our drugs were explicitly designed for mitochondrial targeting, we assessed mitochondrial localization (Figure 3A) through fluorescent double staining. We utilized the Mito-Tracker Green, a fluorescent probe for mitochondria, along with the red fluorescence emitted by our designed drugs. The yellow area in the overlapping images indicates the colocalization of the photosensitizer with Mito-Tracker Green. The results revealed that cells treated with CTT2P and CTT2P@B NPs exhibited clear colocalization with Mito-Tracker Green, in contrast to TPPOH2 and CTT2. However, the CTT2P@B NPs displayed a more pronounced yellow area than CTT2P alone, as the nanodrugs were more easily taken up by the cells, resulting in increased drug accumulation in the mitochondria. This observation aligns with our design concept; CTT2P and CTT2P@B NPs demonstrate effective mitochondrial localization, targeting the mitochondria upon entering tumor cells under the influence of triphenylphosphine groups.
FIGURE 3. Evaluation of mitochondrial targeting function. (A) Confocal images of the mitochondrial sites of TPPOH2, CTT2, CTT2P, and CTT2P@B NPs in 4T1 cells. Mito-Tracker Green was used to stain the mitochondria in the green channel. The red channel was derived from the emission of the photosensitizer fraction (PS) itself. Merge stands for superimposed image. The Green and red curves in the Plot Profile represent the gray value of Mito-Tracker Green and PS, respectively. Scale bar = 20 μm. (B) Flow cytometry JC-1 method was used to analyze the mitochondrial function of cells treated with different drugs. Red fluorescence: normal mitochondria (J-aggregate); Green fluorescence: depolarized mitochondria (J-monomer).
Next, we evaluated drug-induced impairment of mitochondria in every group by examining the potential of mitochondrial membrane using the positively charged dye JC-1. Prior research has shown that the JC-1 probe is effective in identifying mitochondrial harm. In normal mitochondria, the green monomers of JC-1 can enter the cytoplasm and accumulate, forming numerous red J-aggregates. A change in fluorescence color from red to green suggests the presence of membrane potential decline and considerable damage to the mitochondria (Zhang et al., 2019). Therefore, we quantified mitochondrial damage in 4T1 cells induced by each drug using flow cytometry. The green fluorescence channel (FITC) represented “J-monomer,” while the red fluorescence channel (PE) represented “J-aggregate.” As illustrated in Figure 3B, after being exposed to laser, the CPT, TPPOH2, CTT2, CTT2P, and CTT2P@B NPs groups experienced a decrease in mitochondrial membrane potential (in the right lower quadrant) with loss rates of 35.8%, 19.1%, 40.1%, 52.8%, and 62.1%, respectively. The data indicate that CPT can induce moderate mitochondrial dysfunction, which is consistent with previous reports (Sen et al., 2004). Meanwhile, triphenylphosphine-modified CTT2P caused more damage to mitochondria than TPPOH2 and CTT2 because it more easily accumulated at the mitochondrial site. CTT2P@B NPs could enter cells more quickly and accumulate more drugs at the mitochondrial site, resulting in a higher rate of mitochondrial damage than the molecular drug CTT2P.
Mitochondria play a vital role in cellular respiration and serve as a significant generator of reactive oxygen species (ROS). Therefore, drugs targeting mitochondria can achieve maximum therapeutic efficacy. In order to examine the in vitro anticancer properties of the synthesized medications, we first evaluated the toxic effects of different drugs on 4T1 cells through the CCK-8 assay. 4T1 cells were treated with CPT, TPPOH2, CTT2, CTT2P, and CTT2P@B NPs in the dark or under laser irradiation (Figures 4A,B). Under dark conditions, TPPOH2, CTT2, CTT2P, and CTT2P@B nanoparticles exhibited more than 80% cell viability at concentrations below 10 μM, showing minimal harm to cells in contrast to CPT. CTT2 and CTT2P enhance the stability of the CPT lactone ring and reduce cytotoxicity by modifying the 20-hydroxyl group of CPT. When the concentration of CTT2, CTT2P, and CTT2P@B NPs was above 10 μM, CTT2, and CTT2P, as well as CTT2P@B NPs, showed obvious cytotoxicity in the dark, but CTT2P@B NPs showed less cytotoxicity than CTT2P. This indicates that high concentrations of CTT2, CTT2P, and CTT2P@B NPs still retain some cytotoxicity due to the presence of CPT under dark conditions. Simultaneously, CTT2P@B nanoparticles have the ability to decrease the cytotoxic effects of drugs by facilitating sustained release. When exposed to laser radiation (at a wavelength of 660 nm and intensity of 280 mW cm−2), TPPOH2, CTT2, CTT2P, and CTT2P@B NPs exhibited a significant reduction in cell viability compared to the CPT group. This indicates a dose-dependent and gradually escalating cytotoxic effect. This suggests that ROS-induced TK breakdown and CPT release enhance cell damage, further amplified by drugs targeting mitochondria. Similarly to the results obtained in the dark, the cytotoxicity of a high concentration (20 μM) of CTT2P@B NPs was reduced compared to CTT2P. This reduction was attributed to the relatively small amount of drug accumulation caused by the high concentration of CTT2P@B NPs.
FIGURE 4. Evaluation of cell death in vitro through cytotoxicity assessment and examination of apoptosis and necrosis. (A) The in vitro cytotoxicity of 4T1 cells treated with CPT, TPPOH2, CTT2, CTT2P, and CTT2P@B NPs in the dark was assessed using the CCK-8 assay. Data were presented as the mean ± SD (n = 5). **p < 0.01, ****p < 0.0001. (B) The in vitro cytotoxicity of 4T1 cells treated with TPPOH2, CTT2, CTT2P and CTT2P@B NPs under laser irradiation (660 nm, 280 mW cm−2) was determined using the CCK-8 assay. Data were presented as the mean ± SD (n = 5). **p < 0.01, ****p < 0.0001. (C) Cell apoptosis and necrosis were analyzed using flow cytometry with Annexin V-FITC/PI double staining following treatment with various drugs at a concentration of 5 μM.
Apoptosis rates, which include both early and late apoptosis, were examined through the use of the Annexin V-FITC/PI apoptosis detection kit and flow cytometry. As depicted in Figure 4C, laser-irradiated CTT2P@B NPs induced a significantly higher apoptosis rate (85.82%) compared to that of free drugs CTT2P (63%), CTT2 (38.8%), TPPOH2 (31.3%), and CPT (46.7%) under the same conditions. This result aligns with the trend observed in the in vitro cytotoxicity results and indicates that the cytotoxicity of the drugs is associated with both early and late apoptosis. Furthermore, it confirms that the prodrug CTT2P, modified with a mitochondrial targeting group, exhibits a more effective anti-tumor effect after being encapsulated with BSA.
First, the hemolysis test verified the blood compatibility of each drug. As depicted in Figure 5A, the hemolysis rate of CPT, TPPOH2, CTT2, CTT2P, and CTT2P@B NPs at high concentrations did not exceed 5%. This indicates that the drugs exhibit good blood compatibility and can be safely administered via tail vein injection. We then investigated the biodistribution and drug delivery of CTT2P and CTT2P@B NPs in 4T1 tumor-bearing mice. CTT2P, possessing near-infrared fluorescence, obviates the need for physical packaging with other dyes, allowing it to integrate diagnosis and therapy. To monitor the distribution of medication in the body, an in vivo imaging system (IVIS) was utilized for fluorescence imaging. Images were captured at specific time intervals. As illustrated in Figure 5B and Supplementary Figure S13, higher accumulation was observed at the tumor site of mice treated with free CTT2P at 10 h. However, no significant accumulation occurred at 24 h, indicating rapid clearance of free CTT2P in mice.
FIGURE 5. Biodistribution in vivo. (A) Blood compatibility test of CPT, TPPOH2, CTT2, CTT2P, CTT2P@B NPs. Data were presented as the mean ± SD (n = 3). ***p < 0.001, ****p < 0.0001. (B) Time-lapse live fluorescence imaging of mice with 4T1 tumors following the administration of free CTT2P and CTT2P@B NPs via intravenous injection. (C) Fluorescent images of major organs and tumors were obtained 24 h after injection, using ex vivo methods. (D) The mean fluorescence intensity of each organ and tumor was used to determine the biodistribution of free CTT2P and CTT2P@B NPs in mice. Data were presented as the mean ± SD (n = 3). *p < 0.05.
On the other hand, CTT2P@B NPs showed notable buildup after 4 h, which lasted until 24 h. The fluorescence intensity at the tumor location reached its peak approximately 10 h post-injection, suggesting the highest accumulation of CTT2P@B NPs. Furthermore, ex vivo analysis of major organs and tumors was conducted 24 h post-injection to determine their distribution. As demonstrated in Figures 5C,D, in the heart and kidney of both groups, there were no apparent fluorescence signals, suggesting that neither drug group showed any signs of cardiotoxicity or nephrotoxicity. Liver and tumor tissues exhibited a notably elevated signal in the CTT2P@B NPs group compared to the free CTT2P group. The enhanced fluorescence signal in the entire circulatory system and ex vivo tumor tissues at the exact CTT2P dosage supports the long-circulating effect and tumor-targeting capabilities of CTT2P@B NPs.
The therapeutic efficacy of various drug groups on 4T1-bearing mice was validated through tail vein injection of CPT at a dosage equivalent to 2 mg kg−1. Based on the in vivo distribution results, laser irradiation was conducted 10 h after drug injection. Tumor images and tumor volume curves are depicted in Figures 6A,B. Tumor volume increased rapidly in mice treated with NS after laser irradiation, indicating an inability to impede tumor progression. The tumor volumes in the CPT and TPPOH2 groups were comparable. However, the anti-tumor effect was inadequate, suggesting that neither chemotherapy nor photodynamic therapy alone could inhibit tumor growth. The CTT2 group exhibited a more pronounced anti-tumor effect than the CPT and TPPOH2 groups. However, it still fell short of the CTT2P group, indicating that CTT2P, a molecular drug targeting the mitochondrial region, may produce a more potent cascade effect and a more significant therapeutic impact due to its proximity to the target. Due to enhanced blood circulation time and improved accumulation at the tumor site, the mice in the CTT2P@B group exhibited the highest level of tumor inhibition and anti-tumor impact. The in vivo tumor-suppressing properties of TPPOH2, CTT2, CTT2P, and CTT2P@B NPs were enhanced when exposed to laser radiation, in line with the findings from in vitro tests on cell toxicity and programmed cell death. This further validates that the molecular drug CTT2P, modified with a mitochondrial targeting group, exhibits superior therapeutic efficacy after encapsulation in BSA.
FIGURE 6. In vivo anti-tumor study of each drug in 4T1 tumor-bearing mice. (A) Tumor images of different drug administration treatments after the antitumor study. (B) During the administration, the growth of tumors in mice was observed in each group receiving treatment. Data were presented as the mean ± SD (n = 5), **p < 0.01. (C) The weight of mice in each treatment group was monitored throughout the administration period. Data were presented as the mean ± SD (n = 5).
Furthermore, the mice’s body weight and major organ tissue sections were analyzed using hematoxylin-eosin (H&E) staining to evaluate the biological safety of each treatment group. As depicted in Figure 6C, during the entire experimental period, there was no significant decrease in the weight of mice in any of the groups. The findings suggest that the medications in every category did not have any significant effect on the wellbeing of the mice. No histomorphological changes, bleeding, or infiltration of inflammatory cells were observed in the heart, liver, spleen, lung, and kidney sections stained with H&E in all groups (Figure 7A). This suggests that the current dosage of these medications is biologically safe.
FIGURE 7. Investigation of the effects of each medication on mice with 4T1 tumors through pathological examination. (A) After administering various medications, the major organs (including the heart, liver, spleen, lung, and kidney) were subjected to H&E staining. Scale bar = 50 μm. (B) After administering various medications, tumors were subjected to H&E staining and TUNEL staining. Scale bar = 50 μm.
The tumor tissues were evaluated for histopathological features using H&E staining (Figure 7B). Compared with the NS group, the tumor cells in each treatment group exhibited cell atrophy and nuclear pyknosis. The CTT2P and CTT2P@B NPs groups demonstrated more pronounced nuclear pyknotic phenomena than the CPT, TPPOH2, and CTT2 groups. The apoptosis of tumor tissues was detected by terminal deoxynucleotidyl transferase-mediated dUTP-biotin nick end labeling (TUNEL) staining (Figure 7B), where blue represents living cells, and green represents apoptotic cells. The findings were consistent with the results of H&E staining. The absence of a notable disparity in cell apoptosis between the CPT group and the TPPOH2 group suggests that the anti-tumor effect of single chemotherapy and photodynamic therapy is suboptimal. On the other hand, the TPPOH2, CTT2, CTT2P, and CTT2P@B NPs groups showed a gradual increase in apoptosis, leading to a significant rise in the count of cells undergoing apoptosis. This further validates the excellent anti-tumor effect of CTT2P@B NPs.
In summary, we have successfully designed and synthesized a ROS-responsive prodrug, CTT2P, demonstrating high efficacy in inducing mitochondrial damage and subsequent apoptosis. Furthermore, we have formulated CTT2P@B NPs, encapsulating the prodrug CTT2P within BSA for efficient delivery and controlled release. The photosensitizer is covalently linked to CPT through a ROS-sensitive bond, TK. Additionally, the mitochondrial targeting group, triphenylphosphine, is incorporated to create the covalently linked prodrug, CTT2P. This covalent linkage enhances the specificity of chemotherapeutic drugs, minimizing side effects and synchronizing their in vivo distribution. The CTT2P@B NPs, formed by encapsulating the molecular prodrug CTT2P with BSA, facilitate the transport and release of the drug to tumor cells. Guided by the triphenylphosphine group, the molecular prodrug CTT2P selectively targets the mitochondrial site in tumor cells. Upon 660 nm laser irradiation, the photosensitizer component of the CTT2P prodrug generates a significant amount of ROS. These ROS induce mitochondrial damage and cleave the ROS-sensitive group TK in the prodrug. Furthermore, the accumulated CPT in the mitochondrial region exerts its chemotherapeutic action, generating additional ROS that disrupt the respiratory chain and promote mitochondrial apoptosis. Ultimately, through the synergistic effects of photodynamic therapy using a photosensitizer and chemotherapy with CPT, these drugs effectively enhance mitochondrial apoptosis, leading to cell apoptosis. The CTT2P@B molecular prodrug delivery system demonstrates low toxicity and a remarkable ability to induce mitochondrial apoptosis under laser irradiation. The findings of this research offer valuable information on the potential of organelle-specific methods to trigger apoptosis in cancerous cells.
The original contributions presented in the study are included in the article/Supplementary Material, further inquiries can be directed to the corresponding authors.
The animal study was approved by the Biomedical Research Ethics Committee of Binzhou Medical University (Ethics Section 2023 No. 205). The study was conducted in accordance with the local legislation and institutional requirements.
RW: Data curation, Formal Analysis, Investigation, Methodology, Writing–original draft, Writing–review and editing. HL: Investigation, Writing–original draft. LH: Investigation, Writing–original draft. BH: Investigation, Writing–original draft. YB: Investigation, Writing–original draft. HF: Investigation, Writing–original draft. CS: Investigation, Writing–original draft. RQ: Investigation, Writing–original draft. LM: Formal Analysis, Methodology, Writing–review and editing. JZ: Formal Analysis, Funding acquisition, Methodology, Writing–review and editing.
The author(s) declare financial support was received for the research, authorship, and/or publication of this article. We are grateful for financial support from the National Natural Science Foundation of China (No. 22001020), Foundation of Binzhou Medical University (No. 2019KYQD23) and Yantai Medical Antibacterial Material Innovation Service Platform.
The authors declare that the research was conducted in the absence of any commercial or financial relationships that could be construed as a potential conflict of interest.
All claims expressed in this article are solely those of the authors and do not necessarily represent those of their affiliated organizations, or those of the publisher, the editors and the reviewers. Any product that may be evaluated in this article, or claim that may be made by its manufacturer, is not guaranteed or endorsed by the publisher.
The Supplementary Material for this article can be found online at: https://www.frontiersin.org/articles/10.3389/fbioe.2024.1361966/full#supplementary-material
Agwa, M. M., Elmotasem, H., Elsayed, H., Abdelsattar, A. S., Omer, A. M., Gebreel, D. T., et al. (2023). Carbohydrate ligands-directed active tumor targeting of combinatorial chemotherapy/phototherapy-based nanomedicine: a review. Int. J. Biol. Macromol. 239, 124294. doi:10.1016/j.ijbiomac.2023.124294
Chen, Z., Huang, Q., Song, Y., Feng, X., Zeng, L., Liu, Z., et al. (2023). Cubosomes-assisted transdermal delivery of doxorubicin and indocyanine green for chemo-photothermal combination therapy of melanoma. Biomed. Pharmacother. 166, 115316. doi:10.1016/j.biopha.2023.115316
Chu, B., Qu, Y., He, X., Hao, Y., Yang, C., Yang, Y., et al. (2020). ROS-Responsive camptothecin prodrug nanoparticles for on-demand drug release and combination of chemotherapy and photodynamic therapy. Adv. Funct. Mat. 30 (52). doi:10.1002/adfm.202005918
Daum, S., Reshetnikov, M. S. V., Sisa, M., Dumych, T., Lootsik, M. D., Bilyy, R., et al. (2017). Lysosome-targeting amplifiers of reactive oxygen species as anticancer prodrugs. Angew. Chem. Int. Ed. 56 (49), 15545–15549. doi:10.1002/anie.201706585
Fu, A., Hou, Y., Yu, Z., Zhao, Z., and Liu, Z. (2019). Healthy mitochondria inhibit the metastatic melanoma in lungs. Int. J. Biol. Sci. 15 (12), 2707–2718. doi:10.7150/ijbs.38104
Hong, Z., Chyi, L., Prakash, S., Yun, H. C., Michelle, B., Annapurna, P., et al. (2000). 20-O-Acylcamptothecin derivatives: evidence for lactone stabilization. J. Org. Chem. 65 (15), 4601–4606. doi:10.1021/jo000221n
Jiang, M., Mu, J., Jacobson, O., Wang, Z., He, L., Zhang, F., et al. (2020). Reactive oxygen species activatable heterodimeric prodrug as tumor-selective nanotheranostics. ACS Nano 14 (12), 16875–16886. doi:10.1021/acsnano.0c05722
Khan, M. A., Zafaryab, M., Mehdi, S. H., Ahmad, I., and Rizvi, M. M. (2016). Characterization and anti-proliferative activity of curcumin loaded chitosan nanoparticles in cervical cancer. Int. J. Biol. Macromol. 93 (Pt A), 242–253. doi:10.1016/j.ijbiomac.2016.08.050
Lebepe, T. C., Parani, S., Ncapayi, V., Maluleke, R., Mbaz, G. I. M., Fanoro, O. T., et al. (2021). Graphene oxide-gold nanorods nanocomposite-porphyrin conjugate as promising tool for cancer phototherapy performance. Pharmaceuticals 14 (12), 1295. doi:10.3390/ph14121295
Li, N., Sun, Q., Yu, Z., Gao, X., Pan, W., Wan, X., et al. (2018). Nuclear-targeted photothermal therapy prevents cancer recurrence with near-infrared triggered copper sulfide nanoparticles. ACS Nano 12 (6), 5197–5206. doi:10.1021/acsnano.7b06870
Liu, L.-H., Qiu, W.-X., Li, B., Zhang, C., Sun, L.-F., Wan, S.-S., et al. (2016). A red light activatable multifunctional prodrug for image-guided photodynamic therapy and cascaded chemotherapy. Adv. Funct. Mat. 26 (34), 6257–6269. doi:10.1002/adfm.201602541
Liu, Z., Li, T., Han, F., Wang, Y., Gan, Y., Shi, J., et al. (2019). A cascade-reaction enabled synergistic cancer starvation/ROS-mediated/chemo-therapy with an enzyme modified Fe-based MOF. Biomater. Sci. 7 (9), 3683–3692. doi:10.1039/c9bm00641a
Luo, C., Sun, B., Wang, C., Zhang, X., Chen, Y., Chen, Q., et al. (2019). Self-facilitated ROS-responsive nanoassembly of heterotypic dimer for synergistic chemo-photodynamic therapy. J. Control. Release 302, 79–89. doi:10.1016/j.jconrel.2019.04.001
Luo, J., Miao, Z., Huang, X., Yang, Y., Liu, M., Shen, G., et al. (2023). Translational albumin nanocarrier caging photosensitizer for efficient cancer photodynamic therapy. Front. Bioeng. Biotechnol. 11, 1132591. doi:10.3389/fbioe.2023.1132591
Mallick, A., Kuman, M. M., Ghosh, A., Das, B. B., and Basu, S. (2018). Cerberus nanoparticles: cotargeting of mitochondrial DNA and mitochondrial topoisomerase I in breast cancer cells. ACS Appl. Nano Mat. 1 (5), 2195–2205. doi:10.1021/acsanm.8b00279
Mayr, J., Heffeter, P., Groza, D., Galvez, L., Koellensperger, G., Roller, A., et al. (2017). An albumin-based tumor-targeted oxaliplatin prodrug with distinctly improved anticancer activity in vivo. Chem. Sci. 8 (3), 2241–2250. doi:10.1039/c6sc03862j
Mehta, M. M., Weinberg, S. E., and Chandel, N. S. (2017). Mitochondrial control of immunity: beyond ATP. Nat. Rev. Immunol. 17 (10), 608–620. doi:10.1038/nri.2017.66
Nunnari, J., and Suomalainen, A. (2012). Mitochondria: in sickness and in health. Cell 148 (6), 1145–1159. doi:10.1016/j.cell.2012.02.035
O'connor, A. E., Gallagher, W. M., and Byrne, A. T. (2009). Porphyrin and nonporphyrin photosensitizers in oncology: preclinical and clinical advances in photodynamic therapy. Photochem Photobiol. 85 (5), 1053–1074. doi:10.1111/j.1751-1097.2009.00585.x
Pan, W., Cui, B., Wang, K., Shi, M., Lu, F., Li, N., et al. (2021). ATP-triggered mitochondrial cascade reactions for cancer therapy with nanoscale zeolitic imidazole framework-90. Theranostics 11 (16), 7869–7878. doi:10.7150/thno.59593
Porporato, P. E., Filigheddu, N., Pedro, J. M. B., Kroemer, G., and Galluzzi, L. (2018). Mitochondrial metabolism and cancer. Cell Res. 28 (3), 265–280. doi:10.1038/cr.2017.155
Sen, N., Das, B. B., Ganguly, A., Mukherjee, T., Tripathi, G., Bandyopadhyay, S., et al. (2004). Camptothecin induced mitochondrial dysfunction leading to programmed cell death in unicellular hemoflagellate Leishmania donovani. Cell Death Differ. 11 (8), 924–936. doi:10.1038/sj.cdd.4401435
Shi, S., Zhang, L., Zhu, M., Wan, G., Li, C., Zhang, J., et al. (2018). Reactive oxygen species-responsive nanoparticles based on PEGlated prodrug for targeted treatment of oral tongue squamous cell carcinoma by combining photodynamic therapy and chemotherapy. ACS Appl. Mat. Interfaces 10 (35), 29260–29272. doi:10.1021/acsami.8b08269
Sung, H., Ferlay, J., Siegel, R. L., Laversanne, M., Soerjomataram, I., Jemal, A., et al. (2021). Global cancer Statistics 2020: GLOBOCAN estimates of incidence and mortality worldwide for 36 cancers in 185 countries. CA Cancer J. Clin. 71 (3), 209–249. doi:10.3322/caac.21660
Wang, C., Yang, X., Qiu, H., Huang, K., Xu, Q., Zhou, B., et al. (2023). A co-delivery system based on chlorin e6-loaded ROS-sensitive polymeric prodrug with self-amplified drug release to enhance the efficacy of combination therapy for breast tumor cells. Front. Bioeng. Biotechnol. 11, 1168192. doi:10.3389/fbioe.2023.1168192
Xie, L., Shi, F., Li, Y., Li, W., Yu, X., Zhao, L., et al. (2020). Drp1-dependent remodeling of mitochondrial morphology triggered by EBV-LMP1 increases cisplatin resistance. Signal Transduct. Target Ther. 5 (1), 56. doi:10.1038/s41392-020-0151-9
Xu, F., Liu, M., Li, X., Xiong, Z., Cao, X., Shi, X., et al. (2018). Loading of indocyanine green within polydopamine-coated laponite nanodisks for targeted cancer photothermal and photodynamic therapy. Nanomaterials 8 (5), 347. doi:10.3390/nano8050347
Xu, Q., He, C., Xiao, C., and Chen, X. (2016). Reactive oxygen species (ROS) responsive polymers for biomedical applications. Macromol. Biosci. 16 (5), 635–646. doi:10.1002/mabi.201500440
Yang, F., Xu, M., Chen, X., and Luo, Y. (2023). Spotlight on porphyrins: classifications, mechanisms and medical applications. Biomed. Pharmacother. 164, 114933. doi:10.1016/j.biopha.2023.114933
Yi, G., Hong, S. H., Son, J., Yoo, J., Park, C., Choi, Y., et al. (2018). Recent advances in nanoparticle carriers for photodynamic therapy. Quant. Imaging. Med. Surg. 8 (4), 433–443. doi:10.21037/qims.2018.05.04
Yin, W., Ke, W., Chen, W., Xi, L., Zhou, Q., Mukerabigwi, J. F., et al. (2019). Integrated block copolymer prodrug nanoparticles for combination of tumor oxidative stress amplification and ROS-responsive drug release. Biomaterials 195, 63–74. doi:10.1016/j.biomaterials.2018.12.032
Yu, C., Kong, L., Tian, J., Zhang, Y., Jia, X., Dang, W., et al. (2023). Photoacoustic imaging-guided triple-responsive nanoparticles with tumor hypoxia relief for improving chemotherapy/photothermal/photodynamic synergistic therapy against breast cancer. Biomed. Pharmacother. 164, 114928. doi:10.1016/j.biopha.2023.114928
Yuan, Y., Liu, J., and Liu, B. (2014). Conjugated-polyelectrolyte-based polyprodrug: targeted and image-guided photodynamic and chemotherapy with on-demand drug release upon irradiation with a single light source. Angew. Chem. Int. Ed. 53 (28), 7163–7168. doi:10.1002/anie.201402189
Yuan, Y. Y., Mao, C. Q., Du, X. J., Du, J. Z., Wang, F., and Wang, J. (2012). Surface charge switchable nanoparticles based on zwitterionic polymer for enhanced drug delivery to tumor. Adv. Mat. 24 (40), 5476–5480. doi:10.1002/adma.201202296
Yue, C., Yang, Y., Zhang, C., Alfranca, G., Cheng, S., Ma, L., et al. (2016a). ROS-responsive mitochondria-targeting blended nanoparticles: chemo- and photodynamic synergistic therapy for lung cancer with on-demand drug release upon irradiation with a single light source. Theranostics 6 (13), 2352–2366. doi:10.7150/thno.15433
Yue, C., Zhang, C., Alfranca, G., Yang, Y., Jiang, X., Yang, Y., et al. (2016b). Near-infrared light triggered ROS-activated theranostic Platform based on Ce6-CPT-UCNPs for simultaneous fluorescence imaging and chemo-photodynamic combined therapy. Theranostics 6 (4), 456–469. doi:10.7150/thno.14101
Zhang, W., Hu, X., Shen, Q., and Xing, D. (2019). Mitochondria-specific drug release and reactive oxygen species burst induced by polyprodrug nanoreactors can enhance chemotherapy. Nat. Commun. 10 (1), 1704. doi:10.1038/s41467-019-09566-3
Zielonka, J., Joseph, J., Sikora, A., Hardy, M., Ouari, O., Vasquez-Vivar, J., et al. (2017). Mitochondria-targeted triphenylphosphonium-based compounds: syntheses, mechanisms of action, and therapeutic and diagnostic applications. Chem. Rev. 117 (15), 10043–10120. doi:10.1021/acs.chemrev.7b00042
Keywords: mitochondria-targeting, photodynamic therapy, bovine serum albumin, camptothecin, ROS-responsive
Citation: Wang R, Li H, Han L, Han B, Bao Y, Fan H, Sun C, Qian R, Ma L and Zhang J (2024) Combining photodynamic therapy and cascade chemotherapy for enhanced tumor cytotoxicity: the role of CTT2P@B nanoparticles. Front. Bioeng. Biotechnol. 12:1361966. doi: 10.3389/fbioe.2024.1361966
Received: 27 December 2023; Accepted: 24 January 2024;
Published: 12 February 2024.
Edited by:
Song Ma, Chinese Academy of Sciences (CAS), ChinaReviewed by:
Wei Shen, Anhui University of Chinese Medicine, ChinaCopyright © 2024 Wang, Li, Han, Han, Bao, Fan, Sun, Qian, Ma and Zhang. This is an open-access article distributed under the terms of the Creative Commons Attribution License (CC BY). The use, distribution or reproduction in other forums is permitted, provided the original author(s) and the copyright owner(s) are credited and that the original publication in this journal is cited, in accordance with accepted academic practice. No use, distribution or reproduction is permitted which does not comply with these terms.
*Correspondence: Liying Ma, bWFsaXlpbmdiekAxNjMuY29t; Jiajing Zhang, amlhamluZ196QGJ6bWMuZWR1LmNu
Disclaimer: All claims expressed in this article are solely those of the authors and do not necessarily represent those of their affiliated organizations, or those of the publisher, the editors and the reviewers. Any product that may be evaluated in this article or claim that may be made by its manufacturer is not guaranteed or endorsed by the publisher.
Research integrity at Frontiers
Learn more about the work of our research integrity team to safeguard the quality of each article we publish.