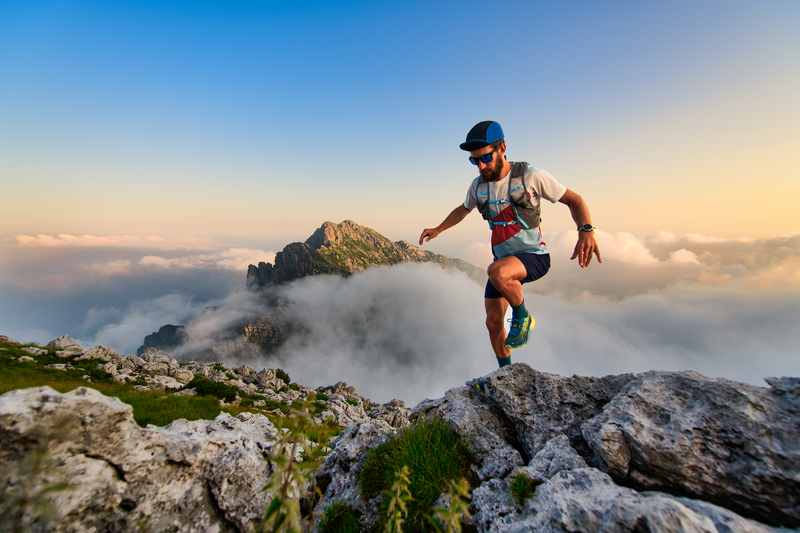
95% of researchers rate our articles as excellent or good
Learn more about the work of our research integrity team to safeguard the quality of each article we publish.
Find out more
ORIGINAL RESEARCH article
Front. Bioeng. Biotechnol. , 25 June 2024
Sec. Biomechanics
Volume 12 - 2024 | https://doi.org/10.3389/fbioe.2024.1357182
The number of lung transplantations is limited due to the shortage of donor lungs fulfilling the standard criteria. The ex vivo lung perfusion (EVLP) technique provides the ability of re-evaluating and potentially improving and treating marginal donor lungs. Accordingly, the technique has emerged as an essential tool to increase the much-needed donor lung pool. One of the major EVLP protocols, the Lund protocol, characterized by high pulmonary artery flow (100% of cardiac output [CO]), an open atrium, and a cellular perfusate, has demonstrated encouraging short-EVLP duration results. However, the potential of the longer EVLP duration of the protocol is yet to be investigated, a duration which is considered necessary to rescue more marginal donor lungs in future. This study aimed to achieve stable 8-h EVLP using an open-atrium cellular model with three different pulmonary artery flows in addition to determining the most optimal flow in terms of best lung performance, including lung electrolytes and least lung edema formation, perfusate and tissue inflammation, and histopathological changes, using the porcine model. EVLP was performed using a flow of either 40% (n = 6), 80% (n = 6), or 100% (n = 6) of CO. No flow rate demonstrated stable 8-h EVLP. Stable 2-h EVLP was observed in all three groups. Insignificant deterioration was observed in dynamic compliance, peak airway pressure, and oxygenation between the groups. Pulmonary vascular resistance increased significantly in the 40% group (p < .05). Electrolytes demonstrated an insignificant worsening trend with longer EVLP. Interleukin-8 (IL-8) in perfusate and tissue, wet-to-dry weight ratio, and histopathological changes after EVLP were insignificantly time dependent between the groups. This study demonstrated that stable 8-h EVLP was not feasible in an open-atrium cellular model regardless of the flow of 40%, 80%, or 100% of CO. No flow was superior in terms of lung performance, lung electrolytes changes, least lung edema formation, minimal IL-8 expression in perfusate and tissue, and histopathological changes.
Lung transplantation is an established treatment for non-malignant end-stage pulmonary disease (Aigner et al., 2012). However, the intervention remains limited due to the scarcity of donor lungs meeting the standard criteria for transplantation (Aigner et al., 2012). Ex vivo lung perfusion (EVLP) has emerged as a novel method to expand the donor pool by evaluating, improving, and treating marginal donor lungs that otherwise would have been rejected (Ali et al., 2023).
Currently, there are three different EVLP systems and protocols—Lund, Toronto, and Organ Care System—used in clinical settings (Steen et al., 2007; Cypel et al., 2008; Loor et al., 2017; Sommer et al., 2019). Of these, two of the major protocols, Lund and Toronto, are applied in majority of the institutions (Steen et al., 2007; Cypel et al., 2008). The Toronto protocol, characterized by low pulmonary artery flow (40% of cardiac output [CO]), a closed atrium, and an acellular perfusate (LCAA), is the most comprehensively studied and continuously evolving protocol with promising results after short (4 h in human lungs) and long durations of EVLP (12–24 h to 3 days in pig lungs) (Cypel et al., 2008; Yeung et al., 2017; Takahashi et al., 2021; Ali et al., 2022). In contrast, the Lund protocol, distinguished by high pulmonary artery flow (100% of CO), an open atrium, and a cellular perfusate (HOAC), has demonstrated encouraging results after a short period of EVLP (1–4 h in human and pig lungs); however, the potential of an effective longer EVLP duration needs to be further investigated (Erasmus et al., 2006; Niikawa et al., 2020). There is no consensus as to which strategy is the most beneficial in terms of post-EVLP lung performance, least edema formation, perfusate and tissue inflammation, histopathological changes, and electrolyte balance in the perfusate, which has been associated with lung function deterioration, particularly pertaining to prolonged EVLP (Koike et al., 2011; Lin et al., 2018; Nilsson et al., 2018). However, one study has demonstrated no difference between the strategies, although with slightly more pronounced lung edema in the LCAA model in a pig model (Nilsson et al., 2018). One pivotal difference, which has been persistently debated between these two strategies, is the pulmonary artery flow at which lungs are perfused with no clarification of the most optimal EVLP perfusate flow (Nilsson et al., 2018; Beller et al., 2020). Nevertheless, there is a growing recognition that the future EVLP system, including perfusate flow, needs to facilitate prolonged lung preservation in order to utilize advanced diagnostics to predict transplant outcome and perform therapeutic interventions within the platform so that more marginal lungs can be rescued (Ali et al., 2023). While the LCAA model continues to successfully attain some of these objectives (Cypel et al., 2008; Yeung et al., 2017; Takahashi et al., 2021; Ali et al., 2022), the ability of the HOAC protocol has yet to demonstrate advancement toward prolonged lung preservation (Erasmus et al., 2006; Niikawa et al., 2020).
The purpose of this experimental study was to use the Vivoline LS1 system with the open-atrium cellular EVLP strategy in order to 1) determine the potential of 8-h EVLP and 2) examine solely the effect of three different perfusate flows (40%, 80%, and 100% of estimated CO) on the lung performance, including lung electrolytes, lung edema formation, perfusate and tissue inflammation, and histopathological changes, using the porcine model.
Domestic female pigs (50–60 kg) were used under an experimental protocol approved by the Institutional Animal Care and Use Committee (IACUC) at the University of Copenhagen, 2021-15-0201-01045. The pigs were housed in an animal facility affiliated with the University of Copenhagen, where they received care in compliance with the “Principles of Laboratory Animal Care,” formulated by “Danish Animal Research Legislation.”
All pigs were pre-medicated with Zoletil (Zoletil® Vet, Virbac, Denmark) (0.14 mL/kg). General anesthesia was achieved with 5 mg/kg 2,5% sodium pentobarbital (Pentothal, MTC Pharmaceuticals, Cambridge, Canada) intravenously (i.v.). Anesthesia was maintained with 15 mg/kg/h i.v. propofol (Propolipid® 10 mg/mL, Fresenius Kabi AB, Uppsala, Sweden) and 15 μg/kg/min i.v fentanyl (50 μg/mL, Hameln Pharma plus GMBH, Hameln, Saksa/Germany).
The pigs were intubated with an endotracheal tube of size 8.0 mm (Portex, Sims, Markham, Canada) through the mouth. Ventilation was performed using a volume-controlled ventilator (Servo Ventilator, Siemens-Elema Ab, Sweden). The fraction of inspired oxygen (FiO2) was 50%. The respiratory rate was adjusted to an end-tidal CO2 of 5–5.5 kPa. The tidal volume was set at 6–8 mL/kg, and positive end-expiratory pressure (PEEP) was set at 5 cm H2O. The baseline arterial blood gas measurements were obtained before sternotomy.
After sternotomy, main pulmonary artery (PA) cannulation, and washed red blood cell collection, the aorta was clamped upon arrhythmia. The PA was antegradely perfused with 2 L of cold Perfadex (Perfadex, XVIVO Perfusion AB, Gothenburg, Sweden). At this stage, the pigs were pronounced dead (cardiac arrest with no cardioplegia and no volume/hemodynamic and ventilation resuscitation). The lungs were harvested, weighted, stored in cold saline, and kept on ice. After 2 h of cold ischemia, the lungs were cannulated and connected to the EVLP circuit. Washed red blood cells were prepared in a cell saver after exsanguination. The pigs were randomly assigned each day to an EVLP target flow of either 40% of CO (used in the Toronto protocol) (n = 6), 80% of CO (chosen arbitrary) (n = 6), or 100% of CO (used in the Lund protocol) (n = 6) (section details are given in Supplementary Material S1A).
Vivoline LS1 (Vivoline Medical AB, Lund, Sweden) was used for this experiment (Figure 1). The system was primed with 2 L of STEEN solution (XVIVO Perfusion AB, Gothenburg, Sweden), heparin, meropenem, methylprednisolone, and washed red blood cells to a hematocrit level of 10%–15% (Supplementary Material S1B). Rewarming of the lungs with a target temperature of 37°C was commenced after de-airing of the PA cannula and the subsequent connection of the lungs to the circuit. The antegrade flow was initiated at 10% of estimated CO (pigs: CO = 70 mL/min/kg (Wallinder et al., 2014)), with a gradual increase to either 40% , 80%, or 100% of estimated CO for the reconditioning phase at FiO2 0.5 at 37°C (Table 1). Lung evaluation was performed at FiO2 1.0 every 2 h, while evaluation with FiO2 0.21 was conducted in the last hour before EVLP termination. Ventilation was started at 32°C, with incremental changes in the ventilatory parameters according to the temperature and EVLP phases (Table 1). The baseline lung parameters (dynamic compliance [DC], pulmonary vascular resistance [PVR], peak airway pressure [PawP], and oxygenation) and perfusate gasses (pH, sodium, potassium, lactate, and glucose) were taken after 10 min of target flow (hour 0), with subsequent hourly reconditioning assessments (Table 1). The lung parameters and perfusate gasses were evaluated every 2 h (Table 1). If needed, perfusate gasses were corrected on an hourly basis as follows: pH was maintained between 7.35 and 7.45 with 1 mL of isotonic trometamol (Addex-THAM 20 mL, Fresenius Kabi AB, Uppsala, Sweden) for every unit below zero in the base excess (Wallinder et al., 2014). Calcium was maintained at >3 mmol/L with 10 mL calcium gluconate. Glucose was maintained at >5 mmol/L with 100 mg/mL glucose (Fresenius Kabi). All the lung function parameters were recorded from the EVLP and respiratory monitors.
Figure 1. Schematic diaphragm of the components in a Vivoline LS1 circuit (Vivoline Medical AB, Lund, Sweden). PA, pulmonary artery; LA, left atrium; HCU, heater–cooler unit; TP, temperature probe.
A successful prolonged EVLP was pre-defined as 8 h if both of the following criteria were met: 1) DC > 15 mL/cm H2O, which was also considered a parameter of stable EVLP, and 2) an achievable target perfusion flow. If none of these two parameters were accomplished during the reconditioning hour, a decision was made to terminate the EVLP toward the end of that hour before the evaluation phase. EVLP was continued if one of these parameters was feasible until the 8-h mark in order to observe the trends.
Perfusate samples for cytokine analysis were collected every hour from the left atrium port and stored at −80°C until analysis. Both the lung sides and all lobes were used to collect the lung tissue for cytokine analysis and microscopic evaluation. Once a lung side and a lobe were chosen arbitrarily for each case, the lung tissues were collected from the same lung side and lobe before and after the EVLP. One portion of the collected lung tissue (before and after) was snap-frozen on dry ice and stored at −80°C for cytokine analysis. A separate portion of the collected lung tissue (before and after) was fixated in formalin for microscopic evaluation.
The lung samples were fixed in 10% buffered formalin for 24 h, embedded in paraffin, sectioned into a thickness of 5 µm, and stained by hematoxylin and eosin before the evaluation of pathological changes under light microscopy. Histopathologic grading of lung injury was assessed using the parameters of interstitial edema, intra-alveolar edema, arteriolar thickening, vascular thrombosis, hemorrhage, and cell infiltration (Medeiros et al., 2012). The severity of these findings was graded on a 4-point scale as follows: 0, absent; 1, mild; 2, moderate; and 3, severe (Medeiros et al., 2012). The tissue slices were blindly evaluated by two independent reviewers (HK and SNB). Any discrepancies were resolved through consensus.
Immediately after EVLP termination, a 1 × 1 × 1-cm biopsy was taken from the right or left lower lobe. The ratio of the weights of the portions before and after drying (60°C in an oven for 24 h) was calculated to evaluate lung edema after EVLP (Wallinder et al., 2014).
Inflammatory markers in the perfusate and lung tissue were measured using commercially available enzyme-linked immunosorbent assay (ELISA) kits for porcine cytokine interleukin (IL)-8, IL-10, tumor necrosis factor-⍺ (TNF-⍺), and hypoxia inducible factor-1⍺ (HIF-1⍺). The kits were used according to the manufacturer’s instructions (section details are given in Supplementary Material S1C).
Continuous data are presented as the mean ± standard deviation or standard error of the mean. Analysis of variance (ANOVA) was performed to compare the differences among more than two groups. When the statistical significance was indicated by ANOVA, a pair-wise post hoc test (Tukey’s test) was performed to identify the differences between the groups. Changes within the groups were assessed by paired t-tests. Perfusion hours were analyzed using Kaplan–Meier and log-rank tests. Statistical analysis was derived from the time of the start of EVLP (hour 0) till the EVLP termination of each lung. To address the truncated data variation, each outcome was divided by its EVLP hour, followed by the average analysis of each group. A p-value <0.05 was considered statistically significant. All statistical analyses were performed in RStudio (version 2022.07.2).
No significant difference in pig body weight (40%: 54.7 ± 4.5 kg; 80%: 51.2 ± 1.9 kg; and 100%: 54.5 ± 2.1 kg; p = 0.9) and arterial PO2/FiO2 at lung retrieval (40%: 592.0 ± 40.6 mmHg; 80%: 608.3 ± 40.5 mmHg; and 100%: 522.0 ± 82.6 mmHg; p = 0.06)) was found between the groups. Perfusion hours based solely on the ability to run the target perfusate flow were 6.17 ± 2.23 in 40%, 4.17 ± 2.4 in 80%, and 3.67 ± 1.9 in the 100% group (p = 0.10) (Figure 2). Stable perfusion hours were only observed in the first 2 h in all groups (Figures 2, 3).
Figure 2. Perfusion hours were 8, 8, 4, 8, 6, and 3 h in the 40% group; 4, 3, 2, 2, 6, and 8 h in the 80% cohort; and 2, 5, 6, 2, 2, and 5 h in the 100% group (recorded as 8-h time points in all groups). EVLP, ex vivo lung perfusion; %, percent.
Figure 3. Lung parameters during EVLP perfusion in 40% (n = 6), 80% (n = 6), and 100% (n = 6) flow rate groups with standard error of the mean. (A) Dynamic compliance, (B) pulmonary vascular resistance, (C), peak airway pressure, and (D) oxygenation. *, Significance between the groups using Tukey’s test (40% vs. 80% and 100%: p < .05; 80% vs. 100%: p = 0.02). p, p-value; EVLP, ex vivo lung perfusion; %, percent.
The lung parameters were stable in the first 2 h, followed by a worsening trend throughout the EVLP period in all the groups (Figures 3A–D). No group achieved stable 8-h EVLP as DC was observed to insignificantly decrease <15 mL/cm H2O after 2 h in all the groups (p = 0.66) (Figure 3A). PVR increased significantly between all the groups, with the highest increase in the 40% cohort (Tukey’s test: 40% vs. 80% and 100% groups, p < 0.05) (Figure 3B). A similar increasing trend was demonstrated by PawP in all the groups (p = 0.73) (Figure 3C). Oxygenation was insignificantly stable for the first 4 hours, with a subsequent decrease in all the groups (p = .28) (Figure 3D). All lung parameters were insignificantly exacerbated in all the groups with a FiO2 increase (50%–100%) and decrease (50%–21%).
Corresponding electrolyte concentrations were also noted to aggravate in all the groups (Figures 4A–E). The pH, lactate, sodium, and potassium values increased insignificantly between the groups (p = 0.05, p = 0.61, p = 0.71, and p = 0.65, respectively [Figures 4A–D]), whereas the glucose values decreased insignificantly in all the groups (p = 0.46) (Figure 4E).
Figure 4. Trend of electrolyte concentration during EVLP perfusion in 40% (n = 6), 80% (n = 6), and 100% (n = 6) flow groups with standard error of the mean. (A) pH, (B) lactate, (C) sodium, (D) potassium, and (E) glucose. EVLP, ex vivo lung perfusion; %, percent.
Pro-inflammatory IL-8 levels in the perfusate and tissue demonstrated a time-dependent increase, with no difference between or within the groups (Figures 5A, B). Due to the repeated gross difference in ELISA duplicate testing of IL-10, TNF, and HIF concentration levels, no reliable statistical analysis could be performed for these cytokines.
Figure 5. (A) Hourly pro-inflammatory IL-8 levels in the perfusate with standard error of the mean, and (B) mean changes in IL-8 production in lung tissue from baseline (H0) and end of EVLP (HT) in 40% (n = 6), 80% (n = 6), and 100% (n = 6) flow groups. Error bars represent standard error values. IL-8, interleukin-8; EVLP, ex vivo lung perfusion; p, p-value.
Edema formation was visibly attenuated in all the groups after EVLP (Figures 6A–C). The wet-to-dry weight ratio (WDR) increased in all three groups after EVLP, although it was time-dependently slightly higher in the 40% group (p = 0.73) (Figures 7A, B). Time-dependent cumulative pathological scoring of lung injury demonstrated no significant difference between or within the groups before and after EVLP (Figures 8A–C).
Figure 6. Appearance of lungs before (B) and after (A) 6-h EVLP in the (A) 40% flow rate group, (B) 80% flow rate group, and (C) 100% flow rate group. All lungs were perfused in a supine position. EVLP, ex vivo lung perfusion.
Figure 7. Mean wet-to-dry weight ratio of the perfused lungs in 40% (n = 6), 80% (n = 6), and 100% (n = 6) flow groups before (A) and after (B) the EVLP. Error bars represent standard error values. p, p-value; %, percent.
Figure 8. General comparison of histopathological lung injury before and after EVLP between the (A) 40% flow rate group, (B) 80% flow rate group, and (C) 100% flow rate group. ◆, arteriolar thickening, cell infiltration, (arrow) hemorrhage, and *, alveolar. EVLP, ex vivo lung perfusion.
Notably, results from 3-h EVLP and onward are cautiously interpreted on account of the conspicuous variation in the number of pigs between the groups.
The mounting body of evidence demonstrates reproducible stable prolonged EVLP with the LCAA strategy (Cypel et al., 2008; Yeung et al., 2017; Takahashi et al., 2021; Ali et al., 2022). This is in contrast to the HOAC approach, where the potential of stable prolonged EVLP remains undetermined with limited robust and consistent data (Erasmus et al., 2006; Niikawa et al., 2020). The longest assessed HOAC EVLP duration is 6 h in porcine lungs, with results indicating high PVR and maximum ventilation pressure, suggestive of lung injury (Erasmus et al., 2006). However, unpublished data of HOAC EVLP have been cited to perfuse porcine lungs for 8 h without lung edema formation, but conclusive evidence is yet to be reported (Wierup et al., 2006). In the present study, stable 8-h perfusion was not feasible with either lower perfusate flow (LPF) (40%) or higher perfusate flow (HPF) (80% and 100%). All three perfusate flows demonstrated deteriorating trends in DC, PVR, PawP, and oxygenation, which is suggestive of severe lung injury. Several deleterious factors may have contributed to damage the integrity of the lung structure. In the HPF groups, the most plausible explanation may be the exposure of excessive hydrostatic pressure, which encouraged mechanistic damage of pulmonary vasculature, resulting in greater fluid permeability, thus leading to advanced lung edema formation (Okamoto et al., 2019; Beller et al., 2020). This mechanism has led to the adoption of LPF in the LCAA strategy (Cypel et al., 2008). Whether LPF alone has beneficial effects on prolonging LCAA EVLP success is not investigated; however, studies have demonstrated that even lower perfusate flow (20% of CO) in the LCAA system may further reduce lung edema (Beller et al., 2020). In the present study, reducing the perfusate flow was equally inferior as HPFs in terms of DC, PawP, and oxygenation. Interestingly, PVR as an isolated parameter became significantly higher in the LPF group than in the HPF groups. These findings may be because the lower flow in combination with the open atrium creates a cyclical open–close phenomenon at the capillary level with ventilation exacerbated at low after-load pressures, leading to altered permeability and edema formation (Broccard et al., 2002; Peták et al., 2002; Linacre et al., 2016). Another potential explanation may be that the absence of venous after-load pressure in the pulmonary circulation altered the distribution in the lung perfusion zones with either the lack or overflow of the perfusion, thus contributing to the damage of the lung (Peták et al., 2002; Linacre et al., 2016). Interestingly, our LPF results are in contrast with those of limited previous studies demonstrating stable lung parameters for more than 8 h in a low-flow, open-atrium, cellular (LOAC) strategy (50% of CO) (Loor et al., 2017; Sommer et al., 2019). This may be because these studies used an EVLP system (Organ Care System), which is notably different in terms of the circulatory, ventilatory, and priming setups from a Vivoline LS1 system. In addition, their reconditioning protocols (replenishment regime and recruitment maneuverers) are significantly diverse, which may explain their favorable outcomes compared to our study. Nevertheless, our LPF findings are supported by another study that demonstrated an increase in PVR and peak inspiratory pressure and a decrease in compliance and oxygenation in a setting of LPF with an open atrium compared to a closed atrium (Linacre et al., 2016). However, an increase in PVR alone in our LPF group cannot be fully delineated, especially given that the LPF group was the only group in which most lungs reached unstable 8-h EVLP. One may explain this contradiction with insufficient generation of PVR in these specific lungs to obstruct the target flow. However, the exact mechanism behind it cannot be thoroughly elucidated except the known fact that PVR determination is based on the perfusate flow rate, which may have influenced the higher PVR in the lower perfusate flows.
Preserved lung viability after short EVLP durations has been demonstrated in LCAA and HOAC strategies with promising results after transplantation (Wierup et al., 2006; Cypel et al., 2011; Henriksen et al., 2014; Nilsson et al., 2019; Buttar et al., 2024). In our study, lung parameters were within acceptable values in the first 2 h, suggesting a stable 2-h EVLP. However, deterioration was already apparent during the second hour in all perfusate flows, indicating edema formation with a continuing decrease in lung performance in the subsequent hours. These findings are in line with the fact that the HOAC model was proposed as a method to evaluate marginal lungs within a short duration (1–2 h) of EVLP due to the concern of lung damage associated with concomitant high hydrostatic pressure (Steen et al., 2007; Okamoto et al., 2019; Beller et al., 2020). Nevertheless, 4-h stable EVLP with the HOAC model in pig lungs and 4-h stable EVLP with the LPF open-atrium cellular model in human lungs have been demonstrated (Valenza et al., 2014; Nilsson et al., 2018). Interestingly, in the present study, oxygenation as an isolated parameter was found to be stable for 4 h. This may indicate that oxygenation alone may not be a reliable indicator of lung viability. It has been demonstrated that lung assessment conducted solely based on oxygenation can be misleading as its deterioration can be delayed in an ex vivo setting (Yeung et al., 2012). Therefore, an evaluation of other physiologic parameters such as DC, PawP, and PVR is crucial to obtain a full picture of the functional capacity of the lungs (Cypel et al., 2009; Yeung et al., 2012; Spratt et al., 2017).
As all perfusate flows have performed equally unsatisfactorily in the present study, one may hypothesize on the lack of deleterious underlying factors. One of them could have been that cold ischemia time (CIT) of 2 h in a prolonged EVLP set point was not sufficient in terms of induced lung injury. To date, no pig study has compared different perfusate flows with 2-h CIT using the open-atrium cellular strategy in a prolonged time-point EVLP. However, studies with 2-h CIT, LPF, and 4-h cellular EVLP have demonstrated no difference in the development of lung edema compared with acellular perfusate (Roman et al., 2015). The same results have been demonstrated by studies with 24-h CIT, HPF, and 12-h cellular EVLP compared with acellular perfusate (Becker et al., 2016; Steinmeyer et al., 2018). This indicates that CIT duration may not influence the true difference if all cases have been subjected to the same CIT duration regardless of EVLP duration, perfusate flow, or perfusate composition. Thus, our results cannot be explained by a short 2-h CIT as it is sufficiently harmful injury to the lungs. Nevertheless, longer CIT hours have been associated with diminishing reconditioning potential due to severe ischemia reperfusion injury (IRI) (Steinmeyer et al., 2018; Okamoto et al., 2019).
Another general key difference between LCAA and HOAC strategies is that perfusate in the LCAA model is periodically replenished, whereas the HOAC setup corrects perfusate composition in an hourly manner (Steen et al., 2007; Cypel et al., 2008). However, there is one study with the LOAC strategy that has also implemented a replenishment regime with promising results (Loor et al., 2017). In the present study, electrolytes demonstrated a steady deterioration in an hourly manner in short and long EVLP duration in all groups. These results may be because perfusate correction during the EVLP was not sufficiently attained despite hourly correction with an acceptable follow-up gas analysis. This suggests that perfusate components are consumed rapidly with longer EVLP; thus, the need for perfusate correction should be assessed multiple times within an hour and not be limited by one acceptable hourly correction, as demonstrated in a previous study (Sommer et al., 2019). Nevertheless, deterioration of electrolytes in prolonged EVLP is inevitable even with standard replenishment of the perfusate in the LCAA model, although the exacerbation is observed after 12 h (Cypel et al., 2008). Considering this factor, periodical replenishment of the perfusate appears to play one of the key roles in enabling stable prolonged EVLP rather than correcting electrolytes (Cypel et al., 2008; Loor et al., 2017). A recent study from the LCAA model group focuses on further stabilization of homeostasis with a demonstration of even longer stable EVLP, thus emphasizing the significance of sufficient perfusate constituents (Takahashi et al., 2021).
In lung IRI, immune cells are induced to generate inflammatory cytokines, which increase capillary permeability, resulting in lung edema (de Perrot et al., 2003; Den et al., 2010). In this study, pro-inflammatory cytokine (IL-8) in perfusate and tissue demonstrated a time-dependent increase in all groups with no difference between the groups. The increase in the IL-8 levels may correspond to a severe increase in capillary permeability and, thus, lung edema, as observed in all the groups. Previous studies with the HOAC model have demonstrated similar results; however, there is no prior evidence of the IL-8 trend in the low-flow cellular model (Nilsson et al., 2018; Okamoto et al., 2019). Nevertheless, IL-8 in the low-flow acellular setup demonstrates results similar to that of our low-flow cellular group (Nilsson et al., 2018), which is in line with severe edema formation supported by time-dependent EVLP results of WDR and post-EVLP histology between the groups.
The main limitation of this study is the discrepancy in EVLP hours between the groups, in particular, the difference in the number of remaining lungs on EVLP after 2 h. This inconsistency results in an inequitable comparison of the groups after 2 h. Given that one of the main purposes of the study was to demonstrate the potential of 8-h EVLP with the open-atrium cellular technique, we found the necessity to analyze data based on the cumulative EVLP hours of each group. Nonetheless, 2-h trends between the groups have been noted. Another limitation of this study is the small number of cases in addition to the lack of blinded randomization. Thus, our study does not provide definitive evidence of the infeasibility of 8-h EVLP and no difference in the development of lung edema between the three compared perfusate flows using the open-atrium cellular EVLP strategy. Lastly, the lungs were only flushed antegradely with 2 L of Perfadex, which may have had an adverse effect on the outcomes. Nevertheless, considering that all three flow groups were subjected to the same amount of Perfadex, one may have equalized the outcome effect across the groups.
In conclusion, 8-h EVLP is not feasible in an open-atrium cellular pig model in the presented EVLP setup regardless of perfusate flow of 40%, 80%, or 100% of CO. Nevertheless, this study demonstrates a stable 2-h EVLP within all three perfusate groups. No perfusate flow is superior in terms of lung performance, lung electrolyte changes, minimal IL-8 expression in perfusate and tissue, detrimental histopathological changes, and lung edema formation. Modifications in the applied EVLP protocol, in particular, the addition of a replenishment regime, may improve the ability of longer EVLP duration with better post-EVLP outcomes. Studies with equivalent long EVLP hours in each flow group are required to determine the true difference in the measured parameters between the perfusate flows.
The raw datasets used and/or analysed during the current study are available from the corresponding author on reasonable request.
The animal study was approved by the Animal Care Committee at the University of Copenhagen, 2021-15-0201-01045. The animals received care in compliance with the “Principles of Laboratory Animal Care” formulated by the Danish animal research legislation. The study was conducted in accordance with the local legislation and institutional requirements.
SB: conceptualization, data curation, formal analysis, investigation, methodology, project administration, resources, software, visualization, writing–original draft, and writing–review and editing. HM-S: supervision, validation, and writing–review and editing. MP: supervision, validation, and writing–review and editing. HK: supervision, validation, and writing–review and editing. TL: supervision, validation, and writing–review and editing. RP: supervision, validation, and writing–review and editing. CM: supervision, validation, and writing–review and editing.
The author(s) declare that financial support was received for the research, authorship, and/or publication of this article. The funding body was XVIVO perfusion who provided STEEN solution, EVLP circuits for the experiments, and a grant for the primary investigator of the study (SB). They played no role in the study design, data collection, analyses, interpretation, or decision to publish the manuscript.
The authors thank associate professor Julie L. Forman from Section of Biostatistics, University of Copenhagen, for assistance with statistical analysis.
The authors declare that the research was conducted in the absence of any commercial or financial relationships that could be construed as a potential conflict of interest.
All claims expressed in this article are solely those of the authors and do not necessarily represent those of their affiliated organizations, or those of the publisher, the editors, and the reviewers. Any product that may be evaluated in this article, or claim that may be made by its manufacturer, is not guaranteed or endorsed by the publisher.
The Supplementary Material for this article can be found online at: https://www.frontiersin.org/articles/10.3389/fbioe.2024.1357182/full#supplementary-material
HOAC, High pulmonary artery flow, an Open Atrium, and a Cellular perfusate; HPF, high perfusate flow; LCAA, Low pulmonary artery flow, a Closed Atrium, and a Acellular perfusate; LOAC, Low pulmonary artery flow, an Open Atrium, and a Cellular perfuse; LPF, Low Perfusate Flow.
Aigner, C., Slama, A., Hötzenecker, K., Scheed, A., Urbanek, B., Schmid, W., et al. (2012). Clinical ex vivo lung perfusion—pushing the limits. Am. J. Transplant. 12 (7), 1839–1847. doi:10.1111/j.1600-6143.2012.04027.x
Ali, A., Keshavjee, S., and Cypel, M. (2023). THE PRESENT AND FUTURE OF EX-VIVO LUNG PERFUSION. Eur. J. Transplant. 1, 21–27. doi:10.57603/ejt-005
Ali, A., Nykanen, A. I., Beroncal, E., Brambate, E., Mariscal, A., Michaelsen, V., et al. (2022). Successful 3-day lung preservation using a cyclic normothermic ex vivo lung perfusion strategy. EBioMedicine 83, 104210. doi:10.1016/j.ebiom.2022.104210
Becker, S., Steinmeyer, J., Avsar, M., Höffler, K., Salman, J., Haverich, A., et al. (2016). Evaluating acellular versus cellular perfusate composition during prolonged ex vivo lung perfusion after initial cold ischaemia for 24 hours. Transpl. Int. 29 (1), 88–97. doi:10.1111/tri.12649
Beller, J. P., Byler, M. R., Money, D. T., Chancellor, W. Z., Zhang, A., Zhao, Y., et al. (2020). Reduced-flow ex vivo lung perfusion to rehabilitate lungs donated after circulatory death. J. Heart Lung Transplant. 39 (1), 74–82. doi:10.1016/j.healun.2019.09.009
Broccard, A. F., Vannay, C., Feihl, F., and Schaller, M. D. (2002). Impact of low pulmonary vascular pressure on ventilator-induced lung injury. Crit. care Med. 30 (10), 2183–2190. doi:10.1097/00003246-200210000-00002
Buttar, S. N., Schultz, H. H. L., Møller-Sørensen, H., Perch, M., Petersen, R. H., and Møller, C. H. (2024). Long-term outcomes of lung transplantation with ex vivo lung perfusion technique. Front. Transplant. 3, 1324851. doi:10.3389/frtra.2024.1324851
Cypel, M., Rubacha, M., Yeung, J., Hirayama, S., Torbicki, K., Madonik, M., et al. (2009). Normothermic ex vivo perfusion prevents lung injury compared to extended cold preservation for transplantation. Am. J. Transplant. 9 (10), 2262–2269. doi:10.1111/j.1600-6143.2009.02775.x
Cypel, M., Yeung, J. C., Hirayama, S., Rubacha, M., Fischer, S., Anraku, M., et al. (2008). Technique for prolonged normothermic ex vivo lung perfusion. J. heart lung Transplant. 27 (12), 1319–1325. doi:10.1016/j.healun.2008.09.003
Cypel, M., Yeung, J. C., Liu, M., Anraku, M., Chen, F., Karolak, W., et al. (2011). Normothermic ex vivo lung perfusion in clinical lung transplantation. N. Engl. J. Med. 364 (15), 1431–1440. doi:10.1056/nejmoa1014597
Den, H. W. A., Gielis, J. F., Lin, J. Y., Van Schil, P. E., De Windt, L. J., and Moens, A. L. (2010). Lung ischemia-reperfusion injury: a molecular and clinical view on a complex pathophysiological process. Am. J. Physiology-Heart Circulatory Physiology 1. doi:10.1152/ajpheart.00251.2010
de Perrot, M., Liu, M., Waddell, T. K., and Keshavjee, S. (2003). Ischemia–reperfusion–induced lung injury. Am. J. Respir. Crit. care Med. 167 (4), 490–511. doi:10.1164/rccm.200207-670so
Erasmus, M. E., Fernhout, M. H., Elstrodt, J. M., and Rakhorst, G. (2006). Normothermic ex vivo lung perfusion of non-heart-beating donor lungs in pigs: from pretransplant function analysis towards a 6-h machine preservation. Transpl. Int. 19 (7), 589–593. doi:10.1111/j.1432-2277.2006.00318.x
Henriksen, I. S., Møller-Sørensen, H., Møller, C. H., Zemtsovski, M., Nilsson, J. C., Seidelin, C. T., et al. (2014). First Danish experience with ex vivo lung perfusion of donor lungs before transplantation. Dan. Med. J. 61 (3), A4809.
Koike, T., Yeung, J. C., Cypel, M., Rubacha, M., Matsuda, Y., Sato, M., et al. (2011). Kinetics of lactate metabolism during acellular normothermic ex vivo lung perfusion. J. heart lung Transplant. 30 (12), 1312–1319. doi:10.1016/j.healun.2011.07.014
Lin, H., Chen, M., Tian, F., Tikkanen, J., Ding, L., Cheung, H. Y., et al. (2018). α1-Anti-trypsin improves function of porcine donor lungs during ex-vivo lung perfusion. J. Heart Lung Transplant. 37 (5), 656–666. doi:10.1016/j.healun.2017.09.019
Linacre, V., Cypel, M., Machuca, T., Nakajima, D., Hashimoto, K., Zamel, R., et al. (2016). Importance of left atrial pressure during ex vivo lung perfusion. J. Heart Lung Transplant. 35 (6), 808–814. doi:10.1016/j.healun.2016.02.008
Loor, G., Howard, B. T., Spratt, J. R., Mattison, L. M., Panoskaltsis-Mortari, A., Brown, R. Z., et al. (2017). Prolonged EVLP using OCS lung: cellular and acellular perfusates. Transplantation 101 (10), 2303–2311. doi:10.1097/TP.0000000000001616
Medeiros, I. L., Pêgo-Fernandes, P. M., Mariani, A. W., Fernandes, F. G., do Vale Unterpertinger, F., Canzian, M., et al. (2012). Histologic and functional evaluation of lungs reconditioned by ex vivo lung perfusion. J. heart lung Transplant. 31 (3), 305–309. doi:10.1016/j.healun.2011.10.005
Niikawa, H., Okamoto, T., Ayyat, K. S., Itoda, Y., Sakanoue, I., Farver, C. F., et al. (2020). Cellular ex vivo lung perfusion beyond 1 hour may improve marginal donor lung assessment. J. Surg. Res. 250, 88–96. doi:10.1016/j.jss.2019.09.073
Nilsson, T., Gielis, J. F., Slama, A., Hansson, C., Wallinder, A., Ricksten, S. E., et al. (2018). Comparison of two strategies for ex vivo lung perfusion. J. Heart Lung Transplant. 37 (2), 292–298. doi:10.1016/j.healun.2017.07.001
Nilsson, T., Wallinder, A., Henriksen, I., Nilsson, J. C., Ricksten, S. E., Møller-Sørensen, H., et al. (2019). Lung transplantation after ex vivo lung perfusion in two Scandinavian centres. Eur. J. Cardio-Thoracic Surg. 55 (4), 766–772. doi:10.1093/ejcts/ezy354
Okamoto, T., Wheeler, D., Farver, C. F., and McCurry, K. R. (2019). Transplant suitability of rejected human donor lungs with prolonged cold ischemia time in low-flow acellular and high-flow cellular ex vivo lung perfusion systems. Transplantation 103 (9), 1799–1808. doi:10.1097/tp.0000000000002667
Peták, F., Habre, W., Hantos, Z., Sly, P. D., and Morel, D. R. (2002). Effects of pulmonary vascular pressures and flow on airway and parenchymal mechanics in isolated rat lungs. J. Appl. physiology 92 (1), 169–178. doi:10.1152/jappl.2002.92.1.169
Roman, M., Gjorgjimajkoska, O., Neil, D., Nair, S., Colah, S., Parmar, J., et al. (2015). Comparison between cellular and acellular perfusates for ex vivo lung perfusion in a porcine model. J. Heart Lung Transplant. 34 (7), 978–987. doi:10.1016/j.healun.2015.03.023
Sommer, W., Salman, J., Avsar, M., Hoeffler, K., Jansson, K., Siemeni, T. N., et al. (2019). Prediction of transplant outcome after 24-hour ex vivo lung perfusion using the Organ Care System in a porcine lung transplantation model. Am. J. Transpl. 19 (2), 345–355. doi:10.1111/ajt.15075
Spratt, J. R., Mattison, L. M., Iaizzo, P. A., Brown, R. Z., Helms, H., Iles, T. L., et al. (2017). An experimental study of the recovery of injured porcine lungs with prolonged normothermic cellular ex vivo lung perfusion following donation after circulatory death. Transpl. Int. 30 (9), 932–944. doi:10.1111/tri.12981
Steen, S., Ingemansson, R., Eriksson, L., Pierre, L., Algotsson, L., Wierup, P., et al. (2007). First human transplantation of a nonacceptable donor lung after reconditioning ex vivo. Ann. Thorac. Surg. 83 (6), 2191–2194. doi:10.1016/j.athoracsur.2007.01.033
Steinmeyer, J., Becker, S., Avsar, M., Salman, J., Höffler, K., Haverich, A., et al. (2018). Cellular and acellular ex vivo lung perfusion preserve functional lung ultrastructure in a large animal model: a stereological study. Respir. Res. 19 (1), 238–245. doi:10.1186/s12931-018-0942-5
Takahashi, M., Cheung, H. Y., Watanabe, T., Zamel, R., Cypel, M., Liu, M., et al. (2021). Strategies to prolong homeostasis of ex vivo perfused lungs. J. Thorac. Cardiovasc. Surg. 161 (6), 1963–1973. doi:10.1016/j.jtcvs.2020.07.104
Valenza, F., Rosso, L., Coppola, S., Froio, S., Palleschi, A., Tosi, D., et al. (2014). Ex vivo lung perfusion to improve donor lung function and increase the number of organs available for transplantation. Transpl. Int. 27 (6), 553–561. doi:10.1111/tri.12295
Wallinder, A., Hansson, C., Steen, S., Hussein, A. A., Sjöberg, T., and Dellgren, G. (2014). A simplified preservation method for lungs donated after cardiac death. J. Heart Lung Transplant. 33 (5), 528–535. doi:10.1016/j.healun.2014.01.854
Wierup, P., Haraldsson, Å., Nilsson, F., Pierre, L., Scherstén, H., Silverborn, M., et al. (2006). Ex vivo evaluation of nonacceptable donor lungs. Ann. Thorac. Surg. 81 (2), 460–466. doi:10.1016/j.athoracsur.2005.08.015
Yeung, J. C., Cypel, M., Machuca, T. N., Koike, T., Cook, D. J., Bonato, R., et al. (2012). Physiologic assessment of the ex vivo donor lung for transplantation. J. Heart Lung Transplant. 31 (10), 1120–1126. doi:10.1016/j.healun.2012.08.016
Keywords: ex vivo lung perfusion, cellular/acellular perfusate, open/closed atrium, prolonged ex vivo lung perfusion, ex vivo lung perfusion duration, ex vivo lung perfusion strategies
Citation: Buttar SN, Møller-Sørensen H, Perch M, Kissow H, Lilleør TNB, Petersen RH and Møller CH (2024) Porcine lungs perfused with three different flows using the 8-h open-atrium cellular ex vivo lung perfusion technique. Front. Bioeng. Biotechnol. 12:1357182. doi: 10.3389/fbioe.2024.1357182
Received: 17 December 2023; Accepted: 27 May 2024;
Published: 25 June 2024.
Edited by:
Jun Liao, University of Texas at Arlington, United StatesReviewed by:
Lin Yang, Helmholtz Association of German Research Centres (HZ), GermanyCopyright © 2024 Buttar, Møller-Sørensen, Perch, Kissow, Lilleør, Petersen and Møller. This is an open-access article distributed under the terms of the Creative Commons Attribution License (CC BY). The use, distribution or reproduction in other forums is permitted, provided the original author(s) and the copyright owner(s) are credited and that the original publication in this journal is cited, in accordance with accepted academic practice. No use, distribution or reproduction is permitted which does not comply with these terms.
*Correspondence: Sana N. Buttar, U2FuYWJ1dHRhckBob3RtYWlsLmNvbQ==
Disclaimer: All claims expressed in this article are solely those of the authors and do not necessarily represent those of their affiliated organizations, or those of the publisher, the editors and the reviewers. Any product that may be evaluated in this article or claim that may be made by its manufacturer is not guaranteed or endorsed by the publisher.
Research integrity at Frontiers
Learn more about the work of our research integrity team to safeguard the quality of each article we publish.