- 1Indian Institute of Science (IISc), Bangalore, India
- 2Nelson Mandela African Institution of Science and Technology, Arusha, Tanzania
- 3Chemical Engineering Department, Federal University of Technology, Minna, Nigeria
Bio-printed hydrogels have evolved as one of the best regenerative medicine and tissue engineering platforms due to their outstanding cell-friendly microenvironment. A correct hydrogel ink formulation is critical for creating desired scaffolds that have better fidelity after printing. Gelatin and its derivatives have sparked intense interest in various biomedical sectors because of their biocompatibility, biodegradability, ease of functionalization, and rapid gelling tendency. As a result, this report emphasizes the relevance of gelatin-based hydrogel in fabricating bio-printed scaffolds for orthopedic applications. Starting with what hydrogels and bio-printing are all about. We further summarized the different gelatin-based bio-printing techniques explored for orthopedic applications, including a few recent studies. We also discussed the suitability of gelatin as a biopolymer for both 3D and 4D printing materials. As extrusion is one of the most widely used techniques for bio-printing gelatin-based, we summarize the rheological features of gelatin-based bio-ink. Lastly, we also elaborate on the recent bio-printed gelatin-based studies for orthopedics applications, the potential clinical translation issues, and research possibilities.
1 Introduction
Restoring a significant large bone or cartilage defect caused by illnesses, accidents, or trauma is one of the most challenging tasks in orthopedic therapeutic settings (Agarwal et al., 2021; Hu et al., 2024; Huang et al., 2024; Martins et al., 2024). While the regeneration of orthopedic tissue is a gradual process, it is also a complicated one that necessitates a wide range of abilities and knowledge. In the past, despite numerous attempts to resolve these difficulties. However, the present success is very little. The existing techniques have several disadvantages, including sluggish bio-integration and a significant risk of infection (Moriarty et al., 2019; Hou et al., 2024; Wu et al., 2024). Providing a close and comparable 3D milieu to natural tissue is critical to repairing faulty bone and cartilage, along with significant cell attachment, proliferation, differentiation, and the required bioactive component (Sood et al., 2021). Bioprinting has lately evolved as the best method for producing patient-specific cell-laden scaffolds for tissue regeneration, such as bone and cartilage. Bio-printing poses a significant edge to traditional fabricating approaches regarding scaffold geometry control, scaffold dimension specificity to properly match the intended place, 3D scaffold microstructures environment, and encapsulated cells’ good spatiotemporal dispersion (Sun et al., 2020; Cheng et al., 2021).
The classifications of 3D printing techniques in tissue engineering applications include 1) cell seeding on an already printed porous volume for cell adherence and proliferation to achieve an engineered tissue and 2) mixing cells with a bio-friendly ink before 3D printing to achieve better interactions and control of cells with their microenvironment. However, different strategies have distinct benefits and drawbacks. Among the bioprinting methods, the extrusion-based process of bioprinting stands out due to its cost-effectiveness, simplicity, and scalability, and thus, it is the most often employed (Cheng Y. et al., 2019; Chen et al., 2023). Appropriate rheological qualities, such as shear thinning behavior and thixotropy properties, are critical with this approach because they govern the extruding manner via a nozzle under pressure and the ability to preserve the intended form post-deposition (Li et al., 2019). As a result, it is critical to engineer a functional hydrogel ink with desired rheological and mechanical characteristics (Gopinathan and Noh, 2018; Gao et al., 2019). Furthermore, the bio-inks must be biocompatible, biodegradable, and tissue-specific without adversely affecting the encapsulated cells and adjacent tissues.
Hydrogels are a class of 3D network polymers with enormous capacity to expand and retain a high-water quantity. Recently, hydrogels have emerged as the most widely used material to make 3D-printed constructs for various tissue engineering applications. Painfully, hydrogels’ poor degradation rate and weak mechanical characteristics restrict their use as bone tissue engineering (BTE) biopolymers. Gelatin is an intriguing biomaterial for hydrogel formulation among natural polymers because of its gelation capabilities (Cheng et al., 2018; Choi et al., 2018; Yan et al., 2018; Nabavinia et al., 2019). As a low-cost polymer, gelatin offers good biomaterial features such as biodegradability, biocompatibility, cell adherence, and proliferation. In addition, gelatin is generally produced by mild hydrolysis of collagen (Saravanan et al., 2017; Hasan et al., 2018; Kim et al., 2018). It has also been used with other biomaterials to increase cell adhesion (Piao and Chen, 2017). Gelatin possesses exceptional biological properties due to the RGD (Arg-Gly-Asp) sequence, making it a superior choice for BTE to other biopolymers. Furthermore, it stimulates osteoclasts, contributing to enhanced osteogenesis (Kruppke et al., 2020; Wu et al., 2020). Despite its numerous biomaterial features, gelatin’s mechanical qualities and rapid breakdown restrict its application as a hard tissue engineering material (Hasan et al., 2018; Nabavinia et al., 2019). Adding reinforcing components to form a composite material is a valuable method for improving the characteristics of materials. Several researchers have recently published various research articles on bio-nanocomposites for orthopedics applications. These bio-nanocomposite bone replacements usually include granules, powders, porous or solid constructs, and bioactive metal prosthesis coatings (Yekta et al., 2018; Shuai et al., 2018; Shuai et al., 2019a; Shuai et al., 2019b; Kordjamshidi et al., 2019; Saber-Samandari et al., 2019). Various reinforcing materials have been explored in the past, which includes hydroxyapatite (HAp) (Nabavinia et al., 2019), graphene, and carbon nanotubes (Piao and Chen, 2017; Yan et al., 2018).
More than 500 papers have been published for cartilage tissue bioprinting (ref. Scopus search 3D Bio-printing, cartilage) (Figure 2A) and more than 800 for bone (ref. Scopus search 3D Bio-printing, Bone) (Figure 2B). Moreover, half of those publications were reported in the recent 3 years, indicating that it is an active area of research. Gelatin and its derivatives have received a lot of interest among these published studies because of its simplicity of synthesis at a cheap cost, acceptable biocompatibility, transparent structure for cell monitoring, photo-crosslink ability, and customizable physical and chemical characteristics (Xiao et al., 2019). However, few review papers on gelatin-based 3D bioprinting have been published thus far (Wang et al., 2017; Ying et al., 2018). To our knowledge, these review papers only cover a small portion of gelatin derivatives and applications, with no mention of orthopedic uses. Therefore, this review article summarized the most recent advances in bioprinted gelatin-based formulations for orthopedic repair. The interconnectivity summary between the gelatin-based formulations, printability, and biological functions of orthopedic cells. Gelatin-based bio-printing presents status and critical challenges summary to achieve more effective clinical translation. Figure 1 shows the trends for bioprinting of (a) BTE and (b) Cartilage tissue engineering (CTE).
2 3D bioprinting methods for gelatin-based inks
Micro molding, the most common method of creating gelatin-based 3D structures, fosters diverse cell activities. However, the bioprinting method has taken over due to the possibility of introducing typical complexity of 3D design, resolution, and spatial control over the fabricating process (Hölzl et al., 2016; Alhaskawi et al., 2024; Bhardwaj et al., 2024). The viscosity of bio-ink is a crucial aspect to consider in bio-printing approaches, particularly extrusion-based techniques (Catros et al., 2011). Pure gelatin bioprinting is difficult due to its intrinsic temperature sensitivity and poor viscosity at ambient temperature or higher. As a result, it could not meet the criterion for various tissues. Because of the various chemical and mechanical characteristics linked with different tissues and to mimic tissue ECM in nature, gelatin-based bio-ink has primarily been used in a modified form. Some of these alterations include concentration optimizations, functionalization with other molecules, and the insertion of second phases into the gelatin matrix. These parameters can also influence bio-ink characteristics, making them suited for specific tissues.
2.1 Extrusion based bioprinting
A wide range of 3D bio-printing technologies is widely accessible (Vijayavenkataraman et al., 2018; Vanaei et al., 2021), such as extrusion, inkjet, microfluidic, stereolithography, and laser-based techniques. These techniques enable variability in resolution levels, printing precision, working volumes, acceptable bio-inks, and the capacity to inculcate cells due to their material processing principles. Furthermore, their production speed and total consumption prices differ, resulting in efficiency differences. As a result, the extrusion bioprinting technology appears to be the most promising option for producing therapeutically relevant scaffolds (cm range) (Malda et al., 2013; Herzog et al., 2024; Padhy et al., 2024). Extrusion printing involves layer-by-layer bio-ink deposition on the printing platform through a cartridge nozzle (Figure 3A). However, in the absence of cells, the deposited substance is called biomaterial ink, and with cells, it should be referred to as bio-ink (Groll et al., 2019). Extrusion printing can be either pneumatically or mechanically propelled. In this printing method, various parameters that inform the printing qualities include effective flow rate, printing speed, and rheological qualities of the gel. These bioprinting approaches entail straightforward equipment, simplicity of use, and minimal cost. Another feature that distinguishes this technology is its high manufacturing volume, excellent precision (up to a micrometer level), and tremendous design flexibility (Schwab et al., 2020). Several computer-aided design (CAD) models, which are simple, easy to use, and consist of medical photographs of patients’ specific tissues, are freely or commercially accessible.
Gelatin and its derivatives are often used as hydrogel materials in extrusion bioprinting because they have minimal cell toxicity, strong biocompatibility, high accessibility, excellent rheological characteristics, and easy/flexible handling. However, maintaining good form accuracy and post-printing stability, particularly at physiological temperatures, as well as mechanical qualities that match the intended tissue, can be difficult due to gelatin’s inherent poor mechanical characteristics and temperature reactivity. Solving the latter problem entails altering gelatin with a molecule that imparts chemical or enzymatic crosslinking properties. Such modifications can aid post-printing stability/degradation because pure gelatin only has physical crosslinking depending on temperature. In addition, yield stress is essential in identifying the best biomaterial ink for extrusion printing methods. For example, static yield stress (αstat) is necessary for proper gel flow through the nozzle. While the dynamic yield stress (αdyn) determines the gel flow regulation. The bio-ink must possess both rigidity to facilitate the continuous filament extrusion through the nozzle in conjunction with minor deformation for shear thinning behavior necessary during extrusion bioprinting (Smith et al., 2018) (Figure 2). Printing gelatin at temperatures much below its melting point is the most popular method due to its gelation and viscous nature at such temperatures, which facilitate the printing handling.
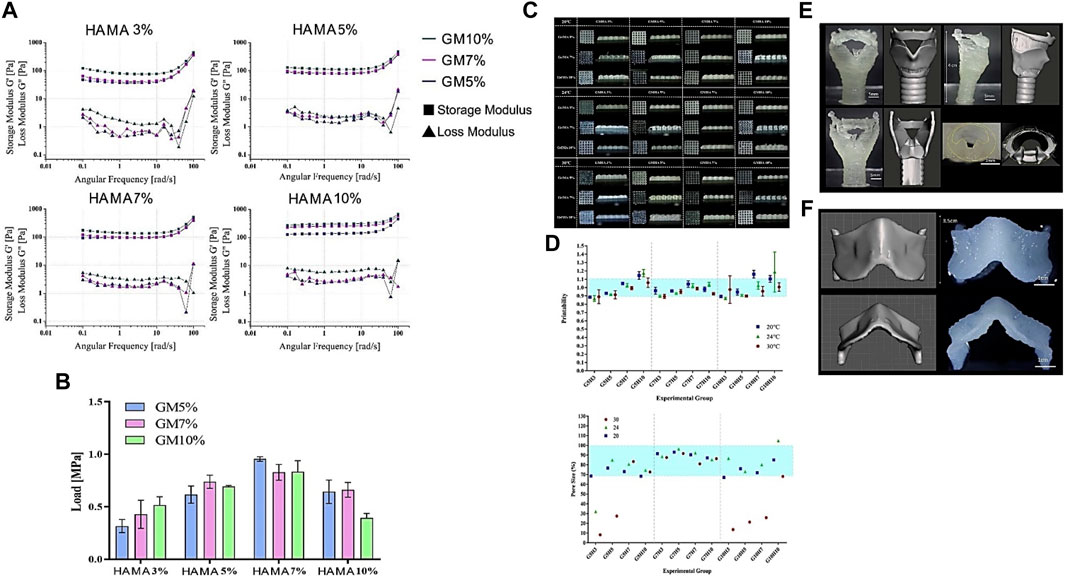
FIGURE 2. Rheological, Mechanical, and Printability assessment of various bio-ink formulations illustrating (A) Viscoelasticity. (B) Compressive strength (C) GM/HAMA bio-ink 3D printed mesh scaffolds (D) Printability measurement using mesh images (E) Rabbit larynx CAD model and 3D Printed larynx with G7H5 bio-ink. (F) Rabbit thyroid cartilage CAD model and 3D Printed thyroid cartilage with G7H5 bio-ink. Reproduced with permission (Lee et al., 2020).
2.2 Light based bioprinting
Recently, light-based bioprinting has attracted much interest in the biomedical field, especially tissue engineering, due to its suitability to generate complex tissue architectures. The commonly available light-based bioprinting techniques include mask-based stereolithography (SLA), laser-based SLA, and digital light processing (DLP). DLP stands out among all these technologies due to its simplicity, versatility, and cost-effectiveness in printing intricate and complex tissues (Zhang et al., 2023). DLP bioprinting is a form of bioprinting process based on modified stereolithography. The operating method uses light to selectively harden a bio-ink layer by layer, additively producing a build (Figure 3B). DLP printers cure bioink planes by plane using a digital light projector. The single-layer projection onto the printing plane for photo-curing in DLP helps it to outperform the other bioprinting technologies. In addition, the printing time is constant in one layer, regardless of the complicated design. Consequently, the printer requires a vertically movable stage, greatly simplifying printer control. As of now, the reported SLAbioprinting technology resolution is around 100 µm and printing durations of below 1 h (Gou et al., 2014) while retaining extremely high cell viability (>90%). This great cell survivability and biocompatibility are due to no external shear stress on cells during bioprinting (Derakhshanfar et al., 2018), as opposed to the extrusion bioprinting technique. However, the material-light interaction, such as the physical characteristics of the bio-ink paired with the photocrosslinking process parameters, heavily influences the mechanical features and resolution of the 3D printed structures (Wang et al., 2018). Based on the photoinitiators, UV or visible light sources are widely employed in the DLP bioprinting technology. Two mechanisms-acryloyl-based crosslinking (Yue et al., 2015) and the thiol-ene click reaction (Greene et al., 2017), are routinely employed to establish covalent bonding in bio-inks by photocrosslinking without considerable cytotoxicity of encapsulated cells. As a result, when utilizing hydrogel macromers as bio-inks, the hydrogel should be modified with an alkenyl or acryloyl functional group. Many academia have published various studies using DLP for 3D bioprinting constructions for orthopedics, particularly cartilage tissue, employing various second-phase polymers (s) such as polyethylene glycol diacrylate (PEGDA) (Zhu et al., 2018), hyaluronic methacrylate (HAMA) (Lam et al., 2019), and silk fibroin methacrylate (SFMA) (Tao et al., 2022).
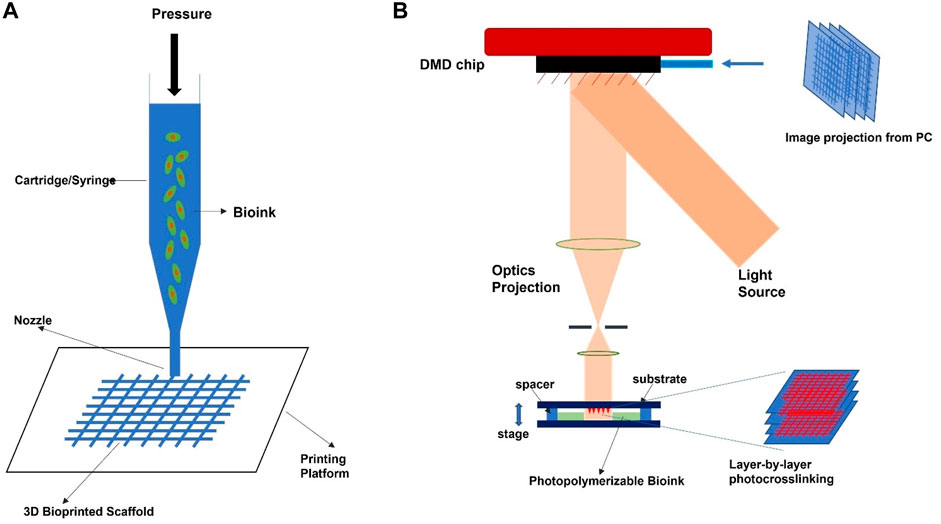
FIGURE 3. Schematic illustration diagram of common bioprinting technology for orthopedic applications. (A) extrusion-based technique, (B) light-based technique.
2.3 Gelatin as a 3D bioprinting material for orthopedics applications
Gelatin is an intriguing biocompatible protein with a massive spectrum of physical and chemical features. These fantastic characteristics enable the production of high and low-viscosity gelatin-based bio-inks for various applications, including orthopedics. Gelatin improves bio-ink viscosity for extrusion printing methods because of its temperature-gelation phenomenon. At low temperatures, gelation of gelatin occurs and becomes very viscous; therefore, their physical gelation can temporarily stabilize the printed structure post-printing (Tajima et al., 2018; Yang et al., 2023). In contrast, the low viscosity of the bio-ink is necessary for light-based printing processes. The modified form of gelatin, known as gelatin derivatives, is the most common type used for different tissue engineering applications. One typical modification method is the methacrylation of gelatin, which results in gelatin methacrylate (GM) (Claaßen et al., 2018). Currently, the bio-ink formulations based on GM for specific cell type support to engineer or reconstruct functional tissues are ongoing (Wenz et al., 2017; Sun et al., 2018).
HAp, which accounts for approximately 60% of human B.T., and tricalcium phosphate (TCP) support osteogenic differentiation (Calabrese et al., 2016). These materials (HAp and TCP) are used extensively for orthopedic applications, and bioprinting is no exception. Adding these second-phase materials improves gelatin’s viscosity and its derivatives, which is critical for 3D extrusion bioprinting. However, it also produces biocompatible ink that aids orthopedic regeneration. Wenz et al. (2016) demonstrated a pro-osteogenic impact of GM bio-ink containing HAp. Anada et al. (2019) revealed octacalcium phosphate as a pro-osteogenic influence in GM in a comparable study. They further demonstrated greater vascular sprouting in GM hydrogels by lowering biopolymer concentrations.
Gelatin bio-printing would need effective management of its physical characteristics, especially during extrusion bio-printing because of its temperature sensitivity. Its melting point ranges between 30°C and 37°C based on the bloom intensity, concentration, and pH, making it unstable by melting under physiological conditions. Several ways have been investigated to address this constraint, including forming a permanent peptide link between the amino acids to preserve the structure’s stability at physiological temperature and culture media. Photocrosslinking and enzymatic crosslinking are the most often used techniques to stabilize gelatin-based printed constructs. Both systems have their advantages and disadvantages. The photocrosslinking of methacryloyl groups in GM occurs quickly (in seconds) upon exposure to light or UV, providing structural integrity to the printed construct (Pepelanova et al., 2018). In comparison, enzymatic crosslinking is more time-demanding (minutes) than photo-based crosslinking but less hazardous to cells. Because enzymatic approaches do not have uncrosslinked monomers or generate free radicals, which are not cell friendly, the reason for their cytocompatibility; however, both crosslinking methods provide permanent chain networks, thus providing gelatin chains needed stability for bio-printed structure’s mechanical strength. Enzymes are typically added to gelatin to help form peptide bonds between glutamine-carbonyl group residues and lysine-amino groups in the gelatin chain (Irvine et al., 2015; Naharros Molinero et al., 2024). The peptide bonds stabilize the printed construct and provide mechanical integrity. However, a photoinitiator is added to generate the free radical that initiates the crosslinking in the photocrosslinking method. Because of substrate selectivity, enzymatic crosslinking methods have a low prevalence of side effects. The enzymatic method also removes the requirement for specialized equipment as well as additional photosensitive chemicals that may be harmful (Irvine et al., 2015; Asim et al., 2023).
3 Chemical structure and rheological properties of gelatin
Gelatin originated from a Latin word called gelatos, which means frozen/stiff. Gelatin sources include animals via thermal denaturation/partial hydrolysis. Figure 4A depicts gelatin’s chemical structure (Thakur et al., 2017). Gelatin contains around 88% protein, 10% moisture, and 1%–2% salts, with a dry-weight protein concentration of 98%–99% (Valcarcel et al., 2021). In addition, it consists of several molecular weight chains with molecular weights of (240–375 kDa) hydroxyproline, (160–250 kDa) proline, and (80–125 kDa) Glycine. Proline (20%–24%), hydroxyproline (20%–24%), and glycine (27%–35%) are the primary gelatin amino acids (Jafari et al., 2020) (Figure 4B). Gelatin is categorized into two categories based on the pretreatment of gelatin during the extraction process. Type A gelatin with 6–9 isoionic points is derived through an acid treatment process, while type B gelatin with 5 isoionic points is derived via an alkali treatment procedure (Hosseinkhani et al., 2015). In comparison, type A gelatin has a higher important amino acid content than type B, including threonine, cystine, lysine, hydroxyproline, glycine, alanine, proline, isoleucine, and leucine (Alam and Shubhra, 2015). Furthermore, more significant component amounts (concentration) improve gelatin characteristics and boost strength. In terms of bonding, gelatin is stabilized by various covalent bonds, and many weak bonds regulate its flexibility and separation. Gelatin at low temperatures can form hydrogen bonds and give collagen fold shape structure. Furthermore, the hydrogen bonds stabilize the triple helical arrangement, resulting from triple helix glycine residues and developing weak interactions with the oxygen in the carbonyl group (Kessler et al., 2021). Gelatin gel’s rheological or mechanical qualities are essential in characterization and product making, particularly in the pharmaceutical, biomedical, and food industries. It is convenient to make homogenous gelatin gel in a composition between 1% and 50% w/v (Djabourov et al., 1993).
3.1 Rheological tunability of gelatin-based printable inks for orthopedic applications
Gelatin has excellent rheological characteristics, but its heat-sensitive nature at physiological temperatures, which significantly impacts these properties, is a disadvantage. Various ways, however, have been used to improve the characteristics or molecular dynamics of gelatin bio-inks. The most common method is to cool it down to achieve a sol-gel transition and print (Bohidar and Jena, 1993). In addition, the addition of biopolymers (Lim et al., 2018; Sathish et al., 2022), proteins (Deng et al., 2021), particles (Diloksumpan et al., 2020; Ratheesh et al., 2020; Tavares et al., 2021), and their mixtures (Benning et al., 2017; Tian et al., 2021) can also improve gelatin printability and shape fidelity. Various researchers have investigated blending gelatin and its derivatives with other polymers to enhance its printability and rheological features. One of these studies combined 5% HAMA and 7% GM to produce a gel with improved rheological characteristics, consistent mechanical qualities, and printability (Lee et al., 2020).
4 Gelatin-based hydrogels printed formulation for CTE
4.1 Cartilage tissue characteristics
Cartilage is an elastic structural connective tissue with low avascularity and cellularity. Cartilage aids stress absorption, minimizes joint friction, and helps in supporting bone growth. The cartilage tissue’s elasticity features are due to its unique extracellular matrix (ECM), which consists mostly of proteoglycans and collagen II. The cartilage cells secrete a proteoglycan-rich platform, allowing water absorption by the tissue and retaining flexibility. Hydrogel systems resemble cartilage matrix molecular structures. Therefore, hydrogel systems are the best option to repair or regenerate cartilage tissue. In addition, they can supply the necessary biological and physical signals for stem cell and chondrocyte proliferation and differentiation.
The classifications of cartilage include elastic, fibro, and hyaline cartilage (Wang et al., 2022a; Sha ban, 2024). The elastic cartilage has a dense network of collagen elastic fibers responsible for its flexibility. Typical examples of elastic cartilage are the outer ear and epiglottis. On the other hand, hyaline cartilage possesses a closely packed smooth surface collagen network, which helps it be flexible and tough. In addition, hyaline cartilage is the commonest cartilage, and bone joint articular cartilage is a typical example. Furthermore, the cartilage within the bone, which acts as an ossification center or growth plate, is another example of hyaline cartilage. However, fibrocartilage is the best suited for support and stiffness due to bundles of collagen fiber embedded with chondrocytes; hence, it is the toughest among all cartilages. Fibrocartilage is present in the meniscus and intervertebral discs in articulating joints. The mechanical qualities of hyaline cartilage, such as articular cartilage, are caused by its biphasic nature. Water interacts with ions in the fluid phase, whereas collagen fibers interact with proteoglycans in the solid part. The liquid phase shift in the fibrous network under loading results in the tissue’s viscoelastic nature (Wilson et al., 2005). Proteoglycans’ charged sidechains preserve the differential osmotic pressure within the surrounding tissues and cartilage, helping in tissue viscoelastic behavior and water retention and the of the tissue.
Articular cartilage absorbs shock and cushioning in the joint when the body moves. Articular cartilage is extensively dependent on its compressive qualities. As a result, any materials intended for articular cartilage regeneration via the bio-printing method should possess comparable attributes. Furthermore, the intervertebral disc (IVD) structure is more complicated than the articular cartilage structure (Crump et al., 2023; She et al., 2023). The IVD annulus pulposus concentric fibrous layers encircled the nucleus pulposus, which carries the axial compressive loads. The cartilaginous endplates link the intervertebral discs’ top and bottom surfaces to the surrounding vertebrae. The IVD’s primary purpose is to take up and disperse loads applied to the spine when we move. Gravity’s axial compression is the fundamental stress on discs. The outer annulus fibrosus distributes the pressure axially. In addition, the body movement torsion lateral and bending stresses are also taken care of by IVDs. Furthermore, IVDs have compressive moduli ranging from 10 to 20 MPa and tensile moduli ranging from 2.6 to 3.5 MPa. Nevertheless, its mechanical shear stiffness is an essential attribute in our body’s axial movement and also involves the discs, which range from 20 to 300 N/mm anterior-posteriorly and 40–300 N/mm laterally (Grace et al., 2015). The material replicating the nucleus pulposus must be a firm supporting hydrogel. The material imitating the annulus fibrosus must include fiber-reinforcing aligned sections that can withstand axial stresses. When creating material solutions for particular cartilage tissues, tissue architecture, and mechanical qualities must be addressed because gelatin provides a hydrogel system with easily modulable mechanical characteristics based on the crosslinking concentration and degree and, as a result, commonly utilized for cartilage 3D bioprinting. Adding second-phase materials or changing gelatin bio-ink formulations can facilitate tunability to meet the desired cartilage tissue. The typical biomechanical properties of cartilage are illustrated in Table 1.
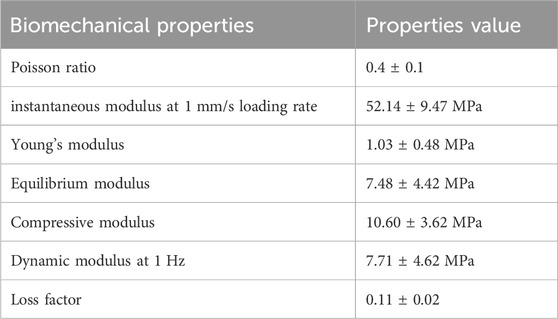
TABLE 1. Example of biomechanical properties of cartilage (Kabir et al., 2021).
4.2 3D-printed cartilage construct requirements
Cartilage regeneration is a considerable clinical concern because of the tissue’s avascularity and poor cell density. Only symptomatic therapies are available for cartilage defects caused by illnesses such as osteoarthritis or trauma. In addition, autologous, abrasion chondroplasty, and allograft are advanced surgical methods only performed in chronic patients. These procedures, however, have many disadvantages, such as graft necrosis, donor site morbidity, shortage of donor sites, and absence of desirable geometry. Therefore, tissue engineering technologies that can assist cartilage tissue regeneration without requiring invasive surgery are an appropriate therapy option. Gelatin-based biomaterial systems can readily offer biophysical signals for cartilage cell growth and differentiation by mimicking the hydrated state of cartilage tissue. As previously indicated, the material characteristics of synthetic cartilage tissue will be the critical element in developing the bio-ink formulation.
Gelatin and its derivatives have previously been utilized with other materials to satisfy the demands of various cartilage tissues. According to one research, a larger quantity of GM + HAMA increased bio-ink stiffness and the synthesis of cartilaginous proteins matrix, resulting in a high premature phenotype. Even after ECM formation, the resultant biomimetically stratified structures preserved their gradient-like system and significantly increased COL2A1 gene expression (+178%) (Shopperly et al., 2022). Similarly, Deng et al. (2021) found that the combination of GM and SFMA gel showed acceptable mechanical characteristics in vitro. However, the combination of GM and SF grafted parathyroid hormone (SF-PTH) gel reduced chondrocyte enlargement and was advantageous in producing hyaline cartilage ECM. The in vivo investigations showed that the scaffolds derived from the variety of GM and SF-PTH/GM and SFMA gels enhance osteochondral engineering and retain a large amount of hyaline cartilage phenotype. Hence, combining gelatin with other biopolymers has not only shown outstanding mechanical features but also demonstrated an improved functionalities.
Gelatin and its derivative’s compression modulus may be easily increased by increasing crosslinking density and weight by volume (w/v) composition, although the acceptable shear/tensile characteristics are difficult to acquire. Mixing gelatin with other polymers produces interpenetrating networks with enhanced crosslinking capabilities. The hydrogel can sustain tensile/shear stresses because of its interpenetrating network. Several investigations have looked into gelatin in conjunction with several other methacrylate biopolymers. A classic example is research in which they created a printing resin of 10% GM and varied amounts of PEGDA. They observed that incorporating PEGDA into GM ink considerably increases printing resolution. Furthermore, the compressive investigation reveals that the modulus of the bio-printed scaffolds rises proportionately with the concentrations of PEGDA (Zhu et al., 2018).
4.3 Gelatin-based bio-ink formulations for CTE
Several cartilage engineering experiments employed gelatin and its derivatives as the primary inks and bio-inks components. Most of these studies focus on creating novel printable functional materials that might be cell-friendly and enable the printing of cartilage tissue, including ear or some meniscus portion, with the goal of tissue engineering. Printed cell-laden, well-defined constructs with patient-specific geometries have the potential to function as space fillers (Kreller et al., 2021; Murali and Parameswaran, 2024). Gelatin is mixed with other materials to enhance the scaffold’s material printability, mechanical characteristics, and long-term stability. In addition, biological activity (usually MSCs or chondrocytes) includes cell spreading, differentiation, and proliferation. Physical sol-gel transformation and enzymatic, chemical, or photochemical crosslinking are all used crosslinking processes. However, the differentiation of MSCs needs a few weeks of culture for chondrocyte formation; therefore, the long-time stability of cell-encapsulated scaffolds is critical (Chu et al., 2021). Table 2 comprises the recently developed gelatin-based bio-inks and the study parameters.
4.4 Cartilage tissue bio-printing
Gelatin has an intriguing viscoelastic property and chondrogenic potential, making it a clear choice of material for cartilage tissue bioprinting. Concentration variations, other biopolymer additions, and additives applications can also readily change gelatin’s rheological characteristics. Even though gelatin and its derivatives have the required physical qualities as biopolymers for printing and cartilage regeneration, they also have binding or bioactive sites for cell signaling and upregulation of chondrogenic pathways. Despite these characteristics, it lacks structural stability and integrity at physiological temperatures. As a result, hybrid systems that can provide stability to the hydrogel system have received much attention in cartilage 3D bioprinting. Therefore, the subsequent paragraph entails a summary of these strategies.
Researchers have used methacrylic groups to create photochemical polymerizations by functionalizing gelatin. The fabrication of physiologically stable and crosslinked structures is achievable by using photoresponsive polymer. In a study (Gu et al., 2018), the encapsulation of primary human chondrocytes in 10% (w/v) GM printed scaffold assisted by a reversible physical crosslinking technique. In addition, the UV light irreversibly crosslinked these printed constructions, ensuring their stability. Encapsulated chondrocyte metabolic activity and proliferation were higher in chondrocytes printed at ideal temperatures than in lower temperatures.
On the other hand, Lim et al. (2018) used tyramine and methacryloyl to dual-functionalize gelatin. The in vivo observation of implanted chondroprogenitor cells inside the printed hydrogel favors neo-cartilage production. The new hydrogel has a glue characteristic that promotes chondrogenesis and allows for safe lateral incorporation into chondral lesions (Figure 5). Hence, the highlighted studies suggest that gelatin-based methacrylic functionalized gel has minimal toxicity and appreciable printability. In addition to being non-toxic, it also supports chondrogenesis in both in vitro and in vivo studies.
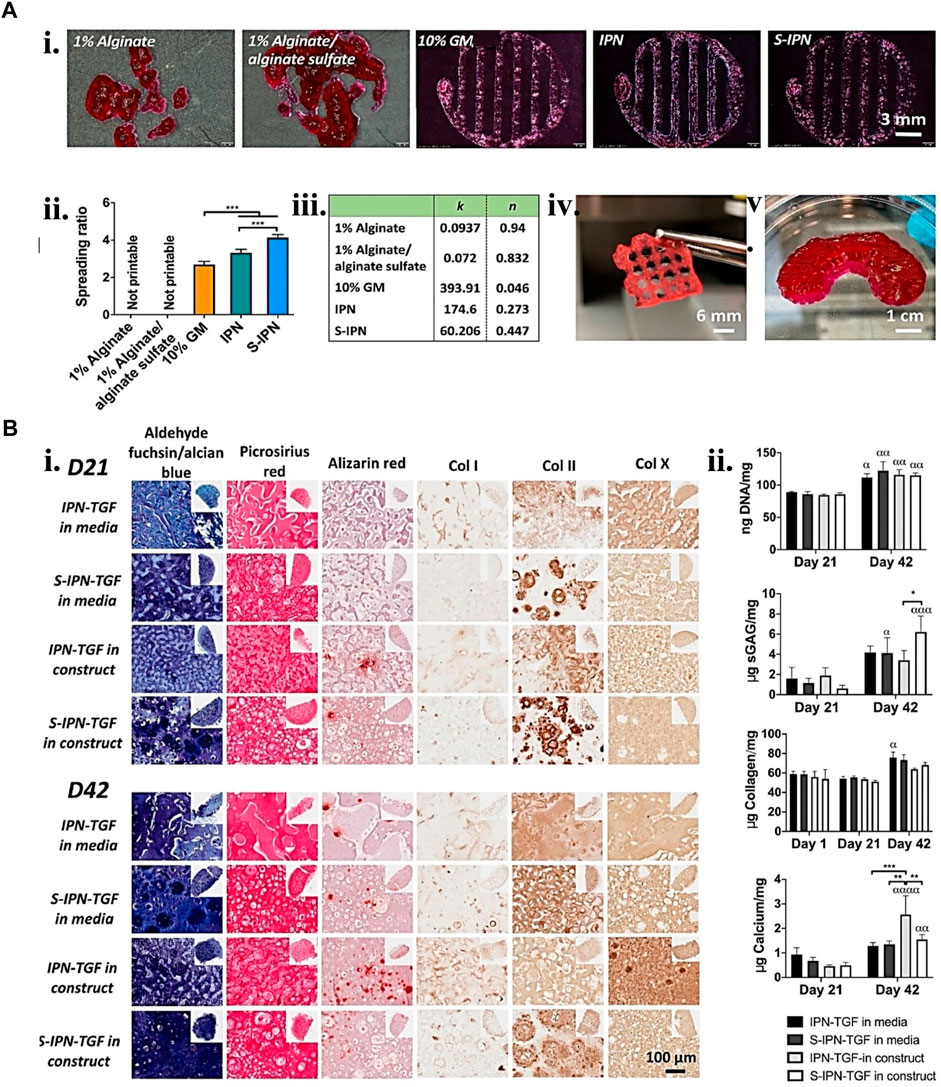
FIGURE 5. Gelatin biopolymers-based bio-ink for CTE showing (A) printability. i. Different gelatin-based formulations 3D printed structure ii. Printed structure spreading ratio. iii. Shear thinning coefficient data from fitting power law to the shear viscosity linear region of the various bio-inks. iv. S-IPN Printed 3D grid construct. v. S-IPN printed meniscus construct. (B) S-IPN and IPN in vitro chondrogenesis study using TGF- β3 in either construct or cell culture media. i. Immunohistochemical and histological staining for calcium, collagen, sulfated glycosaminoglycan (sGAG), collagen type I, II, and X deposition at day 21 and 42 time points. ii. quantification of collagen, DNA, sGAG, and calcium deposited per construct. Reproduced with permission from Wang et al. (2021a).
De Moor et al. (2021) discovered how to modify the phenotypic of spheroid-laden structures by altering the physicochemical parameters of the hydrogel. The phenotypic modification aims to determine the influence of the spheroid maturation level prior to bio-printing on the construct phenotype. Interestingly, the late-stage spheroids printed with a 10 w/v % GM ink produced the best outcomes among the other w/v % studied. Therefore, GM is envisaged to be a suitable material for such applications.
Recently, the production of various biopolymer(s) and gelatin-based hydrogels resulted in fine-tuning their physicochemical and biological features. One of those research involved the development of a novel bio-resin by combining GM, methacrylate poly(vinyl alcohol) (PVA-MA), and a visible light transition metal-based photoinitiator. The developed transitional metal-based system has a high molar absorptivity, which aids the bio-printed scaffolds’ high-resolution 25–50 µm features. The high-resolution cell-laden hydrogel constructions with properly printed complicated and organized architecture demonstrated good cell survival, homogeneous distribution, and functioning (Lim et al., 2018). Likewise, in another work, a cell-laden bio-ink including human adipose-derived mesenchymal stem cells (hADSCs), GM, and HAMA was biopen-produced and maintained in the chondrogenic stimulus for 8 weeks in vitro. A thorough investigation revealed that the technique resulted in the creation of human hyaline-like cartilage (Onofrillo et al., 2018). Hence, it can be noted from both findings described in this paragraph that the incorporation of the second-phase materials does not pose any harm to the cells but enhances the physical integrity and stability of the printed constructs.
On the other hand, Stier et al. (Diloksumpan et al., 2020) disconnected the traditional association between polymer content, stiffness, and equilibrium degree of swelling. They also investigated building hydrogels with graded hydrogel compositions using layer-wise printing and following multiple tests of various biopolymer combinations combining GM, HAMA, and chondroitin sulfate, including the degree of methacrylate. The resultant glycosaminoglycan-graded hydrogel was stable for 28 days. Finally, the encapsulated chondrocytes were alive and formed a new matrix. Similarly, Lee et al. (2020) extrusion 3D bio-printed various GM and HAMA ratios for cartilage regeneration. The G7H5 (GM 7% and HAMA 5%) bio-ink formulation was best suited for constructing a more intricate larynx geometry, which included the thyroid cartilage, hyoid bone, cricoid cartilage, cervical trachea, and arytenoid cartilage. This bio-ink additionally offered a suitable milieu for the in vitro and in vivo chondrogenesis of tonsil-derived MSCs (TMSCs). Hence, the tunability of materials offers enormous benefits in CTE.
The present bio-inks are time-consuming and lack the structural support for a high-shape fidelity scaffold. Overcoming this can reduce the duration required for gel preparation and proper cell dispersion and preserve the predetermined geometry during printing with no extra help. Furthermore, high permeability may allow cell proliferation uniformity in bio-printed constructs, thus helping heal homogenous tissue. As a result, a good permeability time-sharing structure-supporting (TSHSP) hydrogel containing 0.75% CMC (carboxymethyl cellulose), 1% AHA (aldehyde-hyaluronic acid), 0.5% 4-arm poly (ethylene glycol) succinimidyl glutarate (PEG-SG), and 1% gelatin was developed in a study. The quick crosslinking dynamic of AHA/N-carboxymethyl chitosan constituted the basis for the TSHSP mechanism. The in vitro studies of nerves, muscles, and cartilage cells displayed homogenous cell development and impressive biological specificities (Chen et al., 2021). Including growth factors inside the bio-ink is a potential technique to accelerate tissue regeneration. Heparin’s binding solid affinity for alginate sulfate is a tool that can facilitate its adherence to alginate. This property has been used in one study to create a sulfated interpenetrating network (IPN) bio-ink comprising an alginate sulfate functionalized alginate-GM. This bio-ink was 3D printed and not only allowed the continuous discharge of transforming growth factor-3 (TGF-3) and the release of other proteins. It also creates an environment that helps strong in vitro chondrogenesis with no indication of hypertrophy or mineralization over long culture durations (Wang B. et al., 2021). Therefore, a second-phase functional polymer can facilitate and improve gelatin-based bio-ink for cartilage regeneration.
The subjection of printed hydrogel to high quantities of reactive oxygen species (ROS) at defects may impair their phenotypic and functioning, reducing regeneration efficiency. Therefore, an anti-oxidative multifunctional bio-ink is developed in a study to circumvent the ROS challenges. The bio-printed construct increased cell adherence and chondrogenesis of incorporated stem cells. Most notably, after incubation with H2O2, the hydrogel could protect the incorporated stem cells against overexpression of the MMP13 catabolic gene and ROS-facilitated cartilage-specific downregulation such as ACAN and COL2 anabolic genes (Shi et al., 2022). Hence, small molecules with specific functionalities can be incorporated into a bio-ink formulation for a specified function. This is one of the tremendous benefits that hydrogels offer, especially gelatin-based, which has enormous side chains with the potential for functionalization.
Aside from combining a polysaccharide with gelatin and its derivatives to create a cell-laden architecture, some research has looked at diverse protein-derived biopolymers. Cellular hypertrophy is one of the primary issues with today’s gold-standard synthetic cartilage. This results in temporary cartilage that eventually undergoes endochondral ossification to generate bone trabeculae. Chawla et al. (2017) looked at six 3D bio-printed silk-gelatin scaffold conditions to see which produced the most significant results regarding articular cartilage development. In the presence of TGF-1, bone marrow mesenchymal stem cells (BMSCs) undergo hypertrophic differentiation, whereas in the absence of TGF-1, the incorporated BMSCs in bio-printed silk-gelatin gel undergo articular cartilage-type differentiation.
The majority of biopolymers utilized for cartilage regeneration require crosslinking. As a result, different cross-linkers are rarely employed and are invariably harmful to cells. Therefore, the silk fibroin (SF) capacity to undergo secondary structure formation, which induced gelation coupled with a bulking agent, gelatin, produced a crosslinker-free bio-printed construct. Furthermore, the design promotes encapsulated chondrocyte development and proliferation, as well as the production of cartilaginous ECM. The chondrogenic gene expression increase with limited chondrocyte hypertrophy also confirmed the suitability of the developed formulation (Singh et al., 2019). Another interesting, person-specific polymer source is platelet-rich plasma (PRP), widely used as a therapeutic adjuvant for cartilage injury repair. However, PRP treatments in clinics are unsatisfactory and need improvement, particularly in bioactivity maintenance. As a result, Irmak and Gümüşderelioglu (2020) demonstrated a 3D bio-printed photo-crosslinked cell-laden construct containing GM and PRP for tissue-specific constructions. Analyses of in vitro studies indicated an enhancement of ATDC5 differentiation and proliferation in the regularly light-applied GM/PRP gel in the absence of any external chemical agents. More recent examples of gelatin-biopolymers used in bioink for CTE are listed in Table 2.
Mixing gelatin and its derivatives with particulate materials may improve its bioactivity and mechanical characteristics. Kosik-Kozioł et al. (2019) proposed a biomimetic bio-printed gel comprising β-tricalcium phosphate (TCP), alginate, and GM for creating a calcified type of cartilage using an extrusion-based bioprinting approach. The printed structures suitability assessment for cartilage regeneration using RT-qPCR for gene expression quantification such as osteogenic (ALPL, BGLAP) and important chondrogenic (COL1, COL2, COL10A1, ACAN) gene markers. Additionally, fluorescent immunocytochemistry assesses the printed construct quality. Another study used three different bioprinting techniques to bioprint GM, calcium phosphate, and glycosaminoglycan additive biopolymer to assess which bioprinting technique is more cell-friendly. Among the three examined 3D bioprinting processes, DLP printed structures permitted the most significant observed growth in cell number after 7 days (Bedell et al., 2022). The results demonstrate how different bioprinting methods can affect the viability of the cell-laden construct. In another study, a short fiber-reinforced double-network bio-ink was 3D printed to provide an anatomically correct and mechanically adjustable construct for CTE (Figure 6). The addition of short PLLA fibers increases printing fidelity and promotes the generation of mechanically robust constructions. Furthermore, this mechanically reinforced alginate/GM double-network bio-ink is biocompatible and promotes in vitro chondrogenesis of bone marrow-derived stromal cells (Wang et al., 2022b). Hence, particle materials have been shown to provide bioactivities and mechanical functionalities to printed constructs. More recent examples of gelatin particle-based use in bioink for CTE are listed in Table 3.
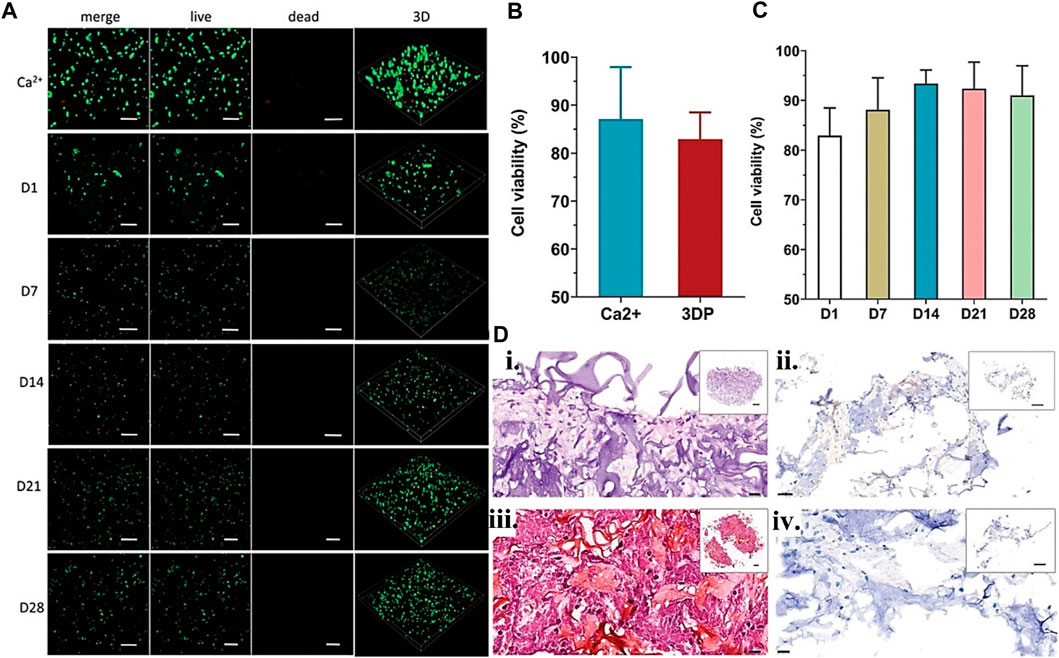
FIGURE 6. Gelatin plus nanoparticle for CTE showing (A) Live/deal cell staining confocal image for BMSCc encapsulated scaffolds from day 1–28-day point with no significant difference in viability. (B) The Ca2+ physically crosslinked and 3D-printed BMSCs viability at day 1 using unpaired t-test, and (C) for various day points using one-way ANOVA. (D) In vitro, scaffold cartilage paraffin sections stained with (i) H&E, (ii) immunohistochemistry, and Safranin O/Fast Green for (iii) type II collagen and (iv) aggrecan. Reproduced with permission from Wang et al. (2022b).
PCL and other synthetic polymers have previously been used to support 3D printing cell-laden structures for self-standing tissue constructions. These strategies are frequently implemented concurrently (i.e., simultaneous printing of plastics and cell-laden bio-inks). Research proved that auricular cartilage repair employing PCL as scaffolding, GM and HAMA layers printed in between, and Lutrol F-127 as sacrificial material. The obtained mechanical characteristics of the resultant hybrid construct are like those of natural cartilage. The printing procedure did not affect the proliferation or viability of hMSC supplied inside the bio-ink (Chung et al., 2020). In another work, PCL microchambers were pre-printed to direct the formation of cellular spheroids. The biomechanical characteristics and composition of the bio-printed construct were comparable to natural cartilage (Daly and Kelly, 2019). In the same version, Ruiz-Cantu et al. (2020) bio-printed chondrocyte cell-laden GM in between PCL plastic structures to improve the mechanical and biological qualities of the developed 3D structure. After 50 days of culture, the 3D bio-printed constructions revealed neocartilage development and mechanical rates similar to nasal alar cartilage. The composite structures’ collagen type II and glycosaminoglycans presence also demonstrated neocartilage development. Even though PCL provides hydrogels with considerable mechanical support, its structural integration with hydrogel is problematic. To find better-automated support solutions. In one study, cellulose nanocrystals support the structural component of a mechanically reinforced hydrogel ink and GM/HAMA ATDC5 cell-laden bio-ink (Figure 7). The printed hybrid scaffolds displayed high mechanical stability, and the printing phase did not primarily affect the survival of ATDC5-encapsulated cells in the scaffold (Fan et al., 2020). More recent examples of gelatin hybrid system use in bioink for CTE are listed in Table 4.
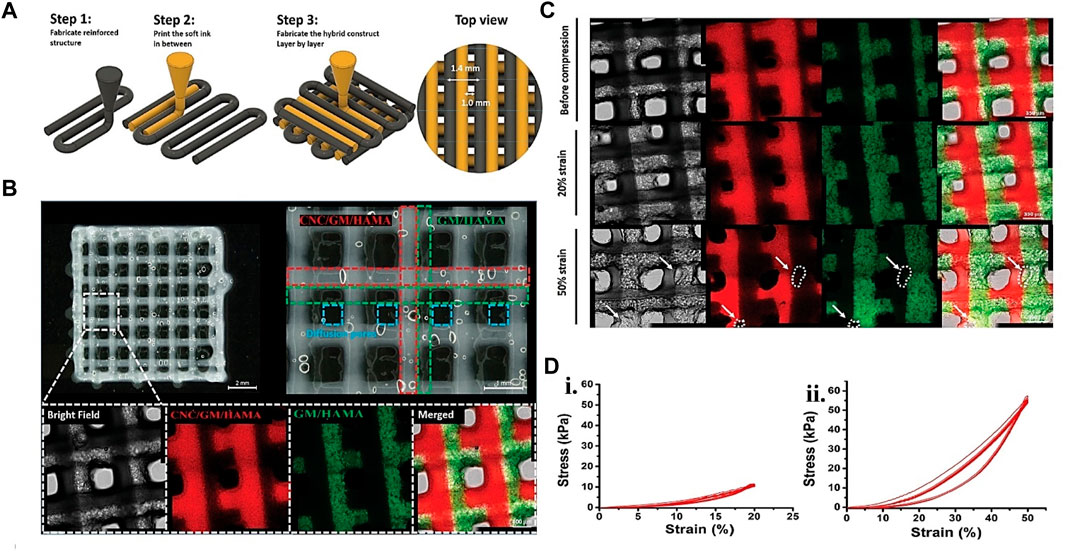
FIGURE 7. Gelatin plus polymer for CTE showing (A) Schematic representation of hybrid printing steps and (B) Hybrid printed confocal and optical micrographs of the hybrid printed scaffold. The confocal image’s green and red dotted lines represent the GM/HAMA and CNC-reinforced biopolymer, respectively. The red fluorescence signifies the rhodamine-labeled GM for GM/HAMA, while the green part is the FITC-labeled GM for the CNC-reinforced system. (C) Confocal images showing the hybrid structure integrity after 20% strain compression in 10 cycles. The white dots and the arrow on 50% strain compression analysis result in 10 cycles revealing structural defects. (D) (i) stress-strain graph for 20% strain during 10 compression cycles. (ii) stress-strain graph for 50% during 10 compression cycles. Adjusted and reproduced with permission from Fan et al. (2020).
5 Gelatin-based hydrogels printed formulation for BTE
5.1 Natural bone characteristics
Bone gives the human body structure and stability. It is a mineralized hard tissue that may regenerate on a smaller scale. More minor bone fractures heal on their own, and they are also often treated using the clinical casting method. However, in case of a significant fracture defect, the defect site will not heal without the help of implants or surgical intervention. Significant bone fracture defects, also called critical-sized bone defects, are often 1–2 cm in size or bigger or when the bone circumference loss is >50% owing to disease, high-energy trauma, or accident (Nauth et al., 2018; Liu F. et al., 2023; Han et al., 2023; Su et al., 2023; Xia et al., 2023). These deformities are challenging to treat since surgical procedures only stabilize the bone fracture. Still, biological material is required to occupy the defective space and assist in new tissue formation. As a result, bioprinting technology, coupled with tissue engineering, offers enormous promise for treating large bone defects. Additionally, printing necessary shapes using clinical defect area photographs to achieve a correct match. There are two kinds of bone tissue: cancellous and cortical. The cortical bone is a thick, dense exterior layer that accounts for 4/5 of bone mass. Cortical bone comprises densely packed osteons with concentric rings surrounding a central canal. Additionally, cortical bone is anisotropic and has a 5%–15% porosity (Morgan et al., 2018; Rodriguez Palomo et al., 2023; Zhou et al., 2023). Furthermore, the cortical bone has a transverse elastic modulus of 10.1 ± 2.4 GPa and a longitudinal elastic modulus of 17.9 ± 3.9 GPa (Reilly and Burstein, 1975). The less dense, lighter, and spongy interior is called cancellous bone and comprises trabeculae. To produce the core bone, the cancellous bone forms a thin but robust interconnectivity with a porosity of about 40%–95% (Morgan et al., 2018). Finally, trabecular bone moduli vary from 10 to 3,000 MPa (Morgan and Keaveny, 2001; Siriphannon and Rukchonlatee, 2023).
The bio-ink bone ultrastructure imitation can boost the production of mechanically robust bone in an in vivo environment since the mechanical qualities of bone are challenging to recreate in the bio-printed strategy. Bone is a nanocomposite containing the protein-mineral crystal. The ECM of bone contains collagen type I, which functions as a scaffold for crystallizing the deposited calcium phosphate to carbonated HAp nanocrystals (Murab et al., 2020). Combining collagen type I present in amino acid residues of hydroxyproline with HAp produces relatively large binding energy nanocomposites between 63 and 126 kJmol−1 (Cutini et al., 2019). Because of this robust nanocomposite ultrastructure, bone tissue has high compressive strength and flexibility. Therefore, if the bio-inks formulation has a similar chemistry to HAp nanocomposite production, in that case, they may be able to aid in the regeneration of mechanically robust bone tissue. Thus, gelatin-based biopolymer has the potential to be a chemically acceptable bio-ink solution for bone tissue bioprinting due to its inherent cell-friendly behavior and the possibility of its mechanical structure tunability.
5.2 3D printed bone constructs requirements
The present surgical procedures for treating large-size bone deformities involve induced membranes, allografts, autografts, and transfer (bone). However, all the mentioned surgical procedures have drawbacks (Bezstarosti et al., 2021; Dalfino et al., 2023). Autografts have the flaws of lack of geometry conformity, secondary morbidity, and a lack of greater graft availability. Conversely, allografts have disadvantages such as transfection, core necrosis, and lack of geometry conformance. Until recently, tissue engineering concepts have served as excellent solutions to all the problems associated with the current therapy methodology. Shape conformation is solved via 3D printing and bioprinting since scaffolds are fabricated in the precise shape and size of the defect location utilizing the defect area medical pictures. The primary critical requirement of the biopolymer is to give recruited cells with a bone-like ultrastructure and chemistry. This bone-like chemistry would provide progenitor cells like BMSCs with physical and chemical signals to develop toward osteogenesis and bone-unique ECM deposition.
Various mineral particles such as HAp may be mixed with hydrogels such as gelatin to improve the bio-ink mechanical qualities such as stiffness while also giving biochemical signals for bMSC development. In one study, after 14 days of printing, a biopolymer system involving HAp, gelatin, and alginate stimulates the osteogenic differentiation of (adipose-derived mesenchymal stem cells) ADSCs (Wang et al., 2016). In contrast, in the control group (alginate-gelatin), the bio-ink system containing HAp improved bone deposition in the mice. The improvement in bone mineralization suggests that particulate HAp can activate osteogenic signaling pathways, creating mineralized, mechanically stable bone tissue. In another study, Sharma et al. (2019) recently bio-printed SF-G-CaCl2 constructs to investigate the effect of calcium release in bone formation. However, the bio-printed scaffolds containing calcium particles enhance the osteogenesis of hMSCs via 1) upregulating the osteogenic markers like OPN, RUNX2, ON, alkaline phosphatase (ALP), and COL I gene expression, 2) upregulating the osteocytic markers such as PDPN, SOST, and DMP 1 gene expression; 3) upregulating the BMP2, BMP4, and β-catenin gene expression; and 4) facilitating mineral deposition, then the scaffolds without calcium. As a result, biochemical and biophysical signals can facilitate the development of gelatin-based bio-inks for BTE. Although, composite formations of mechanical toughness offer a better significant benefit since they can maintain the bio-printed scaffolds in vivo while aiding in more bone formation.
5.3 Gelatin-based bio-ink formulations for BTE
Based on the literature, the wide use of gelatin-based biopolymers as materials for printing bone regeneration scaffolds is apparent. The investigations generally focused on techniques to improve the material’s osteogenic differentiation capability and mechanical characteristics of the scaffolds, as well as on achieving increased vascularized structures (Alcala-Orozco et al., 2020; Zhang et al., 2020; Yang et al., 2022). Pure gelatin or its derivative inks and mixes with other biopolymers, similar to cartilage printing inks, were proposed. Furthermore, printed materials loaded with bioactive materials, including calcium oxide or silica nanoparticles (Tavares et al., 2021), active glasses (Ojansivu et al., 2019), HAp nanoparticles (Allen et al., 2022), or tricalcium phosphate (Jalandhra et al., 2022), have been created. The mineral improves the printed scaffolds’ bioactivity, often enhancing mechanical characteristics, biocompatibility, and higher natural tissue biomimicry. The use of photochemical crosslinking is more than other crosslinking types, such as physical and enzymatic crosslinking. Table 3 shows some recent instances of gelatin-based inks for printing in BTE.
5.4 Bone tissue bio-printing
Autografts and allografts are still the conventional surgical therapy for large-sized bone defects. Both provide mechanical stability and strong integration but possess several drawbacks. The major weaknesses of autografts remain donor site scarcity and morbidity for significant deformity and the unavailability of preferred shapes for implant fitting. In addition, allografts are costly, rare, and include the danger of disease transmission. Larger allografts acquire necrotic cores because they are not coupled to the host’s circulatory network and generate secondary problems. Therefore, bio-inks with biophysical and biochemical signals that can be bio-printed to match the large defect area might be a feasible technique for such BTE. Irmak et al. (2019) used a microwave approach to create a more elastic and robust 3D bio-printed GM than the standard method. Superior mechanical characteristics, increasing cellular survival, adhesion, proliferation, mineralization, ALP activity, and osteogenic genes mRNA expression levels of preosteoblastic MC3T3-E1 cells were found in the formulated hydrogels. This research exemplifies how gelatin may be adjusted to increase its mechanical properties. Celikkin et al. (2022) 3D printed a 5% GM containing MSC. The in vivo findings indicate excellent tissue integration, with no evidence of fibrotic encapsulation or impaired bone growth. Epithelial-mesenchymal interaction (EMI) is an essential element in bone healing. Anything that increases EMI production will inevitably encourage BTE. Recently, epithelial, MSC, and GM cells were 3D printed to aid in promoting EMI by cell recombination. The dimensional culture pattern offered an excellent atmosphere for DPCs and HERS cells to develop mineral deposition patterns, as seen by eosin staining, hematoxylin staining, Masson staining, and immunohistochemistry investigation of the printed construct in vivo. As a result of their interactions, they enhance alveolar bone repair (Tang et al., 2022). Hence, bioinks having both physical and biochemical cues offer enormous potential in BTE.
Combining gelatin and its derivatives with other biopolymers to create an interconnected network and a more mechanically stable hydrogel is an effective strategy for increasing bioactivity. During the osteogenic development of MSC, silk-gelatin bio-ink was bio-printed to stimulate the Indian hedgehog (IHH) and canonical Wnt/-catenin pathways (TVA-BMSC). The encapsulated cell’s early differentiation markers, mid and mid-to-late-stage markers, and terminal osteocytic gene expression demonstrate the construct’s suitability. Furthermore, T3 incorporation and endochondral ossification modeling facilitate the activation of Wnt/-catenin, PTH, and IHH pathways. As a result, stem cell osteogenic differentiation potential and mineralization are enhanced (Chawla et al., 2018). In another dimension, Ma et al. (2017) studied the ECM stem cell interactions via a bioprinting approach to achieve an optimal ECM for alveolar bone repair. The bio-ink formulation consisting of PEGDA and GM was bio-printed with periodontal ligament stem cells (PDLSCs). Finally, an in vivo investigation employing 4/1 GM/PEGDA revealed that PDLSC-laden gel with an optimal formulation outperformed the other formulations for bone development. Similarly, in another study, three biocompatible biopolymers, HA, hydroxyethyl acrylate (HEA), and GM, were employed as cell carriers for bone cell loading in lattice shapes. The 3D bio-printed product demonstrated stable rheology and outstanding biocompatibility (Noh et al., 2019). These studies revealed the importance of bio-ink formulation to encapsulate cell functionalities. More recent examples of gelatin-biopolymer systems used in bioink for BTE are listed in Table 5.
To protect cells against extrusion printing shear stress, the use of microgel cell encapsulated core-shell structure is desirable. One study (Fan et al., 2020) fabricated a core-shell structure consisting of an alginate shell layer and type I collagen core layer microgel using a multichannel microfluidic device to achieve a better cell viable product. The materials SFMA, GM, and microgels were combined and 3D printed. Compared to a 15% SFMA/GM construct, the microgels-15% SFMA/GM construct demonstrated improved biocompatibility and bone formation capability. In most fabrication processes, including additive manufacturing, quickly developing efficient vascularized tissue by 3D-printed constructs remains difficult. Li et al. (2022a) presented a solution to this problem whereby they established and bio-printed a new bio-ink formulation consisting of deferoxamine (DFO)-loaded ethosomes (Eth), GM, and GGMA. The sustained release of DFO from the gel having DFO enhances its mineralization, migration of endothelial cell and tube formation, and osteoblast ALP expression (Figure 8).
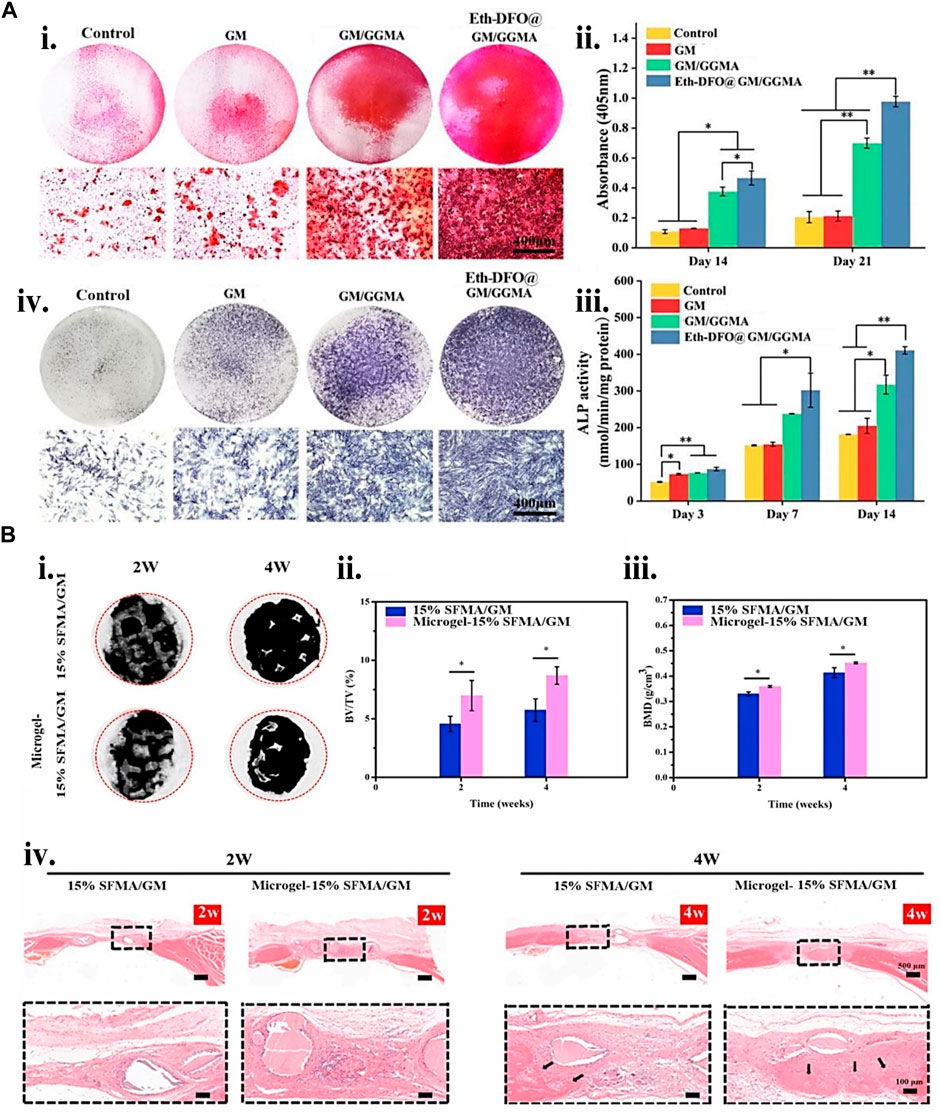
FIGURE 8. Gelatin biopolymers-based bio-ink for BTE showing (A) deferoxamine (DFO)-loaded ethosomes GM/GGMA (Eth-DFO@GM/GGMA) construct osteogenic and angiogenic capacity in vitro. (i) HUVECs revealing Analytical and original tube formation post 9 h culture. (ii) the number of meshes, master segments, and the number of junctions in quantitative analysis. (iii) 21 days BMSCs culture of Alizarin Red S (ARS) staining digital and microscopic images of various bio-ink groups. (iv) Day 14 ALP staining of BMSCs encapsulated construct microscopic and digital images. (v) ALP activity quantitative analysis. (B) (i) Micro-CT micrograph of 2 and 4 weeks post-implantation of 3D reconstruction in a rat cranial bone defects. (ii) 2 and 4 weeks bone volume (BV)/tissue volume (TV) and (iii) bone mass density (BMD) analysis postoperatively. (iv) H&E staining histological analysis at 2 and 4 weeks. The black arrows indicate the new bone formation. Adjusted and reproduced with permission from Li et al. (2022a).
Because gelatin is short of physical stability, adding nano or microparticles may improve the bio-ink physical and mechanical characteristics through nano/micro composites. Interestingly, using the same particle may additionally aid regeneration by carrying bioactive compounds. These mechanical characteristics increases may give biomechanical signals for differentiating mesenchymal stem cells into osteogenic lineages. One recent study (Chung et al., 2020) showed that Laponite® (LPN) and GM were 3D bio-printed to create effective cell-instructive scaffolds. The in vitro nanocomposite study demonstrated high form integrity, human bone marrow mesenchymal stem cells (hBMSC) survivability, and improved osteogenic differentiation support. VEGF-loaded LPN-GM scaffolds revealed considerably greater vascular penetration than GM-VEGF scaffolds. Yu et al. (2020a), on the other hand, bio-printed two bio-polymer gels of strontium-doped calcium silicate powder (FGSr) and fish gelatin methacrylate (FGM). The bio-printed composite outperformed FGM scaffolds regarding mechanical properties, biocompatibility, and osteogenesis differentiation of human Wharton jelly-derived mesenchymal stem cells (WJMSC). Similar results were obtained by embedding silanated silica particles (Choi et al., 2021) and nano-attapulgite (nano-ATP) (Figure 9) (Liu C. et al., 2023) in other studies. Hence, incorporating particles provides mechanical integrity to the cell-laden construct and offers biomechanical cues and bioactivity, enhancing the BTE. More recent examples of gelatin particle systems used in bioink for BTE are listed in Table 6.
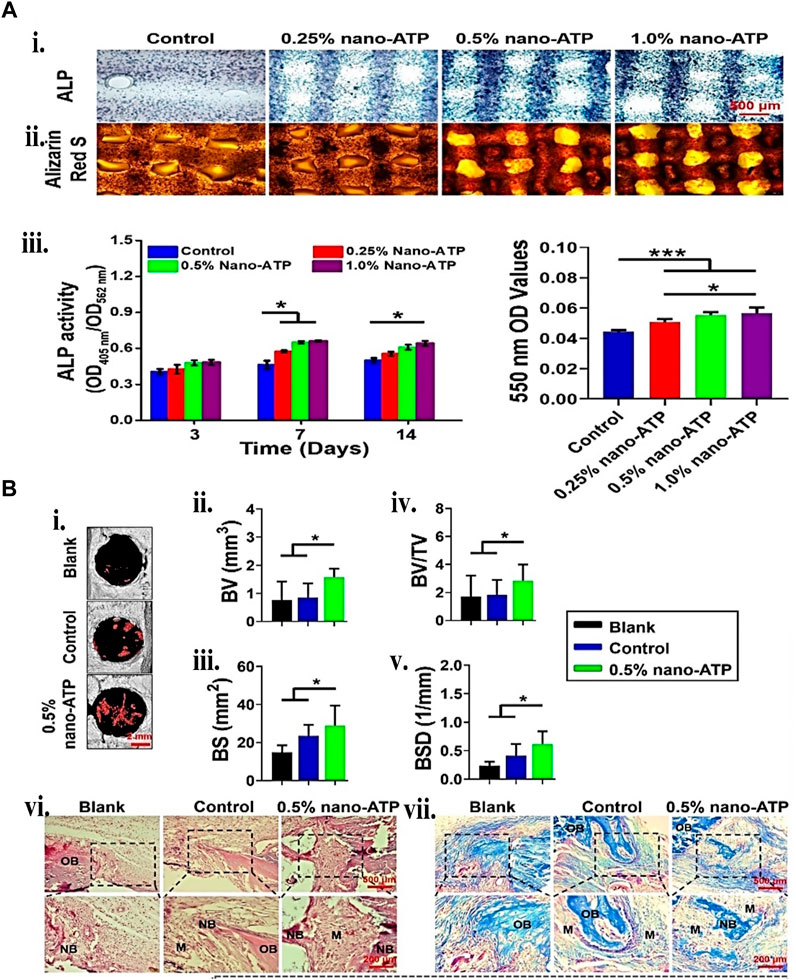
FIGURE 9. Gelatin plus nanoparticle for BTE showing (A) 3D bio-printed BMSCs-laden cell-instructive osteogenesis ability. (i) Day 14 ALP and ARS staining micrograph. (ii) ALP quantitative activity measured between the 405 nm and 562 nm ratio. (iii) calcium minerals deposition quantification by measuring the ARS mineralized stained in the scaffolds. (B) New bone formation histological and micro-computed tomography (μCT) assessment. (i) 2 weeks post-surgery, 3D reconstructed μCT micrographs. The red mark inside the circle indicates the new bone formed. (ii) BV, (iii) bone surface (BS), (iv) BV/TV, and (v) bone surface density (vi) 2 weeks bone defect H&E and Golder’s Trichome staining. (vii) Day 5 and 7 Osteoclasts TRAP staining. Reproduced with permission from Liu et al. (2023b).
Creating a functional and biomimetic nanocomposite bio-ink is another viable option that some researchers have taken advantage of by developing a bio-printed scaffold for orthopedic intervention. A study used (nano-silica) nSi, gelatin, and alginate to bio-print cell-laden rat bone marrow mesenchymal stem cells (rBMSCs) ECM mimicking structure. The nSi in the bio-ink improves the mechanical strength and printability of the encapsulated rBMSCs and triggers osteogenic differentiation. The in vivo investigation further validated the formulation’s potential for critical size defect bone repair (Liu et al., 2020). Similarly, another study created graphene oxide (GO)/alginate/gelatin hMSC-laden bio-ink to build bone-mimicking constructs via a bioprinting approach (Zhang et al., 2021).
Improving osteogenesis through neuropeptide release and neural network restoration is an appealing technique for healing large-size bone deformation. Although the defect area sympathetic nervous system (SNS) is stimulated by traumatic bone defects, causing severe catecholamine release obstructing quick bone repair. In one study (Li S. et al., 2022), nifedipine, a calcium channel blocker, was incorporated in the bio-ink to lower catecholamine concentrations in the bone defect location and promote bone regeneration. The released nifedipine restricted nerve cells’ calcium channels, preventing the activation of SNS and, eventually, reducing catecholamine production. Therefore, reducing catecholamine release facilitates an increase in the bone repair of a critical-size calvarial defect rat model by migration of BMSCs, inhibiting osteoclastogenesis in vitro and promoting osteogenic differentiation.
Bone tissue has a significant vascularization. The interaction of vascular and osteogenic cells is essential for developing these two very different tissue types and their physiological maintenance and repair. One study (Leucht et al., 2020) investigated an all-gelatin-based toolkit containing GM, acetylated GM (GMA), and gelatin to adjust the bio-inks characteristics toward increased printability and more significant support of vascular network creation. The co-culture of bio-printed hADSCs and human dermal microvascular endothelial cells (HDMECs) of constructs revealed tissue-specific functional cells. Interaction influenced the vascular-like architecture creation and maintenance, boosting osteogenesis. On the other hand, Shahabipour et al. (Xia et al., 2023) bio-printed an osteon-like structure by depositing osteogenic and angiogenic bio-inks from the coaxial nozzle shell and core areas. The bio-inks comprise gelatin, GM, alginate, and HAp nanoparticles with preferential HUVECs cells for the core and MC3T3 for the shell: the bio-printed coaxial structure-maintained survivability and the expression of angiogenic and osteogenic factors better than the traditional structure. Similarly, instead of HAp, amine-functionalized copper (Cu)-doped mesoporous bioactive glass nanoparticles (ACuMBGNs) were employed in another study (Zhu et al., 2022).
Because of the macrophage polarization failure and the bone defect site inflammatory milieu, large bone deformation remains a huge therapeutic problem, especially for diabetic patients. Chemicals material or chemicals with anti-inflammatory properties can get around this problem. In one of the recent studies (Sun X. et al., 2021), they combined GM, 4-arm PEG, RAW264.7 macrophages, BMSCs, and mesoporous silica nanoparticles (MSNs) loaded with BMP-4. MSNs substantially increased the mechanical strength and sustained the release of BMP-4. The released BMP-4 enhanced the polarization of RAW264.7 to M2 phase macrophages, facilitating the production of anti-inflammatory components and lower pro-inflammatory factor levels, enhancing rat model bone regeneration.
A hybrid system is another technique investigated for mechanically improving and creating a sturdy 3D build. This technique uses a synthetic polymer scaffold framework that meets the tissue regeneration requirements of strong mechanical characteristics, for example, orthopedic and a self-soft-regulating milieu for cells. In one study (Liu et al., 2022), GM/PCL scaffold was bio-printed and pretreated with Wnt3a loaded ST2 (bone marrow stromal cell line) for 24 h. The 24-h pretreatment increased the cell viability, proliferation, mineralization, and osteogenic differentiation of the encapsulated ST2 in vitro and improved osteogenesis and angiogenesis in a large-size bone defect of calvarial mice, shown in Figure 10. In a similar version, Firouzian et al. (2020) created a biomimetic rat tissue construct to simulate the heterogeneous mechanical characteristics of spinal cord tissue. The cell-laden gelatin/alginate/fibrinogen and primary rat neural cells printed in between the PLGA collagen-coated platform to mimic the soft cell tissue microenvironment. The post 14 days culture analysis of the cell viability, scaffold interface, and immunostaining indicates a homogeneous spread of stable, elongated, healthy neurites and neural cells. However, Nulty et al. (Morgan and Keaveny, 2001) formulated a fibrin-based bio-ink containing HUVEC and hBMSCs for the pre-vascularization of printed PCL scaffolds. The implanted hybrid device in rats with significant femoral bone deficiencies supports new bone formation. The in vivo analysis using Micro-computed tomography (CT) angiography demonstrated enhanced vascularization and large new bone formation. Hence, plastic materials do not only serve the purpose of mechanical support but also give the microenvironment of biomimetic bone tissue.
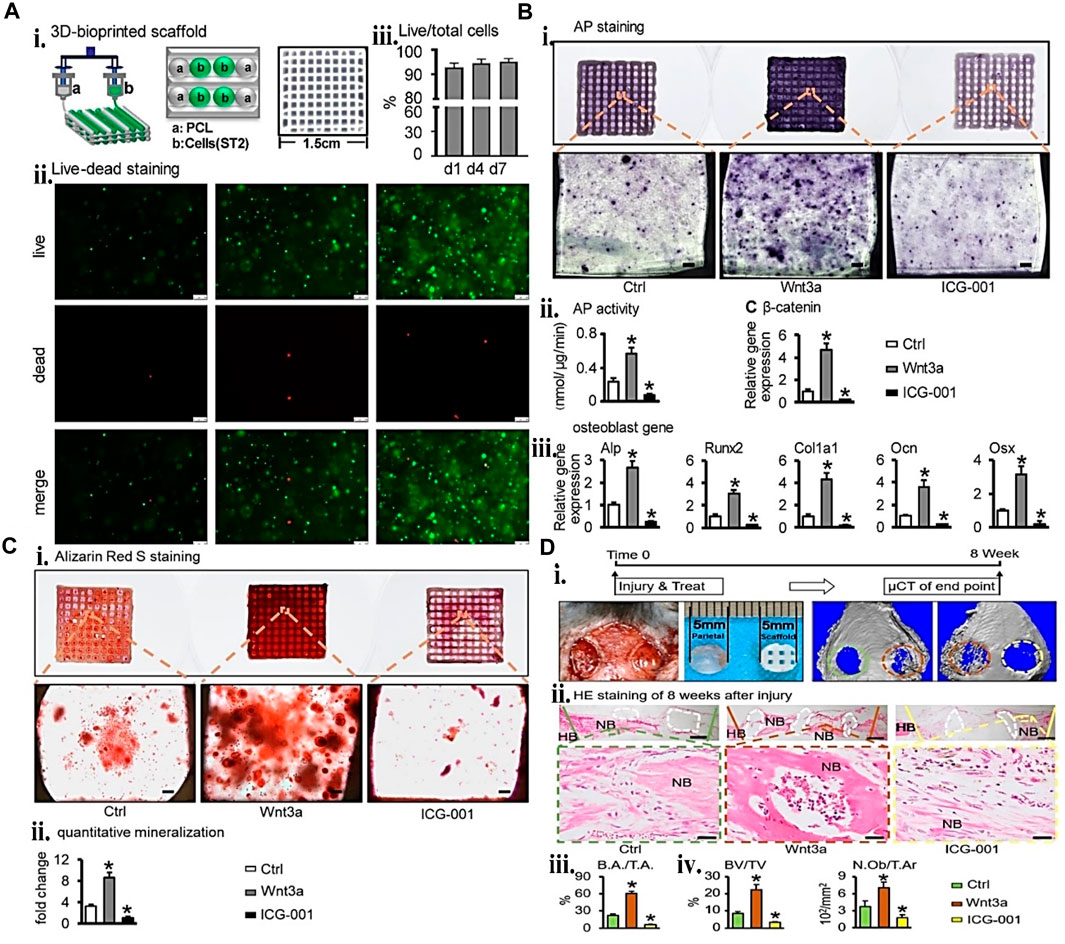
FIGURE 10. Gelatin plus polymer plastic for BTE showing (A) Cell viability assay and ST2/GM/PCL hybrid construct fabrication. (i) schematic representation and gross image of the 3D printed construct. (ii) live/dead staining fluorescence images. (iii) cell viability quantifications at 1,4, and 7-day points. The one-way ANOVA confirms that the cell viability was not statistically different. (B) Osteogenic activity of ST2/GM/PCL hybrid system. (i) ALP staining and (ii) ALP activity quantification. (iii) key factor β-catenin expression of Wnt signaling and (iv) osteogenic markers genes (Runx2, Ocn, Colla1, ALP, and Osx). (C) Mineralization of ST2/GM/PCL hybrid system. (i) ARS staining, (ii) mineralization quantification. (D) Animal study of critical-size defects in mice. (i) Micro-CT at week 8 after implantation of ST2/GM/PCL hybrid system (the control group is shown in the green circle while the brown circle depicts the Wnt3a group, and the ICG-001 group is the yellow circle). (ii) Bone defect section tissue H&E staining. The white dotted area represents PCL, and HB indicates the host bone. (iii) Bone area (B.A) per tissue area (T.A) quantitative analysis. (iv) BV/TV statistical histomorphometry analysis, Osteoblast per tissue area (N.O/T.A). Every study was compared to the control group. Reproduced with permission from Liu et al. (2022).
Large and open bone defects are extremely at risk of pathogens, which can result in high infection chances and delay bone healing. A scaffold with dual osteoinduction and bacterial suppression functionalities is required to promote the effective healing of infectious bone lesions. One recent study (Wang M. et al., 2021) created a hybrid system comprising modified cells using PCL/mesoporous bioactive glass/DOX and bio link. The in vitro and in vivo investigations demonstrated that the fabricated hybrid system could actively produce BMP2, which helps stimulate osteoblast development, causes ectopic bone synthesis, and has antimicrobial properties. More recent examples of gelatin hybrid systems used in bioink for CTE are listed in Table 7.
Volumetric bioprinting (VBP) has recently emerged as a revolutionary technique that utilizes light projections to fabricate centimeter-scale tissue constructs within seconds (Bernal et al., 2019; Kelly et al., 2019; Rizzo et al., 2021; Gehlen et al., 2023). This nozzle-free approach leverages existing imaging techniques like CT scans to create complex 3D structures with high resolution and exceptional cell viability. One recent study demonstrated the potential of VBP for enhanced in vitro bone formation using 3D endothelial co-culture (Gehlen et al., 2023). They identified a soft bioink formulation (5% GelMA, 0.05% LAP) that promotes cell-matrix interactions and communication within the 3D construct. This optimized bioink led to increased expression of bone-specific markers in co-cultured constructs compared to monocultures, suggesting accelerated osteogenic differentiation. Additionally, they successfully established a perfusable pre-vascularized bone construct (Figure 11), paving the way for future studies on bone tissue maturation and function. While promising, further research is needed to address limitations. The developed constructs exhibit limited matrix mineralization and lack mature osteocyte markers. Future studies could explore higher cell densities using optical tuning methods and incorporate additional factors like co-culture with macrophages/osteoclasts and mechanical stimulation to enhance osteogenesis (Sims and Walsh, 2012; Wittkowske et al., 2016; Bernal et al., 2022). Another work used endothelial co-culture and tomographic volumetric bioprinting (VBP) to achieve ultrafast bone tissue model bio-manufacturing. The heterocellular contacts of 3D endothelial co-cultures improve osteogenic development in printed settings. The elevated early osteocytic markers gene expression in 3D co-cultures post 3 weeks validated this osteogenic differentiation enhancement (Gehlen et al., 2022). Overall, volumetric bioprinting holds immense potential for revolutionizing BTE. Addressing the existing limitations and exploring the suggested future directions are crucial for advancing this technology toward clinical applications and creating functional bone replacements.
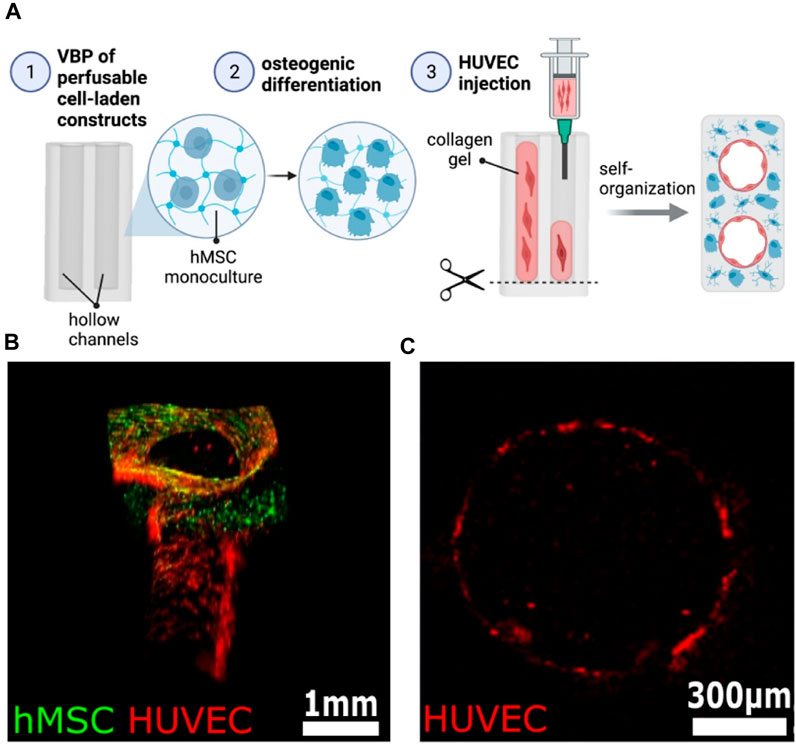
FIGURE 11. Establishment of a heterocellular perfusable pre-vascularization model. (A) Schematic of the experimental procedure for endothelial channel lining in 3D bioprinted constructs. (B) The 3D rendered confocal image of an endothelium-lined channel on day 14 demonstrates successful cell integration (hMSCs: green, HUVECs: red). (C) Cross-section confocal image confirms continuous endothelial lining within the channel (HUVECs: red). Scale bars: B = 1 mm, C = 300 µm. Reproduced with permission ref. Gehlen et al. (2023).
6 4D Bio-printing of gelatin-based bio-inks for orthopedic application
4D printing has evolved to counteract the shortcomings of invariability and complicated structures in tissue engineering and other bio-related disciplines, which are difficult to make via 3D printing (Li et al., 2017; Mahmood et al., 2023; Wang et al., 2023). Skylar Tibbits, an MIT professor, offered a newfangled notion at a TED (technology, entertainment, design) conference that resourcefully channeled the universe’s ingenuity in 3D toward 4D printing. As a result, an additional factor, time, must be considered in addition to the already known x, y, and z-axes geometry in 3D printing (Choi et al., 2015; Sajjad et al., 2023). The 4D printing approach allows the printed construct to vary in form (give dynamicity). Thus, it functions throughout the transformation with the help of the necessary stimuli, such as water (Sydney Gladman et al., 2016), pH (Zhang et al., 2013), thermal (Guo et al., 2018), magnetic (Kokkinis et al., 2015), and so on. Printing in 4D is rapidly expanding its bounds in almost every area, including biomimetics (Momeni and Ni, 2018), electronics (Hua et al., 2018), origami (Janbaz et al., 2016), fashion (Zarek et al., 2016), and a promising biomedical domain [devices (Zarek et al., 2017), tissue engineering (Hendrikson et al., 2017), and so on]—to investigate its dynamism.
The significant advancements in 3D and 4D printing capabilities in biomedicine have generated a subset of 3D and 4D bioprinting attributable to the actualization of physiologically suitable biopolymers involving cell incorporation. As a result, the emergence of 4D bioprinting has induced organ printing dynamicity, such as the heart and other biomedical objects, to maintain tempo with organic physiological characteristics, rendering sensitivity to the surrounding environment (Ambekar and Kandasubramanian, 2019; Rastogi and Kandasubramanian, 2019). The attribution of the 4D bio-printed construct’s responsiveness may be due to cell maturation or the biopolymer shape memory effect, which tries to instill functionalities into the bio-printed construct. Shape memory and smart materials, which have an extraordinary characteristic of storing the translation information between the parent and programmed geometry when subjected to an appropriate microenvironment (stimulus), have intrigued several scientists around the globe with therapies and medications. Nonetheless, both shape memory or smart materials and the stimulus must encourage the physiological systems functioning of the human body. Water-sourced stimulants, for instance, enrich swelling cell-laden scaffolds for varied geometries, including curving, folding, and bending, depending on the bio-printed scaffold anisotropy. Similarly, heat (close to physiological temperature) and magnetic stimuli can cause changes while sustaining cell viability. Cell maturation allows tissue creation over a long duration to mimic the natural complexities in a manufactured 3D structure for practical functioning (Korde and Kandasubramanian, 2019; Yang et al., 2019).
According to Scopus information, despite gelatin’s value (whether in composite or pristine form), its 4D applicability in tissue regeneration and other (bio) engineering applications, such as orthopedic, remains quantitatively sparse. However, Ding et al. (2022) presented a simple method for creating a resilient and adjustable gradient via multi or single-material one-step 4D bio-fabrication (Figure 12). Various photocurable biopolymers such as GM, PEG, alginate, and their derivatives were bio-printed and examined for layer gradient degree of crosslinking with the help of a UV absorber. Furthermore, the developed simple printing strategies can be applied to other hydrogel-based applications, including ion-transfer printing, photomask-aided microfabrication, photo-patterning, and 3D bio-printing for more sophisticated construct architectures. Finally, a 4D bone-like tissue development study established proof-of-concept 4D tissue engineering.
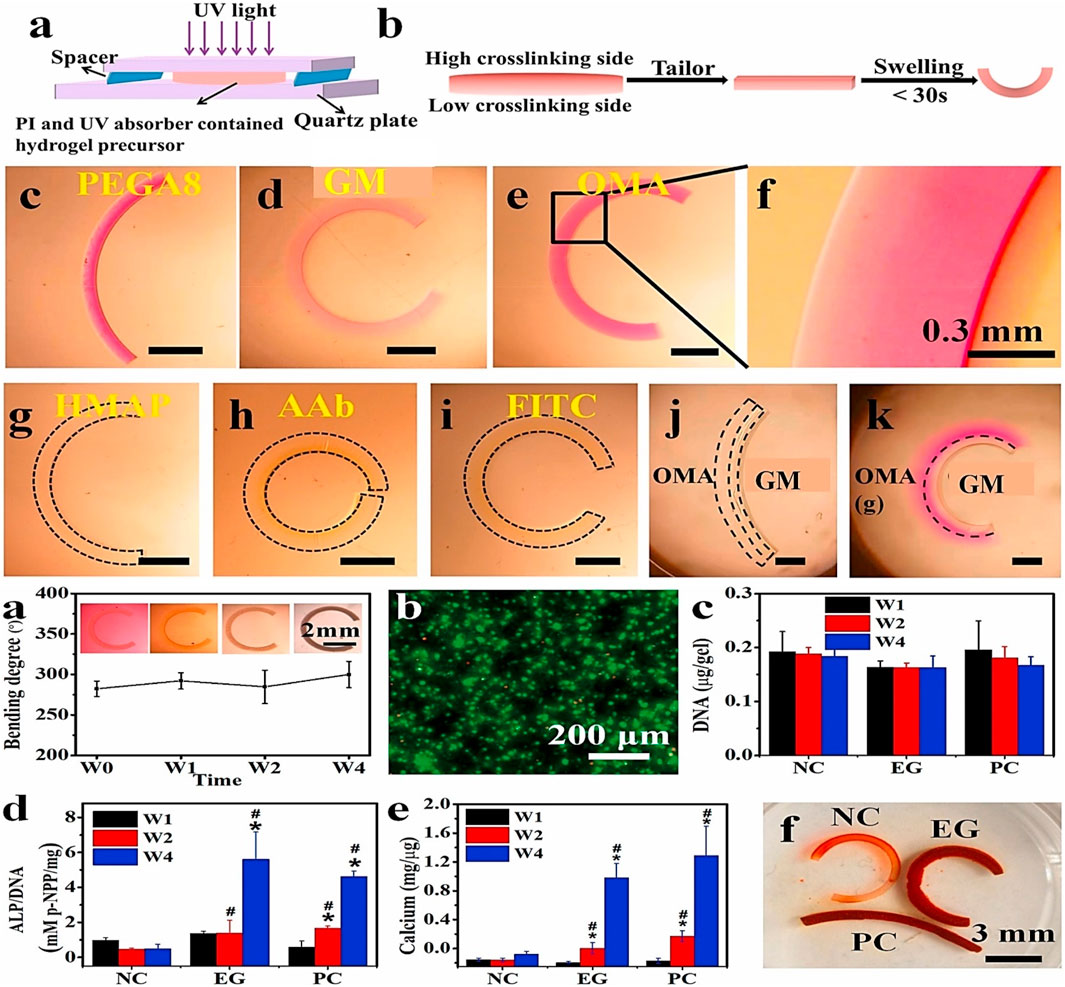
FIGURE 12. A typical gelatin-based 4D bioprinting for orthopedic application showing (A) hydrogel crosslinking gradient schematic, (B) deformation illustration of a gradient hydrogel after swelling. Curling demonstration of (C) PEGDA, (D) GM and (E) OMA achieved under RhB (0.03% w/v) UV absorber. (F) Zoom out micrograph of OMA hydrogel revealing continuous gradient; OMA curved hydrogel bar using various UV absorbers such as (G) FITC (0.03% w/v), (H) AAb (0.05% w/v), and (I) HMAP (0.01% w/v); bilayer hydrogel bars derived using (J) OMA/GM and (K) OMA(g)/GM illustrating the multi-material fabrication feasibility. The OMA(g) represents the OMA gradient hydrogel. Reproduced with permission from Ding et al. (2022).
A few years ago, the invention of 5D printing technology employed five axes to create curves, and more sophisticated things came to life. 6D printing technology now combines the ideas of 4D and 5D printing to create constructs that change geometry with time in reaction to external inputs. Future research will incorporate a mix of multi-dimensional printing technologies and intelligent materials. Multi-dimensional additive manufacturing technology will push the printing dimension to higher degrees of structural flexibility and printing efficacy, good qualities for a wide range of orthopedic applications.
7 Challenges in clinical translation
After scanning patients’ limbs, patient-specific 3D printing of fracture objects is becoming increasingly common. These scaffolds feature a snap closure and personalized fit, perforated and porous to increase skin visibility and reduce skin irritation to help minimize issues in the casting approach. Contrarily, overcoming the bio-printing of orthopedic tissues, many hurdles faced at the clinical stage are imperative before patient use. First and foremost, finding suitable and consistent recruitment of bio-printed cells to obtain an appropriate tissue is still challenging. In an ideal situation, the encapsulated cells must be derived or harvested from the patient to bio-print an appropriate tissue. The isolation of patient bMSCs requires the aspiration of bone marrow, followed by cell expansion in a Good Manufacturing Practices (GMP) facility and printing using a bio-ink formulation created according to GMP procedures. This technique’s intricacy is time-demanding, leading to a lag between tissue construct separation, actual implantation, and printing. As a result, those time-consuming techniques may not be therapeutically feasible when the patient requires rapid assistance.
Second, GMP facilities’ availability globally in hospitals is minimal, limiting the clinic translation to a few locations. Furthermore, the insurance company’s unwillingness to support, the added expenses, and the funding by the national health systems to make and use these products without clinical proof are a few examples of the challenges. As a result, its translatability is further limited to a few chosen medical institutes, significantly limiting the early phases of development. Finally, and probably most critically, a stumbling block exists in the research process. There are not enough surgeon scientists, tissue engineers, and scientists who can perform substantial preclinical animal testing, which serves as a prerequisite before clinical studies. In fact, several animal experiments are conducted on small animals, such as rats, thus providing minimal mechanical behavior information under physiological pressures. Increasing geometries and implant size is critical when analyzing biomaterials for human clinical translation.
Nevertheless, big-size animal models with similar features of physiological tissue stresses and implant sizes may not consistently reproduce the large joints of human biomechanics due to our upright and bipedal nature. Finally, industrial engagement is critical to translation. Due to competing interests, industrial partnerships are challenging to oversee; therefore, most academic institutions cannot handle and control the connections systematically and efficiently due to their intrinsic complexity and available resources. Even with these reservations, a significant partnership involving clinicians, fundamental research, and industry is necessary to take the developed bio-formulations from the laboratory to the bedside regularly and efficiently. As a result, in-depth animal experiments, ethical partnerships, interdisciplinary teams, and collaborations involving industries might pave the path for 3D bio-printable gelatin systems to scale through translation.
8 Conclusion and future prospects
Bioprinting involves the use of hydrogels and specified cells to create cell-laden constructs with the aim of engineering a particular tissue. In contrast to traditional fabrication techniques, bioprinting offers various interesting benefits, including excellent spatiotemporal resolution, desired nano/microstructures at a preferred location on the bio-printed construct, and large throughput production. The 3D bioprinting process has substantially improved printing speed, resolution, and accuracy due to rapidly emerging technologies in mechanical instruments and software. Developing a good bio-ink with appropriate biocompatibility, mechanical and rheological qualities, and printability is critical in generating a desired orthopedic cell-laden construct. Notably, gelatin is mechanically weak and cannot be stable for long; however, these two factors are important for BTE. Additionally, bioactive substances which may promote chondrogenesis and osteogenesis are also absent. As a result, gelatin and its derivative are mixed with other functional nanomaterials/polymers to develop bio-ink with adjustable characteristics.
Additionally, it is necessary to consider developing the ink to sustain various kinds of cells based on their needs. The developed bio-formulations should also support the uniform distributions of chondrocytes with the bio-printed construct and ECM repair, which is critical for CTE. Nevertheless, depending on the target site’s need, it should be able to manage the breakdown of gelatin-based scaffolds. Additionally, including various stimuli-responsive materials in bio-ink and investigating implanted cells’ response to stimuli exposure is also important.
Microfluidic networks can be printed within the scaffold to mimic blood vessels, ensuring vital nutrient and oxygen flow to implanted cells and engineered tissues. This paves the way for treating conditions like osteoporosis and fractures by printing porous scaffolds that promote bone ingrowth and faster healing. While challenges in bioink stability, long-term compatibility, and printing techniques remain, the future of 3D bioprinting with gelatin is incredibly promising. With continued research, this technology could offer personalized solutions for healing, regeneration, and, ultimately, improved quality of life for countless patients. It is worth noting that creating a commercially practical gelatin-based 3D printed product necessitates using principles from numerous fields, including software, instrumentation, biotechnology, material science, chemistry, and so on. As a result, academics worldwide with diverse skills should collaborate with the industry to create innovative bio-ink formulations on a big scale to improve patients’ quality of life.
Author contributions
YW: Writing–original draft, Writing–review and editing, Conceptualization, Data curation, Validation, Visualization. IK: Writing–original draft, Writing–review and editing. SD: Conceptualization, Data curation, Validation, Visualization, Writing–original draft, Writing–review and editing.
Funding
The authors declare that no financial support was received for the research, authorship, and/or publication of this article.
Conflict of interest
The authors declare that the research was conducted in the absence of any commercial or financial relationships that could be construed as a potential conflict of interest.
Publisher’s note
All claims expressed in this article are solely those of the authors and do not necessarily represent those of their affiliated organizations, or those of the publisher, the editors and the reviewers. Any product that may be evaluated in this article, or claim that may be made by its manufacturer, is not guaranteed or endorsed by the publisher.
References
Agarwal, T., Tan, S. A., Onesto, V., Law, J. X., Agrawal, G., Pal, S., et al. (2021). Engineered herbal scaffolds for tissue repair and regeneration: recent trends and technologies. Biomed. Eng. Adv. 2, 100015. doi:10.1016/j.bea.2021.100015
Alam, A. M., and Shubhra, Q. T. (2015). Surface modified thin film from silk and gelatin for sustained drug release to heal wound. J. Mater. Chem. B 3 (31), 6473–6479. doi:10.1039/c5tb00920k
Alcala-Orozco, C. R., Mutreja, I., Cui, X., Hooper, G. J., Lim, K. S., Woodfield, T. B., et al. (2022). Hybrid biofabrication of 3D osteoconductive constructs comprising Mg-based nanocomposites and cell-laden bioinks for bone repair. Bone 154, 116198. doi:10.1016/j.bone.2021.116198
Alcala-Orozco, C. R., Mutreja, I., Cui, X., Kumar, D., Hooper, G. J., Lim, K. S., et al. (2020). Design and characterisation of multi-functional strontium-gelatin nanocomposite bioinks with improved print fidelity and osteogenic capacity. Bioprinting 18, e00073. doi:10.1016/j.bprint.2019.e00073
Alhaskawi, A., Zhou, H., Dong, Y., Zou, X., Ezzi, S. H. A., Kota, V. G., et al. (2024). Advancements in 3D-printed artificial tendon. J. Biomed. Mater. Res. Part B Appl. Biomaterials 112 (2), e35364. doi:10.1002/jbm.b.35364
Allen, N. B., Abar, B., Johnson, L., Burbano, J., Danilkowicz, R. M., and Adams, S. B. (2022). 3D-bioprinted GelMA-gelatin-hydroxyapatite osteoblast-laden composite hydrogels for bone tissue engineering. Bioprinting 26, e00196. doi:10.1016/j.bprint.2022.e00196
Ambekar, R. S., and Kandasubramanian, B. (2019). Progress in the advancement of porous biopolymer scaffold: tissue engineering application. Industrial Eng. Chem. Res. 58 (16), 6163–6194. doi:10.1021/acs.iecr.8b05334
Anada, T., Pan, C. C., Stahl, A., Mori, S., Fukuda, J., Suzuki, O., et al. (2019). Vascularized bone-mimetic hydrogel constructs by 3D bioprinting to promote osteogenesis and angiogenesis. Int. J. Mol. Sci. 20 (5), 1096. doi:10.3390/ijms20051096
Asim, S., Tabish, T. A., Liaqat, U., Ozbolat, I. T., and Rizwan, M. (2023). Advances in gelatin bioinks to optimize bioprinted cell functions. Adv. Healthc. Mater. 12, 2203148. doi:10.1002/adhm.202203148
Barceló, X., Eichholz, K. F., Garcia, O., and Kelly, D. J. (2022). Tuning the degradation rate of alginate-based bioinks for bioprinting functional cartilage tissue. Biomedicines 10 (7), 1621. doi:10.3390/biomedicines10071621
Bedell, M. L., Torres, A. L., Hogan, K. J., Wang, Z., Wang, B., Melchiorri, A. J., et al. (2022). Human gelatin-based composite hydrogels for osteochondral tissue engineering and their adaptation into bioinks for extrusion, inkjet, and digital light processing bioprinting. Biofabrication 14 (4), 045012. doi:10.1088/1758-5090/ac8768
Benning, L., Gutzweiler, L., Tröndle, K., Riba, J., Zengerle, R., Koltay, P., et al. (2017). Cytocompatibility testing of hydrogels toward bioprinting of mesenchymal stem cells. J. Biomed. Mater. Res. - Part A 105 (12), 3231–3241. doi:10.1002/jbm.a.36179
Bernal, P. N., Bouwmeester, M., Madrid-Wolff, J., Falandt, M., Florczak, S., Rodriguez, N. G., et al. (2022). Volumetric bioprinting of organoids and optically tuned hydrogels to build liver-like metabolic biofactories. Adv. Mater. 34 (15), 2110054. doi:10.1002/adma.202110054
Bernal, P. N., Delrot, P., Loterie, D., Li, Y., Malda, J., Moser, C., et al. (2019). Volumetric bioprinting of complex living-tissue constructs within seconds. Adv. Mater. 31 (42), 1904209. doi:10.1002/adma.201904209
Bezstarosti, H., Metsemakers, W. J., van Lieshout, E. M. M., Voskamp, L. W., Kortram, K., McNally, M. A., et al. (2021). Management of critical-sized bone defects in the treatment of fracture-related infection: a systematic review and pooled analysis. Archives Orthop. Trauma Surg. 141 (7), 1215–1230. doi:10.1007/s00402-020-03525-0
Bhardwaj, D., Singhmar, R., Garg, M., Gupta, D., Dhiman, A., Soo Han, S., et al. (2024). Designing advanced hydrogel inks with direct ink writing based 3D printability for engineered biostructures. Eur. Polym. J. 205, 112736. doi:10.1016/j.eurpolymj.2023.112736
Bohidar, H. B., and Jena, S. S. (1993). Kinetics of sol–gel transition in thermoreversible gelation of gelatin. J. Chem. Phys. 98 (11), 8970–8977. doi:10.1063/1.464456
Calabrese, G., Giuffrida, R., Fabbi, C., Figallo, E., Lo Furno, D., Gulino, R., et al. (2016). Collagen-hydroxyapatite scaffolds induce human adipose derived stem cells osteogenic differentiation in vitro. PLoS one 11 (3), 0151181. doi:10.1371/journal.pone.0151181
Catros, S., Guillotin, B., Bačáková, M., Fricain, J. C., and Guillemot, F. (2011). Effect of laser energy, substrate film thickness and bioink viscosity on viability of endothelial cells printed by laser-assisted bioprinting. Appl. Surf. Sci. 257 (12), 5142–5147. doi:10.1016/j.apsusc.2010.11.049
Celikkin, N., Mastrogiacomo, S., Dou, W., Heerschap, A., Oosterwijk, E., Walboomers, X. F., et al. (2022). In vitro and in vivo assessment of a 3D printable gelatin methacrylate hydrogel for bone regeneration applications. J. Biomed. Mater. Res. - Part B Appl. Biomaterials 110 (9), 2133–2145. doi:10.1002/jbm.b.35067
Chai, N., Zhang, J., Zhang, Q., Du, H., He, X., Yang, J., et al. (2021). Construction of 3D printed constructs based on microfluidic microgel for bone regeneration. Compos. Part B Eng. 223, 109100. doi:10.1016/j.compositesb.2021.109100
Chawla, S., Kumar, A., Admane, P., Bandyopadhyay, A., and Ghosh, S. (2017). Elucidating role of silk-gelatin bioink to recapitulate articular cartilage differentiation in 3D bioprinted constructs. Bioprinting 7, 1–13. doi:10.1016/j.bprint.2017.05.001
Chawla, S., Sharma, A., Bandyopadhyay, A., and Ghosh, S. (2018). Developmental biology-inspired strategies to engineer 3D bioprinted bone construct. ACS Biomaterials Sci. Eng. 4 (10), 3545–3560. doi:10.1021/acsbiomaterials.8b00757
Chen, H., Fei, F., Li, X., Nie, Z., Zhou, D., Liu, L., et al. (2021). A structure-supporting, self-healing, and high permeating hydrogel bioink for establishment of diverse homogeneous tissue-like constructs. Bioact. Mater. 6 (10), 3580–3595. doi:10.1016/j.bioactmat.2021.03.019
Chen, S., Tomov, M. L., Ning, L., Gil, C. J., Hwang, B., Bauser-Heaton, H., et al. (2023). Extrusion-based 3D bioprinting of adhesive tissue engineering scaffolds using hybrid functionalized hydrogel bioinks. Adv. Biol. 7, 2300124. doi:10.1002/adbi.202300124
Cheng, L., Suresh K, S., He, H., Rajput, R. S., Feng, Q., Ramesh, S., et al. (2021). 3D printing of micro-and nanoscale bone substitutes: a review on technical and translational perspectives. Int. J. Nanomedicine 16, 4289–4319. doi:10.2147/ijn.s311001
Cheng, L., Yao, B., Hu, T., Cui, X., Shu, X., Tang, S., et al. (2019b). Properties of an alginate-gelatin-based bioink and its potential impact on cell migration, proliferation, and differentiation. Int. J. Biol. Macromol. 135, 1107–1113. doi:10.1016/j.ijbiomac.2019.06.017
Cheng, W., Chen, Y., Teng, L., Lu, B., Ren, L., and Wang, Y. (2018). Antimicrobial colloidal hydrogels assembled by graphene oxide and thermo-sensitive nanogels for cell encapsulation. J. colloid interface Sci. 513, 314–323. doi:10.1016/j.jcis.2017.11.018
Cheng, Y., Chan, K. H., Wang, X. Q., Ding, T., Li, T., Lu, X., et al. (2019a). Direct-ink-Write 3D printing of hydrogels into biomimetic soft robots. ACS Nano 13 (11), 13176–13184. doi:10.1021/acsnano.9b06144
Choi, D. J., Park, S. J., Gu, B. K., Kim, Y. J., Chung, S., and Kim, C. H. (2018). Effect of the pore size in a 3D bioprinted gelatin scaffold on fibroblast proliferation. J. Industrial Eng. Chem. 67, 388–395. doi:10.1016/j.jiec.2018.07.013
Choi, E., Kim, D., Kang, D., Yang, G. H., Jung, B., Yeo, M., et al. (2021). 3D-printed gelatin methacrylate (GelMA)/silanated silica scaffold assisted by two-stage cooling system for hard tissue regeneration. Regen. Biomater. 8 (2), rbab001. doi:10.1093/rb/rbab001
Choi, J., Kwon, O. C., Jo, W., Lee, H. J., and Moon, M. W. (2015). 4D printing technology: a review. 3D Print. Addit. Manuf. 2 (4), 159–167. doi:10.1089/3dp.2015.0039
Chu, Y., Huang, L., Hao, W., Zhao, T., Zhao, H., Yang, W., et al. (2021). Long-term stability, high strength, and 3D printable alginate hydrogel for cartilage tissue engineering application. Biomed. Mater. 16 (6), 064102. doi:10.1088/1748-605x/ac2595
Chung, J. H. Y., Kade, J. C., Jeiranikhameneh, A., Ruberu, K., Mukherjee, P., Yue, Z., et al. (2020). 3D hybrid printing platform for auricular cartilage reconstruction. Biomed. Phys. Eng. Express 6 (3), 035003. doi:10.1088/2057-1976/ab54a7
Cidonio, G., Alcala-Orozco, C. R., Lim, K. S., Glinka, M., Mutreja, I., Kim, Y. H., et al. (2019). Osteogenic and angiogenic tissue formation in high fidelity nanocomposite Laponite-gelatin bioinks. Biofabrication 11 (3), 035027. doi:10.1088/1758-5090/ab19fd
Claaßen, C., Claaßen, M. H., Truffault, V., Sewald, L., Tovar, G. E. M., Borchers, K., et al. (2018). Quantification of substitution of gelatin methacryloyl: best practice and current pitfalls. Biomacromolecules 19 (1), 42–52. doi:10.1021/acs.biomac.7b01221
Crump, K. B., Alminnawi, A., Bermudez-Lekerika, P., Compte, R., Gualdi, F., McSweeney, T., et al. (2023). Cartilaginous endplates: a comprehensive review on a neglected structure in intervertebral disc research. JOR spine 6 (4), e1294. doi:10.1002/jsp2.1294
Cutini, M., Corno, M., Costa, D., and Ugliengo, P. (2019). How does collagen adsorb on hydroxyapatite? Insights from ab initio simulations on a polyproline type II model. J. Phys. Chem. C 123 (13), 7540–7550. doi:10.1021/acs.jpcc.7b10013
Dalfino, S., Savadori, P., Piazzoni, M., Connelly, S. T., Giannì, A. B., Del Fabbro, M., et al. (2023). Regeneration of critical-sized mandibular defects using 3D-printed composite scaffolds: a quantitative evaluation of new bone formation in in vivo studies. Adv. Healthc. Mater. 12 (21), 2300128. doi:10.1002/adhm.202300128
Daly, A. C., and Kelly, D. J. (2019). Biofabrication of spatially organised tissues by directing the growth of cellular spheroids within 3D printed polymeric microchambers. Biomaterials 197, 194–206. doi:10.1016/j.biomaterials.2018.12.028
De Moor, L., Fernandez, S., Vercruysse, C., Tytgat, L., Asadian, M., De Geyter, N., et al. (2020). Hybrid bioprinting of chondrogenically induced human mesenchymal stem cell spheroids. Front. Bioeng. Biotechnol. 8, 484. doi:10.3389/fbioe.2020.00484
De Moor, L., Minne, M., Tytgat, L., Vercruysse, C., Dubruel, P., Van Vlierberghe, S., et al. (2021). Tuning the phenotype of cartilage tissue mimics by varying spheroid maturation and methacrylamide-modified gelatin hydrogel characteristics. Macromol. Biosci. 21 (5), 2000401. doi:10.1002/mabi.202000401
Deng, C., Yang, J., He, H., Ma, Z., Wang, W., Zhang, Y., et al. (2021). 3D bio-printed biphasic scaffolds with dual modification of silk fibroin for the integrated repair of osteochondral defects. Biomaterials Sci. 9 (14), 4891–4903. doi:10.1039/d1bm00535a
Derakhshanfar, S., Mbeleck, R., Xu, K., Zhang, X., Zhong, W., and Xing, M. (2018). 3D bioprinting for biomedical devices and tissue engineering: a review of recent trends and advances. Bioact. Mater. 3 (2), 144–156. doi:10.1016/j.bioactmat.2017.11.008
Diloksumpan, P., de Ruijter, M., Castilho, M., Gbureck, U., Vermonden, T., van Weeren, P. R., et al. (2020). Combining multi-scale 3D printing technologies to engineer reinforced hydrogel-ceramic interfaces. Biofabrication 12 (2), 025014. doi:10.1088/1758-5090/ab69d9
Ding, A., Lee, S. J., Ayyagari, S., Tang, R., Huynh, C. T., and Alsberg, E. (2022). 4D biofabrication via instantly generated graded hydrogel scaffolds. Bioact. Mater. 7, 324–332. doi:10.1016/j.bioactmat.2021.05.021
Djabourov, M., Lechaire, J.-P., and Gaill, F. (1993). Structure and rheology of gelatin and collagen gels. Biorheology 30, 191–205. doi:10.3233/bir-1993-303-405
Fan, Y., Yue, Z., Lucarelli, E., and Wallace, G. G. (2020). Hybrid printing using cellulose nanocrystals reinforced GelMA/HAMA hydrogels for improved structural integration. Adv. Healthc. Mater. 9 (24), 2001410. doi:10.1002/adhm.202001410
Firouzian, K. F., Song, Y., Lin, F., and Zhang, T. (2020). Fabrication of a biomimetic spinal cord tissue construct with heterogenous mechanical properties using intrascaffold cell assembly. Biotechnol. Bioeng. 117 (10), 3094–3107. doi:10.1002/bit.27459
Gao, F., Ruan, C., and Liu, W. (2019). High-strength hydrogel-based bioinks. Mater. Chem. Front. 3 (9), 1736–1746. doi:10.1039/c9qm00373h
Gehlen, J., Qiu, W., Schädli, G. N., Müller, R., and Qin, X. H. (2022). Tomographic volumetric bioprinting of heterocellular bone-like tissues in seconds. Acta Biomater. 156, 49–60. doi:10.1016/j.actbio.2022.06.020
Gehlen, J., Qiu, W., Schädli, G. N., Müller, R., and Qin, X. H. (2023). Tomographic volumetric bioprinting of heterocellular bone-like tissues in seconds. Acta Biomater. 156, 49–60. doi:10.1016/j.actbio.2022.06.020
Gonzalez-Fernandez, T., Tenorio, A. J., Campbell, K. T., Silva, E. A., and Leach, J. K. (2021). Alginate-based bioinks for 3D bioprinting and fabrication of anatomically accurate bone grafts. Tissue Eng. - Part A 27 (17-18), 1168–1181. doi:10.1089/ten.tea.2020.0305
Gopinathan, J., and Noh, I. (2018). Recent trends in bioinks for 3D printing. Biomaterials Res. 22 (1), 11–15. doi:10.1186/s40824-018-0122-1
Gou, M., Qu, X., Zhu, W., Xiang, M., Yang, J., Zhang, K., et al. (2014). Bio-inspired detoxification using 3D-printed hydrogel nanocomposites. Nat. Commun. 5 (1), 3774–3779. doi:10.1038/ncomms4774
Grace, D O., Connell, J. K. L., O Klineberg, E., and Klineberg, E. O. (2015). Tissue engineering a biological repair strategy for lumbar disc herniation. BioResearch Open Access 4 (1), 431–445. doi:10.1089/biores.2015.0034
Greene, T., Lin, T., Andrisani, O. M., and Lin, C. (2017). Comparative study of visible light polymerized gelatin hydrogels for 3D culture of hepatic progenitor cells. J. Appl. Polym. Sci. 134 (11). doi:10.1002/app.44585
Groll, J., Burdick, J. A., Cho, D. W., Derby, B., Gelinsky, M., Heilshorn, S. C., et al. (2019). A definition of bioinks and their distinction from biomaterial inks. Biofabrication 11 (1), 013001. doi:10.1088/1758-5090/aaec52
Gu, Y., Zhang, L., Du, X., Fan, Z., Wang, L., Sun, W., et al. (2018). Reversible physical crosslinking strategy with optimal temperature for 3D bioprinting of human chondrocyte-laden gelatin methacryloyl bioink. J. Biomaterials Appl. 33 (5), 609–618. doi:10.1177/0885328218805864
Guo, J., Zhang, R., Zhang, L., and Cao, X. (2018). 4D printing of robust hydrogels consisted of agarose nanofibers and polyacrylamide. ACS Macro Lett. 7 (4), 442–446. doi:10.1021/acsmacrolett.7b00957
Han, J., Wu, J., Xiang, X., Xie, L., Chen, R., Li, L., et al. (2023). Biodegradable BBG/PCL composite scaffolds fabricated by selective laser sintering for directed regeneration of critical-sized bone defects. Mater. Des. 225, 111543. doi:10.1016/j.matdes.2022.111543
Hasan, M. M., Nuruzzaman Khan, M., Haque, P., and Rahman, M. M. (2018). Novel alginate-di-aldehyde cross-linked gelatin/nano-hydroxyapatite bioscaffolds for soft tissue regeneration. Int. J. Biol. Macromol. 117, 1110–1117. doi:10.1016/j.ijbiomac.2018.06.020
Hendrikson, W. J., Rouwkema, J., Clementi, F., van Blitterswijk, C. A., Farè, S., and Moroni, L. (2017). Towards 4D printed scaffolds for tissue engineering: exploiting 3D shape memory polymers to deliver time-controlled stimulus on cultured cells. Biofabrication 9 (3), 031001. doi:10.1088/1758-5090/aa8114
Henrionnet, C., Pourchet, L., Neybecker, P., Messaoudi, O., Gillet, P., Loeuille, D., et al. (2020). Combining innovative bioink and low cell density for the production of 3D-bioprinted cartilage substitutes: A pilot study. Stem Cells Int. 2020, 2487072. doi:10.1155/2020/2487072
Herzog, J., Franke, L., Lai, Y., Gomez Rossi, P., Sachtleben, J., and Weuster-Botz, D. (2024). 3D bioprinting of microorganisms: principles and applications. Bioprocess Biosyst. Eng., 1–19. doi:10.1007/s00449-023-02965-3
Hölzl, K., Lin, S., Tytgat, L., Van Vlierberghe, S., Gu, L., and Ovsianikov, A. (2016). Bioink properties before, during and after 3D bioprinting. Biofabrication 8 (3), 032002. doi:10.1088/1758-5090/8/3/032002
Hosseinkhani, H., Abedini, F., Ou, K., and Domb, A. J. (2015). Polymers in gene therapy technology. Polym. Adv. Technol. 26 (2), 198–211. doi:10.1002/pat.3432
Hou, Y., Wang, W., and Bartolo, P. (2024). The effect of graphene and graphene oxide induced reactive oxygen species on polycaprolactone scaffolds for bone cancer applications. Mater. Today Bio 24, 100886. doi:10.1016/j.mtbio.2023.100886
Hu, Y., Tang, L., Wang, Z., Yan, H., Yi, X., Wang, H., et al. (2024). Inducing in situ M2 macrophage polarization to promote the repair of bone defects via scaffold-mediated sustained delivery of luteolin. J. Control. Release 365, 889–904. doi:10.1016/j.jconrel.2023.11.015
Hua, D., Zhang, X., Ji, Z., Yan, C., Yu, B., Li, Y., et al. (2018). 3D printing of shape changing composites for constructing flexible paper-based photothermal bilayer actuators. J. Mater. Chem. C 6 (8), 2123–2131. doi:10.1039/c7tc05710e
Huang, X., Lou, Y., Duan, Y., Liu, H., Tian, J., Shen, Y., et al. (2024). Biomaterial scaffolds in maxillofacial bone tissue engineering: a review of recent advances. Bioact. Mater. 33, 129–156. doi:10.1016/j.bioactmat.2023.10.031
Irmak, G., Demirtaş, T. T., and Gumusderelioglu, M. (2019). Highly methacrylated gelatin bioink for bone tissue engineering. ACS Biomaterials Sci. Eng. 5 (2), 831–845. doi:10.1021/acsbiomaterials.8b00778
Irmak, G., and Gümüşderelioglu, M. (2020). Photo-activated platelet-rich plasma (PRP)-based patient-specific bio-ink for cartilage tissue engineering. Biomed. Mater. (Bristol) 15 (6), 065010. doi:10.1088/1748-605x/ab9e46
Irvine, S. A., Agrawal, A., Lee, B. H., Chua, H. Y., Low, K. Y., Lau, B. C., et al. (2015). Printing cell-laden gelatin constructs by free-form fabrication and enzymatic protein crosslinking. Biomed. Microdevices 17 (1), 16. doi:10.1007/s10544-014-9915-8
Jafari, H., Lista, A., Siekapen, M. M., Ghaffari-Bohlouli, P., Nie, L., Alimoradi, H., et al. (2020). Fish collagen: extraction, characterization, and applications for biomaterials engineering. Polymers 12 (10), 2230. doi:10.3390/polym12102230
Jalandhra, G., Romanazzo, S., Nemec, S., Roohani, I., and Kilian, K. A. (2022). Ceramic omnidirectional bioprinting in cell-laden suspensions for the generation of bone analogs. J. Vis. Exp. (186), 2022. doi:10.3791/63943
Janbaz, S., Hedayati, R., and Zadpoor, A. (2016). Programming the shape-shifting of flat soft matter: from self-rolling/self-twisting materials to self-folding origami. Mater. Horizons 3 (6), 536–547. doi:10.1039/c6mh00195e
Kabir, W., Di Bella, C., Choong, P. F., and O’Connell, C. D. (2021). Assessment of native human articular cartilage: a biomechanical protocol. CARTILAGE 13 (2_Suppl. l), 427S–437S. doi:10.1177/1947603520973240
Kelly, B. E., Bhattacharya, I., Heidari, H., Shusteff, M., Spadaccini, C. M., and Taylor, H. K. (2019). Volumetric additive manufacturing via tomographic reconstruction. Science 363 (6431), 1075–1079. doi:10.1126/science.aau7114
Kessler, J. L., Kang, G., Qin, Z., Kang, H., Whitby, F. G., Cheatham, T. E., et al. (2021). Peptoid residues make diverse, hyperstable collagen triple-helices. J. Am. Chem. Soc. 143 (29), 10910–10919. doi:10.1021/jacs.1c00708
Kim, H., Yang, G. H., Choi, C. H., Cho, Y. S., and Kim, G. (2018). Gelatin/PVA scaffolds fabricated using a 3D-printing process employed with a low-temperature plate for hard tissue regeneration: fabrication and characterizations. Int. J. Biol. Macromol. 120, 119–127. doi:10.1016/j.ijbiomac.2018.07.159
Kim, S. H., Kwon, J. S., Cho, J. G., Park, K. G., Lim, T. H., Kim, M. S., et al. (2021). Non-invasive in vivo monitoring of transplanted stem cells in 3D-bioprinted constructs using near-infrared fluorescent imaging. Bioeng. Transl. Med. 6 (2), e10216. doi:10.1002/btm2.10216
Kokkinis, D., Schaffner, M., and Studart, A. R. (2015). Multimaterial magnetically assisted 3D printing of composite materials. Nat. Commun. 6 (1), 8643. doi:10.1038/ncomms9643
Korde, J. M., and Kandasubramanian, B. (2019). Fundamentals and effects of biomimicking stimuli-responsive polymers for engineering functions. Industrial Eng. Chem. Res. 58 (23), 9709–9757. doi:10.1021/acs.iecr.9b00683
Kordjamshidi, A., Saber-Samandari, S., Ghadiri Nejad, M., and Khandan, A. (2019). Preparation of novel porous calcium silicate scaffold loaded by celecoxib drug using freeze drying technique: fabrication, characterization and simulation. Ceram. Int. 45 (11), 14126–14135. doi:10.1016/j.ceramint.2019.04.113
Kosik-Kozioł, A., Costantini, M., Mróz, A., Idaszek, J., Heljak, M., Jaroszewicz, J., et al. (2019). 3D bioprinted hydrogel model incorporating β -tricalcium phosphate for calcified cartilage tissue engineering. Biofabrication 11 (3), 035016. doi:10.1088/1758-5090/ab15cb
Kreller, T., Distler, T., Heid, S., Gerth, S., Detsch, R., and Boccaccini, A. (2021). Physico-chemical modification of gelatine for the improvement of 3D printability of oxidized alginate-gelatine hydrogels towards cartilage tissue engineering. Mater. Des. 208, 109877. doi:10.1016/j.matdes.2021.109877
Kruppke, B., Ray, S., Alt, V., Rohnke, M., Kern, C., Kampschulte, M., et al. (2020). Gelatin-modified calcium/strontium hydrogen phosphates stimulate bone regeneration in osteoblast/osteoclast co-culture and in osteoporotic rat femur defects—in vitro to in vivo translation. Molecules 25 (21), 5103. doi:10.3390/molecules25215103
Lam, T., Dehne, T., Krüger, J. P., Hondke, S., Endres, M., Thomas, A., et al. (2019). Photopolymerizable gelatin and hyaluronic acid for stereolithographic 3D bioprinting of tissue-engineered cartilage. J. Biomed. Mater. Res. - Part B Appl. Biomaterials 107 (8), 2649–2657. doi:10.1002/jbm.b.34354
Lee, J. S., Park, H. S., Jung, H., Lee, H., Hong, H., Lee, Y. J., et al. (2020). 3D-printable photocurable bioink for cartilage regeneration of tonsil-derived mesenchymal stem cells. Addit. Manuf. 33, 101136. doi:10.1016/j.addma.2020.101136
Leucht, A., Volz, A. C., Rogal, J., Borchers, K., and Kluger, P. J. (2020). Advanced gelatin-based vascularization bioinks for extrusion-based bioprinting of vascularized bone equivalents. Sci. Rep. 10 (1), 5330. doi:10.1038/s41598-020-62166-w
Li, L., Lin, Q., Tang, M., Duncan, A. J. E., and Ke, C. (2019). Advanced polymer designs for direct-ink-write 3D printing. Chem. – A Eur. J. 25 (46), 10768–10781. doi:10.1002/chem.201900975
Li, S., Li, Z., Yang, J., Ha, Y., and Zhou, X. (2022b). Inhibition of sympathetic activation by delivering calcium channel blockers from a 3D printed scaffold to promote bone defect repair. Adv. Healthc. Mater. 11 (16), 2200785. doi:10.1002/adhm.202200785
Li, Y.-C., Zhang, Y. S., Akpek, A., Shin, S. R., and Khademhosseini, A. (2017). 4D bioprinting: the next-generation technology for biofabrication enabled by stimuli-responsive materials. Biofabrication 9 (1), 012001. doi:10.1088/1758-5090/9/1/012001
Li, Z., Li, S., Yang, J., Ha, Y., Zhang, Q., Zhou, X., et al. (2022a). 3D bioprinted gelatin/gellan gum-based scaffold with double-crosslinking network for vascularized bone regeneration. Carbohydr. Polym. 290, 119469. doi:10.1016/j.carbpol.2022.119469
Lim, K. S., Levato, R., Costa, P. F., Castilho, M. D., Alcala-Orozco, C. R., van Dorenmalen, K. M. A., et al. (2018). Bio-resin for high resolution lithography-based biofabrication of complex cell-laden constructs. Biofabrication 10 (3), 034101. doi:10.1088/1758-5090/aac00c
Liu, B., Li, J., Lei, X., Cheng, P., Song, Y., Gao, Y., et al. (2020). 3D-bioprinted functional and biomimetic hydrogel scaffolds incorporated with nanosilicates to promote bone healing in rat calvarial defect model. Mater. Sci. Eng. C 112, 110905. doi:10.1016/j.msec.2020.110905
Liu, C., Dai, T., Wu, X., Ma, J., Liu, J., Wu, S., et al. (2023b). 3D bioprinting of cell-laden nano-attapulgite/gelatin methacrylate composite hydrogel scaffolds for bone tissue repair. J. Mater. Sci. Technol. 135, 111–125. doi:10.1016/j.jmst.2022.07.011
Liu, F., Sun, T., An, Y., Ming, L., Li, Y., Zhou, Z., et al. (2023a). The potential therapeutic role of extracellular vesicles in critical-size bone defects: spring of cell-free regenerative medicine is coming. Front. Bioeng. Biotechnol. 11, 1050916. doi:10.3389/fbioe.2023.1050916
Liu, G., Chen, J., Wang, X., Liu, Y., Ma, Y., and Tu, X. (2022). Functionalized 3D-printed ST2/gelatin methacryloyl/polcaprolactone scaffolds for enhancing bone regeneration with vascularization. Int. J. Mol. Sci. 23 (15), 8347. doi:10.3390/ijms23158347
Liu, X., Gaihre, B., George, M. N., Miller, A. L., Xu, H., Waletzki, B. E., et al. (2021). 3D bioprinting of oligo(poly[ethylene glycol] fumarate) for bone and nerve tissue engineering. J. Biomed. Mater. Res. - Part A 109 (1), 6–17. doi:10.1002/jbm.a.37002
Ma, Y., Ji, Y., Zhong, T., Wan, W., Yang, Q., Li, A., et al. (2017). Bioprinting-based PDLSC-ECM screening for in vivo repair of alveolar bone defect using cell-laden, injectable and photocrosslinkable hydrogels. ACS Biomaterials Sci. Eng. 3 (12), 3534–3545. doi:10.1021/acsbiomaterials.7b00601
Mahmood, A., Akram, T., Shenggui, C., and Chen, H. (2023). Revolutionizing manufacturing: a review of 4D printing materials, stimuli, and cutting-edge applications. Compos. Part B Eng. 266, 110952. doi:10.1016/j.compositesb.2023.110952
Malda, J., Visser, J., Melchels, F. P., Jüngst, T., Hennink, W. E., Dhert, W. J. A., et al. (2013). 25th anniversary article: engineering hydrogels for biofabrication. Adv. Mater. 25 (36), 5011–5028. doi:10.1002/adma.201302042
Martins, S. C. R., Marques, M. d. C., Vidal, M. G., Tolentino, P. H. M. P., Dinelli, R. G., Fernandes, G. V. d. O., et al. (2024). Is the facial bone wall critical to achieving esthetic outcomes in immediate implant placement with immediate restoration? A systematic review. Clin. Exp. Med. 33, 0. doi:10.17219/acem/173573
Momeni, F., and Ni, J. (2018). Nature-inspired smart solar concentrators by 4D printing. Renew. Energy 122, 35–44. doi:10.1016/j.renene.2018.01.062
Morgan, E. F., and Keaveny, T. M. (2001). Dependence of yield strain of human trabecular bone on anatomic site. J. Biomechanics 34 (5), 569–577. doi:10.1016/s0021-9290(01)00011-2
Morgan, E. F., Unnikrisnan, G. U., and Hussein, A. I. (2018). Bone mechanical properties in healthy and diseased states. Annu. Rev. Biomed. Eng. 20, 119–143. doi:10.1146/annurev-bioeng-062117-121139
Moriarty, T. F., Harris, L. G., Mooney, R. A., Wenke, J. C., Riool, M., Zaat, S. A. J., et al. (2019). Recommendations for design and conduct of preclinical in vivo studies of orthopedic device-related infection. J. Orthop. Res. 37 (2), 271–287. doi:10.1002/jor.24230
Murab, S., Gruber, S. M., Lin, C. Y. J., and Whitlock, P. (2020). Elucidation of bio-inspired hydroxyapatie crystallization on oxygen-plasma modified 3D printed poly-caprolactone scaffolds. Mater. Sci. Eng. C 109, 110529. doi:10.1016/j.msec.2019.110529
Murali, A., and Parameswaran, R. (2024). Extrusion 3D printing advances for craniomaxillofacial bone tissue engineering. Polymer-Plastics Technol. Mater., 1–24. doi:10.1080/25740881.2024.2307351
Nabavinia, M., Khoshfetrat, A. B., and Naderi-Meshkin, H. (2019). Nano-hydroxyapatite-alginate-gelatin microcapsule as a potential osteogenic building block for modular bone tissue engineering. Mater. Sci. Eng. C 97, 67–77. doi:10.1016/j.msec.2018.12.033
Naharros Molinero, A., Caballo-González, M. Á., de la Mata, F. J., and García-Gallego, S. (2024). Shell formulation in soft gelatin capsules: design and characterization. Adv. Healthc. Mater. 13 (1), 2302250. doi:10.1002/adhm.202302250
Nauth, A., Schemitsch, E., Norris, B., Nollin, Z., and Watson, J. T. (2018). Critical-size bone defects: is there a consensus for diagnosis and treatment? J. Orthop. Trauma 32, S7–S11. doi:10.1097/bot.0000000000001115
Noh, I., Kim, N., Tran, H. N., Lee, J., and Lee, C. (2019). 3D printable hyaluronic acid-based hydrogel for its potential application as a bioink in tissue engineering. Biomaterials Res. 23 (1), 3. doi:10.1186/s40824-018-0152-8
Nulty, J., Freeman, F. E., Browe, D. C., Burdis, R., Ahern, D. P., Pitacco, P., et al. (2021). 3D bioprinting of prevascularised implants for the repair of critically-sized bone defects. Acta Biomater. 126, 154–169. doi:10.1016/j.actbio.2021.03.003
Ojansivu, M., Rashad, A., Ahlinder, A., Massera, J., Mishra, A., Syverud, K., et al. (2019). Wood-based nanocellulose and bioactive glass modified gelatin-alginate bioinks for 3D bioprinting of bone cells. Biofabrication 11 (3), 035010. doi:10.1088/1758-5090/ab0692
Onofrillo, C., Duchi, S., O’Connell, C. D., Blanchard, R., O’Connor, A. J., Scott, M., et al. (2018). Biofabrication of human articular cartilage: a path towards the development of a clinical treatment. Biofabrication 10 (4), 045006. doi:10.1088/1758-5090/aad8d9
Otto, I. A., Capendale, P., Garcia, J., de Ruijter, M., van Doremalen, R., Castilho, M., et al. (2021). Biofabrication of a shape-stable auricular structure for the reconstruction of ear deformities. Mater. Today Bio 9, 100094. doi:10.1016/j.mtbio.2021.100094
Padhy, C., Amin, M., and Sundaram, S. (2024). “Advances, risks, and challenges of 3D bioprinting,” in Mechanical engineering in biomedical applications: bio-3D printing, biofluid mechanics, implant design, biomaterials, computational biomechanics, tissue mechanics, 43–76.
Pepelanova, I., Kruppa, K., Scheper, T., and Lavrentieva, A. (2018). Gelatin-methacryloyl (GelMA) hydrogels with defined degree of functionalization as a versatile toolkit for 3D cell culture and extrusion bioprinting. Bioeng. (Basel) 5 (3), 55. doi:10.3390/bioengineering5030055
Piao, Y., and Chen, B. (2017). Synthesis and mechanical properties of double cross-linked gelatin-graphene oxide hydrogels. Int. J. Biol. Macromol. 101, 791–798. doi:10.1016/j.ijbiomac.2017.03.155
Rastogi, P., and Kandasubramanian, B. (2019). Breakthrough in the printing tactics for stimuli-responsive materials: 4D printing. Chem. Eng. J. 366, 264–304. doi:10.1016/j.cej.2019.02.085
Ratheesh, G., Vaquette, C., and Xiao, Y. (2020). Patient-specific bone particles bioprinting for bone tissue engineering. Adv. Healthc. Mater. 9 (23), 2001323. doi:10.1002/adhm.202001323
Reilly, D. T., and Burstein, A. H. (1975). The elastic and ultimate properties of compact bone tissue. J. Biomechanics 8 (6), 393–405. doi:10.1016/0021-9290(75)90075-5
Rizzo, R., Ruetsche, D., Liu, H., and Zenobi-Wong, M. (2021). Optimized photoclick (bio) resins for fast volumetric bioprinting. Adv. Mater. 33 (49), 2102900. doi:10.1002/adma.202102900
Rodriguez Palomo, A., Østergaard, M., and Birkedal, H. (2023). Bone hierarchical structure: heterogeneity and uniformity. Adv. Funct. Mater., 2307026. doi:10.1002/adfm.202307026
Ruiz-Cantu, L., Gleadall, A., Faris, C., Segal, J., Shakesheff, K., and Yang, J. (2020). Multi-material 3D bioprinting of porous constructs for cartilage regeneration. Mater. Sci. Eng. C 109, 110578. doi:10.1016/j.msec.2019.110578
Saber-Samandari, S., Mohammadi-Aghdam, M., and Saber-Samandari, S. (2019). A novel magnetic bifunctional nanocomposite scaffold for photothermal therapy and tissue engineering. Int. J. Biol. Macromol. 138, 810–818. doi:10.1016/j.ijbiomac.2019.07.145
Sajjad, R., Chauhdary, S. T., Anwar, M. T., Zahid, A., Khosa, A. A., Imran, M., et al. (2023). A Review of 4D Printing-Technologies, Shape Shifting, Smart Materials, and Biomedical Applications. Adv. Industrial Eng. Polym. Res. doi:10.1016/j.aiepr.2023.08.002
Saravanan, S., Chawla, A., Vairamani, M., Sastry, T., Subramanian, K., and Selvamurugan, N. (2017). Scaffolds containing chitosan, gelatin and graphene oxide for bone tissue regeneration in vitro and in vivo. Int. J. Biol. Macromol. 104, 1975–1985. doi:10.1016/j.ijbiomac.2017.01.034
Sathish, P. B., Gayathri, S., Priyanka, J., Muthusamy, S., Narmadha, R., Krishnakumar, G. S., et al. (2022). Tricomposite gelatin-carboxymethylcellulose-alginate bioink for direct and indirect 3D printing of human knee meniscal scaffold. Int. J. Biol. Macromol. 195, 179–189. doi:10.1016/j.ijbiomac.2021.11.184
Schipani, R., Scheurer, S., Florentin, R., Critchley, S. E., and Kelly, D. J. (2020). Reinforcing interpenetrating network hydrogels with 3D printed polymer networks to engineer cartilage mimetic composites. Biofabrication 12 (3), 035011. doi:10.1088/1758-5090/ab8708
Schwab, A., Levato, R., D’Este, M., Piluso, S., Eglin, D., and Malda, J. (2020). Printability and shape fidelity of bioinks in 3D bioprinting. Chem. Rev. 120 (19), 11028–11055. doi:10.1021/acs.chemrev.0c00084
Schwarz, S., Kuth, S., Distler, T., Gögele, C., Stölzel, K., Detsch, R., et al. (2020). 3D printing and characterization of human nasoseptal chondrocytes laden dual crosslinked oxidized alginate-gelatin hydrogels for cartilage repair approaches. Mater. Sci. Eng. C 116, 111189. doi:10.1016/j.msec.2020.111189
Sha ban, M. (2024). “Histology and biomechanics of cartilage,” in Cartilage tissue and knee joint biomechanics (Elsevier), 25–35.
Shahabipour, F., Tavafoghi, M., Aninwene, G. E., Bonakdar, S., Oskuee, R. K., Shokrgozar, M. A., et al. (2022). Coaxial 3D bioprinting of tri-polymer scaffolds to improve the osteogenic and vasculogenic potential of cells in co-culture models. J. Biomed. Mater. Res. - Part A 110 (5), 1077–1089. doi:10.1002/jbm.a.37354
Sharma, A., Desando, G., Petretta, M., Chawla, S., Bartolotti, I., Manferdini, C., et al. (2019). Investigating the role of sustained calcium release in silk-gelatin-based three-dimensional bioprinted constructs for enhancing the osteogenic differentiation of human bone marrow derived mesenchymal stromal cells. ACS Biomaterials Sci. Eng. 5 (3), 1518–1533. doi:10.1021/acsbiomaterials.8b01631
She, Y., Tang, S., Zhu, Z., Sun, Y., Deng, W., Wang, S., et al. (2023). Comparison of temporomandibular joint disc, meniscus, and intervertebral disc in fundamental characteristics and tissue engineering. J. Biomed. Mater. Res. Part B Appl. Biomaterials 111 (3), 717–729. doi:10.1002/jbm.b.35178
Shi, W., Fang, F., Kong, Y., Greer, S. E., Kuss, M., Liu, B., et al. (2022). Dynamic hyaluronic acid hydrogel with covalent linked gelatin as an anti-oxidative bioink for cartilage tissue engineering. Biofabrication 14 (1), 014107. doi:10.1088/1758-5090/ac42de
Shopperly, L. K., Spinnen, J., Krüger, J., Endres, M., Sittinger, M., Lam, T., et al. (2022). Blends of gelatin and hyaluronic acid stratified by stereolithographic bioprinting approximate cartilaginous matrix gradients. J. Biomed. Mater. Res. - Part B Appl. Biomaterials 110 (10), 2310–2322. doi:10.1002/jbm.b.35079
Shuai, C., Guo, W., Wu, P., Yang, W., Hu, S., Xia, Y., et al. (2018). A graphene oxide-Ag co-dispersing nanosystem: dual synergistic effects on antibacterial activities and mechanical properties of polymer scaffolds. Chem. Eng. J. 347, 322–333. doi:10.1016/j.cej.2018.04.092
Shuai, C., Li, Y., Wang, G., Yang, W., Peng, S., and Feng, P. (2019b). Surface modification of nanodiamond: toward the dispersion of reinforced phase in poly-l-lactic acid scaffolds. Int. J. Biol. Macromol. 126, 1116–1124. doi:10.1016/j.ijbiomac.2019.01.004
Shuai, C., Zan, J., Qi, F., Wang, G., Liu, Z., Yang, Y., et al. (2019a). nMgO-incorporated PLLA bone scaffolds: enhanced crystallinity and neutralized acidic products. Mater. Des. 174, 107801. doi:10.1016/j.matdes.2019.107801
Sims, N. A., and Walsh, N. C. (2012). Intercellular cross-talk among bone cells: new factors and pathways. Curr. Osteoporos. Rep. 10, 109–117. doi:10.1007/s11914-012-0096-1
Singh, Y. P., Bandyopadhyay, A., and Mandal, B. B. (2019). 3D bioprinting using cross-linker-free silk-gelatin bioink for cartilage tissue engineering. ACS Appl. Mater. Interfaces 11 (37), 33684–33696. doi:10.1021/acsami.9b11644
Siriphannon, P., and Rukchonlatee, S. (2023). “High density polyethylene/calcium silicate hybrid composite: preparation, characterization and in-vitro bioactivity,” in Materials science forum (Baech, Switzerland: Trans Tech Publ).
Smith, P. T., Basu, A., Saha, A., and Nelson, A. (2018). Chemical modification and printability of shear-thinning hydrogel inks for direct-write 3D printing. Polymer 152, 42–50. doi:10.1016/j.polymer.2018.01.070
Sood, A., Gupta, A., and Agrawal, G. (2021). Recent advances in polysaccharides based biomaterials for drug delivery and tissue engineering applications. Carbohydr. Polym. Technol. Appl. 2, 100067. doi:10.1016/j.carpta.2021.100067
Stier, S., Rebers, L., Schönhaar, V., Hoch, E., and Borchers, K. (2019). Advanced formulation of methacryl- and acetyl-modified biomolecules to achieve independent control of swelling and stiffness in printable hydrogels. J. Mater. Sci. Mater. Med. 30 (3), 35. doi:10.1007/s10856-019-6231-0
Su, N., Villicana, C., Barati, D., Freeman, P., Luo, Y., and Yang, F. (2023). Stem cell membrane-coated microribbon scaffolds induce regenerative innate and adaptive immune responses in a critical-size cranial bone defect model. Adv. Mater. 35 (10), 2208781. doi:10.1002/adma.202208781
Sun, H., Hu, C., Zhou, C., Wu, L., Sun, J., Zhou, X., et al. (2020). 3D printing of calcium phosphate scaffolds with controlled release of antibacterial functions for jaw bone repair. Mater. Des. 189, 108540. doi:10.1016/j.matdes.2020.108540
Sun, M., Sun, X., Wang, Z., Guo, S., Yu, G., and Yang, H. (2018). Synthesis and properties of gelatin methacryloyl (GelMA) hydrogels and their recent applications in load-bearing tissue. Polymers 10 (11), 1290. doi:10.3390/polym10111290
Sun, X., Ma, Z., Zhao, X., Jin, W., Zhang, C., Ma, J., et al. (2021b). Three-dimensional bioprinting of multicell-laden scaffolds containing bone morphogenic protein-4 for promoting M2 macrophage polarization and accelerating bone defect repair in diabetes mellitus. Bioact. Mater. 6 (3), 757–769. doi:10.1016/j.bioactmat.2020.08.030
Sun, Y., Wu, Q., Zhang, Y., Dai, K., and Wei, Y. (2021a). 3D-bioprinted gradient-structured scaffold generates anisotropic cartilage with vascularization by pore-size-dependent activation of HIF1α/FAK signaling axis. Nanomedicine Nanotechnol. Biol. Med. 37, 102426. doi:10.1016/j.nano.2021.102426
Sydney Gladman, A., Matsumoto, E. A., Nuzzo, R. G., Mahadevan, L., and Lewis, J. A. (2016). Biomimetic 4D printing. Nat. Mater. 15 (4), 413–418. doi:10.1038/nmat4544
Tajima, S., Tobita, M., and Mizuno, H. (2018). Current status of bone regeneration using adipose-derived stem cells.
Tang, H., Bi, F., Chen, G., Zhang, S., Huang, Y., Chen, J., et al. (2022). 3D-bioprinted recombination structure of Hertwig’s epithelial root sheath cells and dental papilla cells for alveolar bone regeneration. Int. J. Bioprinting 8 (3), 512. doi:10.18063/ijb.v8i3.512
Tao, J., Zhu, S., Zhou, N., Wang, Y., Wan, H., Zhang, L., et al. (2022). Nanoparticle-stabilized emulsion bioink for digital light processing based 3D bioprinting of porous tissue constructs. Adv. Healthc. Mater. 11 (12), 2102810. doi:10.1002/adhm.202102810
Tavares, M. T., Gaspar, V. M., Monteiro, M. V., S Farinha, J. P., Baleizão, C., and Mano, J. F. (2021). GelMA/bioactive silica nanocomposite bioinks for stem cell osteogenic differentiation. Biofabrication 13 (3), 035012. doi:10.1088/1758-5090/abdc86
Thakur, S., Govender, P. P., Mamo, M. A., Tamulevicius, S., and Thakur, V. K. (2017). Recent progress in gelatin hydrogel nanocomposites for water purification and beyond. Vacuum 146, 396–408. doi:10.1016/j.vacuum.2017.05.032
Tian, Y., Liu, M., Liu, Y., Shi, C., Wang, Y., Liu, T., et al. (2021). The performance of 3D bioscaffolding based on a human periodontal ligament stem cell printing technique. J. Biomed. Mater. Res. - Part A 109 (7), 1209–1219. doi:10.1002/jbm.a.37114
Trucco, D., Sharma, A., Manferdini, C., Gabusi, E., Petretta, M., Desando, G., et al. (2021). Modeling and fabrication of silk fibroin-gelatin-based constructs using extrusion-based three-dimensional bioprinting. ACS Biomaterials Sci. Eng. 7 (7), 3306–3320. doi:10.1021/acsbiomaterials.1c00410
Valcarcel, J., Fraguas, J., Hermida-Merino, C., Hermida-Merino, D., Piñeiro, M. M., and Vázquez, J. A. (2021). Production and physicochemical characterization of gelatin and collagen hydrolysates from turbot skin waste generated by aquaculture activities. Mar. drugs 19 (9), 491. doi:10.3390/md19090491
Vanaei, S., Parizi, M., Salemizadehparizi, F., and Vanaei, H. (2021). An overview on materials and techniques in 3D bioprinting toward biomedical application. Eng. Regen. 2, 1–18. doi:10.1016/j.engreg.2020.12.001
Vijayavenkataraman, S., Yan, W. C., Lu, W. F., Wang, C. H., and Fuh, J. Y. H. (2018). 3D bioprinting of tissues and organs for regenerative medicine. Adv. Drug Deliv. Rev. 132, 296–332. doi:10.1016/j.addr.2018.07.004
Vurat, M. T., Şeker, Ş., Lalegül-Ülker, Ö., Parmaksiz, M., Elçin, A. E., and Elçin, Y. M. (2022). Development of a multicellular 3D-bioprinted microtissue model of human periodontal ligament-alveolar bone biointerface: towards a pre-clinical model of periodontal diseases and personalized periodontal tissue engineering. Genes Dis. 9 (4), 1008–1023. doi:10.1016/j.gendis.2020.11.011
Wang, B., Díaz-Payno, P. J., Browe, D. C., Freeman, F. E., Nulty, J., Burdis, R., et al. (2021a). Affinity-bound growth factor within sulfated interpenetrating network bioinks for bioprinting cartilaginous tissues. Acta Biomater. 128, 130–142. doi:10.1016/j.actbio.2021.04.016
Wang, F., Liu, C., Yang, H., Wang, H., Zhang, H., Zeng, X., et al. (2023). 4D printing of ceramic structures. Addit. Manuf. 63, 103411. doi:10.1016/j.addma.2023.103411
Wang, M., Deng, Z., Guo, Y., and Xu, P. (2022a). Designing functional hyaluronic acid-based hydrogels for cartilage tissue engineering. Mater. Today Bio 17, 100495. doi:10.1016/j.mtbio.2022.100495
Wang, M., Li, H., Yang, Y., Yuan, K., Zhou, F., Liu, H., et al. (2021b). A 3D-bioprinted scaffold with doxycycline-controlled BMP2-expressing cells for inducing bone regeneration and inhibiting bacterial infection. Bioact. Mater. 6 (5), 1318–1329. doi:10.1016/j.bioactmat.2020.10.022
Wang, M., Zhao, J., and Luo, Y. (2022b). 3D contour printing of anatomically mimetic cartilage grafts with microfiber-reinforced double-network bioink. Macromol. Biosci. doi:10.1002/mabi.202200179
Wang, X., Ao, Q., Tian, X., Fan, J., Tong, H., Hou, W., et al. (2017). Gelatin-based hydrogels for organ 3D bioprinting. Polymers 9 (9), 401. doi:10.3390/polym9090401
Wang, X. F., Song, Y., Liu, Y. S., Sun, Y. c., Wang, Y. g., Wang, Y., et al. (2016). Osteogenic differentiation of three-dimensional bioprinted constructs consisting of human adipose-derived stem cells in vitro and in vivo. PLoS ONE 11 (6), 0157214. doi:10.1371/journal.pone.0157214
Wang, Z., Kumar, H., Tian, Z., Jin, X., Holzman, J. F., Menard, F., et al. (2018). Visible light photoinitiation of cell-adhesive gelatin methacryloyl hydrogels for stereolithography 3D bioprinting. ACS Appl. Mater. interfaces 10 (32), 26859–26869. doi:10.1021/acsami.8b06607
Wehrle, M., Koch, F., Zimmermann, S., Koltay, P., Zengerle, R., Stark, G. B., et al. (2019). Examination of hydrogels and mesenchymal stem cell sources for bioprinting of artificial osteogenic tissues. Cell. Mol. Bioeng. 12 (6), 583–597. doi:10.1007/s12195-019-00588-x
Wenz, A., Borchers, K., Tovar, G. E. M., and Kluger, P. J. (2017). Bone matrix production in hydroxyapatite-modified hydrogels suitable for bone bioprinting. Biofabrication 9 (4), 044103. doi:10.1088/1758-5090/aa91ec
Wenz, A., Janke, K., Hoch, E., Tovar, G. E., Borchers, K., and Kluger, P. J. (2016). Hydroxyapatite-modified gelatin bioinks for bone bioprinting. BioNanoMaterials 17 (3-4), 179–184. doi:10.1515/bnm-2015-0018
Wilson, W., van Donkelaar, C., van Rietbergen, B., and Huiskes, R. (2005). A fibril-reinforced poroviscoelastic swelling model for articular cartilage. J. Biomechanics 38 (6), 1195–1204. doi:10.1016/j.jbiomech.2004.07.003
Wittkowske, C., Reilly, G. C., Lacroix, D., and Perrault, C. M. (2016). In vitro bone cell models: impact of fluid shear stress on bone formation. Front. Bioeng. Biotechnol. 4, 87. doi:10.3389/fbioe.2016.00087
Wu, I.-T., Kao, P. F., Huang, Y. R., and Ding, S. J. (2020). In vitro and in vivo osteogenesis of gelatin-modified calcium silicate cement with washout resistance. Mater. Sci. Eng. C 117, 111297. doi:10.1016/j.msec.2020.111297
Wu, J., Cheng, X., Chen, J., and Pei, X. (2024). The development of magnesium-based biomaterials in bone tissue engineering: a review. J. Biomed. Mater. Res. Part B Appl. Biomaterials 112 (1), e35326. doi:10.1002/jbm.b.35326
Xia, D., Qin, Y., Guo, H., Wen, P., Lin, H., Voshage, M., et al. (2023). Additively manufactured pure zinc porous scaffolds for critical-sized bone defects of rabbit femur. Bioact. Mater. 19, 12–23. doi:10.1016/j.bioactmat.2022.03.010
Xiao, S., Zhao, T., Wang, J., Wang, C., Du, J., Ying, L., et al. (2019). Gelatin methacrylate (GelMA)-Based hydrogels for cell transplantation: an effective strategy for tissue engineering. Stem Cell Rev. Rep. 15 (5), 664–679. doi:10.1007/s12015-019-09893-4
Yan, X., Yang, J., Chen, F., Zhu, L., Tang, Z., Qin, G., et al. (2018). Mechanical properties of gelatin/polyacrylamide/graphene oxide nanocomposite double-network hydrogels. Compos. Sci. Technol. 163, 81–88. doi:10.1016/j.compscitech.2018.05.011
Yang, G. H., Yeo, M., Koo, Y. W., and Kim, G. H. (2019). 4D bioprinting: technological advances in biofabrication. Macromol. Biosci. 19 (5), 1800441. doi:10.1002/mabi.201800441
Yang, J., Deng, C., Shafiq, M., Li, Z., Zhang, Q., Du, H., et al. (2022). Localized delivery of FTY-720 from 3D printed cell-laden gelatin/silk fibroin composite scaffolds for enhanced vascularized bone regeneration. Smart Mater. Med. 3, 217–229. doi:10.1016/j.smaim.2022.01.007
Yang, J., He, H., Li, D., Zhang, Q., Xu, L., and Ruan, C. (2023). Advanced strategies in the application of gelatin-based bioink for extrusion bioprinting. Bio-Design Manuf. 6 (5), 586–608. doi:10.1007/s42242-023-00236-4
Yekta, H. J., Shahali, M., Khorshidi, S., Rezaei, S., Montazeran, A. H., Samandari, S. S., et al. (2018). Mathematically and experimentally defined porous bone scaffold produced for bone substitute application. Nanomedicine J. 5 (4), 227–234. doi:10.22038/nmj.2018.05.00007
Ying, G., Jiang, N., Yu, C., and Zhang, Y. S. (2018). Three-dimensional bioprinting of gelatin methacryloyl (GelMA). Bio-Design Manuf. 1 (4), 215–224. doi:10.1007/s42242-018-0028-8
Yu, C. T., Wang, F. M., Liu, Y. T., Lee, A. K. X., Lin, T. L., and Chen, Y. W. (2020a). Enhanced proliferation and differentiation of human mesenchymal stem cell-laden recycled fish gelatin/strontium substitution calcium silicate 3D scaffolds. Appl. Sci. Switz. 10 (6), 2168. doi:10.3390/app10062168
Yu, C. T., Wang, F. M., Liu, Y. T., Ng, H. Y., Jhong, Y. R., Hung, C. H., et al. (2020b). Effect of bone morphogenic protein-2-loaded mesoporous strontium substitution calcium silicate/recycled fish gelatin 3D cell-laden scaffold for bone tissue engineering. Processes 8 (4), 493. doi:10.3390/pr8040493
Yue, K., Trujillo-de Santiago, G., Alvarez, M. M., Tamayol, A., Annabi, N., and Khademhosseini, A. (2015). Synthesis, properties, and biomedical applications of gelatin methacryloyl (GelMA) hydrogels. Biomaterials 73, 254–271. doi:10.1016/j.biomaterials.2015.08.045
Zarek, M., Layani, M., Eliazar, S., Mansour, N., Cooperstein, I., Shukrun, E., et al. (2016). 4D printing shape memory polymers for dynamic jewellery and fashionwear. Virtual Phys. Prototyp. 11 (4), 263–270. doi:10.1080/17452759.2016.1244085
Zarek, M., Mansour, N., Shapira, S., and Cohn, D. (2017). 4D printing of shape memory-based personalized endoluminal medical devices. Macromol. rapid Commun. 38 (2), 1600628. doi:10.1002/marc.201600628
Zhang, J., Eyisoylu, H., Qin, X. H., Rubert, M., and Müller, R. (2021). 3D bioprinting of graphene oxide-incorporated cell-laden bone mimicking scaffolds for promoting scaffold fidelity, osteogenic differentiation and mineralization. Acta Biomater. 121, 637–652. doi:10.1016/j.actbio.2020.12.026
Zhang, J., Wehrle, E., Adamek, P., Paul, G. R., Qin, X. H., Rubert, M., et al. (2020). Optimization of mechanical stiffness and cell density of 3D bioprinted cell-laden scaffolds improves extracellular matrix mineralization and cellular organization for bone tissue engineering. Acta Biomater. 114, 307–322. doi:10.1016/j.actbio.2020.07.016
Zhang, X., Li, Y., and Zhang, Y. (2023). Applications of light-based 3D bioprinting and photoactive biomaterials for tissue engineering. Materials 16 (23), 7461. doi:10.3390/ma16237461
Zhang, X., Yang, P., Dai, Y., Ma, P., Li, X., Cheng, Z., et al. (2013). Multifunctional Up-converting nanocomposites with smart polymer brushes gated mesopores for cell imaging and thermo/pH dual-responsive drug controlled release. Adv. Funct. Mater. 23 (33), 4067–4078. doi:10.1002/adfm.201300136
Zhou, J., Cui, Z., Zhang, B., Kundu, T., and Sevostianov, I. (2023). The effect of porosity on the elastic properties of cortical bone and ultrasound propagation. Int. J. Eng. Sci. 182, 103772. doi:10.1016/j.ijengsci.2022.103772
Zhou, S., Dong, X., Chen, C., Ma, L., Wu, Y., Zhou, Y., et al. (2021). Efficacy and safety of eculizumab for paroxysmal nocturnal hemoglobinuria: A systematic review and meta-analysis. J. Pediatr. Hematol. Oncol. 43 (6), 203–210. doi:10.1097/MPH.0000000000002178
Zhu, H., Monavari, M., Zheng, K., Distler, T., Ouyang, L., Heid, S., et al. (2022). 3D bioprinting of multifunctional dynamic nanocomposite bioinks incorporating Cu-doped mesoporous bioactive glass nanoparticles for bone tissue engineering. Small 18 (12), 2104996. doi:10.1002/smll.202104996
Keywords: bioprinting, gelatin, bioinks, scaffolds, bone, tissue engineering
Citation: Waidi YO, Kariim I and Datta S (2024) Bioprinting of gelatin-based materials for orthopedic application. Front. Bioeng. Biotechnol. 12:1357460. doi: 10.3389/fbioe.2024.1357460
Received: 18 December 2023; Accepted: 14 February 2024;
Published: 13 March 2024.
Edited by:
Vijayavenkataraman Sanjairaj, New York University Abu Dhabi, United Arab EmiratesReviewed by:
Annachiara Scalzone, Center for Advanced Biomaterials for Healthcare (IIT), ItalyArindam Chakraborty, Adamas University, India
Indumathi Sathisaran, National Institute of Technology Calicut, India
Copyright © 2024 Waidi, Kariim and Datta. This is an open-access article distributed under the terms of the Creative Commons Attribution License (CC BY). The use, distribution or reproduction in other forums is permitted, provided the original author(s) and the copyright owner(s) are credited and that the original publication in this journal is cited, in accordance with accepted academic practice. No use, distribution or reproduction is permitted which does not comply with these terms.
*Correspondence: Sudipto Datta, c3VkaXB0b2RhdHRhMTk5MEBnbWFpbC5jb20=