- 1Center for Nanoscience and Nanotechnology, Institute for Convergence Science and Technology, Sharif University of Technology, Tehran, Iran
- 2Department of Pharmacology, School of Medicine, Shahid Beheshti University of Medical Sciences, Tehran, Iran
- 3Department of Nanobiotechnology, Faculty of Biological Sciences, Tarbiat Modares University, Tehran, Iran
- 4Department of Materials Science and Engineering, Sharif University of Technology, Tehran, Iran
- 5Center for Bioscience and Technology, Institute for Convergence Science and Technology, Sharif University of Technology, Tehran, Iran
In recent years, the amnion (AM) has emerged as a versatile tool for stimulating tissue regeneration and has been of immense interest for clinical applications. AM is an abundant and cost-effective tissue source that does not face strict ethical issues for biomedical applications. The outstanding biological attributes of AM, including side-dependent angiogenesis, low immunogenicity, anti-inflammatory, anti-fibrotic, and antibacterial properties facilitate its usage for tissue engineering and regenerative medicine. However, the clinical usage of thin AM sheets is accompanied by some limitations, such as handling without folding or tearing and the necessity for sutures to keep the material over the wound, which requires additional considerations. Therefore, processing the decellularized AM (dAM) tissue into a temperature-sensitive hydrogel has expanded its processability and applicability as an injectable hydrogel for minimally invasive therapies and a source of bioink for the fabrication of biomimetic tissue constructs by recapitulating desired biochemical cues or pre-defined architectural design. This article reviews the multi-functionality of dAM hydrogels for various biomedical applications, including skin repair, heart treatment, cartilage regeneration, endometrium regeneration, vascular graft, dental pulp regeneration, and cell culture/carrier platform. Not only recent and cutting-edge research is reviewed but also available commercial products are introduced and their main features and shortcomings are elaborated. Besides the great potential of AM-derived hydrogels for regenerative therapy, intensive interdisciplinary studies are still required to modify their mechanical and biological properties in order to broaden their therapeutic benefits and biomedical applications. Employing additive manufacturing techniques (e.g., bioprinting), nanotechnology approaches (e.g., inclusion of various bioactive nanoparticles), and biochemical alterations (e.g., modification of dAM matrix with photo-sensitive molecules) are of particular interest. This review article aims to discuss the current function of dAM hydrogels for the repair of target tissues and identifies innovative methods for broadening their potential applications for nanomedicine and healthcare.
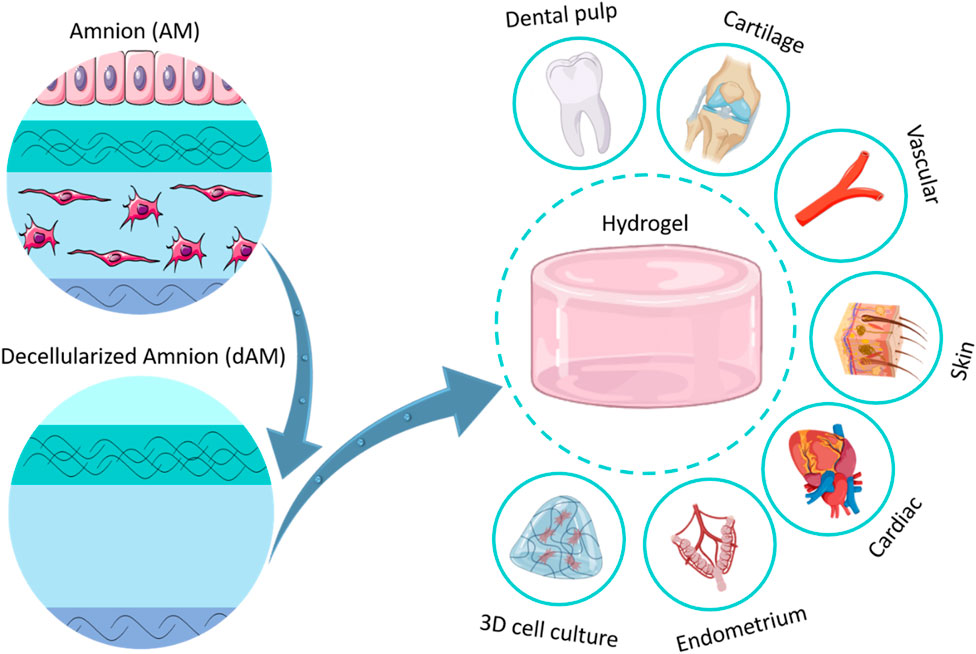
GRAPHICAL ABSTRACT | The Figure was partly generated using Servier Medical Art, provided by Servier, licensed under a Creative Commons Attribution 3.0 unported license.
1 Introduction
Damage to tissues can be caused due to several reasons, such as sudden or unexpected accidents, genetic disorders, congenital anomalies, and trauma (Krafts, 2010). In simple cases, the body can recover tissue through inflammation, cell proliferation, and tissue regeneration (Poss, 2010). However, in serious injuries, medical interventions such as transplanting tissue substitutes (i.e., autografts, allografts, or xenografts) or stimulating tissue regeneration using tissue-engineered constructs (TECs) are required (Gaharwar et al., 2020). Among various TECs or bio-derived scaffolds, the amniotic membrane also named as amnion (AM) has gained great attention for tissue engineering (TE) and regenerative medicine (RM), thanks to its exquisite biological characteristics, including excellent biocompatibility, anti-bacterial, anti-inflammatory, anti-fibrotic, immunomodulatory and angiogenic properties (Munoz-Torres et al., 2023). AM meets all basic requirements of TE, including a rich source of stem cells, growth factors, and bioactive molecules as well as extracellular matrix (ECM) components that further highlight its eligibility for therapeutic purposes (Jahanafrooz et al., 2023).
AM has been discovered as a potential candidate for skin regeneration and reconstruction surgeries since the early 20th century (John, 2003). The first report of using AM as a skin replacement was done by Davis in 1910 (Davis, 1910). Later in 1913, AM was used by Stern et al. for treating burn and ulcerated wounds (Stern, 1913). Since then, AM has shown promising results in the repair of various tissues including, the heart, ocular surface, vascular, cartilage, uterus, etc (Cornwell et al., 2009; Horn et al., 2019; Hossain et al., 2020; Lacorzana, 2020). Despite the advantageous clinical results obtained by grafting AM, there are some challenges for surgeons to handle thin AM tissue without tearing or folding, which limits the incorporation of fresh AM into routine clinical applications to some extent (Murphy et al., 2017; Dadkhah Tehrani et al., 2021). Besides, the dense structure of the AM tissue is known to limit the penetration of cells to the wounded site (Nasiry et al., 2021; Nasiry et al., 2022; Khalatbary et al., 2023; Nasiry et al., 2023). One of the methods to solve these issues was to use its dehydrated form, which is easier to handle and can be stored at room temperature with a shelf life of about 5 years (Fetterolf and Snyder, 2012). Although the dehydrated AM tissue has shown promising outcomes for treating wounds, it lacks efficacy for the treatment of large or irregularly shaped injuries (Murphy et al., 2017). Therefore, processing of the AM tissue into a hydrogel can afford easier handling by gelation inside any nonuniform defect injuries. To this end, some researchers have developed a gel formula based on the incorporation of AM powder or AM extracts into a hydrogel solution (Murphy et al., 2017; Rahman et al., 2019; Islam et al., 2023). In other studies, the development of an injectable hydrogel through the enzymatic digestion of decellularized amnion (dAM) powder in a mildly acidic solution has been performed (Ryzhuk et al., 2018). This category of hydrogels forms physical crosslinking by incubation of the pH-neutralized dAM solution at the physiologic temperature (Li et al., 2022).
The applications of AM tissue and AM-derived stem cells in different fields of TE and RM are well-covered in recent literature (Jafari et al., 2021; Elkhenany et al., 2022; Hu et al., 2023). This review aims to comprehensively explain the advanced multifaceted therapeutic functions of hydrogels derived from AM or dAM for tissue regeneration. The structural features and components of AM are introduced and various biological functions of the tissue are presented and discussed. The commercial products based on AM, including their features and shortcomings, are also demonstrated. Besides, the most relevant fabrication processes of the AM/dAM hydrogels and their applications in the engineering and regeneration of heart, skin, cartilage, vascular, endometrium and fetal membrane, and dental pulp are critically reviewed. Finally, recent insights into the modification of AM/dAM-derived hydrogels, such as designing hybrid hydrogels by incorporating supporting polymeric constituents, rapid photocrosslinking process through grafting light-sensitive functional groups, and nanoparticles, are concluded.
2 Structural and biochemical features of amnion
The human placenta is considered a biowaste for hospitals and is discarded after the baby is born (Mamede et al., 2012). Hence, the placenta is an available and cost-effective source of tissue that does not require extreme ethical considerations for its clinical usage (Haghshenas et al., 2022). AM is the innermost fetal membrane of the placenta that protects the fetus during pregnancy (Figure 1A). The outer layer is called the chorion plate, which contacts with the mother’s cells (Salah et al., 2020). AM is a thin (0.02–0.5 mm) and translucent tissue that does not possess any muscles, nerves, or vessels, and its nutrition occurs due to diffusion (Toda et al., 2007). As depicted in Figure 1B, the human AM is composed of three main layers, including the epithelium, basement membrane, and stromal (Niknejad et al., 2008). The epithelial cells secrete a wide range of growth factors (GF) and cytokines, including epidermal GF (EGF), vascular endothelial GF (VEGF), keratinocyte GF (KGF), basic fibroblast GF (bFGF), alpha- and beta-transforming GF (TGF-α, TGF-β), interleukin-8 (IL-8), angiogenin, serine protease inhibitor (serpin) E1, insulin-like GF (IGF), and their binding proteins (IGFBP) (Favaron et al., 2015). These cells are firmly attached to the basement membrane that is a supply of sulfated proteoglycans (e.g., heparan sulfate) and as a permeable barrier allows the transport of several macromolecules, including α-actinin, spectrin, vimentin, laminin, desmoplakin, cytokeratins, etc. (Ohno et al., 1983; Liu et al., 2019; Shariatzadeh et al., 2021). The basement membrane of AM also contains collagen (type III, IV, and V) and various non-collagenous glycoproteins, such as elastin, laminin, nidogen, fibronectin, and vitronectin as well as glycosaminoglycans like hyaluronic acid (Arki et al., 2023; Fitriani et al., 2023). The stromal layer is composed of three sublayers, including 1) compact layer, 2) fibroblast layer, and 3) intermediate layer (Dadkhah Tehrani et al., 2021). The compact layer which is located beneath the basement membrane is a cell-free connective tissue that mainly consists of collagen (type I, III, V, and VI) (Sadler, 2022). The next layer, which is the thickest layer of AM, contains mesenchymal fibroblast-like cells and collagen-rich ECM which significantly affects the tensile strength of the AM tissue (Mamede et al., 2012). The outermost layer is a cell-free nonfibrillar matrix containing type III collagen, proteoglycans, glycoproteins, and hydrated glycoproteins that separate the AM from the underlying chorion (Toda et al., 2007). This layer is called the “spongy layer” because the proteoglycans and glycoproteins components inside this layer produce a spongy appearance (Fénelon et al., 2021). It is noteworthy to mention that collagen I and II and elastin regulate the tensile strength of the AM, while collagen III is responsible for the elasticity (Mamede et al., 2012). Therefore, it can be concluded that AM is a rich source of various proteins, GF, and GAGs, including collagen (types I, III, IV, V, and VI), fibronectin, elastin, nidogen, and hyaluronic acid that support the proliferation and differentiation of cells, and encourage re-epithelialization (Rana et al., 2020).
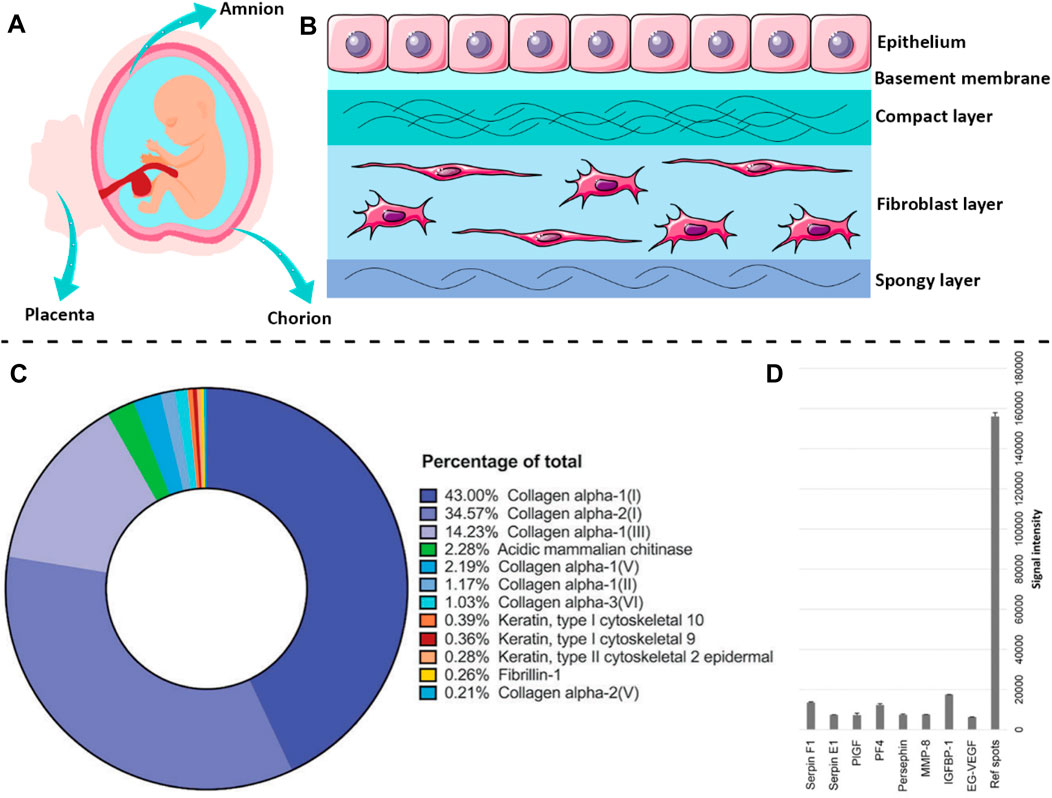
FIGURE 1. The structural architecture of AM tissue and biochemical components within the dAM matrix. A scheme of human (A) placenta and (B) AM membrane. (C) The main proteins of the dAM matrix evaluated by mass spectroscopy [Reprinted from Comperat et al. (2023), Copyright (2023), with permission from Wiley-VCH GmbH]. (D) The most frequent cytokines in dAM hydrogel assessed by the angiogenesis array [Reprinted from Ryzhuk et al. (2018), Copyright (2023), with permission from Elsevier]. Panel 1B was partly generated using Servier Medical Art, provided by Servier, licensed under a Creative Commons Attribution 3.0 unported license.
Decellularization of native tissues and organs is a prerequisite step for their safe implementation for TE applications, since the resident cells may cause intense host immunologic reactions after transplantation and transplant rejection (Bhattacharjee et al., 2020). Accordingly, various mechanical, chemical, and enzymatic techniques and a combination of these methods have been adopted for the successful removal of cells from tissues (Arrizabalaga and Nollert, 2018). Although the decellularization process is quite a promising tool for diminishing cellular components of tissues and organs, it may also cause the loss of some bioactive ECM components (Kim et al., 2020). Hence, it is important to choose a decellularization agent in a way that provides sufficient cell removal without seriously damaging the ECM structure and existing biochemical cues (Kim et al., 2017). Regardless of losing some of the ECM components during the decellularization process, a sufficient amount of bioactive components still remain within the dAM matrix to provide reliable functionality for TE applications (Wassmer and Berishvili, 2020). As reported by Comperat et al. (2023), the dAM matrix is mainly composed of structural proteins such as collagen, and fibrillin-1, and cytoskeletal-associated proteins (e.g., keratin-type I) (Figure 1C). The results of the angiogenesis assay also suggest that the dAM matrix is a valuable source of various proteins and GF, including placenta (PlGF), platelet factor 4 (PF4), insulin-like binding protein 1 (IGFBP-1), and endocrine gland-derived vascular endothelial (EG-VEGF) (Figure 1D).
3 Bio-functionality of amnion
Although AM is an avascular tissue, it plays an important role as a tissue for the production of several bioactive molecules, including growth factors, cytokines, and vasoactive peptides (Cunningham et al., 2014). As schematically shown in Figure 2A, AM is a biocompatible tissue that provides outstanding biological characteristics, such as low immunogenicity, anti-inflammatory, anti-fibrotic, and anti-bacterial properties along with side-dependent angiogenesis (Elkhenany et al., 2022). In several studies, for example, Favaron et al. (2015), Babajani et al. (2022a), Babajani et al. (2022b), Biniazan et al. (2022), Jafari et al. (2023), AM-derived stem cells with angiogenic, immunosuppressive, and anti-tumoral properties have been found of interest for tissue regeneration. The various types of ECM components are mostly secreted from resident cells of AM to form different sublayers of AM as described thoroughly in the previous section. In the following, the regenerative effects induced by bioactive constituents of the amnion matrix, e.g., proteins, growth factors, and cytokines, are presented and discussed. It is noteworthy that the biological properties of AM tissue, as similar to other native tissues, may be more pronounced than the decellularized AM (dAM) because the decellularization process is potentially susceptible to the loss of some part of the bioactive and functional constituents of native tissues (Elkhenany et al., 2022). However, exploiting an optimized decellularization process may guarantee the preservation of a considerable portion of these biochemical cues within dECM biomaterials (Saldin et al., 2017).
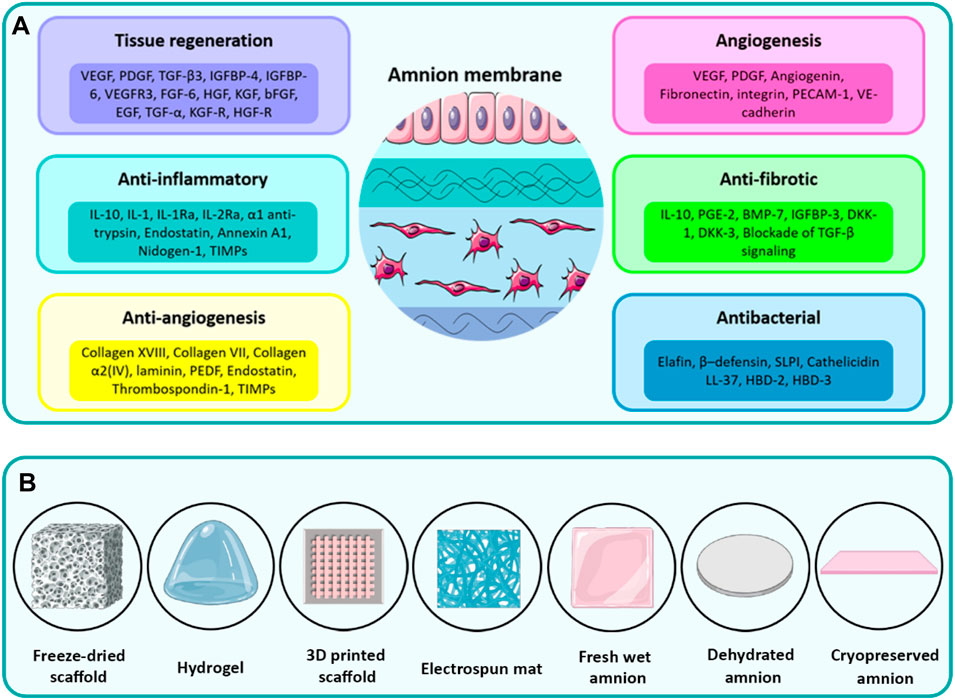
FIGURE 2. (A) The diverse therapeutic properties of the amnion induced by different inherent cytokines, growth factors, and ECM components. (B) Various types of processing methods to fabricate biomedical scaffolds from AM. Panel 2A was partly generated using Servier Medical Art, provided by Servier, licensed under a Creative Commons Attribution 3.0 unported license.
3.1 Anti-inflammatory
So far, different mechanisms have been proposed for explaining the anti-inflammatory effect of AM by different generations of researchers in this field. The anti-inflammatory of AM is ascribed to its inhibiting effect on the infiltration of inflammatory cells to an injured site (Shimmura et al., 2001). The soluble factors secreted from epithelial cells (e.g., interleukin-1 receptor antagonist (IL-1Ra), IL-2Ra, IL-10, and endostatin) are also effective (Hao et al., 2000; Li et al., 2005). These factors hinder the immune cells and reduce angiogenesis and tumor growth by restricting the proliferation of endothelial cells. Particularly, IL-10 suppresses the activity of pro-inflammatory cytokines, such as IL-1, IL-6, IL-8, interferon-γ (IFN-γ), and tumor necrosis factor-alpha (TNF-α) (Silini et al., 2017; Koelink et al., 2020). The anti-inflammatory property of AM is further speculated to be due to the suppression effect of its stromal matrix on the expression of IL-1α and IL-1β pro-inflammatory cytokines (Niknejad et al., 2008). Amnion also enables the expression of tissue inhibitors of metalloproteinase (TIMPs)-1, 2, 3, and 4, which can suppress the ECM digestive function of matrix metalloproteinases (MMPs) produced by macrophages and polymorphonuclear cells (Kim et al., 2000). The ability of AM to combat inflammation can also be explained by its protease inhibitors, such as α1 anti-trypsin and inter-α-trypsin (Elkhenany et al., 2022). Antimicrobial peptides (AMPs) within AM, such as human beta-defensins (HBDs), cathelicidin, and histones are capable of attenuating inflammation induced by lipopolysaccharide (LPS) (Guaní-Guerra et al., 2010). Furthermore, the protease and elastase inhibitory effects of elafin and secretory leucocyte proteinase inhibitor (SLPI) control the inflammatory responses in the surfaces of tissues suspected of contamination (Zare-Bidaki et al., 2017).
3.2 Antibacterial and anti-viral activity
AM exhibits promising results in controlling the infection of wounds because it not only acts as an adherent shield over an injured tissue to inhibit the infiltration of bacteria but also regulates the expression of molecules with antibacterial or antiviral properties (King et al., 2007). Ni et al. (1997) have stated that AM offers anti-viral properties due to the presence of cystatin E in its matrix which is an analog for cysteine proteinase inhibitor. The collagen fibers within the AM matrix can also contribute to reduced bacterial accumulation and inhibited hematoma formation, owing to their hemostatic property (Baradaran Rafii et al., 2007). The epithelial cells of AM can secrete AMPs, such as β-defensin 1-3 (HBD 1–3), LL37, histone H2B, and elafin which are helpful in the management of wound infection (Kim et al., 2002; Tehrani et al., 2013). The elastase inhibitors expressed by AM, including elafin and SLPI proteins that contain Whey acidic peptide (WAP) motifs, are also responsible for anti-inflammatory and antibacterial properties (Mohan et al., 2017; Tehrani et al., 2017; Lohajaroensub et al., 2022). Moreover, the AM contains various AMPs, including human neutrophil peptides 1-3, calprotectin (MRP8/14), lysozyme, and ubiquitin that exhibit antimicrobial function (Kim et al., 2007). The AMPs may exhibit antimicrobial effects through several pathways, but the most frequent mechanism of action involves the electrostatic interactions between positively charged AMPs and negative bacterial membranes (Zasloff, 2002). The quantitative mass spectroscopy of amnion/chorion extract has detected several ribonucleases [e.g., RNaseT2, RNaseK6, RNase7, RNase5 (angiogenin), RNase H2 subunit C, RNase pancreatic] and hydrolase components with antibacterial potential that destroy the biofilms (Yadav et al., 2017). The lysozyme secreted by epithelial cells of AM is capable of hydrolyzing the peptidoglycan backbone of bacterial cells, damaging their cell membrane, followed by lysis of bacteria (Yadav et al., 2017).
In a study, the antimicrobial activity of cryo-preserved AM (containing viable cells) was compared to the air-dried or freeze-thawed devitalized AM (with non-viable cells) counterpart (Mao et al., 2017). The results showed that the antimicrobial property of AM is governed by soluble antimicrobial factors secreted from endogenous viable cells, whereas non-viable cells in dehydrated devitalized AM do not participate in the synthesis of antimicrobial proteins. Recently, Tehrani et al. (2017) have reported that the exposure of AM to IL-1β may improve the secretion of antimicrobial peptides, including elafin, cathelicidin LL-37, HBD-2, and HBD-3. In addition to protein-based antimicrobial compounds existing in the AM, lactoferrin and hyaluronic acid (HA) components may also participate in anti-inflammatory and antibacterial responses (Zare-Bidaki et al., 2017).
3.3 Immunomodularity
The clinical application of AM has shown negligible immune response without acute rejection (Niknejad et al., 2008). This finding may be attributed to low expression of human leucocyte antigens (HLA) class I and no expression of HLA class II by epithelial cells (Hori et al., 2006). Herein, no expression of immunogenic markers, including CD86, CD40, CD80, HLA-A, HLA-B, HLA-D, and HLA-DR, are noticed which determines low immunogenicity (Magatti et al., 2018). The presence of immune-regulatory factors, e.g., HLA-G, IL-10, TGF-β, HGF, prostaglandin E2 (PGE2), and indoleamine 2,3-dioxygenase (IDO) are also responsible for its immune privilege through the suppressive effect on the proliferation of T cells and the CD8+ activity (Parolini et al., 2008; Silini et al., 2017). The HLA-G is an immunosuppressive molecule that interacts with the ILT4 receptor on monocytes and dendritic cells or the ILT2 receptor on various immune cells, such as natural killer cells, B-cells, monocytes, and dendritic cells (Wassmer and Berishvili, 2020). The interaction of HLA-G with different types of immune cells can in turn inhibit their proliferation, and immunoglobulin secretion, or attenuate their innate cytotoxicity (Kapasi et al., 2000; Selmani et al., 2008).
The cells existent in AM are highly capable of blocking immune responses by influencing immune cells’ function and secretion (Wassmer and Berishvili, 2020). For example, the conditioned medium extracted from AM has shown apoptotic effects on in vitro cultured neutrophil cells, whereas the direct in vivo administration of epithelial cells of AM exhibited a significant influence on decreasing the infiltration of neutrophil cells (Zhou et al., 2003; Tan et al., 2014). The transplantation of AM cells has shown an inhibiting effect on the infiltration of immune cells (e.g., T cells, monocytes, and macrophages) to the injured site, thereby reducing intense inflammatory responses (Liu et al., 2012). The immunosuppressive action of AM cells or conditioned media derived from AM is related to the bioactive modulators secreted from these cells and their ability to induce M2 macrophages over M1 macrophages (Magatti et al., 2017; Silini et al., 2017). The polarized forms of macrophages (i.e., M1 and M2) are known for their opposite actions in the tissue regeneration process, where the M1 macrophages boost the inflammatory phase of healing and M2 macrophages contribute to the regeneration process while showing some anti-inflammatory effects (Barboni et al., 2012). Magatti et al. have reported that conditioned media derived from in vitro culture of MSC cells of AM can impede the proliferation and differentiation of B lymphocytes through the action of prostanoids such as PGE2 (Magatti et al., 2020). The AM cells can also regulate the cytokine and chemokine secretion of immune dendritic cells to increase anti-inflammatory cytokines such as IL-10 or decrease the release of proinflammatory mediators, including IL-8, IL-12p70, TNF-α, and MIP-1α (Magatti et al., 2015). The immunosuppressive effect may also be linked to the expression of the Fas ligand by epithelial and mesenchymal cells (Chopra and Thomas, 2013).
3.4 Angiogenesis duality
Studies have demonstrated that AM is able to suppress neovascularization due to its anti-angiogenesis property (Shao et al., 2004). This anti-angiogenesis can be explained by the physical barrier effect that inhibits the permeation of pro-angiogenesis factors (Kobayashi et al., 2002). It can also happen as a result of ECM proteins, including collagen α2 (IV), laminin-1, laminin-5, fibronectin, collagen type VII, integrin 4, and integrin 6, which are known for their inhibition effect on corneal neovascularization (Fukuda et al., 1999). Moreover, the amniotic cells can secrete cytokines with an anti-angiogenesis effect, such as endostatin and thrombospondin-1 (Hossain et al., 2019; Bakhshandeh et al., 2021; de la Torre et al., 2021). Thrombospondin-1 is a potential anti-angiogenic peptide produced by mesenchymal cells (Ju et al., 2022), whereas endostatin is an anti-angiogenesis factor that prevents the growth of endothelial cells (Ghazani et al., 2022). Furthermore, some proteins, including collagen XVIII, IL-10, IL-1Ra, TIMP-1, TIMP-2, TIMP-3, and TIMP-4 exhibit anti-angiogenic activity (Hao et al., 2000). On the other hand, epithelial cells may play a role in mediating anti-angiogenic characteristics through the production of IL-1Ra, TIMP-3, and TIMP-4 (Parolini et al., 2009). For instance, the pigment epithelium-derived factor (PEDF) expressed by AM has been shown to act as a potent chemical inhibitor of angiogenesis (Shao et al., 2004).
The pro-angiogenic activity of AM has also been reported in the literature (Niknejad et al., 2013; Niknejad and Yazdanpanah, 2014; Yazdanpanah et al., 2015; Abbasi-Kangevari et al., 2019). Niknejad et al. (2013) have shown that the angiogenesis effect of AM is a side-dependent phenomenon. They have demonstrated that the vessel formation in a dorsal skinfold chamber rat model is enhanced when the AM is positioned epithelial side, whereas the vascularization is suppressed when the material is positioned stromal side. The pro-angiogenic effect of AM may originate from PDGF, VEGF, and angiogenin secreted by mesenchymal cells (Farhadihosseinabadi et al., 2018). On the other hand, fibronectin is able to promote angiogenesis by activating the ERK signaling pathway via interacting with PDGF, EGF, and bFGF (Hu et al., 2023). Tsai et al. (2007) have concluded that the upregulating the expression of integrin, platelet-endothelial cell adhesion molecule-1 (PECAM-1), and VE-cadherin adhesion molecules in the cultured endothelial cells make them a potential candidate as vascular grafts.
3.5 Anti-fibrosis
Fibrosis is a well-recognized hypertrophic pathological characteristic observed during the wound healing process that occurs due to excess secretion of fibroblast cells activated by TGF-β1 (Garrido et al., 2018). TGF-β1 stimulates fibrogenesis by encouraging the synthesis of ECM and the deposition of collagen by resident cells (Gonçalves et al., 2014). The anti-fibrotic characteristic of AM is attributed to its inhibition effect on the expression of TGF-β1 receptors in fibroblast cells (Arki et al., 2023). For example, the AM patch has been used in the treatment of liver fibrosis for reducing collagen deposition by down-regulating the pro-fibrotic factors, such as TGF-β1 and IL-1 (Sant’Anna et al., 2016). Studies also have shown that AM can downregulate the expression of apelin ligands in cirrhosis liver (Garrido et al., 2018). The paracrine signaling activated by the release of soluble factors from resident cells of AM tissue is effective in reducing the activity of pro-fibrotic and pro-inflammatory factors, such as TGF-β, IL-6, TNF-α, and PDGF accompanied by increasing the expression of anti-inflammatory cytokines, including IL-10 (Sant’Anna et al., 2016; Silini et al., 2015; Manuelpillai et al., 2010). A study by Mao et al. (2019) showed that condition media prepared from viable lyophilized AM (VLAM) tissue provides antifibrotic properties due to the presence of anti-fibrotic cytokines, such as HGF and IL-1β. The VLAM was also effective in reducing the expression of collagen I and α-SMA as pro-fibrotic factors.
The epithelial cells of AM can further participate in alleviating abnormal fibril arrangement by secreting anti-fibrotic factors, such as bone morphogenetic protein-7 (BMP-7), PGE2, and IL-10 (Andrewartha and Yeoh, 2019). Similarly, mesenchymal cells exhibit an anti-fibrotic effect by blocking the Wnt/β-catenin signaling via secretion of IGFBP-3, Dickkopf-1 (DKK-1), and Dickkopf-3 (DKK-3) (Liu et al., 2022). In addition, the conditioned medium released from mesenchymal cells of AM presents an antifibrotic effect by decreasing the levels of α-SMA and collagen I as well as increasing the MMP-2, MMP-9, and MMP-13 levels, which help degrade excess ECM (Fu et al., 2018).
3.6 Tissue regeneration and re-epithelialization
The basement membrane of AM is an appropriate matrix for encouraging the migration, adhesion, and differentiation of epithelial cells, which ultimately accelerates the re-epithelization of the wound site (Nazanin et al., 2022). The expression of various growth factors, such as TGF-α, EGF, KGF, bFGF, HGF, and GF receptors, including KGF-R and HGF-R, play major roles in wound healing and tissue regeneration (Koizumi et al., 2000). Various types of collagenous and non-collagenous proteins also significantly contribute to tissue repair by supporting cell activities (Murri et al., 2018). Altogether, these mentioned biochemical cues accelerate tissue regeneration by encouraging cell proliferation and angiogenesis (Chen et al., 2021).
4 Commercial products
Due to the outstanding therapeutic function of AM for treating wounds and regeneration of tissues, there are several international companies producing health products based on AM. The immune privilege of AM-derived stem cells, including epithelial and mesenchymal cells, through a low expression of class I antigens (HLA-A, B, C) and no expression of class II antigens (HLA-DR) have made it possible to commercialize AM-related products without decellularization (Srinivasan et al., 2020). Therefore, AM-based products are mostly dehydrated or cryo-preserved forms of amnion and seldom are decellularized (e.g., Biovance). To further enhance the mechanical stability and ease of handling, some of the AM-related products are composed of both layers of amnion and chorion together, including AmniEffect, EpiFix, AmniFix, AmniBurn, and AmnioExcel Plus. The AM-based commercial products and their structural features along with specific medical applications are summarized in Table 1. As mentioned in Table 1, some of these products have obtained health approval from the Food and Drug Administration (FDA) and the American Association of Tissue Banks (AATB). The other portion of these products are under clinical investigation to get required health confirmations or even in the very early stages of development. Nevertheless, the approved AM products are considered aseptic healthcare products that do not show adverse effects on the healing process of patients. However, when using these products, it is of great importance to meticulously follow the usage protocol of the manufacturer and pay attention to the storage notes. Moreover, the sterility of the product should not be compromised. Furthermore, the AM product is supposed to be placed from the epithelial side on the wound bed to encourage angiogenesis (Munoz-Torres et al., 2023). Among all AM products, there is only one product in gel form (i.e., AmnioBarrier) which is under development to be tested for clinical trials and is not on the market yet. However, since the regulations for assessing AM tissue-derived products are standardized and well-established by regulatory organizations in healthcare, the production of processed tissue products, such as AM gel hopefully seems to be possible in the near future.
5 Biomedical applications of AM-derived hydrogels
The therapeutic functionality has motivated researchers to process AM by various methods to prepare TE scaffolds (Fenelon et al., 2023). These methods involve using fresh AM sheets, dehydrated AM sheets, cryopreserved AM sheets, freeze-dried microporous scaffold, electrospun mat, 3D printed constructs, and pepsin-solubilized dAM-derived hydrogels (Figure 2B). The application of AM tissue or other types of AM-derived scaffolds has thoroughly been reviewed in recent studies (Dadkhah Tehrani et al., 2021). The main focus of the current work is exploring the development of AM-derived hydrogel and its widespread applications in TE and RM.
The preparation of hydrogels from the decellularized extracellular matrix (dECM) consists of 1) enzymatic digestion into monomeric protein components and 2) the temperature-induced gelation of pH-neutralized pre-gel solution (Saldin et al., 2017). In the first step, the cleavage of the terminal telopeptide region of collagen within dECM material by the pepsin enzyme enables digesting in dilute acidic solutions (Li et al., 2022). The removal of terminal peptides of collagen also attenuates the immunogenicity of the dECM-derived hydrogels (Lynn et al., 2004). In the second step, the reformation of intermolecular bonds within monomeric protein units forms a three-dimensional (3D) gel network (Johnson et al., 2011). The loss of water during incubation at physiologic temperature increases the entropy within the dECM pre-gel solution and subsequently forms aggregates of collagenous subunits in a so-called “self-assembly” process (Saldin et al., 2017).
The processing steps of AM tissue into a temperature-responsive injectable hydrogel include: 1) dissection of AM from underlying chorion tissue, 2) washing blood clots, 3) decellularization of AM tissue, 4) lyophilization and pulverization, 5) enzymatic digestion with pepsin, 6) incubation of pH-neutralized dAM solution at physiologic temperature (Kafili et al., 2022). Figure 3 shows these steps and the potential applications of the derived hydrogels. Processing AM into a hydrogel form has some advantages over the tissue form. The first benefit of AM hydrogel over AM tissue is related to the homogeneous distribution of biochemical cues in the hydrogel structure. Although the AM is considered a rich source of various proteins and bioactive growth factors, the distribution of these components varies in its different sublayers (Dadkhah Tehrani et al., 2021). This inhomogeneous distribution of the biomolecules may interfere with the aimed therapeutic characteristic (Jahanafrooz et al., 2023). For instance, the stromal side of AM exhibits better outcomes for wound healing applications as a result of EGF and TGF-β content (Gicquel et al., 2009), whilst the epithelial side shows promising results for ophthalmology surgeries due to its low content of TGF-β (Walkden, 2020). This medical choice is based on the fact that a high TGF-β content offers a stimulating effect on the over-deposition of collagen that may lead to hypertrophic scar formation and fibrosis, which in turn causes the loss of corneal transparency (Torricelli et al., 2016). Conversely, dAM hydrogels provide a more homogenous distribution of bioactive factors within 3D networks. On the other hand, despite the low immunogenicity of AM tissue, there is always a risk of inflammatory responses arising after transplantation (Gholipourmalekabadi et al., 2020). This issue can preferably be addressed by using decellularized AM tissue for the fabrication of injectable and temperature-sensitive hydrogels (Ryzhuk et al., 2018). Some of the other profits of dAM-derived hydrogel over AM or dAM tissues may include easier handling, better cell permeability, and control over tailoring mechanical properties by changing synthesis parameters, such as concentration, digestion time, etc. (Kafili et al., 2023a; Jahanafrooz et al., 2023).
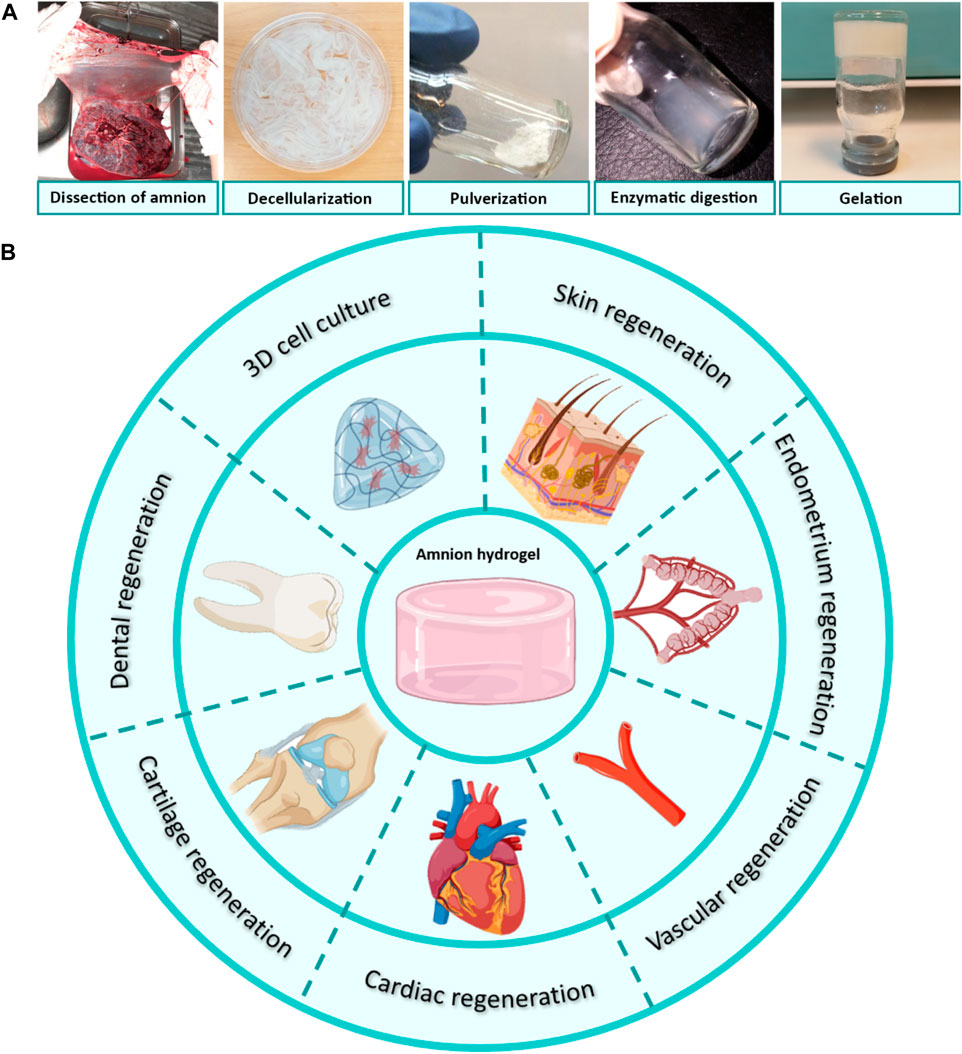
FIGURE 3. (A) Processing of dAM tissue into temperature-responsive hydrogels through dissection from placenta tissue, decellularization, lyophilization, pulverization, enzymatic digestion by pepsin in an acidic solution, and gel formation after incubation of pH-neutralized dAM solution at the physiologic temperature [Reprinted from Kafili et al. (2022), Copyright (2023), with permission from Elsevier]. (B) Tissue engineering applications of AM/dAM hydrogels. Panel 3B was partly generated using Servier Medical Art, provided by Servier, licensed under a Creative Commons Attribution 3.0 unported license.
The therapeutic properties of dAM hydrogels are multifaceted. So far, dAM hydrogels have been employed for the regeneration of the heart, skin, dental pulp, cartilage, vascular, fetal membrane, endometrium, and to a lesser extent for cell carrier vehicles (Figure 3B). It is noteworthy to mention that these studies are carried out as in vitro and in vivo setups. Considering the potential of AM tissue in wider biomedical applications, such as oral mucosa regeneration, bone repair, corneal regeneration, urinary bladder reconstruction, vaginoplasty, etc., its hydrogel has not yet been fully exploited. We believe that dAM hydrogels can be used in broader fields of medical therapies in the future, as it is reviewed in the following sections. A summary of recent advancements is shown in Table 2.
5.1 Tissue engineering and regeneration
The processing of AM/dAM into a hydrogel form has just become a focus of research in the last decade. However, even in such a short time, it has proven its therapeutic potential in the field of tissue engineering and regeneration of injured tissues for a broad range of tissue types. These biomedical applications of AM/dAM hydrogels based on target tissue are summarized in the following sections.
5.1.1 Skin regeneration
Murphy et al. (2017) were among the pioneering research groups to develop amnion hydrogels by utilizing a photo-crosslinkable hyaluronic acid-based hydrogel containing solubilized amnion (HA-SAM). The HA-SAM liquid could be placed on the wound bed and crosslinked within several seconds of being exposed to UV light. An important feature was the utilization of native AM tissue instead of decellularized AM tissue. The solubilization process could kill the viable cells inside the AM tissue, inhibiting the immune rejection concerns. Further studies determined that the HA-SAM hydrogel provided accelerated wound closure, enhanced re-epithelialization, and higher density of small blood vessels compared to HA hydrogel-treated and non-treated wounds. The promoted neovascularization observed in wounds treated with HA-SAM hydrogel was most probably attributed to the presence of growth factors, such as fibroblast growth factor (FGF) family, epidermal growth factor receptor (EGF-R), and VEGF preserved within SAM (Cross and Claesson-Welsh, 2001). In another study, Murphy et al. (2020) showed that treating full-thickness wounds with HA-SAM hydrogel or AM powder resulted in faster wound closure rate, faster re-epithelialization, and minimum contraction compared to other treatment groups, including sterile bandage, HA hydrogel without SAM, and two commercial products of AmnioGraft (cryo-preserved amnion sheet) and Graftjacket (decellularized human dermis matrix). The better performance of AM-related products compared to other treatments could be attributed to the preservation of various biochemical cues (e.g., heparin sulfate, chondroitin sulfate, and other GAGs and proteoglycans) that regulate the regeneration of tissue (Murphy et al., 2020). The outcomes of skin regeneration by these AM products in the porcine model revealed the potential of these AM-derived products for translational medicine (Murphy et al., 2020). Zhang et al. (2021) applied a methacrylated gelatin (GelMA) layer over a methacrylated decellularized human AM (AdECMMA) to fabricate a photo-crosslinkable hydrogel for wound healing. This rationale design of the biomimetic bilayer was based on the mechanical support provided by the GelMA layer and the bioactivity provided by the AdECMMA component. Their results showed that the bilayer AdECMMA-GelMA skin substitute supported the transformation of fibroblasts into myofibroblasts and enhanced wound contraction during the wound-healing process. Furthermore, the bilayer scaffold exhibited promoting effects on collagen deposition and angiogenesis in vivo. These findings further elaborated the preference of the dAM-derived biomaterial compared to other prevalent biocompatible materials, such as GelMA, owing to its rich bioactive components (Zhang et al., 2021). Chen et al. (2023) have developed a composite hydrogel derived from a mixture of AdECMMA and GelMA for treating skin wounds. The wound healing process was improved accompanied by keratinization of the epidermal layer in the AdECMMA-GelMA group compared to the GelMA-only group. Interestingly, the infiltration of inflammatory cells in the tissue treated with composite hydrogel was significantly less than that in the wounds treated with GelMA-only hydrogel, which is likely due to intrinsic anti-inflammatory characteristic of dAM matrix (Chen et al., 2023). Nasiry et al. (2021) prepared three-dimensional (3D) microporous scaffolds by lyophilization of dAM hydrogels for the treatment of diabetic wounds. The engraftment of dAM-derived scaffolds into diabetic wounds caused upregulation of regeneration markers, including TGF-β, bFGF, and VEGF, and downregulation of pro-inflammatory cytokines, including TNF-α and IL-1β. The collagen deposition was also increased in diabetic wounds, which could be due to the presence of collagen fibers or stimulation of collagen synthesis by fibroblasts as a result of bFGF and TGF-β cytokines preserved in the dAM scaffold (Nasiry et al., 2021).
The insufficient printability of dAM-derived hydrogels is similar to other dECM-derived hydrogels due to their low viscosity and time-limiting temperature-sensitive gelation process (Kim et al., 2020). We have recently designed and developed a printable nanoengineered bioink based on dAM hydrogels for wound healing (Kafili et al., 2023b). We have shown that the employment of sodium alginate (Alg), as a structurally supportive component, in combination with dAM hydrogels provides enough stability for 3D printing of self-standing tubular constructs. We have also investigated the effect of Laponite nanosilicate as a physical crosslinker and rheology-modifying agent on the improvement of printing quality. Laponite is a disc-shaped two-dimensional nanoclay with a thickness of ∼1 nm and a diameter of about 25–30 nm (Kafili et al., 2023c). The anisotropic distribution of electric charges on the surface/edge of Laponite nanosilicates enables them to interact with various biopolymers non-covalently and strengthen the polymeric networks via the formation of physical crosslinks (Lokhande et al., 2018). Our results have affirmed that non-covalent electrostatic interactions between the positively charged edges of Laponite with anionic Alg or negatively charged surfaces of Laponite and amine groups of the dAM matrix improve the mechanical stability and rheological characteristics required for better printability (Kafili et al., 2023b). Nevertheless, nozzle clogging due to the formation of nanosilicate aggregates in ion-containing dAM solution was likely to occur when the Laponite concentration exceeded 2 %w/v. On the other hand, the addition of nanosilicates cooperates in the acceleration of cell proliferation and migration as a result of the bioactivity of ions degraded from the nanosilicates (Kafili et al., 2023b). In an attempt to improve the dispersibility of Laponite in dAM hydrogels, we have examined the effect of stabilization of nanosilicates by amine-terminated polyethylene glycol (AT-PEG) (Kafili et al., 2022). We have demonstrated that layer-by-layer self-assembly of collagen fibers and Laponite clusters occurs in Laponite-containing dAM hydrogels due to the disparate hydrophilic nature of nanosilicates and the dAM matrix. The coating of Laponite nanosilicates with the hydrophilic AT-PEG agent improves their distribution in the hydrophilic dAM matrix (Kafili et al., 2022). Therefore, dAM hydrogels containing PEG-modified nanosilicates are a potential candidate for TE applications, particularly for skin regeneration.
Rahman et al. (2019) have shown the advantageous effect of AM/Aloe vera (AV)/carboxymethyl cellulose sodium (CMC-Na) hydrogels for the medication of second-degree burns. Their results have determined that the healing rate of burn wounds treated with AV hydrogel is higher than that of AM-treated and AM/AV-treated wounds. However, more scar formation is noticeable after 4 weeks. Herein, the scar formation in the AM group is less than in the AV and AM/AV groups. The AM/AV group also exhibits a higher inflammatory score after 18 days of treatment. Therefore, it can be deduced that the synergistic effect of AM/AV facilitates the re-epithelialization process without affecting wound healing in terms of the inflammatory response, healing rate, remaining scar mark, and angiogenesis. Rana et al. (2020) have developed a hydrogel containing AM, collagen, and CMC-Na gelling agent for treating second-degree burn wounds. Their results indicate that the AM-containing hydrogel facilitates the healing process without a sign of scar formation and promotes re-epithelialization in a quicker time, as compared to the control groups (i.e., 1% silver sulfadiazine and the non-treated group as positive and negative control, respectively). Islam et al. (2023) have shown the positive effect of titanium dioxide (TiO2) nanoparticles (NPs) in AM gels containing Carbopol 934 (as a gelling agent) for the treatment of second-degree burn wounds. Their study reveals that treating burn wounds with AM-TiO2 gels enhances wound closure and re-epithelialization while encouraging vascular formation. Their results also exhibit less scar formation after the treatment of burn wounds, which is an important feature from the aesthetic point of care. Similarly, the antibacterial, anti-inflammatory, and anti-angiogenic properties of silver NPs for the treatment of burn wounds have been shown in several studies (Pourali and Yahyaei, 2016; Huang et al., 2022; Ajaykumar et al., 2023). For instance, Jhumi et al. (2023) have shown that the incorporation of Ag NPs into AM gels accelerates the healing of second-degree burn wounds in terms of expedited re-epithelialization and elevated contraction. It is noteworthy that in this study and many others (Rahman et al., 2019; Rana et al., 2020; Islam et al., 2023; Jhumi et al., 2023), AM tissue has been utilized without decellularization.
5.1.2 Heart regeneration and treatment
Myocardial infarction (MI) is a heart failure-associated issue caused by blockage of blood flow to the heart followed by ischemia and tissue death (Kim et al., 2018). The disability of the myocardial tissue to regenerate itself after MI leads to scar formation during left ventricular (LV) regeneration and eventually heart failure (Tallquist and Molkentin, 2017). So far, various tissue engineering (TE)-based treatments, including stem cell therapies, cardiac patches, and injectable hydrogels, have been employed to improve cardiac regeneration after MI (Hashimoto et al., 2018). However, studies on the application of dAM-derived hydrogels for heart treatment are rare. The angiogenesis, antifibrotic, and anti-inflammatory properties of the dAM-derived matrix offer an exquisite alternative for the treatment of heart post-MI (Blume et al., 2021). In a recent study by Henry et al. (2020), the treatment of MI-induced myocardium using injectable dAM hydrogels has been demonstrated. They have shown that dAM-treated myocardium with acute infarction exhibits higher left ventricular ejection fraction (LVEF), enhanced fractional shortening, and reduced infarct size compared with the PBS-treated control group.
5.1.3 Vascular tissue engineering
Cardiovascular diseases are among the common complications faced by modern societies that may lead to death in some cases (Newman et al., 2017). One of the main medical procedures for treating cardiovascular diseases is the replacement of the vasculature with artificial grafts (Adipurnama et al., 2017). Synthetic vascular grafts suffer from the occurrence of stenosis or thrombus after transplantation due to their intrinsic procoagulant property and low cell adhesion rate (Radke et al., 2018). Among various dECM-derived materials, dAM has attracted abundant attention for vascular replacement due to its biological properties, including cytocompatibility, anti-inflammation, anti-fibrosis, and low immunogenicity (Swim et al., 2019; Cheng et al., 2021; Wang et al., 2023). Nevertheless, the dAM sheet lacks the appropriate processability required for vascular reconstruction while its rapid biodegradability limits in vivo applications (Wehmeyer et al., 2015). Peng et al. (2020) have developed a dAM hydrogel-based biomaterial as an artificial vascular intima that mimics the structural and functional features of natural blood vessels (Figure 4Ai). The designed vascular graft is composed of a thermosensitive dAM hydrogel crosslinked with alginate dialdehyde (ADA) via chief-base reaction. The crosslinking is preceded by imine linkage between aldehyde groups of ADA and amine groups of dAM. The hydrogel is further grafted with Arg-Glu-Asp-Val polypeptide (REDV) via 1-ethyl-3-(3-dimethyl aminopropyl) carbo-diimide (EDC)/N-hydroxy sulfosuccinimide (NHS) catalysis to mimic the anticoagulant characteristic of natural blood vessels (Figure 4Aii). The REDV peptide is an endothelial cell (EC)-specific ligand that is commonly utilized for the modification of vascular substitutes because of its potential to stimulate rapid endothelialization while inhibiting platelet adhesion (Yang et al., 2015). As shown in Figure 4Aiii, this vascular graft shows selectivity for supporting the adhesion and proliferation of ECs while impeding the attachment and proliferation of smooth muscle cells (SMCs) (Peng et al., 2020). Implantation of the ADA/REDV-dAM vascular graft in rabbit models provides a template for EC growth along with hindering thrombosis occurrence. Lei et al. (2020) have developed a vascular graft based on dAM-polyacrylamide (PAM)-alginate (Alg) hydrogels to support the adhesion, proliferation, and migration of ECs while inhibiting the activation of platelets. This vascular graft enhances the secretion of nitrogen oxide (NO) and prostacyclin (PGI2) by HUVECs, which in turn play important roles in vascular remodeling. Besides, the graft exhibits an anti-calcification effect, which is presumably related to the presence of anti-inflammatory factors within the dAM matrix. These factors significantly downregulate the secretion of calcification-related proteins by inflammatory mediators, such as TNF-α and IL-6 (Pober and Sessa, 2015; Lei et al., 2020).
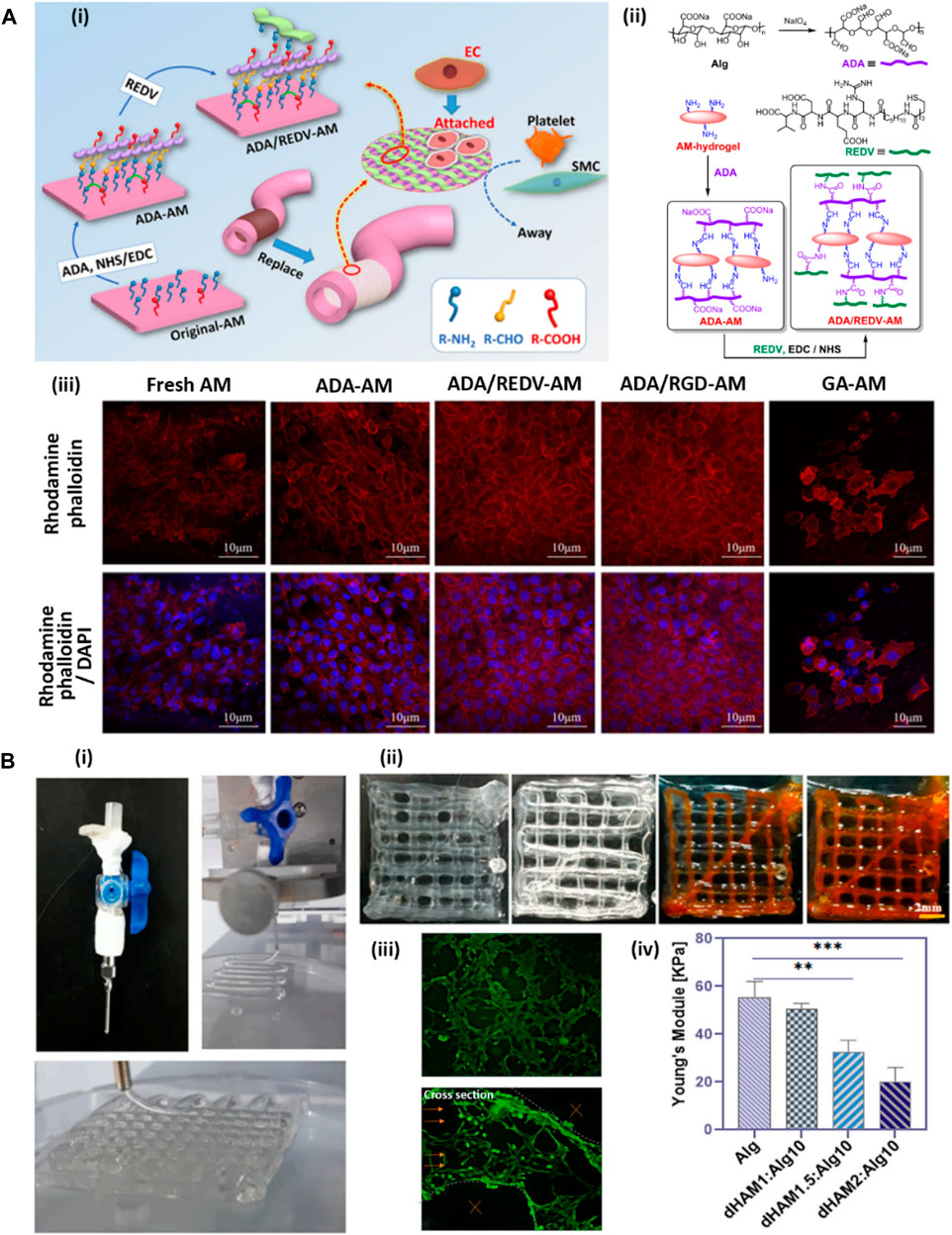
FIGURE 4. Development of AM-based hydrogels for vascular tissue engineering [adapted with permission from Peng et al. (2020), Copyright (2020) American Chemical Society]. (A) ADA-dAM hybrid hydrogel modified with REDV peptide as a vascular graft. (A-i) Representative image of modification steps of dAM hydrogel as an artificial vascular graft. (A-ii) Chemical bondings between dAM, ADA, and REDV peptide. (A-iii) CLSM images of HUVECs loaded in various dAM-based hydrogels, including fresh dAM, ADA-dAM, ADA/REDV-dAM, ADA/RGD-dAM, and GA-crosslinked dAM hydrogels stained with rhodamine-phalloidin for cytoskeleton organization and DAPI for cell nuclei. (B) Coaxial bioprinting of vascularized constructs [Reprinted from Heidari et al. (2023), Copyright (2023), with permission from Elsevier]. (B-i) Bioprinting of lattice structure using coaxial nozzle containing cell-loaded Alg-dAM particulate bioink as sheath and CaCl2 crosslinker solution as core material. (B-ii) Perfussability of the microchannels in 4-layer bioprinted construct exhibited through injection of orange dye. (B-iii) Live-dead staining of HUVECs in bioprinted microchannels and their cross section indicating tubulogenesis. (B-iv) Mechanical properties of lattice constructs printed using bioinks containing various amounts of dAM powder.
In a recent study, a cell-laden construct with vessel-like microchannels was 3D bioprinted using a coaxial nozzle with cell-encapsulated Alg bioink containing particulate dAM tissue as sheath and CaCl2 crosslinker solution as core material (Figure 4Bi) (Heidari et al., 2023). By changing the feed rate of core and sheath materials, perfusable microchannels with a thickness between 100 and 400 μm were successfully fabricated (Figure 4Bii). This study confirmed that HUVECs encapsulated in optimized bioink with 0.6 %w/v dAM particle content within the bioprinted construct were able to arrange themselves toward tube formation as a sign of the formation of blood vessels (Figure 4Biii). Therefore, these types of bioprinted constructs may have great potential for engineering pre-vascularized thick tissues. However, using the particulate dAM in the bioink formulation led to a declined elastic modulus of the bioprinted constructs as a result of weak chemical bonding between Alg and dAM particles (Figure 4Biv). Moreover, the high content of dAM particles in the bioink hydrogel caused reduced pore sizes by filling the pore areas within the bioprinted scaffold, which may harm nutrient transportation and cell activities, such as cell proliferation. Therefore, it seems that using the solubilized form of dAM as bioink can be considered a better alternative instead of the direct incorporation of dAM particles in the formulation to resolve the above-mentioned challenges (Lee et al., 2020). In another study done by Comperat et al. (2023), bioinks comprised of 0.3% methacrylated decellularized amnion (AdECMMA) or methacrylated decellularized chorion (CdECMMA) reinforced with 1.5% methacrylated hyaluronic acid (Hya-MA) was utilized for 3D bioprinting of vascularized explants. The matured tissue constructs bioprinted with HUVECs encapsulated in AdECMMA-HyaMA and CdECMMA-HyaMA bioinks demonstrated a better capillary-like structure organization and vasculogenic networks compared to HyaMA-only bioink as the control group. However, the incorporation of CdECMMA or AdECMMA in the bioink did not provide significantly different results for the in vitro maturation of ECs.
5.1.4 Cartilage regeneration
Osteoarthritis (OA) is a disease condition of cartilage degeneration caused by inflammation induced by the expression of IL-1β and TNF-α pro-inflammatory cytokines (Heinegård and Saxne, 2011). Bhattacharjee et al. (2020) have employed a dAM hydrogel, as a platform for delivering adipose-derived stem cells (ADSCs), to decrease IL-1β induced inflammation in stimulated chondrocytes. ADSCs are well-known for their paracrine effects on inhibiting inflammation and down-trending cartilage degeneration through secreting anti-inflammatory factors (e.g., IL-10, IL-1RA, and TGF-β). On the other hand, the AM is capable of suppressing pro-inflammatory cytokines, including IL-1α and IL-1β, and decreasing the expression of matrix-degrading factors (MMPs) through the presence of natural MMP inhibitors within its matrix (Niknejad et al., 2008). Moreover, the elastase inhibitors, including elafin, SLPI, and β-defensin within AM, are responsible for their potential anti-inflammatory and antibacterial properties (King et al., 2007). Hence, dAM hydrogels for carrying ADSCs not only support the viability, proliferation, and stemness of stem cells but also provide a synergistic effect on reducing catabolic responses of inflamed chondrocytes (Bhattacharjee et al., 2020). In another study, Bhattacharjee et al. (2022) investigated the effect of intraarticular injecting of the dAM hydrogel with or without ADSCs into a collagenase-induced OA rat model. They have shown that both cell-free dAM hydrogels and ADSC suspensions provide comparable outcomes in decreasing inflammation-induced damage and promoting tissue regeneration in articular cartilage. Meanwhile, the encapsulation of ADSCs into dAM hydrogels synergetically enhances the therapeutic effect.
Chitosan, a linear polysaccharide derived from chitin, is well-recognized for its biocompatibility and antibacterial properties (Tohidi et al., 2022). The combination of chitosan with the dAM matrix containing various types of collagen (especially collagen type IV) offers an interesting choice of biomaterial for biomimicking the cartilage-related microenvironment (Toniato et al., 2020). Toniato et al. (2020) have developed a hybrid chitosan-dAM hydrogel for articular cartilage TE. Despite tailoring the mechanical properties by adjusting the concentration of dAM (2.5, 5, and 10%w/v) at constant chitosan concentration (2%w/v), the highest obtained elastic modulus (i.e., ∼80 kPa for 2% chitosan-5% dAM hydrogel) is still inferior to that of native articular cartilage [500–2000 kPa (Zhou et al., 2018)]. Therefore, chitosan-dAM hydrogels require further mechanical support to better mimic the cartilage tissue regarding mechanical properties. Hussin et al. (2011) have fabricated 3D scaffolds for cartilage TE by incorporating the HAM particulates (without decellularization) into fibrin hydrogels. The resemblance of the chondrocyte-embedded fibrin-HAM hydrogel to hyaline cartilage with the capability of secreting sulfated GAG has been demonstrated.
5.1.5 Endometrium and fetal membrane regeneration
Intrauterine adhesion (IUA), also known as Asherman syndrome (AS), is a uterus disease situation related to the formation of fibrotic tissue in the uterine cavity as a result of damage to the endometrium (Hooker et al., 2014). Decellularized and lyophilized AM is one of the ECM-derived biomaterials that have been utilized clinically for treating intrauterine damage by suppressing TGF-β1 (which is responsible for developing IUA) (Chen et al., 2018). However, the fresh dAM membrane is very difficult to fix and suture into the uterus cavity. Hence, processing dAM into hydrogels provides the opportunity to fill irregularly shaped cavities. Islam et al. (2023) have recently employed a thermosensitive dAM hydrogel to prevent IUA in rat models. It has been shown that the injection of the dAM hydrogel into the uterus cavity can significantly decrease fibrosis formation through re-epithelialization of the damaged endometrium (Figures 5Ai, ii), while supporting angiogenesis within the regenerated endometrium (Figures 5Aiii, iv).
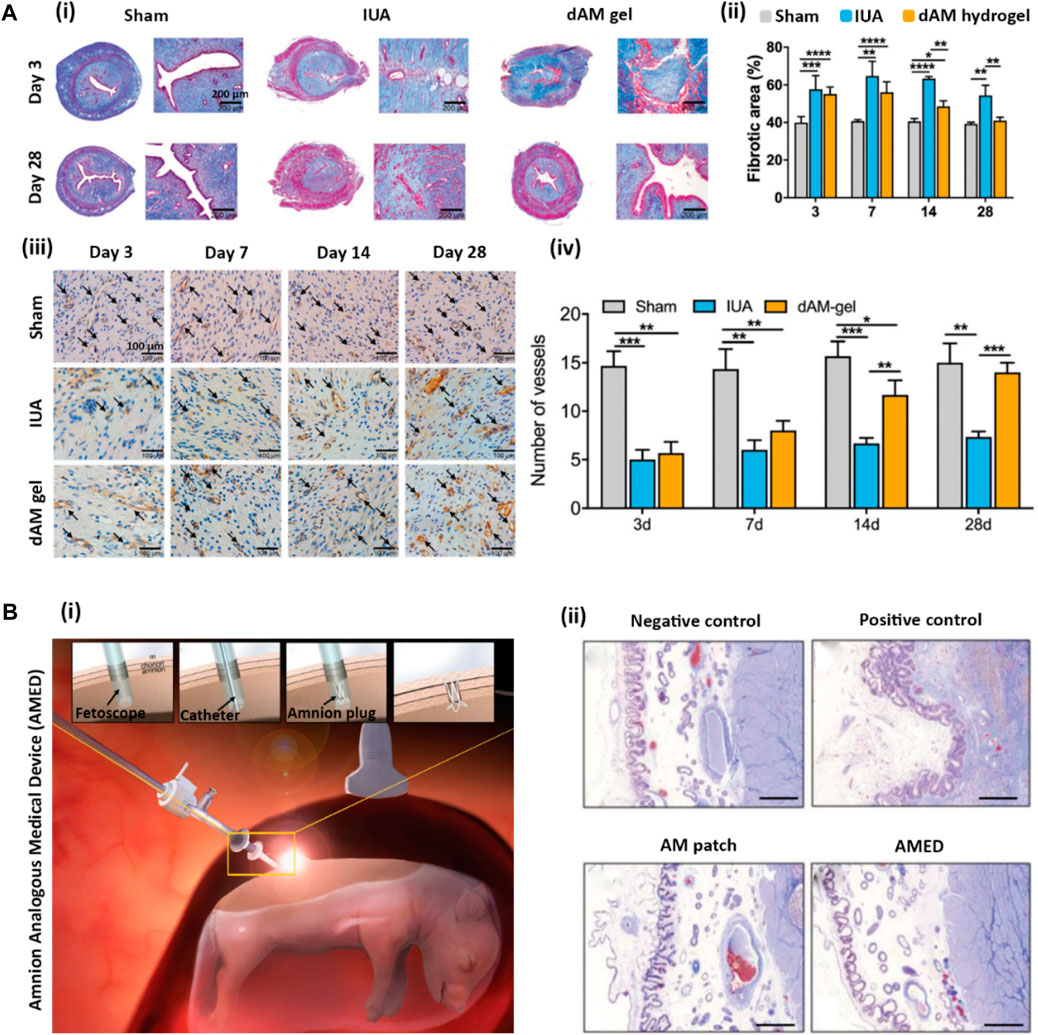
FIGURE 5. (A) Endometrium regeneration using dAM hydrogel [Reprinted from Li et al. (2022), Copyright (2022), with permission from Royal Society of Chemistry]. (A-i) Histological Masson’s trichrome staining of uterine after 3–28 days after operation in the sham operation group (Sham), endometrium damage group (IUA), and dAM hydrogel treated group (dAM gel); (A-ii) Quantification of fibrotic area in uterine treated from different groups; (A-iii) IHC staining of CD31 at 3, 7, 14, and 28 days post-operation (Black arrows show the capillaries); (A-iv) quantification of number of blood vessels. (B) Healing of fetal membrane using developed decellularized amnion analogous medical device (AMED) [Reprinted from Lee et al. (2018), Copyright (2018), with permission from Wiley]. (B-i) Fetoscopic surgery procedure using inserted AMED to fill the amniotic cavity; (B-ii) Histological Masson’s trichrome staining of en bloc sections of uterine in negative control (unmanipulated sac), positive control (unclosed entry site), and fetoscopic access site covered with commercial AmnioGraft patch and with AMED.
The rupture of fetal membranes during pregnancy is a serious issue that endangers the fetus and leads to premature delivery (Chmait et al., 2017). Lee et al. (2018) have designed a 3D-printed dAM-analogous medical device (AMED) comprising a polycaprolactone (PCL) framework filled with a dAM hydrogel for treating fetal membrane defects (Figure 5Bi). The fabricated AMED exhibits surgical handling privilege along with biocompatibility, non-immunogenicity, and proper healing of AM compared to other commercial equivalents (such as AmnioGraft patch, Bio-Tissue Inc.) to seal the fetal membrane and block the leakage of amniotic fluid (Figure 5Bii). As the developed AMED is easy to apply by a fetoscopic instrument, the device significantly decreases the surgical time in comparison with the transplantation of AmnioGraft.
5.1.6 Dental pulp regeneration
An appropriate scaffold for endodontic regeneration requires biocompatibility to support the growth, proliferation, and differentiation of cells to the odontogenic lineage (Yuan et al., 2011). So far, various types of scaffolds using natural, synthetic, and composite biomaterials have been utilized for the restoration of endodontic functions (Moussa and Aparicio, 2019). Among the various types of scaffolds, injectable hydrogels are a preferable option to fill the canal roots with complex geometry (Chang et al., 2017). The dAM tissue as a biological scaffold is considered a promising candidate for dental pulp regeneration, mostly owing to its angiogenic and anti-inflammatory properties along with its accessibility (Honjo et al., 2015). Bakhtiar et al. (2022) have investigated the potential of spongy scaffolds derived from dAM hydrogels for regenerative endodontics. They have shown that the subcutaneous implantation of scaffolds derived from dAM hydrogels can cause mild to moderate inflammatory responses as evidenced by the migration of mononuclear inflammatory cells, such as lymphocytes and macrophages, and primary multinucleated giant cells to the implanted scaffolds. Interestingly, both the cell-free scaffolds and human dental pulp stem cells (hDPSCs)-loaded scaffolds have exhibited pulp-like tissue growth in the root canal area accompanied by angiogenesis after 7 weeks of implantation. The observed regeneration capacity is attributed to the presence of bioactive molecules, including bFGF and TGF-β, in the dAM matrix (Kim et al., 2012). However, a mild fibrosis formation has also been observed (Bakhtiar et al., 2022). In another study, pulp regeneration has been studied by injectable dAM hydrogels with or without hDPSCs (Bakhtiar et al., 2023). To reduce the biodegradability of the hydrogel, Genipin (up to 10 mM) was utilized to react with free amine residues of dECM (Nagaoka et al., 2014; Výborný et al., 2019). In vivo studies on the tissue regeneration in the root canal model implanted in rat calvaria have revealed that the hDPSC-seeded dAM hydrogels do not cause a significant difference in pulp-like tissue formation compared to cell-free counterparts. Therefore, the pulp-like tissue regeneration is likely attributed to the migration of housing calvarial progenitor cells instead of the odontogenic differentiation of hDPSC cells. No significant differences in infiltration of inflammatory cells, angiogenesis, and fibrosis have also been noticed. In summary, the dAM hydrogels seem to be useful for the formation of vascularized pulp-like tissue without sufficient effect on the formation of tubular dentin structure (Bakhtiar et al., 2023).
5.1.7 Ocular regeneration
Both corneal tissue and AM share some mutual characteristics like thin thickness, being avascular, and proper light transmittance (Deihim et al., 2016). Therefore, AM has a long history of ocular transplantation for treating corneal injuries or diseases by promoting re-epithelialization, reducing scar formation and neovascularization, and controlling inflammatory responses (Pogozhykh et al., 2020). However, AM transplantation is prone to dissolving and rapid degradation that burdens multiple painful surgeries and might cause allergic reactions in some cases as an allograft (Shimazaki et al., 2004). Therefore, processing dAM into in situ forming hydrogels seems to be a practical solution to address these issues.
Yazdanpanah et al. have shown that thermoresponsive corneal dECM (dCO)-derived hydrogel possesses proper transparency as well as bioactivity to be used in ocular TE applications, owing to the presence of essential growth factors and proteins, such as collagen, lumican, keratocan, and laminin within corneal dECM (Yazdanpanah et al., 2021). They also compared the ECM composition of dCO with dAM and showed that these two tissue matrices share a great portion of similar proteins. Moreover, the dAM contained proteins like decorin and periostin, which are well-known for their therapeutic effects on the cornea (Yazdanpanah et al., 2021; R Mohan et al., 2011). These comparisons pave the way for the application of dAM hydrogel for ocular regeneration as well. We even predict that thermoresponsive dAM hydrogel can even provide better dynamic mechanical properties compared to dCO hydrogel at the same concentration by comparing available data in the literature [e.g., G′∼ 40 Pa for dCO hydrogel (Yazdanpanah et al., 2021) as compared to G′∼1.5 kPa for dAM hydrogel (Li et al., 2022) at the same concentration of 15 mg.mL−1]. The higher mechanical strength of dAM hydrogel compared to dCO hydrogel can enhance the structural stability of hydrogel upon application on ocular defects. However, to the best of our knowledge, there is no publication about the application of dAM hydrogels for ocular regeneration or TE, yet. Nevertheless, the incorporation of AM extract into various hydrogel formulations for treating ocular diseases has been exploited in several cases. For example, Luo et al. (2021) proposed the application of dAM extract (dAME) in different ways, such as eye drops, and the incorporation of dAME in temperature-sensitive P407 hydrogels or freeze-dried PVA tablets to treat corneas with acid burns. Treatment of severe corneal burns with these three alternatives was accompanied by the best results in terms of less stromal opacity and faster corneal regeneration for in situ forming dAME containing P407 hydrogel, followed by second best results for dAME containing PVA tablets, and moderate results for dAME liquid drop. The better therapeutic effect of dAME incorporated into a hydrogel can be due to sustained and long-term release of dAME compared to two other administration methods that improve its efficiency and reduce its loss during treatment. Moreover, the mRNA expression of TGF-β1 in corneal burns treated with hydrogel and tablet containing dAME was less than that in dAME liquid drop-treated and control groups, which impedes excessive stromal fibrosis. During the inflammatory phase of healing, the corneas treated with dAME incorporated hydrogel and tablet expressed higher LRIG1, which is a protein in corneal cells that prevents inflammatory responses and contributes to corneal transparency. In another study, eye pads were fabricated by loading AM extract (AME) in GelMA hydrogel and were used for treating ocular chemical injuries (Chen et al., 2020). The AME-GelMA eye pads showed similar re-epithelialization compared to AM transplantation, while the expression of TGF-β1 in AME-GelMA treated groups was lower than that in AM transplanted and control groups leading to the occurrence of less scar hyperplasia. In addition to these therapeutic effects, the AME-loaded GelMA eye pads have the advantage of processability and easier handling over AM tissue and can be fabricated in a dome shape to fit the conjunctival sac.
5.2 Cell therapy and cellular studies
In addition to the therapeutic effect of AM hydrogel for various TE and regenerative applications, it can further be utilized for other biological applications. For instance, AM hydrogel has shown excellent potential as a cell carrier for stem cell therapy (Ryzhuk et al., 2018). Moreover, it has the potential to be used as an in vitro platform to study cell behaviors, such as cell-matrix interactions, and the mechanistic effect of matrix on cell phenotypes, or be applied for disease modeling and drug screening purposes (Deus et al., 2022). Accordingly, these applications are discussed in the following sub-section.
5.2.1 Cell carrier and cell culture platform
During the last decade, the delivery of stem cells to injured sites has gained great attention and has been applied in several pre-clinical and clinical experiments (Margiana et al., 2022). Stem cells are known to regulate the regeneration process of injured tissues by secreting various types of cytokines and growth factors (Murphy et al., 2013). However, the delivery of cells into the target tissue still suffers from insufficient cell survival and cell engraftment after transplantation. For instance, mesenchymal stem cells (MSCs) are required to adhere and spread in the desired environment to survive, thereby cell suspension delivery significantly degrades their integration into the target tissue (Phinney and Prockop, 2007). Systemic delivery of stem cells also faces serious issues related to their entrapment in the capillaries of untargeted tissues (Kraitchman et al., 2005). Therefore, the delivery of stem cells in a biocompatible carrier into the desired defect site can ensure their viability and survival after transplantation (Slaughter et al., 2009). Ryzhuk et al. (2018) have shown the potential of dAM hydrogels for the delivery of different cell sources, including placenta-derived mesenchymal stem cells (PMSCs), by supporting their viability and biological functionality. The implantation of dAM hydrogel in the back of rats exhibits less immune cell infiltration as compared to collagen hydrogel. The lower density of CCR7+ cells (macrophage marker) in dAM hydrogel compared to collagen hydrogel further affirms less immune reaction. Deus et al. (2022) have developed photo-crosslinkable methacrylated decellularized amnion (AdECMMA) hydrogels as a versatile platform for cell culture and TE. The results demonstrate that the degree of methacrylation has a significant effect on the morphology and viability of the encapsulated hBM-MSCs due to variations in the viscoelastic properties of hydrogels and their structural porosity. Micro- and nano-topographical grooves were made on the surface of the hydrogels and used as a guidance pattern for hBM-MSCs alignment. It has also been shown that both micro- and nano-features are very effective on cell spreading as compared to the hydrogel without topographical features. Haghshenas et al. (2022) have developed dAM-Alg hybrid hydrogels containing various amounts of dAM (15 mg/mL, 30 mg/mL, 45 mg/mL) for the 3D culture of oocytes. Although the developed hydrogels are efficient carriers for long-term culture of oocytes, no significant differences in antral formation rate, follicle diameter, and estradiol secretion are noticed as compared to the control group (Alg-only hydrogel). This inferior functionality is attributed to the loss of growth factors, such as bFGF and EGF, after decellularization of AM using sodium dodecyl sulfate (SDS) that are known to improve follicle growth (Price, 2016). Hence, the employment of other agents for the decellularization process of AM should be considered for future studies to better preserve biochemical cues within the dAM matrix for delivering oocytes.
6 Conclusion and future prospectives
The AM tissue has a long history in the medical field and has already been approved for clinical applications, such as treating burn wounds and corneal regeneration. There are also several commercial AM-related products for clinical use (Nejad et al., 2021). During the last decade, AM/dAM hydrogels have shown wonderful therapeutic outcomes for the regeneration of damaged tissues (Dadkhah Tehrani et al., 2021). Therefore, clinical applications of AM/dAM hydrogels are a rational and possible trend. Nevertheless, clinicians and researchers need to be more focused on examining the function of AM/dAM hydrogels on large animals and standardizing it for clinical applications to validate its safety and facilitate the approval processes.
Hydrogels derived from AM are considered a valuable platform for various medical therapies, owing to their reservoir of active biochemical cues and excellent bio-functionality (Deus et al., 2022). However, multiple processing steps, including decellularization and enzymatic digestion, can lead to the loss of some ECM components and alleviated mechanical stability (Sasikumar et al., 2019). It has been hypothesized that the utilization of micronized AM inside a hydrogel formulation can address this issue (Heidari et al., 2023). However, these materials may suffer from the inhomogeneous distribution of bioactive AM particles in the hydrogel matrix, interfering with expected therapeutic results. Processing AM/dAM into a hydrogel form or utilizing AM/dAM extracts can be considered practical alternatives to resolve these issues. Besides, the hydrogel derived from dAM privilege over AM hydrogel in the concept of thermo-sensitivity for minimally invasive therapies (Li et al., 2022).
Despite the promising medical outcomes of dAM hydrogels, their low mechanical properties and rapid degradability are some issues that need to be addressed. Several trending methods, such as crosslinking and modifying the hydrogel matrix with methacrylate groups for photo-crosslinking, may be helpful for the reinforcement of dAM hydrogel networks (Deus et al., 2022; Bakhtiar et al., 2023). Moreover, the combination of nanotechnology approaches with dAM hydrogel can be considered a beneficial tool in several biomedical directions. For instance, the incorporation of gold nanorods in dAM hydrogels can be considered for improving electrical conductivity and enhancing the functionality and maturation of cardiomyocyte cells (Zhang et al., 2019). The incorporation of TiO2 and silver NPs can offer antibacterial properties for treating infected or burn wounds as well (Islam et al., 2023; Jhumi et al., 2023). Other biocompatible nanomaterials, such as layered nanosilicates, are also usable for enhancing mechanical stability and printability (Kafili et al., 2023b). These kinds of examples seem to be endless and worth applying to provide superior therapeutic outcomes for AM/dAM hydrogels. Meanwhile, it is of great importance to precisely explore the effectiveness and biosafety of modified AM/dAM hydrogels before in vivo and pre-clinical experiments.
3D bioprinting is a powerful and reliable technique that facilitates controlling the internal structure and bulk dimensions of designed scaffolds (Abaci and Guvendiren, 2020). This fabrication method also provides control over the deposition of desired biomaterial or intended cell types on the exact pre-defined location, which expands the medical relevance of scaffolds by mimicking native biosystems (De Santis et al., 2021). It has been a while since dECM-derived bioinks have proven their priority for TE and RM, owing to their intrinsic biochemical cues, such as growth factors, cytokines, glycosaminoglycans, and regulatory proteins (Choudhury et al., 2018). Nevertheless, the application of dAM bioinks for 3D bioprinting has not been widely explored yet except few studies (Lee et al., 2018; Kafili et al., 2023b; Comperat et al., 2023). The preparation of bioinks based on dAM hydrogels is a huge breakthrough, which broadens its potential for regenerative therapy.
Author contributions
GK: Conceptualization, Writing–original draft, Writing–review and editing. HN: Writing–review and editing. ET: Writing–review and editing. AS: Conceptualization, Investigation, Writing–review and editing, Methodology.
Funding
The authors declare that no financial support was received for the research, authorship, and/or publication of this article.
Conflict of interest
The authors declare that the research was conducted in the absence of any commercial or financial relationships that could be construed as a potential conflict of interest.
The reviewer FS declared a shared affiliation with the author HN to the handling editor at the time of review.
Publisher’s note
All claims expressed in this article are solely those of the authors and do not necessarily represent those of their affiliated organizations, or those of the publisher, the editors and the reviewers. Any product that may be evaluated in this article, or claim that may be made by its manufacturer, is not guaranteed or endorsed by the publisher.
References
Abaci, A., and Guvendiren, M. (2020). Designing decellularized extracellular matrix-based bioinks for 3D bioprinting. Adv. Healthc. Mater. 9 (24), 2000734. doi:10.1002/adhm.202000734
Abbasi-Kangevari, M., Ghamari, S.-H., Safaeinejad, F., Bahrami, S., and Niknejad, H. (2019). Potential therapeutic features of human amniotic mesenchymal stem cells in multiple sclerosis: immunomodulation, inflammation suppression, angiogenesis promotion, oxidative stress inhibition, neurogenesis induction, MMPs regulation, and remyelination stimulation. Front. Immunol. 10, 238. doi:10.3389/fimmu.2019.00238
Abdo, R. (2016). Treatment of diabetic foot ulcers with dehydrated amniotic membrane allograft: a prospective case series. J. Wound Care 25 (7), S4–S9. doi:10.12968/jowc.2016.25.Sup7.S4
Adipurnama, I., Yang, M.-C., Ciach, T., and Butruk-Raszeja, B. (2017). Surface modification and endothelialization of polyurethane for vascular tissue engineering applications: a review. Biomaterials Sci. 5 (1), 22–37. doi:10.1039/C6BM00618C
Ajaykumar, A. P., Mathew, A., Chandni, A. P., Varma, S. R., Jayaraj, K. N., Sabira, O., et al. (2023). Green synthesis of silver nanoparticles using the leaf extract of the medicinal plant, uvaria narum and its antibacterial, antiangiogenic, anticancer and catalytic properties. Antibiotics 12 (3), 564. doi:10.3390/antibiotics12030564
Andrewartha, N., and Yeoh, G. (2019). Human amnion epithelial cell therapy for chronic liver disease. Stem Cells Int. 2019, 1–10. doi:10.1155/2019/8106482
Arki, M. K., Moeinabadi-Bidgoli, K., Hossein-Khannazer, N., Gramignoli, R., Najimi, M., and Vosough, M. (2023). Amniotic membrane and its derivatives: novel therapeutic modalities in liver disorders. Cells 12 (16), 2114. doi:10.3390/cells12162114
Arrizabalaga, J. H., and Nollert, M. U. (2018). Human amniotic membrane: a versatile scaffold for tissue engineering. ACS Biomaterials Sci. Eng. 4 (7), 2226–2236. doi:10.1021/acsbiomaterials.8b00015
Babajani, A., Manzari-Tavakoli, A., Jamshidi, E., Tarasi, R., and Niknejad, H. (2022b). Anti-cancer effects of human placenta-derived amniotic epithelial stem cells loaded with paclitaxel on cancer cells. Sci. Rep. 12 (1), 18148. doi:10.1038/s41598-022-22562-w
Babajani, A., Moeinabadi-Bidgoli, K., Niknejad, F., Rismanchi, H., Shafiee, S., Shariatzadeh, S., et al. (2022a). Human placenta-derived amniotic epithelial cells as a new therapeutic hope for COVID-19-associated acute respiratory distress syndrome (ARDS) and systemic inflammation. Stem Cell Res. Ther. 13 (1), 126–222. doi:10.1186/s13287-022-02794-3
Bakhshandeh, H., Atyabi, F., Soleimani, M., Taherzadeh, E. S., Shahhoseini, S., and Cohan, R. A. (2021). Biocompatibility improvement of artificial cornea using chitosan-dextran nanoparticles containing bioactive macromolecules obtained from human amniotic membrane. Int. J. Biol. Macromol. 169, 492–499. doi:10.1016/j.ijbiomac.2020.12.125
Bakhtiar, H., Ashoori, A., Rajabi, S., Pezeshki-Modaress, M., Ayati, A., Mousavi, M. R., et al. (2022). Human amniotic membrane extracellular matrix scaffold for dental pulp regeneration in vitro and in vivo. Int. Endod. J. 55 (4), 374–390. doi:10.1111/iej.13675
Bakhtiar, H., Mousavi, M. R., Rajabi, S., Pezeshki-Modaress, M., Ayati, A., Ashoori, A., et al. (2023). Fabrication and characterization of a novel injectable human amniotic membrane hydrogel for dentin-pulp complex regeneration. Dent. Mater. 39 (8), 718–728. doi:10.1016/j.dental.2023.06.008
Baradaran Rafii, A. R., Aghayan, H.-R., Arjmand, B., and Javadi, M.-A. (2007). Amniotic membrane transplantation.
Barboni, B., Russo, V., Curini, V., Mauro, A., Martelli, A., Muttini, A., et al. (2012). Achilles tendon regeneration can be improved by amniotic epithelial cell allotransplantation. Cell Transplant. 21 (11), 2377–2395. doi:10.3727/096368912X638892
Barr, S. M. (2014). Dehydrated amniotic membrane allograft for treatment of chronic leg ulcers in patients with multiple comorbidities: a case series. J. Am. Coll. Clin. Wound Specialists 6 (3), 38–45. doi:10.1016/j.jccw.2016.01.002
Bhattacharjee, M., Escobar Ivirico, J. L., Kan, H.-M., Shah, S., Otsuka, T., Bordett, R., et al. (2022). Injectable amnion hydrogel-mediated delivery of adipose-derived stem cells for osteoarthritis treatment. Proc. Natl. Acad. Sci. 119 (4), e2120968119. doi:10.1073/pnas.2120968119
Bhattacharjee, M., Ivirico, J. L. E., Kan, H.-M., Bordett, R., Pandey, R., Otsuka, T., et al. (2020). Preparation and characterization of amnion hydrogel and its synergistic effect with adipose derived stem cells towards IL1β activated chondrocytes. Sci. Rep. 10 (1), 18751. doi:10.1038/s41598-020-75921-w
Bianchi, C., Cazzell, S., Vayser, D., Reyzelman, A. M., Dosluoglu, H., Tovmassian, G., et al. (2018). A multicentre randomised controlled trial evaluating the efficacy of dehydrated human amnion/chorion membrane (EpiFix®) allograft for the treatment of venous leg ulcers. Int. wound J. 15 (1), 114–122. doi:10.1111/iwj.12843
Bianchi, C., Tettelbach, W., Istwan, N., Hubbs, B., Kot, K., Harris, S., et al. (2019). Variations in study outcomes relative to intention-to-treat and per-protocol data analysis techniques in the evaluation of efficacy for treatment of venous leg ulcers with dehydrated human amnion/chorion membrane allograft. Int. Wound J. 16 (3), 761–767. doi:10.1111/iwj.13094
Biniazan, F., Rajaei, F., Darabi, S., Babajani, A., Mashayekhi, M., Vousooghi, N., et al. (2022). Effects of placenta-derived human amniotic epithelial cells on the wound healing process and TGF-β induced scar formation in murine ischemic-reperfusion injury model. Stem Cell Rev. Rep. 18 (6), 2045–2058. doi:10.1007/s12015-022-10355-7
Blume, G. G., Machado-Júnior, P. A. B., Bertinato, G. P., Simeoni, R. B., Francisco, J. C., and Guarita-Souza, L. C. (2021). Tissue-engineered amniotic membrane in the treatment of myocardial infarction: a systematic review of experimental studies. Am. J. Cardiovasc. Dis. 11 (1), 1–11.
Bonvallet, P. P., Damaraju, S. M., Modi, H. N., Stefanelli, V. L., Lin, Q., Saini, S., et al. (2022). Biophysical characterization of a novel tri-layer placental allograft membrane. Adv. Wound Care 11 (2), 43–55. doi:10.1089/wound.2020.1315
Boyar, V., and Galiczewski, C. (2018). Efficacy of dehydrated human amniotic membrane allograft for the treatment of severe extravasation injuries in preterm neonates. Wounds a Compend. Clin. Res. Pract. 30 (8), 224–228.
Chang, B., Ahuja, N., Ma, C., and Liu, X. (2017). Injectable scaffolds: preparation and application in dental and craniofacial regeneration. Mater. Sci. Eng. R Rep. 111, 1–26. doi:10.1016/j.mser.2016.11.001
Chen, J., Wang, M.-W., Xu, J.-J., Wu, X.-Y., and Yao, J. (2020). Gelatin methacryloyl hydrogel eye pad loaded with amniotic extract prevents symblepharon in rabbit eyes. Eur. Rev. Med. Pharmacol. Sci. 24 (19), 10134–10142. doi:10.26355/eurrev_202010_23233
Chen, L., Ye, J., Gao, C., Deng, F., Liu, W., and Zhang, Q. (2023). Design and fabrication of gelatin-based hydrogel loaded with modified amniotic extracellular matrix for enhanced wound healing. Heliyon 9 (10), e20521. doi:10.1016/j.heliyon.2023.e20521
Chen, P., Lu, M., Wang, T., Dian, D., Zhong, Y., and Aleahmad, M. (2021). Human amniotic membrane as a delivery vehicle for stem cell-based therapies. Life Sci. 272, 119157. doi:10.1016/j.lfs.2021.119157
Chen, X., Sun, J., Li, X., Mao, L., Zhou, Y., Cui, L., et al. (2018). Antifibrotic effects of decellularized and lyophilized human amniotic membrane transplant on the formation of intrauterine adhesion. Exp. Clin. Transplant. Official J. Middle East Soc. Organ Transplant. 17 (2), 236–242. doi:10.6002/ect.2017.0284
Cheng, C., Peng, X., Qi, H., Wang, X., Yu, X., Wang, Y., et al. (2021). A promising potential candidate for vascular replacement materials with anti-inflammatory action, good hemocompatibility and endotheliocyte-cytocompatibility: phytic acid-fixed amniotic membrane. Biomed. Mater. 16 (6), 065009. doi:10.1088/1748-605X/ac246d
Chmait, R. H., Kontopoulos, E. V., Chon, A. H., Korst, L. M., Llanes, A., and Quintero, R. A. (2017). Amniopatch treatment of iatrogenic preterm premature rupture of membranes (iPPROM) after fetoscopic laser surgery for twin–twin transfusion syndrome. J. Maternal-Fetal Neonatal Med. 30 (11), 1349–1354. doi:10.1080/14767058.2016.1214123
Choi, C. M., and Jeon, H. S. (2022). Clinical outcomes of in-office sutureless amniotic membrane transplantation in persistent epithelial defect. Korean J. Ophthalmol. KJO. 36 (2), 87–96. doi:10.3341/kjo.2021.0095
Chopra, A., and Thomas, B. S. (2013). Amniotic membrane: a novel material for regeneration and repair. J. Biomim. Biomater. Tissue Eng. 18 (1), 1–8.
Choudhury, D., Tun, H. W., Wang, T., and Naing, M. W. (2018). Organ-derived decellularized extracellular matrix: a game changer for bioink manufacturing? Trends Biotechnol. 36 (8), 787–805. doi:10.1016/j.tibtech.2018.03.003
Chun, B. Y., Kim, H. K., and Shin, J. P. (2013). Dried human amniotic membrane does not alleviate inflammation and fibrosis in experimental strabismus surgery. J. Ophthalmol. 2013, 1–6. doi:10.1155/2013/369126
Comperat, L., Chagot, L., Massot, S., Stachowicz, M. L., Dusserre, N., Médina, C., et al. (2023). Harnessing human placental membrane-derived bioinks: characterization and applications in bioprinting and vasculogenesis. Adv. Healthc. Mater., 2303370. doi:10.1002/adhm.202303370
Cornwell, K. G., Landsman, A., and James, K. S. (2009). Extracellular matrix biomaterials for soft tissue repair. Clin. podiatric Med. Surg. 26 (4), 507–523. doi:10.1016/j.cpm.2009.08.001
Cross, M. J., and Claesson-Welsh, L. (2001). FGF and VEGF function in angiogenesis: signalling pathways, biological responses and therapeutic inhibition. Trends Pharmacol. Sci. 22 (4), 201–207. doi:10.1016/S0165-6147(00)01676-X
Cunningham, B. W., Seiber, B., Riggleman, J. R., Van Horn, M. R., and Bhat, A. (2019). An investigational study of a dual-layer, chorion-free amnion patch as a protective barrier following lumbar laminectomy in a sheep model. J. Tissue Eng. Regen. Med. 13 (9), 1664–1671. doi:10.1002/term.2920
Cunningham, F. G., Leveno, K. J., Bloom, S. L., Spong, C. Y., Dashe, J. S., Hoffman, B. L., et al. (2014). Williams obstetrics. New York: McGraw-Hill Medical.
Dadkhah Tehrani, F., Firouzeh, A., Shabani, I., and Shabani, A. (2021). A review on modifications of amniotic membrane for biomedical applications. Front. Bioeng. Biotechnol. 8, 606982. doi:10.3389/fbioe.2020.606982
Davis, J. (1910). Skin transplantation with a review of 550 cases at the johns hopkins hospital. Johns Hopkins Med. J. 15 (307), 307–398.
Deihim, T., Yazdanpanah, G., and Niknejad, H. (2016). Different light transmittance of placental and reflected regions of human amniotic membrane that could be crucial for corneal tissue engineering. Cornea 35 (7), 997–1003. doi:10.1097/ICO.0000000000000867
de la Torre, P., Paris, J. L., Fernández-de la Torre, M., Vallet-Regí, M., and Flores, A. I. (2021). Endostatin genetically engineered placental mesenchymal stromal cells carrying doxorubicin-loaded mesoporous silica nanoparticles for combined chemo-and antiangiogenic therapy. Pharmaceutics 13 (2), 244. doi:10.3390/pharmaceutics13020244
De Santis, M. M., Alsafadi, H. N., Tas, S., Bölükbas, D. A., Prithiviraj, S., Da Silva, I. A., et al. (2021). Extracellular-matrix-reinforced bioinks for 3D bioprinting human tissue. Adv. Mater. 33 (3), 2005476. doi:10.1002/adma.202005476
Deus, I. A., Santos, S. C., Custódio, C. A., and Mano, J. F. (2022). Designing highly customizable human based platforms for cell culture using proteins from the amniotic membrane. Biomater. Adv. 134, 112574. doi:10.1016/j.msec.2021.112574
Doucette, M., Payne, K. M., Lough, W., Beck, A., Wayment, K., Huffman, J., et al. (2022). Early advanced therapy for diabetic foot ulcers in high amputation risk veterans: a cohort study. Int. J. Low. Extrem. Wounds 21 (2), 111–119. doi:10.1177/1534734620928151
Elkhenany, H., El-Derby, A., Abd Elkodous, M., Salah, R. A., Lotfy, A., and El-Badri, N. (2022). Applications of the amniotic membrane in tissue engineering and regeneration: the hundred-year challenge. Stem Cell Res. Ther. 13 (1), 8–19. doi:10.1186/s13287-021-02684-0
Farhadihosseinabadi, B., Farahani, M., Tayebi, T., Jafari, A., Biniazan, F., Modaresifar, K., et al. (2018). Amniotic membrane and its epithelial and mesenchymal stem cells as an appropriate source for skin tissue engineering and regenerative medicine. Artif. cells, nanomedicine, Biotechnol. 46 (2), 431–440. doi:10.1080/21691401.2018.1458730
Favaron, P. O., Carvalho, R., Borghesi, J., Anunciação, A. R.Ad, and Miglino, M. A. (2015). The amniotic membrane: development and potential applications–a review. Reproduction Domest. animals 50 (6), 881–892. doi:10.1111/rda.12633
Fénelon, M., Catros, S., Meyer, C., Fricain, J.-C., Obert, L., Auber, F., et al. (2021). Applications of human amniotic membrane for tissue engineering. Membranes 11 (6), 387. doi:10.3390/membranes11060387
Fenelon, M., Galvez, P., Kalbermatten, D., Scolozzi, P., and Madduri, S. (2023). Emerging strategies for the biofabrication of multilayer composite amniotic membranes for biomedical applications. Int. J. Mol. Sci. 24 (19), 14424. doi:10.3390/ijms241914424
Fetterolf, D. E., and Snyder, R. J. (2012). Scientific and clinical support for the use of dehydrated amniotic membrane in wound management. Wounds: a compendium of clinical research and practice. Wounds 24 (10), 299–307. PMID: 25876055.
Fitriani, N., Wilar, G., Narsa, A. C., Mohammed, A. F., and Wathoni, N. (2023). Application of amniotic membrane in skin regeneration. Pharmaceutics 15 (3), 748. doi:10.3390/pharmaceutics15030748
Fu, Q., Ohnishi, S., and Sakamoto, N. (2018). Conditioned medium from human amnion-derived mesenchymal stem cells regulates activation of primary hepatic stellate cells. Stem cells Int. 2018, 1–11. doi:10.1155/2018/4898152
Fukuda, K., Chikama, T.-I., Nakamura, M., and Nishida, T. (1999). Differential distribution of subchains of the basement membrane components type IV collagen and laminin among the amniotic membrane, cornea, and conjunctiva. Cornea 18 (1), 73–79. doi:10.1097/00003226-199901000-00013
Gaharwar, A. K., Singh, I., and Khademhosseini, A. (2020). Engineered biomaterials for in situ tissue regeneration. Nat. Rev. Mater. 5 (9), 686–705. doi:10.1038/s41578-020-0209-x
Garrido, M., Escobar, C., Zamora, C., Rejas, C., Varas, J., Córdova, C., et al. (2018). Transplantation of human amniotic membrane over the liver surface reduces hepatic fibrosis in a cholestatic model in young rats. Stem Cells Int. 2018, 1–9. doi:10.1155/2018/6169546
Ghazani, M. A., Soltani, M., Jalali, P., and Hassannejad, R. (2022). A novel numerical and artificial intelligence based approach to study anti-angiogenic drugs: endostatin. Appl. Math. Model. 105, 258–283. doi:10.1016/j.apm.2021.12.033
Gholipourmalekabadi, M., Farhadihosseinabadi, B., Faraji, M., and Nourani, M. R. (2020). How preparation and preservation procedures affect the properties of amniotic membrane? How safe are the procedures? Burns 46 (6), 1254–1271. doi:10.1016/j.burns.2019.07.005
Gicquel, J.-J., Dua, H. S., Brodie, A., Mohammed, I., Suleman, H., Lazutina, E., et al. (2009). Epidermal growth factor variations in amniotic membrane used for ex vivo tissue constructs. Tissue Eng. Part A 15 (8), 1919–1927. doi:10.1089/ten.tea.2008.0432
Gonçalves, J., Tannuri, A., Coelho, M., Bendit, I., and Tannuri, U. (2014). Dynamic expression of desmin, α-SMA and TGF-β1 during hepatic fibrogenesis induced by selective bile duct ligation in young rats. Braz. J. Med. Biol. Res. 47, 850–857. doi:10.1590/1414-431X20143679
Grewal, D. S., and Mahmoud, T. H. (2016). Dehydrated allogenic human amniotic membrane graft for conjunctival surface reconstruction following removal of exposed scleral buckle. Ophthalmic Surg. Lasers Imaging Retina 47 (10), 948–951. doi:10.3928/23258160-20161004-08
Guaní-Guerra, E., Santos-Mendoza, T., Lugo-Reyes, S. O., and Terán, L. M. (2010). Antimicrobial peptides: general overview and clinical implications in human health and disease. Clin. Immunol. 135 (1), 1–11. doi:10.1016/j.clim.2009.12.004
Haghshenas, M., Tavana, S., Zand, E., Montazeri, L., and Fathi, R. (2022). Mouse ovarian follicle growth in an amniotic membrane-based hydrogel. J. Biomaterials Appl. 37 (3), 563–574. doi:10.1177/08853282221094193
Hao, Y., Ma, D. H.-K., Hwang, D. G., Kim, W.-S., and Zhang, F. (2000). Identification of antiangiogenic and antiinflammatory proteins in human amniotic membrane. Cornea 19 (3), 348–352. doi:10.1097/00003226-200005000-00018
Hashimoto, H., Olson, E. N., and Bassel-Duby, R. (2018). Therapeutic approaches for cardiac regeneration and repair. Nat. Rev. Cardiol. 15 (10), 585–600. doi:10.1038/s41569-018-0036-6
Heidari, F., Saadatmand, M., and Simorgh, S. (2023). Directly coaxial bioprinting of 3D vascularized tissue using novel bioink based on decellularized human amniotic membrane. Int. J. Biol. Macromol. 253, 127041. doi:10.1016/j.ijbiomac.2023.127041
Heinegård, D., and Saxne, T. (2011). The role of the cartilage matrix in osteoarthritis. Nat. Rev. Rheumatol. 7 (1), 50–56. doi:10.1038/nrrheum.2010.198
Henry, J. J., Delrosario, L., Fang, J., Wong, S. Y., Fang, Q., Sievers, R., et al. (2020). Development of injectable amniotic membrane matrix for postmyocardial infarction tissue repair. Adv. Healthc. Mater. 9 (2), 1900544. doi:10.1002/adhm.201900544
Hinderland, M. D., and Alan, N. (2012). Revisional tarsal tunnel decompression with Allowrap® DS. Allow. Clin. Rep. Ser., 1–4.
Hofmann, N., Salz, A.-K., Kleinhoff, K., Möhle, N., Börgel, M., Diedenhofen, N., et al. (2021). AmnioClip-plus as sutureless alternative to amniotic membrane transplantation to improve healing of ocular surface disorders. Transplantology 2 (4), 425–432. doi:10.3390/transplantology2040040
Honjo, K.-I., Yamamoto, T., Adachi, T., Amemiya, T., Mazda, O., Kanamura, N., et al. (2015). Evaluation of a dental pulp-derived cell sheet cultured on amniotic membrane substrate. Bio-Medical Mater. Eng. 25 (2), 203–212. doi:10.3233/BME-151270
Hooker, A. B., Lemmers, M., Thurkow, A. L., Heymans, M. W., Opmeer, B. C., Brölmann, H. A., et al. (2014). Systematic review and meta-analysis of intrauterine adhesions after miscarriage: prevalence, risk factors and long-term reproductive outcome. Hum. Reprod. update 20 (2), 262–278. doi:10.1093/humupd/dmt045
Hori, J., Wang, M., Kamiya, K., Takahashi, H., and Sakuragawa, N. (2006). Immunological characteristics of amniotic epithelium. Cornea 25, S53–S58. doi:10.1097/01.ico.0000247214.31757.5c
Horn, A., Saller, J., Cuttica, D., and Neufeld, S. (2019). Review of use of amniotic membrane allograft in total ankle replacements. Foot Ankle Orthop. 4 (4), 2473011419S0022. doi:10.1177/2473011419S00222
Hossain, L., Siddika, A., Adnan, M., Diba, F., Hasan, Z., and Asaduzzaman, S. (2019). Human amniotic membrane and its anti-cancer mechanism: a good hope for cancer therapy. SN Compr. Clin. Med. 1 (7), 487–495. doi:10.1007/s42399-019-00090-5
Hossain, M. L., Rahman, M. A., Siddika, A., Adnan, M., Rahman, H., Diba, F., et al. (2020). Burn and wound healing using radiation sterilized human amniotic membrane and centella asiatica derived gel: a review. Regen. Eng. Transl. Med. 6, 347–357. doi:10.1007/s40883-019-00122-5
Hu, Z., Luo, Y., Ni, R., Hu, Y., Yang, F., Du, T., et al. (2023). Biological importance of human amniotic membrane in tissue engineering and regenerative medicine. Mater. Today Bio 22, 100790. doi:10.1016/j.mtbio.2023.100790
Huang, Y., Bai, L., Yang, Y., Yin, Z., and Guo, B. (2022). Biodegradable gelatin/silver nanoparticle composite cryogel with excellent antibacterial and antibiofilm activity and hemostasis for Pseudomonas aeruginosa-infected burn wound healing. J. Colloid Interface Sci. 608, 2278–2289. doi:10.1016/j.jcis.2021.10.131
Hussin, I., Pingguan-Murphy, B., and Osman, S. (2011). “The fabrication of human amniotic membrane based hydrogel for cartilage tissue engineering applications: a preliminary study,” in 5th Kuala Lumpur International Conference on Biomedical Engineering 2011: (BIOMED 2011), Kuala Lumpur, Malaysia, 20-23 June 2011. (Springer). doi:10.1007/978-3-642-21729-6_205
Islam, M., Karmakar, P. C., Arifuzzaman, M., Karim, N., Akhtar, N., Asaduzzaman, S., et al. (2023). Human amniotic membrane and titanium dioxide nanoparticle derived gel for burn wound healing in a rat model. Regen. Eng. Transl. Med. 9 (2), 249–262. doi:10.1007/s40883-022-00280-z
Jafari, A., Niknejad, H., Rezaei-Tavirani, M., Sarrami-Forooshani, R., Gilanchi, S., and Jafari, Z. (2023). Antiproliferative and apoptotic effects of conditioned medium released from human amniotic epithelial stem cells on breast and cervical cancer cells. Int. J. Immunopathol. Pharmacol. 37, 039463202211507. doi:10.1177/03946320221150712
Jafari, A., Rezaei-Tavirani, M., Farhadihosseinabadi, B., Zali, H., and Niknejad, H. (2021). Human amniotic mesenchymal stem cells to promote/suppress cancer: two sides of the same coin. Stem Cell Res. Ther. 12, 126–211. doi:10.1186/s13287-021-02196-x
Jahanafrooz, Z., Bakhshandeh, B., Behnam Abdollahi, S., and Seyedjafari, E. (2023). Human amniotic membrane as a multifunctional biomaterial: recent advances and applications. J. Biomaterials Appl. 37 (8), 1341–1354. doi:10.1177/08853282221137609
Jhumi, I. J., Arafat, T.-A., Karmakar, P. C., Arifuzzaman, M., Hossain, M. S., Akhtar, N., et al. (2023). Silver nanoparticle incorporated human amniotic membrane gel accelerates second-degree burn wound healing in wister rat. Evidence-Based Complementary Altern. Med. 2023, 1–15. doi:10.1155/2023/9808556
John, T. (2003). Human amniotic membrane transplantation: past, present, and future. Ophthalmol. Clin. N. Am. 16 (1), 43–65. vi. doi:10.1016/s0896-1549(02)00110-4
Johnson, T. D., Lin, S. Y., and Christman, K. L. (2011). Tailoring material properties of a nanofibrous extracellular matrix derived hydrogel. Nanotechnology 22 (49), 494015. doi:10.1088/0957-4484/22/49/494015
Ju, Y., Tang, Z., Dai, X., Gao, H., Zhang, J., Liu, Y., et al. (2022). Protection against light-induced retinal degeneration via dual anti-inflammatory and anti-angiogenic functions of thrombospondin-1. Br. J. Pharmacol. 179 (9), 1938–1961. doi:10.1111/bph.15303
Kafili, G., Kabir, H., Jalali Kandeloos, A., Golafshan, E., Ghasemi, S., Mashayekhan, S., et al. (2023c). Recent advances in soluble decellularized extracellular matrix for heart tissue engineering and organ modeling. J. Biomaterials Appl. 38 (5), 577–604. doi:10.1177/08853282231207216
Kafili, G., Tamjid, E., Niknejad, H., and Simchi, A. (2022). Development of injectable hydrogels based on human amniotic membrane and polyethyleneglycol-modified nanosilicates for tissue engineering applications. Eur. Polym. J. 179, 111566. doi:10.1016/j.eurpolymj.2022.111566
Kafili, G., Tamjid, E., niknejad, H., and Simchi, A. (2023a). Effect of pepsin digestion time on the properties of temperature sensitive human amniotic membrane derived hydrogel. Modares J. Biotechnol. 13 (3), 113–131. Available at: http://biot.modares.ac.ir/article-22-51635-fa.html.
Kafili, G., Tamjid, E., Niknejad, H., and Simchi, A. (2023b). Development of printable nanoengineered composite hydrogels based on human amniotic membrane for wound healing application. J. Mater. Sci. 58, 12351–12372. doi:10.1007/s10853-023-08783-y
Kapasi, K., Albert, S., Yie, S. M., Zavazava, N., and Librach, C. (2000). HLA-G has a concentration-dependent effect on the generation of an allo-CTL response. Immunology 101 (2), 191–200. doi:10.1046/j.1365-2567.2000.00109.x
Khalatbary, A. R., Omraninava, M., Nasiry, D., Akbari, M., Taghiloo, S., Poorhassan, M., et al. (2023). Exosomes derived from human adipose mesenchymal stem cells loaded bioengineered three-dimensional amniotic membrane-scaffold-accelerated diabetic wound healing. Archives Dermatological Res. 315 (10), 2853–2870. doi:10.1007/s00403-023-02709-z
Kim, B. S., Das, S., Jang, J., and Cho, D.-W. (2020). Decellularized extracellular matrix-based bioinks for engineering tissue-and organ-specific microenvironments. Chem. Rev. 120 (19), 10608–10661. doi:10.1021/acs.chemrev.9b00808
Kim, B. S., Kim, H., Gao, G., Jang, J., and Cho, D.-W. (2017). Decellularized extracellular matrix: a step towards the next generation source for bioink manufacturing. Biofabrication 9 (3), 034104. doi:10.1088/1758-5090/aa7e98
Kim, G. H., Uriel, N., and Burkhoff, D. (2018). Reverse remodelling and myocardial recovery in heart failure. Nat. Rev. Cardiol. 15 (2), 83–96. doi:10.1038/nrcardio.2017.139
Kim, H. S., Cho, J. H., Park, H. W., Yoon, H., Kim, M. S., and Kim, S. C. (2002). Endotoxin-neutralizing antimicrobial proteins of the human placenta. J. Immunol. 168 (5), 2356–2364. doi:10.4049/jimmunol.168.5.2356
Kim, J. S., Kim, J. C., Na, B. K., Jeong, J. M., and Song, C. Y. (2000). Amniotic membrane patching promotes healing and inhibits proteinase activity on wound healing following acute corneal alkali burn. Exp. eye Res. 70 (3), 329–337. doi:10.1006/exer.1999.0794
Kim, J.-Y., Lee, S. Y., Park, S.-C., Shin, S. Y., Choi, S. J., Park, Y., et al. (2007). Purification and antimicrobial activity studies of the N-terminal fragment of ubiquitin from human amniotic fluid. Biochimica Biophysica Acta (BBA)-Proteins Proteomics 1774 (9), 1221–1226. doi:10.1016/j.bbapap.2007.06.013
Kim, S. G., Zhou, J., Solomon, C., Zheng, Y., Suzuki, T., Chen, M., et al. (2012). Effects of growth factors on dental stem/progenitor cells. Dent. Clin. N. Am. 56 (3), 563–575. doi:10.1016/j.cden.2012.05.001
King, A., Paltoo, A., Kelly, R., Sallenave, J.-M., Bocking, A., and Challis, J. (2007). Expression of natural antimicrobials by human placenta and fetal membranes. Placenta 28 (2-3), 161–169. doi:10.1016/j.placenta.2006.01.006
Kobayashi, N., Kabuyama, Y., Sasaki, S., Kato, K.-I., and Homma, Y. (2002). Suppression of corneal neovascularization by culture supernatant of human amniotic cells. Cornea 21 (1), 62–67. doi:10.1097/00003226-200201000-00014
Koelink, P. J., Bloemendaal, F. M., Li, B., Westera, L., Vogels, E. W., van Roest, M., et al. (2020). Anti-TNF therapy in IBD exerts its therapeutic effect through macrophage IL-10 signalling. Gut 69 (6), 1053–1063. doi:10.1136/gutjnl-2019-318264
Koizumi, N., Inatomi, T., Sotozono, C., Fullwood, N. J., Quantock, A. J., and Kinoshita, S. (2000). Growth factor mRNA and protein in preserved human amniotic membrane. Curr. eye Res. 20 (3), 173–177. doi:10.1076/0271-3683(200003)2031-9FT173
Kotomin, I., Valtink, M., Hofmann, K., Frenzel, A., Morawietz, H., Werner, C., et al. (2015). Sutureless fixation of amniotic membrane for therapy of ocular surface disorders. PLoS One 10 (5), e0125035. doi:10.1371/journal.pone.0125035
Krafts, K. P. (2010). Tissue repair: the hidden drama. Organogenesis 6 (4), 225–233. doi:10.4161/org.6.4.12555
Kraitchman, D. L., Tatsumi, M., Gilson, W. D., Ishimori, T., Kedziorek, D., Walczak, P., et al. (2005). Dynamic imaging of allogeneic mesenchymal stem cells trafficking to myocardial infarction. Circulation 112 (10), 1451–1461. doi:10.1161/CIRCULATIONAHA.105.537480
Lacorzana, J. (2020). Amniotic membrane, clinical applications and tissue engineering. Review of its ophthalmic use. Arch. Soc. Española Oftalmol. (English Ed). 95 (1), 15–23. doi:10.1016/j.oftale.2019.09.008
Lee, J., Hong, J., Kim, W., and Kim, G. H. (2020). Bone-derived dECM/alginate bioink for fabricating a 3D cell-laden mesh structure for bone tissue engineering. Carbohydr. Polym. 250, 116914. doi:10.1016/j.carbpol.2020.116914
Lee, J. Y., Kim, H., Ha, D. H., Shin, J. C., Kim, A., Ko, H. S., et al. (2018). Amnion-analogous medical device for fetal membrane healing: a preclinical long-term study. Adv. Healthc. Mater. 7 (18), 1800673. doi:10.1002/adhm.201800673
Lei, X., Wu, Y., Peng, X., Zhao, Y., Zhou, X., and Yu, X. (2020). Research on alginate-polyacrylamide enhanced amnion hydrogel, a potential vascular substitute material. Mater. Sci. Eng. C 115, 111145. doi:10.1016/j.msec.2020.111145
Li, H., Niederkorn, J. Y., Neelam, S., Mayhew, E., Word, R. A., McCulley, J. P., et al. (2005). Immunosuppressive factors secreted by human amniotic epithelial cells. Investigative Ophthalmol. Vis. Sci. 46 (3), 900–907. doi:10.1167/iovs.04-0495
Li, X., Li, P., Wang, C., Shang, T., Han, H., Tong, Y., et al. (2022). A thermo-sensitive and injectable hydrogel derived from a decellularized amniotic membrane to prevent intrauterine adhesion by accelerating endometrium regeneration. Biomaterials Sci. 10 (9), 2275–2286. doi:10.1039/D1BM01791H
Lintzeris, D., Yarrow, K., Johnson, L., White, A., Hampton, A., Strickland, A., et al. (2015). Use of a dehydrated amniotic membrane allograft on lower extremity ulcers in patients with challenging wounds: a retrospective case series. Ostomy/wound Manag. 61 (10), 30–36.
Lipový, B., Hladík, M., Štourač, P., and Forostyak, S. (2021). Case report: wound closure acceleration in a patient with toxic epidermal necrolysis using a lyophilised amniotic membrane. Front. Bioeng. Biotechnol. 9, 649317. doi:10.3389/fbioe.2021.649317
Liu, C., Bai, J., Yu, K., Liu, G., Tian, S., and Tian, D. (2019). Biological amnion prevents flexor tendon adhesion in zone II: a controlled, multicentre clinical trial. BioMed Res. Int. 2019, 1–9. doi:10.1155/2019/2354325
Liu, Q.-W., Ying, Y.-M., Zhou, J.-X., Zhang, W.-J., Liu, Z.-X., Jia, B.-B., et al. (2022). Human amniotic mesenchymal stem cells-derived IGFBP-3, DKK-3, and DKK-1 attenuate liver fibrosis through inhibiting hepatic stellate cell activation by blocking Wnt/β-catenin signaling pathway in mice. Stem Cell Res. Ther. 13 (1), 224–318. doi:10.1186/s13287-022-02906-z
Liu, Y. H., Vaghjiani, V., Tee, J. Y., To, K., Cui, P., Oh, D. Y., et al. (2012). Amniotic epithelial cells from the human placenta potently suppress a mouse model of multiple sclerosis. PloS one 7 (4), e35758. doi:10.1371/journal.pone.0035758
Lohajaroensub, R., Sawangmake, C., Rodkhum, C., and Tuntivanich, N. (2022). Expression of antimicrobial peptide genes in the canine amniotic membrane. Veterinary Sci. 9 (5), 200. doi:10.3390/vetsci9050200
Lokhande, G., Carrow, J. K., Thakur, T., Xavier, J. R., Parani, M., Bayless, K. J., et al. (2018). Nanoengineered injectable hydrogels for wound healing application. Acta biomater. 70, 35–47. doi:10.1016/j.actbio.2018.01.045
Luo, Y., Shen, M., Feng, P., Qiu, H., Wu, X., Yang, L., et al. (2021). Various administration forms of decellularized amniotic membrane extract towards improving corneal repair. J. Mater. Chem. B 9 (45), 9347–9357. doi:10.1039/D1TB01848E
Lynn, A., Yannas, I., and Bonfield, W. (2004). Antigenicity and immunogenicity of collagen. J. Biomed. Mater. Res. Part B Appl. Biomaterials 71 (2), 343–354. doi:10.1002/jbm.b.30096
Magatti, M., Caruso, M., De Munari, S., Vertua, E., De, D., Manuelpillai, U., et al. (2015). Human amniotic membrane-derived mesenchymal and epithelial cells exert different effects on monocyte-derived dendritic cell differentiation and function. Cell Transplant. 24 (9), 1733–1752. doi:10.3727/096368914X684033
Magatti, M., Masserdotti, A., Bonassi Signoroni, P., Vertua, E., Stefani, F. R., Silini, A. R., et al. (2020). B lymphocytes as targets of the immunomodulatory properties of human amniotic mesenchymal stromal cells. Front. Immunol. 11, 1156. doi:10.3389/fimmu.2020.01156
Magatti, M., Vertua, E., Cargnoni, A., Silini, A., and Parolini, O. (2018). The immunomodulatory properties of amniotic cells: the two sides of the coin. Cell Transplant. 27 (1), 31–44. doi:10.1177/0963689717742819
Magatti, M., Vertua, E., De Munari, S., Caro, M., Caruso, M., Silini, A., et al. (2017). Human amnion favours tissue repair by inducing the M1-to-M2 switch and enhancing M2 macrophage features. J. tissue Eng. Regen. Med. 11 (10), 2895–2911. doi:10.1002/term.2193
Mamede, A. C., Carvalho, M., Abrantes, A. M., Laranjo, M., Maia, C., and Botelho, M. (2012). Amniotic membrane: from structure and functions to clinical applications. Cell tissue Res. 349, 447–458. doi:10.1007/s00441-012-1424-6
Manuelpillai, U., Tchongue, J., Lourensz, D., Vaghjiani, V., Samuel, C. S., Liu, A., et al. (2010). Transplantation of human amnion epithelial cells reduces hepatic fibrosis in immunocompetent CCl4-treated mice. Cell Transplant. 19 (9), 1157–1168. doi:10.3727/096368910X504496
Mao, Y., Hoffman, T., Dhall, S., Singal, A., Sathyamoorthy, M., Danilkovitch, A., et al. (2019). Endogenous viable cells in lyopreserved amnion retain differentiation potential and anti-fibrotic activity in vitro. Acta Biomater. 94, 330–339. doi:10.1016/j.actbio.2019.06.002
Mao, Y., Hoffman, T., Singh-Varma, A., Duan-Arnold, Y., Moorman, M., Danilkovitch, A., et al. (2017). Antimicrobial peptides secreted from human cryopreserved viable amniotic membrane contribute to its antibacterial activity. Sci. Rep. 7 (1), 13722. doi:10.1038/s41598-017-13310-6
Margiana, R., Markov, A., Zekiy, A. O., Hamza, M. U., Al-Dabbagh, K. A., Al-Zubaidi, S. H., et al. (2022). Clinical application of mesenchymal stem cell in regenerative medicine: a narrative review. Stem Cell Res. Ther. 13 (1), 366–422. doi:10.1186/s13287-022-03054-0
McQuilling, J. P., Sanders, M., Poland, L., Sanders, M., Basadonna, G., Waldrop, N. E., et al. (2019). Dehydrated amnion/chorion improves Achilles tendon repair in a diabetic animal model. Wounds a Compend. Clin. Res. Pract. 31 (1), 19–25.
McQuilling, J. P., Vines, J. B., Kimmerling, K. A., and Mowry, K. C. (2017b). Proteomic comparison of amnion and chorion and evaluation of the effects of processing on placental membranes. Wounds a Compend. Clin. Res. Pract. 29 (6), E36–E40.
McQuilling, J. P., Vines, J. B., and Mowry, K. C. (2017a). In vitro assessment of a novel, hypothermically stored amniotic membrane for use in a chronic wound environment. Int. wound J. 14 (6), 993–1005. doi:10.1111/iwj.12748
Mohan, R., Bajaj, A., and Gundappa, M. (2017). Human amnion membrane: potential applications in oral and periodontal field. J. Int. Soc. Prev. Community Dent. 7 (1), 15. doi:10.4103/jispcd.JISPCD_359_16
Moussa, D. G., and Aparicio, C. (2019). Present and future of tissue engineering scaffolds for dentin-pulp complex regeneration. J. tissue Eng. Regen. Med. 13 (1), 58–75. doi:10.1002/term.2769
Munoz-Torres, J. R., Martínez-González, S. B., Lozano-Luján, A. D., Martínez-Vázquez, M. C., Velasco-Elizondo, P., Garza-Veloz, I., et al. (2023). Biological properties and surgical applications of the human amniotic membrane. Front. Bioeng. Biotechnol. 10, 1067480. doi:10.3389/fbioe.2022.1067480
Murphy, M. B., Moncivais, K., and Caplan, A. I. (2013). Mesenchymal stem cells: environmentally responsive therapeutics for regenerative medicine. Exp. Mol. Med. 45 (11), e54. doi:10.1038/emm.2013.94
Murphy, S. V., Skardal, A., Nelson, R. A., Sunnon, K., Reid, T., Clouse, C., et al. (2020). Amnion membrane hydrogel and amnion membrane powder accelerate wound healing in a full thickness porcine skin wound model. Stem cells Transl. Med. 9 (1), 80–92. doi:10.1002/sctm.19-0101
Murphy, S. V., Skardal, A., Song, L., Sutton, K., Haug, R., Mack, D. L., et al. (2017). Solubilized amnion membrane hyaluronic acid hydrogel accelerates full-thickness wound healing. Stem cells Transl. Med. 6 (11), 2020–2032. doi:10.1002/sctm.17-0053
Murri, M. S., Moshirfar, M., Birdsong, O. C., Ronquillo, Y. C., Ding, Y., and Hoopes, P. C. (2018). Amniotic membrane extract and eye drops: a review of literature and clinical application. Clin. Ophthalmol. 12, 1105–1112. doi:10.2147/OPTH.S165553
Nagaoka, H., Nagaoka, H., Walter, R., Boushell, L. W., Miguez, P. A., Burton, A., et al. (2014). Characterization of genipin-modified dentin collagen. BioMed Res. Int. 2014, 1–7. doi:10.1155/2014/702821
Nasiry, D., Khalatbary, A. R., Abdollahifar, M.-A., Amini, A., Bayat, M., Noori, A., et al. (2021). Engraftment of bioengineered three-dimensional scaffold from human amniotic membrane-derived extracellular matrix accelerates ischemic diabetic wound healing. Archives Dermatological Res. 313 (7), 567–582. doi:10.1007/s00403-020-02137-3
Nasiry, D., Khalatbary, A. R., Abdollahifar, M.-A., Bayat, M., Amini, A., Ashtiani, M. K., et al. (2022). SDF-1α loaded bioengineered human amniotic membrane-derived scaffold transplantation in combination with hyperbaric oxygen improved diabetic wound healing. J. Biosci. Bioeng. 133 (5), 489–501. doi:10.1016/j.jbiosc.2022.01.012
Nasiry, D., Khalatbary, A. R., Noori, A., Abouhamzeh, B., and Jamalpoor, Z. (2023). Accelerated wound healing using three-dimensional amniotic membrane scaffold in combination with adipose-derived stem cells in a diabetic rat model. Tissue Cell 82, 102098. doi:10.1016/j.tice.2023.102098
Naxer, S., Horn, M., and Schittkowski, M. (2018). Processed amniotic membrane for conjunctival reconstruction in complex strabismus surgery. Strabismus 26 (4), 191–197. doi:10.1080/09273972.2018.1502794
Nazanin, M., Mahshad, M., Mehdi, B., and Farzaneh, C. (2022). Enhanced survival and accelerated perfusion of skin flap to recipient site following administration of human amniotic membrane in rat models. J. Plastic, Reconstr. Aesthetic Surg. 75 (11), 4321–4327. doi:10.1016/j.bjps.2022.08.028
Nejad, A. R., Hamidieh, A. A., Amirkhani, M. A., and Sisakht, M. M. (2021). Update review on five top clinical applications of human amniotic membrane in regenerative medicine. Placenta 103, 104–119. doi:10.1016/j.placenta.2020.10.026
Newman, J. H., Rich, S., Abman, S. H., Alexander, J. H., Barnard, J., Beck, G. J., et al. (2017). Enhancing insights into pulmonary vascular disease through a precision medicine approach. A joint NHLBI–Cardiovascular Medical Research and Education Fund Workshop Report. Am. J. Respir. Crit. care Med. 195 (12), 1661–1670. doi:10.1164/rccm.201701-0150WS
Ni, J., Abrahamson, M., Zhang, M., Fernandez, M. A., Grubb, A., Su, J., et al. (1997). Cystatin E is a novel human cysteine proteinase inhibitor with structural resemblance to family 2 cystatins. J. Biol. Chem. 272 (16), 10853–10858. doi:10.1074/jbc.272.16.10853
Niknejad, H., Paeini-Vayghan, G., Tehrani, F., Khayat-Khoei, M., and Peirovi, H. (2013). Side dependent effects of the human amnion on angiogenesis. Placenta 34 (4), 340–345. doi:10.1016/j.placenta.2013.02.001
Niknejad, H., Peirovi, H., Jorjani, M., Ahmadiani, A., Ghanavi, J., and Seifalian, A. M. (2008). Properties of the amniotic membrane for potential use in tissue engineering. Eur. Cells Mater 15, 88–99. doi:10.22203/ecm.v015a07
Niknejad, H., and Yazdanpanah, G. (2014). Opposing effect of amniotic membrane on angiogenesis originating from amniotic epithelial cells. J. Med. Hypotheses Ideas 8 (1), 39–41. doi:10.1016/j.jmhi.2013.08.002
Nilforoushzadeh, M. A., Amirkhani, M. A., Hamidieh, A. A., Seifalian, A. M., and Sisakht, M. M. (2019). Skin regenerative medicine advancements in the Islamic Republic of Iran: a concise review. Regen. Med. 14 (11), 1047–1056. doi:10.2217/rme-2018-0170
Nouri, M., Ebrahimi, M., Bagheri, T., Fatemi, M. J., Najafbeygi, A., Araghi, S., et al. (2018). Healing effects of dried and acellular human amniotic membrane and mepitelas for coverage of skin graft donor areas; a randomized clinical trial. Bull. Emerg. Trauma 6 (3), 195–200. doi:10.29252/beat-060302
Ohno, M., Martinez-Hernandez, A., Ohno, N., and Kefalides, N. A. (1983). Isolation of laminin from human placental basement membranes: amnion, chorion and chorionic microvessels. Biochem. Biophysical Res. Commun. 112 (3), 1091–1098. doi:10.1016/0006-291X(83)91730-8
Parolini, O., Alviano, F., Bagnara, G. P., Bilic, G., Bühring, H.-J., Evangelista, M., et al. (2008). Concise review: isolation and characterization of cells from human term placenta: outcome of the first international Workshop on Placenta Derived Stem Cells. Stem cells 26 (2), 300–311. doi:10.1634/stemcells.2007-0594
Parolini, O., Soncini, M., Evangelista, M., and Schmidt, D. (2009). Amniotic membrane and amniotic fluid-derived cells: potential tools for regenerative medicine? Regen. Med. 4, 275–291. doi:10.2217/17460751.4.2.275
Peng, X., Wang, X., Cheng, C., Zhou, X., Gu, Z., Li, L., et al. (2020). Bioinspired, artificial, small-diameter vascular grafts with selective and rapid endothelialization based on an amniotic membrane-derived hydrogel. ACS Biomaterials Sci. Eng. 6 (3), 1603–1613. doi:10.1021/acsbiomaterials.9b01493
Phinney, D. G., and Prockop, D. J. (2007). Concise review: mesenchymal stem/multipotent stromal cells: the state of transdifferentiation and modes of tissue repair—current views. Stem cells 25 (11), 2896–2902. doi:10.1634/stemcells.2007-0637
Pober, J. S., and Sessa, W. C. (2015). Inflammation and the blood microvascular system. Cold Spring Harb. Perspect. Biol. 7 (1), a016345. doi:10.1101/cshperspect.a016345
Pogozhykh, O., Hofmann, N., Gryshkov, O., von Kaisenberg, C., Mueller, M., Glasmacher, B., et al. (2020). Repeated freezing procedures preserve structural and functional properties of amniotic membrane for application in ophthalmology. Int. J. Mol. Sci. 21 (11), 4029. doi:10.3390/ijms21114029
Poss, K. D. (2010). Advances in understanding tissue regenerative capacity and mechanisms in animals. Nat. Rev. Genet. 11 (10), 710–722. doi:10.1038/nrg2879
Pourali, P., and Yahyaei, B. (2016). Biological production of silver nanoparticles by soil isolated bacteria and preliminary study of their cytotoxicity and cutaneous wound healing efficiency in rat. J. Trace Elem. Med. Biol. 34, 22–31. doi:10.1016/j.jtemb.2015.11.004
Price, C. A. (2016). Mechanisms of fibroblast growth factor signaling in the ovarian follicle. J. Endocrinol. 228 (2), R31–R43. doi:10.1530/joe-15-0414
Radke, D., Jia, W., Sharma, D., Fena, K., Wang, G., Goldman, J., et al. (2018). Tissue engineering at the blood-contacting surface: a review of challenges and strategies in vascular graft development. Adv. Healthc. Mater. 7 (15), 1701461. doi:10.1002/adhm.201701461
Rahman, M. S., Islam, R., Rana, M. M., Spitzhorn, L.-S., Rahman, M. S., Adjaye, J., et al. (2019). Characterization of burn wound healing gel prepared from human amniotic membrane and Aloe vera extract. BMC Complementary Altern. Med. 19, 115–15. doi:10.1186/s12906-019-2525-5
Rana, M. M., Rahman, M. S., Ullah, M. A., Siddika, A., Hossain, M. L., Akhter, M. S., et al. (2020). Amnion and collagen-based blended hydrogel improves burn healing efficacy on a rat skin wound model in the presence of wound dressing biomembrane. Bio-Medical Mater. Eng. 31 (1), 1–17. doi:10.3233/BME-201076
R Mohan, R., Ck Tovey, J., Gupta, R., Sharma, A., and Tandon, A. (2011). Decorin biology, expression, function and therapy in the cornea. Curr. Mol. Med. 11 (2), 110–128. doi:10.2174/156652411794859241
Rosenblum, B. I. (2016). A retrospective case series of a dehydrated amniotic membrane allograft for treatment of unresolved diabetic foot ulcers. J. Am. Podiatric Med. Assoc. 106 (5), 328–337. doi:10.7547/15-139
Ryzhuk, V., Zeng, X.-X., Wang, X., Melnychuk, V., Lankford, L., Farmer, D., et al. (2018). Human amnion extracellular matrix derived bioactive hydrogel for cell delivery and tissue engineering. Mater. Sci. Eng. C 85, 191–202. doi:10.1016/j.msec.2017.12.026
Salah, R. A., Elkhenany, H., and El-Badri, N. (2020). Scaffold engineering using the amniotic membrane. Regen. Med. stem Cell Biol., 323–346. doi:10.1007/978-3-030-55359-3_11
Saldin, L. T., Cramer, M. C., Velankar, S. S., White, L. J., and Badylak, S. F. (2017). Extracellular matrix hydrogels from decellularized tissues: structure and function. Acta biomater. 49, 1–15. doi:10.1016/j.actbio.2016.11.068
Samaniego, A. (2011). Allowrap® surgical barrier remains in the body through the cell proliferation phase of the healing process while other surgical barriers are resorbed. AlloSource White Pap.
Sant'Anna, L. B., Hage, R., Cardoso, M. A. G., Arisawa, E. A., Cruz, M. M., Parolini, O., et al. (2016). Antifibrotic effects of human amniotic membrane transplantation in established biliary fibrosis induced in rats. Cell Transplant. 25 (12), 2245–2257. doi:10.3727/096368916X692645
Sasikumar, S., Chameettachal, S., Cromer, B., Pati, F., and Kingshott, P. (2019). Decellularized extracellular matrix hydrogels—cell behavior as a function of matrix stiffness. Curr. Opin. Biomed. Eng. 10, 123–133. doi:10.1016/j.cobme.2019.05.002
Schmiedova, I., Ozanova, Z., Stastna, E., Kiselakova, L., Lipovy, B., and Forostyak, S. (2021). Case report: freeze-dried human amniotic membrane allograft for the treatment of chronic wounds: results of a multicentre observational study. Front. Bioeng. Biotechnol. 9, 649446. doi:10.3389/fbioe.2021.649446
Selmani, Z., Naji, A., Zidi, I., Favier, B., Gaiffe, E., Obert, L., et al. (2008). Human leukocyte antigen-G5 secretion by human mesenchymal stem cells is required to suppress T lymphocyte and natural killer function and to induce CD4+ CD25highFOXP3+ regulatory T cells. Stem cells 26 (1), 212–222. doi:10.1634/stemcells.2007-0554
Serena, T. E., Yaakov, R., Moore, S., Cole, W., Coe, S., Snyder, R., et al. (2020). A randomized controlled clinical trial of a hypothermically stored amniotic membrane for use in diabetic foot ulcers. J. Comp. Eff. Res. 9 (1), 23–34. doi:10.2217/cer-2019-0142
Shao, C., Sima, J., Zhang, S. X., Jin, J., Reinach, P., Wang, Z., et al. (2004). Suppression of corneal neovascularization by PEDF release from human amniotic membranes. Investigative Ophthalmol. Vis. Sci. 45 (6), 1758–1762. doi:10.1167/iovs.03-0882
Shariatzadeh, S., Shafiee, S., Zafari, A., Tayebi, T., Yazdanpanah, G., Majd, A., et al. (2021). Developing a pro-angiogenic placenta derived amniochorionic scaffold with two exposed basement membranes as substrates for cultivating endothelial cells. Sci. Rep. 11 (1), 22508. doi:10.1038/s41598-021-01922-y
Shimazaki, J., Shimmura, S., and Tsubota, K. (2004). Donor source affects the outcome of ocular surface reconstruction in chemical or thermal burns of the cornea11The authors do not have any proprietary interest in the products mentioned used in this study. Ophthalmology 111 (1), 38–44. doi:10.1016/j.ophtha.2003.02.003
Shimmura, S., Shimazaki, J., Ohashi, Y., and Tsubota, K. (2001). Antiinflammatory effects of amniotic membrane transplantation in ocular surface disorders. Cornea 20 (4), 408–413. doi:10.1097/00003226-200105000-00015
Silini, A. R., Cargnoni, A., Magatti, M., Pianta, S., and Parolini, O. (2015). The long path of human placenta, and its derivatives, in regenerative medicine. Front. Bioeng. Biotechnol. 3, 162. doi:10.3389/fbioe.2015.00162
Silini, A. R., Magatti, M., Cargnoni, A., and Parolini, O. (2017). Is immune modulation the mechanism underlying the beneficial effects of amniotic cells and their derivatives in regenerative medicine? Cell Transplant. 26 (4), 531–539. doi:10.3727/096368916X693699
Slaughter, B. V., Khurshid, S. S., Fisher, O. Z., Khademhosseini, A., and Peppas, N. A. (2009). Hydrogels in regenerative medicine. Adv. Mater. 21 (32-33), 3307–3329. doi:10.1002/adma.200802106
Snyder, R. J., Shimozaki, K., Tallis, A., Kerzner, M., Reyzelman, A., Lintzeris, D., et al. (2016). A prospective, randomized, multicenter, controlled evaluation of the use of dehydrated amniotic membrane allograft compared to standard of care for the closure of chronic diabetic foot ulcer. Wounds: a compendium of clinical research and practice. Wounds. 28 (3), 70–77.
Srinivasan, R. C., Strom, S. C., and Gramignoli, R. (2020). Effects of cryogenic storage on human amnion epithelial cells. Cells 9 (7), 1696. doi:10.3390/cells9071696
Stern, M. (1913). The grafting of preserved amniotic membrane to burned and ulcerated surfaces, substituing skin grafts: a preliminary report. J. Am. Med. Assoc. 60 (13), 973–974. doi:10.1001/jama.1913.04340130021008
Swim, M. M., Albertario, A., Iacobazzi, D., Caputo, M., and Ghorbel, M. T. (2019). Amnion-based scaffold with enhanced strength and biocompatibility for in vivo vascular repair. Tissue Eng. Part A 25 (7-8), 603–619. doi:10.1089/ten.tea.2018.0175
Tallquist, M. D., and Molkentin, J. D. (2017). Redefining the identity of cardiac fibroblasts. Nat. Rev. Cardiol. 14 (8), 484–491. doi:10.1038/nrcardio.2017.57
Tan, J. L., Chan, S. T., Wallace, E. M., and Lim, R. (2014). Human amnion epithelial cells mediate lung repair by directly modulating macrophage recruitment and polarization. Cell Transplant. 23 (3), 319–328. doi:10.3727/096368912X661409
Tehrani, F. A., Ahmadiani, A., and Niknejad, H. (2013). The effects of preservation procedures on antibacterial property of amniotic membrane. Cryobiology 67 (3), 293–298. doi:10.1016/j.cryobiol.2013.08.010
Tehrani, F. A., Modaresifar, K., Azizian, S., and Niknejad, H. (2017). Induction of antimicrobial peptides secretion by IL-1β enhances human amniotic membrane for regenerative medicine. Sci. Rep. 7 (1), 17022–17027. doi:10.1038/s41598-017-17210-7
Thompson, P., Hanson, D. S., Langemo, D., and Anderson, J. (2019). Comparing human amniotic allograft and standard wound care when using total contact casting in the treatment of patients with diabetic foot ulcers. Adv. Skin Wound Care 32 (6), 272–277. doi:10.1097/01.ASW.0000557831.78645.85
Toda, A., Okabe, M., Yoshida, T., and Nikaido, T. (2007). The potential of amniotic membrane/amnion-derived cells for regeneration of various tissues. J. Pharmacol. Sci. 105 (3), 215–228. doi:10.1254/jphs.CR0070034
Tohidi, H., Maleki-Jirsaraei, N., Simchi, A., Mohandes, F., Emami, Z., Fassina, L., et al. (2022). An electroconductive, thermosensitive, and injectable chitosan/pluronic/gold-decorated cellulose nanofiber hydrogel as an efficient carrier for regeneration of cardiac tissue. Materials 15 (15), 5122. doi:10.3390/ma15155122
Toniato, T. V., Stocco, T. D., Martins, D. S., Santanna, L. B., Tim, C. R., Marciano, F. R., et al. (2020). Hybrid chitosan/amniotic membrane-based hydrogels for articular cartilage tissue engineering application. Int. J. Polym. Mater. Polym. Biomaterials 69 (15), 961–970. doi:10.1080/00914037.2019.1636249
Torricelli, A. A., Santhanam, A., Wu, J., Singh, V., and Wilson, S. E. (2016). The corneal fibrosis response to epithelial–stromal injury. Exp. eye Res. 142, 110–118. doi:10.1016/j.exer.2014.09.012
Tsai, G. L., Zilberbrand, D., Liao, W. J., and Horl, L. P. (2021). Healing hard-to-heal diabetic foot ulcers: the role of dehydrated amniotic allograft with cross-linked bovine-tendon collagen and glycosaminoglycan matrix. J. Wound Care 30 (7), S47–S53. doi:10.12968/jowc.2021.30.Sup7.S47
Tsai, S.-H., Liu, Y.-W., Tang, W.-C., Zhou, Z.-W., Hwang, C.-Y., Hwang, G.-Y., et al. (2007). Characterization of porcine arterial endothelial cells cultured on amniotic membrane, a potential matrix for vascular tissue engineering. Biochem. biophysical Res. Commun. 357 (4), 984–990. doi:10.1016/j.bbrc.2007.04.047
Výborný, K., Vallová, J., Kočí, Z., Kekulová, K., Jiráková, K., Jendelová, P., et al. (2019). Genipin and EDC crosslinking of extracellular matrix hydrogel derived from human umbilical cord for neural tissue repair. Sci. Rep. 9 (1), 10674. doi:10.1038/s41598-019-47059-x
Walkden, A. (2020). Amniotic membrane transplantation in ophthalmology: an updated perspective. Clin. Ophthalmol. 14, 2057–2072. doi:10.2147/OPTH.S208008
Wang, B., Wang, X., Kenneth, A., Drena, A., Pacheco, A., Kalvin, L., et al. (2023). Developing small-diameter vascular grafts with human amniotic membrane: long-term evaluation of transplantation outcomes in a small animal model. Biofabrication 15 (2), 025004. doi:10.1088/1758-5090/acb1da
Wassmer, C.-H., and Berishvili, E. (2020). Immunomodulatory properties of amniotic membrane derivatives and their potential in regenerative medicine. Curr. diabetes Rep. 20, 31–10. doi:10.1007/s11892-020-01316-w
Wehmeyer, J. L., Natesan, S., and Christy, R. J. (2015). Development of a sterile amniotic membrane tissue graft using supercritical carbon dioxide. Tissue Eng. Part C. Methods 21 (7), 649–659. doi:10.1089/ten.tec.2014.0304
Yadav, M. K., Go, Y. Y., Kim, S. H., Chae, S.-W., and Song, J.-J. (2017). Antimicrobial and antibiofilm effects of human amniotic/chorionic membrane extract on Streptococcus pneumoniae. Front. Microbiol. 8, 1948. doi:10.3389/fmicb.2017.01948
Yang, J., Khan, M., Zhang, L., Ren, X., Guo, J., Feng, Y., et al. (2015). Antimicrobial surfaces grafted random copolymers with REDV peptide beneficial for endothelialization. J. Mater. Chem. B 3 (39), 7682–7697. doi:10.1039/C5TB01155H
Yazdanpanah, G., Jiang, Y., Rabiee, B., Omidi, M., Rosenblatt, M. I., Shokuhfar, T., et al. (2021). Fabrication, rheological, and compositional characterization of thermoresponsive hydrogel from cornea. Tissue Eng. Part C. Methods 27 (5), 307–321. doi:10.1089/ten.tec.2021.0011
Yazdanpanah, G., Paeini-Vayghan, G., Asadi, S., and Niknejad, H. (2015). The effects of cryopreservation on angiogenesis modulation activity of human amniotic membrane. Cryobiology 71 (3), 413–418. doi:10.1016/j.cryobiol.2015.09.008
Yuan, Z., Nie, H., Wang, S., Lee, C. H., Li, A., Fu, S. Y., et al. (2011). Biomaterial selection for tooth regeneration. Tissue Eng. Part B Rev. 17 (5), 373–388. doi:10.1089/ten.teb.2011.0041
Zare-Bidaki, M., Sadrinia, S., Erfani, S., Afkar, E., and Ghanbarzade, N. (2017). Antimicrobial properties of amniotic and chorionic membranes: a comparative study of two human fetal sacs. J. reproduction Infertil. 18 (2), 218–224. PMCID: PMC5565909.
Zasloff, M. (2002). Antimicrobial peptides of multicellular organisms. nature 415 (6870), 389–395. doi:10.1038/415389a
Zelen, C. M., Gould, L., Serena, T. E., Carter, M. J., Keller, J., and Li, W. W. (2015). A prospective, randomised, controlled, multi-centre comparative effectiveness study of healing using dehydrated human amnion/chorion membrane allograft, bioengineered skin substitute or standard of care for treatment of chronic lower extremity diabetic ulcers. Int. wound J. 12 (6), 724–732. doi:10.1111/iwj.12395
Zelen, C. M., Serena, T. E., Gould, L., Le, L., Carter, M. J., Keller, J., et al. (2016). Treatment of chronic diabetic lower extremity ulcers with advanced therapies: a prospective, randomised, controlled, multi-centre comparative study examining clinical efficacy and cost. Int. wound J. 13 (2), 272–282. doi:10.1111/iwj.12566
Zhang, Q., Chang, C., Qian, C., Xiao, W., Zhu, H., Guo, J., et al. (2021). Photo-crosslinkable amniotic membrane hydrogel for skin defect healing. Acta Biomater. 125, 197–207. doi:10.1016/j.actbio.2021.02.043
Zhang, Y., Fan, W., Wang, K., Wei, H., Zhang, R., and Wu, Y. (2019). Novel preparation of Au nanoparticles loaded Laponite nanoparticles/ECM injectable hydrogel on cardiac differentiation of resident cardiac stem cells to cardiomyocytes. J. Photochem. Photobiol. B Biol. 192, 49–54. doi:10.1016/j.jphotobiol.2018.12.022
Zhou, S., Chen, J., and Feng, J. (2003). The effects of amniotic membrane on polymorphonuclear cells. Chin. Med. J. 116 (05), 788–790. doi:10.3760/cma.j.issn.0366-6999.2003.05.136
Zhou, Y., Liang, K., Zhao, S., Zhang, C., Li, J., Yang, H., et al. (2018). Photopolymerized maleilated chitosan/methacrylated silk fibroin micro/nanocomposite hydrogels as potential scaffolds for cartilage tissue engineering. Int. J. Biol. Macromol. 108, 383–390. doi:10.1016/j.ijbiomac.2017.12.032
Glossary
Keywords: human-derived materials, decellularized extracellular matrix, injectable hydrogels, 3D bioprinting, nanomedicine, safety
Citation: Kafili G, Niknejad H, Tamjid E and Simchi A (2024) Amnion-derived hydrogels as a versatile platform for regenerative therapy: from lab to market. Front. Bioeng. Biotechnol. 12:1358977. doi: 10.3389/fbioe.2024.1358977
Received: 20 December 2023; Accepted: 09 February 2024;
Published: 26 February 2024.
Edited by:
Nahum Rosenberg, National Insurance Institute of Israel, IsraelReviewed by:
Forough Shams, Shahid Beheshti University of Medical Sciences, IranNicola Hofmann, German Society for Tissue Transplantation (DGFG), Germany
Peng Xu, Shanghai Jiao Tong University, China
Copyright © 2024 Kafili, Niknejad, Tamjid and Simchi. This is an open-access article distributed under the terms of the Creative Commons Attribution License (CC BY). The use, distribution or reproduction in other forums is permitted, provided the original author(s) and the copyright owner(s) are credited and that the original publication in this journal is cited, in accordance with accepted academic practice. No use, distribution or reproduction is permitted which does not comply with these terms.
*Correspondence: Abdolreza Simchi, c2ltY2hpQHNoYXJpZi5lZHU=; Golara Kafili, Z19rYWZpbGlAeWFob28uY29t