- Department of Orthopedics, Huashan Hospital, Fudan University, Shanghai, China
Osteoarthritis (OA) is the most common degenerative disease of the joint with irreversible cartilage damage as the main pathological feature. With the development of regenerative medicine, mesenchymal stem cells (MSCs) have been found to have strong therapeutic potential. However, intraarticular MSCs injection therapy is limited by economic costs and ethics. Exosomes derived from MSC (MSC-Exos), as the important intercellular communication mode of MSCs, contain nucleic acid, proteins, lipids, microRNAs, and other biologically active substances. With excellent editability and specificity, MSC-Exos function as a targeted delivery system for OA treatment, modulating immunity, inhibiting apoptosis, and promoting regeneration. This article reviews the mechanism of action of MSC-Exos in the treatment of osteoarthritis, the current research status of the preparation of MSC-Exos and its application of drug delivery in OA therapy.
1 Introduction
Osteoarthritis (OA) is one of the most prevalent forms of arthritis. Data from 2021 indicate that 22% of adults aged 40 and above suffer from knee OA. It is estimated that over 500 million individuals worldwide are affected by OA, making it the fourth leading cause of disability (Quicke et al., 2022). The substantial burden it places on healthcare resources, coupled with the loss of productivity due to unemployment and early retirement, imposes significant societal challenges. Osteoarthritis is a complex degenerative disease involving various components within the synovial joint. Its primary pathological features include the loss of articular cartilage, subchondral bone remodeling, inflammatory changes in synovial tissues, and instability of tendons and ligaments (Hunter and Bierma-Zeinstra, 2019). Among these, the irreversible damage to cartilage has long presented a therapeutic challenge.
Due to the avascular nature of cartilage tissue, its capacity for self-repair is severely limited. Mature chondrocytes have limited proliferative abilities, and the synthesis and metabolism of surrounding cartilage cells alone are insufficient to fill cartilage defects (Wakitani et al., 2004). Currently, there are few drugs available for the clinical treatment of cartilage injuries. Oral medications struggle to reach effective concentrations within joint cavities, and even intra-articular injections are quickly absorbed by lymphatic fluid, resulting in brief therapeutic effects and a heightened risk of infection (Patel et al., 2019). Therefore, research into more potent targeted therapies and effective drug delivery systems is of paramount importance.
In recent years, researchers have continuously developed novel biologics such as growth factors, anti-inflammatory agents, and Platelet-rich plasma (PRP) in an attempt to slow down the progression of OA by nourishing cartilage and suppressing intra-articular inflammatory responses (Hickey et al., 2003; Liang et al., 2022). Mesenchymal stem cells (MSCs) are a type of multipotent stem cell known for their self-renewal and multi-lineage differentiation capabilities. They can influence biological processes through paracrine effects, exhibiting anti-inflammatory, analgesic, and immune-modulating properties, have demonstrated promising results in cartilage repair research (Le et al., 2020).
Exosomes derived from MSCs (MSC-Exos) contain various proteins, mRNAs, and miRNAs, playing crucial roles in intercellular communication. Compared to MSCs, MSC-Exos offer advantages such as enhanced safety, ease of storage, minimal side effects, and fewer ethical concerns (Mendt et al., 2019). Advances in biotechnological techniques further harness their potential in drug delivery systems. This review focuses on the clinical prospects of MSC-Exos in OA treatment. It summarizes the biological characteristics, preparation methods, and explores their potential as a drug delivery system for OA therapy.
2 MSC and MSC-Exos in OA progression
Chondrocytes are the primary cellular components of articular cartilage, enclosed within a dense extracellular matrix (ECM). Under normal conditions, these cells maintain a delicate balance between ECM synthesis and degradation (Ruhlen and Marberry, 2014). However, this equilibrium can be disrupted by factors such as aging, metabolic changes, and mechanical stress, leading to a compromise in cartilage’s structural and functional integrity (Di Rosa et al., 2014). In the early stages of OA, chondrocytes function as environmental sensors within the joint, but their natural repair capacity is limited. Efforts to sustain ECM composition often involve transiently boosting ECM synthesis through pathological changes, such as chondrocyte hypertrophy, marked by increased expression of indicators like Runx2, MMP13, and CoIX. Consequently, these alterations stimulate the production of factors contributing to cartilage degeneration (Xu et al., 2014). While a small fraction of MSCs within the joint cavity plays a role in cartilage repair, their impact is relatively modest. Research has indicated that MSCs or synovial cells present in synovial fluid can be mobilized to the injury site under the influence of growth factors, aiding in cartilage defect repair. However, their effectiveness remains limited (Sekiya et al., 2012).
As the disease progresses, chondrocyte activity becomes dysregulated, hindering proliferation and synthetic metabolism. Cartilage defects worsen, extending to the subchondral bone. In this stage, local blood and MSCs infiltrate the area, forming a hematoma comprising fibrin clots, along with platelet, red blood cell, and white blood cell accumulations. MSCs, primarily derived from bone marrow, secrete various cytokines, including insulin-like growth factor 1, transforming growth factor-β, and platelet-derived growth factor, participating in the repair process (Hickey et al., 2003). Within 2 weeks of injury, MSCs transform into chondrocyte-like cells, synthesizing and secreting new ECM rich in type II collagen and proteoglycans. This tissue, resembling hyaline cartilage, fills the defect. Six to 8 weeks later, this newly formed tissue may transition from fibrous cartilage to hyaline cartilage. However, even if the defect is successfully filled, the resulting cartilage exhibits reduced hardness and low resistance to compression, making it vulnerable to further damage under mechanical loads. In the long term, neighboring chondrocytes may also be impacted, increasing the risk of osteoarthritis spreading beyond the initial localized area (Mobasheri et al., 2002).
OA is no longer regarded as a simple degenerative disease but rather a multifaceted condition involving a interplay of local and systemic factors (Hunter and Bierma-Zeinstra, 2019). The pathology extends across the entire joint, encompassing not only cartilage damage but also synovial membrane thickening, subchondral bone sclerosis, osteophyte formation, and structural alterations in ligaments and surrounding muscles. In the process of OA progression, the interactions between different tissues within the joints are crucial mechanisms in the pathophysiology of OA (Sharma et al., 2013). Mechanical stress between bone and cartilage, as well as synovial inflammation, affects cartilage degeneration (de Lange-Brokaar et al., 2012). Conversely, the altered cartilage cells impact other components within the joint. Extracellular vesicles (EVs) are recognized as vital mediators of intercellular communication. Their role in OA progression within the joints has gained increasing attention. Exosomes released by chondrocytes have a dual effect on OA. EVs secreted by healthy chondrocytes can regulate M2 macrophage penetration, facilitating the elimination of mitochondrial dysfunction and promoting immune recovery (Sun et al., 2019). However, EVs from OA chondrocytes promote autophagy mediated by miR-449a-5p/ATG4B, inhibiting chondrocyte proliferation, enhancing chondrocyte apoptosis, and upregulating the production of pro-inflammatory mediators by macrophages (Ni et al., 2019).
Numerous studies have demonstrated that exosomes derived from mesenchymal stem cells (MSCs) can slow down the progression of OA. Many scholars have proven that MSC-based OA therapy primarily operates through EVs, delivering substances including small RNAs. These substances regulate various biological activities such as chondrocyte apoptosis and senescence, synovial cell proliferation, and the release of pro-inflammatory mediators (Cosenza et al., 2017). EVs exhibit specific cell targeting properties based on their characteristics and sources, and they are biocompatible (Batrakova and Kim, 2015). Therefore, extensive research efforts have been made to delay OA development using MSC-Exos. Ongoing advancements in engineering techniques aim to develop drug delivery systems based on MSC-Exos, paving the way for new approaches in OA treatment.
3 Biological characteristics of MSC-Exos
3.1 Architecture of exosomes
Exosomes, with a diameter of about 40–100 nm, constitute a subset of biological nano-scale spherical lipid bilayer vesicles that have been found to be secreted by most cell types. The term “exosomes” was initially coined in 1981 by Trams et al. (1981), referring to vesicles derived from the plasma membrane. These vesicles were proposed to possess 5′-nucleotide enzyme activity, potentially serving physiological functions and originating from the extrusion of diverse cell line cultures (Harding et al., 1983). Distinguishing exosomes from other extracellular vesicles (EVs), such as microvesicles (MVs) and apoptotic bodies (ABs), can be challenging due to inherent heterogeneity both between and within exosome subtypes, along with overlapping characteristics. Notably, exosomes have been found to encapsulate a rich array of bioactive substances, including proteins, nucleic acids, lipids, cytokines, transcription factor receptors, and more (Théry et al., 2002). The composition of exosomal plasma membranes comprises a variety of lipids, encompassing hexosylceramides, cholesterol, phosphatidylserine, sphingomyelin, and saturated fatty acids (Skotland et al., 2019). Exosomal protein constituents can be broadly classified into two categories: common components, integral to vesicle formation and secretion, and specific components, intimately linked to the cell of origin. Common components encompass a range of proteins that play pivotal roles in processes such as membrane transport and fusion (e.g., Rab GTPases), heat shock responses (e.g.,,HSP70, HSP90), proteins belonging to the four-transmembrane protein superfamily (e.g.,,CD63, CD81), endosomal sorting complex required for transport (ESCRT)-related proteins (e.g., Tsg101, Alix), and integrins, etc. The other one is specific components, which are closely related to their progenitor cells including cell-specific markers like CD45 and MHC-II, which originate from antigen-presenting cells (Henne et al., 2011; Juan and Fürthauer, 2018).
3.2 Biogenesis of MSC-Exos
EVs biogenesis starts within the cell endosomal system. Unlike MVs and ABs, exosomes are not released directly from the plasma membrane, but are generated by inward budding of the multivesicular bodies (MVBs) membrane. MVBs referred to as late sorted endosomes (LEs), invagination of endosomal membranes occures during the maturation of early endsomes into LEs, results in the formation of intraluminal vesicles (ILVs) (Minciacchi et al., 2015). This process causes some cytoplasmic components to be engulfed and enclosed in ILVs, most of them are released outside the cell through fusion of MVBs with plasma membrane and part of them are transported to lysosomes for degradation. Those ILVs released into extracellular space are referred to as “exosomes” (Sahu et al., 2011). In 2010, Sai Kiang Lim’s team first demonstrated the therapeutic effects of MSCs through the secretion of exosomes (Lai et al., 2010). Subsequently, they confirmed that MSC-Exos also originate from endosomes and are rich in GM1 gangliosides, exhibiting high internalization activity (Tan et al., 2013). Further studies have consistently shown that MSC-Exos share similar biomarkers with exosomes derived from other cell sources like Alix, Tsg101, CD9, CD63 or CD81 (Kowal et al., 2016).
ESCRT consists of five functional subcomplexes (ESRCT-0,-I,-II,-III and AAA ATPase Vps4) were sequentially discovered to regulate the budding and release ILVs. Some studies suggest that ILVs can occur in MVBs without ESRCT, the biogenesis of exosomes can now be categorized into ESCRT-dependent and ESCRT-independent mechanisms (Henne et al., 2011). The role of the RAB family of small GTPase proteins, including Rab27a, Rab27b, Rab35, and Rab7, in facilitating vesicular transport between intracellular compartments is well-established. These proteins have also been implicated in orchestrating the intracellular trafficking of vesicles to the plasma membrane, ultimately leading to the release of exosomes. Additionally, the biogenesis of MSC-Exos also depend on extracellular condition. For instance, exosomes derived from MSCs stimulated by hypoxia condition exhibit a higher level of angiogenic activity.
3.3 Biological advantages of MSC-Exos as drug carrier
EVs derived from human cells possess remarkable biocompatibility, low toxicity, and minimal immunogenicity, especially when obtained from autologous sources. MSC-Exos, in particular, have gained significant attention in the treatment of various conditions, including osteoarthritis, tumors, and autoimmune diseases (such as IBD, SLE, and SS), with rare significant adverse reactions reported (Wiklander et al., 2015; Fang et al., 2023). Their bilayered lipid vesicle structure and nanoscale dimensions enable them to remain stable during long-distance transport and penetrate tissue barriers, including the joint cavity. They have demonstrated effective traversal of biological barriers like the blood-brain barrier (BBB) and placental barrier (Rehman et al., 2023). EV markers such as CD55 and CD59 prevent complement and coagulation factor activation, ensuring their widespread and stable distribution in body fluids.
The diverse contents within EVs, including proteins, lipids, and various nucleic acids (such as miRNAs, mRNAs, tRNAs, lncRNAs, and rRNAs), exhibit excellent biocompatibility with various drugs. Besides therapy, they can also carry contrast agents for disease diagnosis (Avgoulas et al., 2023). In many cases, EV-mediated delivery constitutes the primary mechanism of cell therapy. One significant advantage of drug-loaded EVs is their ability to retain certain characteristics of the parent cells. Meanwhile, the characteristics and applications of MSC-Exo from different sources also vary (Table 1). For instance, immune cell-secreted EVs possess robust immunomodulatory capabilities, and studies indicate that mesenchymal stem cell-derived EVs exhibit both immunomodulatory and regenerative functions. MSC-Exos can reduce the expression of inflammatory mediators through various pathways. For example, Zhang et al. demonstrated that MSC-Exos could attract M2 macrophages, reducing the infiltration of pro-inflammatory M1 macrophages and downregulating the expression of cytokines like IL-1β and TNF-α, thus inhibiting inflammation in osteoarthritis (Zhang et al., 2018b). Moreover, when co-cultured with chondrocytes, adipose-derived mesenchymal stem cells (AD-MSCs) and chondrocytes interact through EVs, promoting AD-MSCs chondrogenesis, increasing chondrocyte extracellular matrix synthesis, and inhibiting cell senescence.
MSC-Exos offer significant advantages in terms of targeting ability and editability, greatly enhancing their drug delivery efficiency. After release from parent cells, exosomes can be internalized by targeted recipient cells through various endocytic pathways, including clathrin-dependent and clathrin-independent endocytosis, phagocytosis, and micropinocytosis. If not internalized, they can attach to target cell membranes through ligand-receptor interactions, activating downstream signaling cascades. For instance, the specific ligands on the surface of AD-MSCs-Exos allow them to target chondrocytes. When loaded with miR-29a and miR-29b, these MSC-Exos can directly target the 3’untranslated region of the Collagen-1 gene, influencing cartilage development and homeostasis (Yan et al., 2011). MSC-Exos exhibit strong editability; alterations in parent cell selection and modifications in culture conditions can enhance their bioactive composition. Techniques such as surface modifications and genetic modifications have significantly improved their drug loading efficiency and targeting capabilities, as will be discussed in detail later in this article.
Unlike cell therapy, EVs face fewer ethical restrictions and a lower risk of immune rejection and malignancy. Meanwhile, compared with MSCs, which require strict conditions to maintain their vitality, MSC-Exos can be easily maintained and stored (Okamura et al., 2023). Currently, many researchers utilize artificially derived extracellular vesicles, such as lipid nanoparticles and liposomes, for their studies because they allow for precise engineering design and offer higher reproducibility. However, they lack the inherent biological complexity of MSC-EVs, often carrying single bioactive molecules, and cannot fully replicate the biological functions of MSC-Exos (Li et al., 2021). Therefore, naturally sourced EVs offer superior therapeutic applicability compared to synthetic drug delivery systems like nanoparticles, liposomes, and resin polymers (Figure 1).
4 Preparation of MSC-Exos
4.1 Sources and preparation of MSC
MSCs can be sourced from various tissues, including the umbilical cord, adipose tissue, muscle, dental tissue, salivary glands, menstrual blood, and more. Despite their diverse origins, they share common traits such as self-renewal, multi-lineage differentiation potential, and immunomodulatory properties. The International Society for Cellular Therapy has defined three basic criteria for identifying human MSCs: (1) When maintained under standard culture conditions, MSCs must adhere to plastic surfaces. (2) MSCs must express CD105, CD73 and CD90, while not expressing CD45, CD34, CD14, CD11b, CD79a, CD19 or HLA-DR surface markers. (3) MSCs must be capable of differentiating into osteoblasts, adipocytes, and chondrocytes in vitro (Dominici et al., 2006). MSCs can be isolated from various joint tissues, including synovial tissue, meniscus, ligaments, adipose pads, and cartilage. MSCs can be isolated from various joint tissues, including synovial tissue, meniscus, ligaments, adipose pads, and cartilage.
The exosomes derived from bone marrow mesenchymal stem cells (BMSCs) were initially used in OA treatment due to their easy isolation, proliferation, and storage in clinical settings. However, BMSCs face challenges such as decreased stem cell number and differentiation capacity with aging, traumatic extraction leading to higher pain levels and complications, and limited extractable bone marrow quantity (Li and Zhang, 2019). In contrast, AD-MSCs have a broader source distribution, as adipose tissue is widely found in the body. By processing adipose tissue through techniques like centrifugation and filtering, the stromal vascular fraction (SVF) can be obtained, containing various cells and cytokines, including mesenchymal stem cells. AD-MSCs can be extracted from SVF, mainly obtained through liposuction procedures. While traditional techniques liposuction yield a high amount of fat, there are still risks of damage to soft tissues, blood vessels, nerves, postoperative uneven skin surfaces, and subcutaneous hematomas (Bourin et al., 2013). Upgraded techniques such as hydrodynamic/ultrasound/laser-assisted negative pressure suction reduce complications and yield more active AD-MSCs. Nowadays, researchers are exploring the extraction of adipose tissue from other surgical procedures, such as subcutaneous fat pads removed during knee arthroscopy. Human adipose tissue is abundant, the extraction process is simple, less ethically constrained, and adipose tissue contains a high proportion of stem cells in the stromal fraction, approximately 5%, whereas BMSCs only account for 0.0001%–0.01%, allows for faster expansion and proliferation (Eom et al., 2011).
Standardized preparation procedures for most MSCs sources, including tissue mincing, optional enzymatic digestion, and explant culture, are lacking. For instance, the cultivation of mature AD-MSCs typically involves the following steps: first, cleaning the obtained adipose tissue with PBS to remove excess blood components, local anesthetics, or joint effusion. Next, the tissue is finely chopped, and collagenase is utilized to eliminate fibrillar collagen. After centrifugation and filtration, the separated fat scraps are introduced into the culture medium for cell growth. These cells are usually passaged to the 3rd to 5th generation for subsequent experiments. There are variations in basic culture media, types and concentrations of collagenase, digestion time, centrifugation speed, duration, and other details among different laboratories (Eydt et al., 2016). Some researchers have attempted to enhance AD-MSCs digestion efficiency using matrix gel digestion methods. However, AD-MSCs consistently yield higher quantities compared to BMSCs (Gittel et al., 2013).
The identification of MSCs involves flow cytometric analysis of their surface markers. Surface marker profiles differ among MSCs from various sources. Both AD-MSCs and BMSCs express CD13, CD29, CD34, CD44, CD73, CD90, CD105, CD166, MHC class I, and HLA-ABC on their cell surfaces. Notably, AD-MSCs do not express CD38, CD45, CD106, HLA-DR, DP, DQ (MHC class II), CD80, CD86, CD40, or CD154 (Zimmerlin et al., 2010). The activity of AD-MSCs can be influenced by patients, sites of adipose tissue, and the culture media used. Common methods for assessing AD-MSCs activity include alkaline phosphatase, Safranin O, Masson’s trichrome staining, ELISA, and Western blot experiments. Researchers, such as Schipper et al., have studied AD-MSCs from adipose tissue obtained from various age groups of women and discovered that the activity of AD-MSCs is significantly higher in younger women. Additionally, adipose tissue from the superficial abdominal layer offers advantages over tissue from the upper arm, inner thigh, rotator cuff, and deep abdominal tissue. In male patients, abdominal superficial tissue also demonstrates advantages over deep fat tissue (Mukhamedshina et al., 2019).
4.2 Isolation and identification of MSC-Exos
As a drug carrier within the body, efficiently obtaining pure, safe, and sufficient exosomes from MSCs is of paramount importance. Ensuring the purity and homogeneity of exosomes while achieving high-efficiency large-scale production has posed technical challenges. Several methods have been employed for isolating exosomes from MSCs, with differential centrifugation being the most traditional approach. Currently, MSCs from the 3rd to 6th generation are generally used. When cell confluence reaches 80%–90%, serum-free medium is replaced, and cells are cultured for 24–48 h (Jha et al., 2016). The resulting cell culture supernatant contains not only exosomes but also various components like dead cells, cell fragments, microvesicles, and apoptotic bodies. Ultracentrifugation is employed to separate exosomes based on size and density, followed by identification using transmission electron microscopy (TEM). Differential centrifugation yields high purity but is less efficient. In contrast, ultrafiltration is more suitable for large-scale production due to lower equipment requirements and shorter processing times. Size Exclusion Chromatography (SEC) and Ion Exchange Chromatography (IEC) also yield high-purity exosomes (Koh et al., 2018). Immunoisolation, based on the specific binding of antibodies targeting surface proteins like CD9, CD63, CD81, Alix, and heat shock proteins, offers high purity but is less suitable for large-scale production. However, its high specificity can be used to isolate specific subpopulations of exosomes, holding unique clinical value (Doyle and Wang, 2019).
Exosome identification techniques have matured, with no significant differences in morphology, isolation, and storage conditions between exosomes derived from MSCs and those from other sources. The basic steps for exosome identification involve morphological observation and surface marker identification. Transmission electron microscopy (TEM) and scanning electron microscopy (SEM) are commonly used techniques for exosome observation (Gurung et al., 2021). Cryo-electron microscopy (cryo-EM) provides more accurate biological information, offering higher resolution and allowing imaging of exosomes in their natural hydrated state without chemical fixation or staining, preserving their natural structure. Cryo-electron tomography (Cryo-ET), an extension of cryo-EM, provides detailed structural information through three-dimensional imaging of exosomes.
Exosomes derived from MSCs not only express common surface markers such as CD9 and CD81 but also often express adhesion molecules like CD29, CD44, and CD73 found on MSC membranes. Developing more sensitive and specific surface protein detection methods has been a focus of exosome identification research. Advanced mass spectrometry techniques enable comprehensive analysis of exosomal protein content and help identify potential biomarkers associated with specific diseases or cellular functions. Immunomagnetic bead-based methods and flow cytometry are also commonly used identification methods. High-throughput sequencing has been used to identify and analyze various RNA and protein contents in exosomes. Nanotechnology and microfluidics techniques allow the analysis of individual exosomes. Diogo Fortunato et al. used nanopore sensing and super-resolution microscopy to identify and analyze exosomes from different cell types, providing a valuable method for exosome heterogeneity analysis (Fortunato et al., 2021).
5 MSC-Exos engineering for drug delivery
5.1 Methods to load cargo into MSC-Exos
One of the fundamental challenges in utilizing MSC-Exos for drug delivery lies in efficiently loading them with various therapeutic cargo. There are two primary strategies. In one approach, therapeutic drugs are loaded into parent MSCs, where they are encapsulated within exosomes during their formation. Nucleic acid cargo is often introduced into cells through viruses or plasmid vectors, enriching therapeutic agents in parent cells to secrete engineered exosomes. To facilitate the entry of target proteins into exosomes, researchers merge the target proteins with anchoring proteins known to enter exosomes. Alternatively, modifications such as WW tag and ubiquitination are employed (Sterzenbach et al., 2017). WW tag, consisting of 38–40 amino acid residues forming a three-strand β-fold structural domain, can be recognized by the late structural domain base sequence on Ndfipl, promoting the packaging of target proteins into exosomes (Lu et al., 1999). Small-molecule chemical drugs are often directly loaded into purified exosomes, necessitating overcoming the barrier function of the exosomal membrane. Hydrophobic membrane-permeable molecules can be delivered into exosomes by simple coincubation at certain temperature and pH. Electroporation is a common method for the passive loading of exogenous cargo. It involves temporarily creating small pores in the phospholipid bilayer using an applied electric field. Under the influence of the electric field, drugs enter the exosomes and then the integrity of the exosomal membrane is restored. Similarly, sonication employs soundwaves to temporarily destabilize the exosomal membrane, facilitating cargo incorporation (Wan et al., 2022). Colja et al. (2023) utilized exosomes to deliver the anti-vimentin nanobody Nb79 for treating Glioblastoma, they experimented with four different methods to load the antibody into the exosomes. The final results indicated that sonication proved to be an efficient method for cargo loading.
5.2 Technologies of large-scale exosome production
To meet the demands of exosome-based drug delivery, researchers have explored diverse strategies aimed at increasing exosome yield. One such approach involves the overexpression or modification of key genes related to processes vital for exosome biogenesis, such as membrane binding and MVBs transportation. For instance, enhancing the expression of TSPAN6 and tetraspanin CD9 has been found to release more exosomes by interacting with multifunctional cytosolic adaptors (Schiller et al., 2018). Another avenue explored by researchers is the genetic modification of MSCs, where the introduction of the MYC gene results in significantly elevated MSC-Exo production. However, the use of MYC, being an oncogene, raises apprehensions regarding its clinical safety (Li et al., 2022).
In addition to biochemical stimuli, researchers have endeavored to optimize cell culture equipment. Utilizing innovative methods such as scaffolds, spheroid culture, hollow fiber bioreactors, and microcarrier-based 3D culture techniques, scientists aim to maximize the limited surface area available for cell growth. Interestingly, expanding the cell-to-cell contact area not only facilitates exosome secretion but also promotes cell differentiation and enhances immunoregulatory capabilities. According to reports, 3D spheroid cultures not only augment MSC exosome secretion but also strengthen their anti-inflammatory and pro-angiogenic functions, showcasing the multifaceted benefits of such innovative culture methods (Kim et al., 2018). Stirred tank bioreactors (STRs) achieve mass transfer and control of cultivation parameters, such as temperature, pH, dissolved oxygen, nutrients, and metabolites, through the circulation generated by mixing and aeration. The advantages of this system lie in its scalability, the uniformity it provides, and the controllability of cultivation variables. It can be adjusted to suit various cell types and specific purposes and is suitable for large-scale exosome production (Eibl et al., 2010).
5.3 Surface modification for targeted delivery
The targeted delivery of exosome derived from natural sources is limited, and even exosomes sourced from the same type of cells exhibit significant heterogeneity. Enhancing the targeting ability of exosome loaded with drugs is crucial for improving drug efficiency, reducing side effects, and directly impacting the overall therapeutic outcome. Exosomes sinternalize into cells by fusing with the cell membrane and activating specific signaling pathways through ligand-receptor interactions. Therefore, the most commonly adopted strategy is to modify the surface of exosomes through genetic or chemical methods to enhance their targeting ability. MSCs can undergo genetic modification to produce exosomes with specific surface proteins or peptides, enabling targeted delivery to chondrocytes or synovial cells in osteoarthritic lesions. Jiang Xia et al. fused chondrocyte-affinity peptide (CAP) with lysosome-associated membrane glycoprotein 2b protein on the surface of exosomes (Liang et al., 2020). This modification allowed the exosomes to effectively encapsulate miR-140 and specifically deliver the cargo to extracellular chondrocytes. Importantly, these CAP-modified exosomes were proven to be well retained within the joint cavity after intra-articular injection. Furthermore, they could be delivered to the deep cartilage regions, inhibiting cartilage-degrading proteases effectively (Tao et al., 2017).
MSC-Exos can also be combined with chemically engineered materials that possess targeted properties, such as temperature sensitivity, pH sensitivity, and charge affinity, to enhance their efficacy. For instance, Schiffelers et al. coated phospholipids (DMPE) and polyethylene glycol (PEG) on the membrane of exosomes containing EGFR nanoantibodies. The modified exosome membrane exhibited temperature-dependent fusion characteristics, significantly enhancing its specific binding to tumor cells overexpressing EGFR. After intravenous injection in mice, the modified exosomes could still be detected in the plasma after 60 min, whereas unmodified exosomes were rapidly cleared within 10 min (Meldolesi, 2018). As OA progresses, physiological factors within the joint cavity, such as ROS level, temperature, and pH, undergo changes. Zhang et al. developed a cross-linked network composed of alginate-dopamine (AD), chondroitin sulfate (CS), and regenerated silk fibroin (RSF) to create a highly adhesive hydrogel for wet surfaces (Zhang et al., 2021). When this hydrogel, encapsulating BMSC-Exos, was injected into rat knee joint cartilage defects, it promoted there cruitment of BMSCs by enhancing the action of BMSCs-Exos and facilitated the differentiation of BMSCs into chondrocytes. Xiaole Qi et al. have developed a multifunctional hydrogel delivery system that co-delivers MSC-Exos and Icariin (ICA) to harness their synergistic effects (Zeng et al., 2023). This mussel-inspired system utilizes a multifunctional hydrogel inspired by mussel adhesion, exhibiting thermal sensitivity, self-healing, and adhesive properties. These characteristics enhance the cellular uptake of MSC-Exos and ICA, prolonging their retention and demonstrating enhanced cartilage protection. Utilizing drug-loaded MSC-Exos can enhance their drug-loading efficiency through various strategies, including regulating the culture conditions of the parent cells and utilizing biomaterials to assist in delivery (Table 2).
6 Current studies of MSC-Exos in OA therapy
6.1 Potential of MSC-Exos therapeutics in OA
MSCs have been proven to possess the potential for differentiating into chondrocytes both in vivo and in vitro. As mentioned earlier, following OA cartilage injury, endogenous (from the pericellular matrix or circulating) or exogenous MSCs are recruited to the site of injury, where they partially undergo chondrogenic differentiation, facilitating cartilage regeneration. MSC-Exos influence MSC homing and promote chondrogenic differentiation. Yan Kang et al. utilized MSC-Exo delivery of circRNA_0001236 and found that it enhances MSC chondrogenesis through the miR-3677-3p/Sox9 axis, thereby suppressing cartilage degradation (Mao et al., 2021). However, the quantity of endogenous MSCs is limited, and the homing capacity of exogenous MSCs is restricted. Relying solely on MSC chondrogenesis yields suboptimal results. While MSC-Exos cannot directly increase the number of MSCs, they can enhance cartilage repair through alternative pathways. Tao Shu et al. employed miRNA-210-overexpressing BMSCs-Exos, significantly reducing LPS-induced cartilage injury via the NF-kβ pathway (He et al., 2020). AD-MSCs-Exos deliver miR-100-5p to chondrocytes, significantly enhancing cellular autophagy by inhibiting the mTOR pathway, consequently reducing cartilage damage. Animal experiments have demonstrated substantial gait improvements (Wu et al., 2019).
Inflammatory conditions within the joint cavity accelerate OA progression, often preceding radiological manifestations and significantly correlating with clinical pain. Thus, controlling early OA inflammation is crucial to slowing its progression and improving symptoms. MSCs modulate various immune cells within the joint. Researchers subjected AD-MSCs to inflammatory stimuli TNF-α and IFN-γ, finding increased expression of vascular endothelial growth factor and fibroblast growth factor genes, while pro-inflammatory factors TNFA and PTGS2 gene expression decreased, indicating that AD-MSCs can mitigate inflammation through paracrine effects in inflammatory environments (Sukho et al., 2017). After co-culturing AD-MSCs-Exos with IL-1β stimulated chondrocytes, AD-MSCs-Exos reduced the activity of inflammatory transcription factors, thereby decreasing the expression of inflammatory mediators, exerting a protective effect on the chondrocyte extracellular matrix. Shen et al. (2016) discovered that MSC-Exos express C-C motif chemokine receptor 2 (CCR2), acting as decoys that bind and inhibit the activity of the pro-inflammatory chemokine CCL2, preventing macrophage accumulation and tissue damage. Moreover, MSC-Exos target macrophages, regulating M1/M2 polarization, a phenomenon demonstrated in various disease models. MSC-Exos inhibit T cell proliferation in a dose-dependent manner and induce Treg populations, thereby exerting immunomodulatory effects on inflammatory joint diseases.
Michelle Delco et al. discovered that MSCs package fully functional mitochondria into exosomes, which are then transferred to chondrocytes. This restoration of mitochondrial function in recipient cells maintains cellular vitality, promoting tissue repair (Fahey et al., 2022). This work provides a delivery strategy for cell-free mitochondrial targeted therapy, highlighting the potent therapeutic potential of mitochondrial delivery in poorly vascularized musculoskeletal tissues. Given the excellent delivery capabilities and rich biological functionalities of MSC-Exosomes, their potential as a drug delivery system for treating OA is remarkable, with research in relevant models becoming increasingly mature (Figure 2).
6.2 Application of MSC-Exos based drug delivery systems in OA treatment
Research on cell-free therapy using MSC-Exos lags behind MSC therapy. Currently, the development of Targeted Drug Delivery Systems (DDS) based on MSC-Exos is in its early stages, primarily limited to in vitro or animal experiments. We have summarized representative studies from the past 5 years (Table3). The most frequently applied MSC-Exo delivery involves various microRNAs. Various microRNAs have been proven to be deliverable to chondrocytes or immune cells within the joints, improving OA prognosis through promoting chondrogenesis, inhibiting apoptosis, and immune modulation. A single microRNA can have multiple potential targets. For instance, studies conducted by Wang R et al. have demonstrated that microRNA-135p, when loaded onto MSC-Exos for treating OA, targets genes such as PDGF-BB, MAPK6, and Sp1, influencing the biological functions of various components in joint cartilage, subchondral bone, and immune cells, thereby enhancing OA prognosis (Wang and Xu, 2021; Wang and Xu, 2022).Liang et al. utilized CAP-labeled exosomes, characterized by prolonged joint retention, limited in vivo diffusion, and the ability to deliver miR-140 to the deep cartilage region. This delivery effectively suppressed cartilage-degrading enzymes, thereby alleviating the progression of osteoarthritis in a rat model (Figure 3).
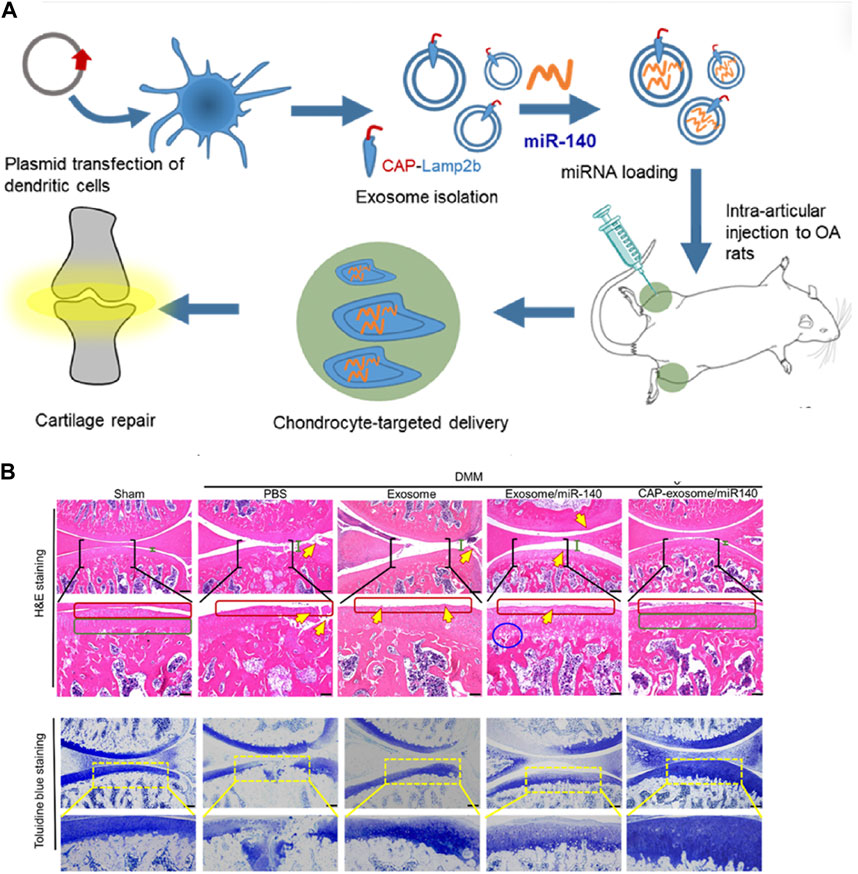
Figure 3. Representative study on MSC-Exos drug delivery for OA therapy. (A) Schematic illustration of surface engineering of exosomes for targeted delivery of miR-140 to chondrocytes for OA treatment. (B) Histological assessment of the cartilage of DMM rats treated with CAP-exosome/miR-140 or exosome/miR-140 preparations: Stained images of the cartilage tissues. Scale bars, 250 μm for the top panel and 100 μm for the bottom panel. Certain regions of the top panel pictures are enlarged in the bottom panel. Green bars indicate the distance of the joint space. Yellow arrows indicate fractures or irregular morphology of the cartilage layer. Red squares indicate the surface of the cartilage layers. The blue circle indicates cavities between cartilage and subchondral bone. Green squares indicate normal, condensed morphology of the cartilage. Reprinted (adapted) with permission from (Liang et al., 2020). Copyright {2024}American Chemical Society.
Animal models of OA are categorized as spontaneous and induced. Spontaneous models include natural occurrence and genetic models. However, due to their lengthy experimental periods, poor controllability, and high costs, they are less commonly utilized. Transgenic mouse OA models, studying the effect of individual genes in the pathogenesis of OA, have yielded good results. Nevertheless, OA is generally believed to result from the interaction of multiple genes, making such models oversimplified in representing the development of OA. Constructing animal models of OA typically involves two main approaches: intra-articular drug injections and joint surgery. Surgical induction, mainly performed on the knee joint, includes procedures like partial or complete meniscectomy, anterior cruciate ligament transection, and medial or lateral collateral ligament transection. The goal is to induce joint instability and mechanical structure changes, making it the mainstream modeling method due to its short modeling period and clear effects (Liu et al., 2016). However, it is considered closer to the development process of post-traumatic osteoarthritis. Chemical induction primarily employs sodium iodate and collagenase. While this method yields rapid results, its effectiveness as a clinical model is questioned, often being used more as a joint pain model (Barton et al., 2017).
As of September 2023, there are 238 registered clinical trials for MSC therapy for OA listed on the National Institutes of Health (NIH) database. The safety and effectiveness of MSC therapy have been largely validated. However, there is only one registered clinical trial related to MSC-Exos (NCT05060107), initiated by Espinoza F in October 2021. The study aims to evaluate the safety of exosomes from allogeneic mesenchymal stromal cells delivered via intra-articular injection in the knee of patients with mild to moderate symptomatic osteoarthritis. They plan to enroll 10 patients in this phase 1 trial with a follow-up period of up to 12 months. However, the experimental results have not been published yet. Generating clinical-grade MSC-exos for OA treatment faces hurdles including standardization of isolation techniques, scalability of production, and ensuring safety and efficacy. Ensuring consistency in yield, purity, and biological activity is vital, as is rigorous quality control for safety and efficacy. Optimizing therapeutic efficacy and navigating regulatory requirements also present challenges (Harrell et al., 2020). It is believed that there will be more clinical trials in the future to validate the effectiveness of MSC-Exos in drug delivery for OA. Upgrading exosome production techniques to reduce economic costs, combined with new biomaterials, opens new avenues for drug delivery strategies. This integration is crucial for the clinical application of MSC-Exos.
7 Conclusion and outlook
The current non-surgical treatments for osteoarthritis are not very effective due to the irreparable damage to cartilage, posing a significant challenge. With the advancements in regenerative medicine, cell therapies, particularly those involving MSCs, have been extensively researched. These studies have shown promising results in various preclinical trials. The use of MSC-Exos to treat osteoarthritis not only overcomes ethical limitations associated with cell therapies but also harnesses their potential as drug carriers. One notable advantage of exosomes is their editability, which plays a crucial role in enhancing their efficacy as drug carriers. Collaboration among researchers from different fields, including molecular biology, nanotechnology, and material science, is vital in developing exosome-based drug delivery systems with stronger targeting capabilities and higher drug-loading efficiency. As efforts focus on improving drug-loading efficiency, future research will also address the need for sustained drug release within the joint cavity. This approach aims to significantly reduce the frequency of intra-articular injections, thereby lowering economic costs and minimizing side effects.
Author contributions
SW: Conceptualization, Data curation, Formal Analysis, Writing–original draft, Writing–review and editing. XH: Data curation, Validation, Writing–original draft. JM: Conceptualization, Visualization, Writing–original draft. GZ: Writing–review and editing. TM: Validation, Visualization, Writing–original draft. KC: Software, Writing–review and editing. GH: Validation, Writing–review and editing. JC: Writing–review and editing, Validation. JS: Writing–review and editing, Resources. SW: Funding acquisition, Resources, Validation, Writing–review and editing.
Funding
The author(s) declare financial support was received for the research, authorship, and/or publication of this article. The research was supported by funding from the Shanghai Municipal Science and Technology Commission’s General Project (21ZR1411000), and the corresponding author of this article is the project leader of the fund.
Conflict of interest
The authors declare that the research was conducted in the absence of any commercial or financial relationships that could be construed as a potential conflict of interest.
Publisher’s note
All claims expressed in this article are solely those of the authors and do not necessarily represent those of their affiliated organizations, or those of the publisher, the editors and the reviewers. Any product that may be evaluated in this article, or claim that may be made by its manufacturer, is not guaranteed or endorsed by the publisher.
References
Avgoulas, D. I., Tasioulis, K. S., Papi, R. M., and Pantazaki, A. A. (2023). Therapeutic and diagnostic potential of exosomes as drug delivery systems in brain cancer. Pharmaceutics 15, 1439. doi:10.3390/pharmaceutics15051439
Barton, K. I., Shekarforoush, M., Heard, B. J., Sevick, J. L., Vakil, P., Atarod, M., et al. (2017). Use of pre-clinical surgically induced models to understand biomechanical and biological consequences of PTOA development. J. Orthop. Res. 35, 454–465. doi:10.1002/jor.23322
Batrakova, E. V., and Kim, M. S. (2015). Using exosomes, naturally-equipped nanocarriers, for drug delivery. J. Control Release 219, 396–405. doi:10.1016/j.jconrel.2015.07.030
Bourin, P., Bunnell, B. A., Casteilla, L., Dominici, M., Katz, A. J., March, K. L., et al. (2013). Stromal cells from the adipose tissue-derived stromal vascular fraction and culture expanded adipose tissue-derived stromal/stem cells: a joint statement of the international federation for adipose therapeutics and science (ifats) and the international society for cellular therapy (isct). Cytotherapy 15, 641–648. doi:10.1016/j.jcyt.2013.02.006
Cai, Y., Li, J., Jia, C., He, Y., and Deng, C. (2020). Therapeutic applications of adipose cell-free derivatives: a review. Stem Cell Res. Ther. 11, 312. doi:10.1186/s13287-020-01831-3
Chen, S., Sun, F., Qian, H., Xu, W., and Jiang, J. (2022). Preconditioning and engineering strategies for improving the efficacy of mesenchymal stem cell-derived exosomes in cell-free therapy. Stem Cells Int. 2022, 1–18. doi:10.1155/2022/1779346
Chen, X., Shi, Y., Xue, P., Ma, X., Li, J., and Zhang, J. (2020). Mesenchymal stem cell-derived exosomal microRNA-136-5p inhibits chondrocyte degeneration in traumatic osteoarthritis by targeting ELF3. Arthritis Res. Ther. 22, 256. doi:10.1186/s13075-020-02325-6
Chen, Z., Wang, H., Xia, Y., Yan, F., and Lu, Y. (2018). Therapeutic potential of mesenchymal cell-derived miRNA-150-5p-expressing exosomes in rheumatoid arthritis mediated by the modulation of MMP14 and VEGF. J. Immunol. 201, 2472–2482. doi:10.4049/jimmunol.1800304
Colja, S., Jovčevska, I., Šamec, N., Romih, R., and Zottel, A. (2023). Sonication is a suitable method for loading nanobody into glioblastoma small extracellular vesicles. Heliyon 9, e15674. doi:10.1016/j.heliyon.2023.e15674
Cosenza, S., Ruiz, M., Toupet, K., Jorgensen, C., and Noël, D. (2017). Mesenchymal stem cells derived exosomes and microparticles protect cartilage and bone from degradation in osteoarthritis. Sci. Rep. 7, 16214. doi:10.1038/s41598-017-15376-8
De Lange-Brokaar, B. J., Ioan-Facsinay, A., Van Osch, G. J., Zuurmond, A. M., Schoones, J., Toes, R. E., et al. (2012). Synovial inflammation, immune cells and their cytokines in osteoarthritis: a review. Osteoarthr. Cartil. 20, 1484–1499. doi:10.1016/j.joca.2012.08.027
Di Rosa, M., Szychlinska, M. A., Tibullo, D., Malaguarnera, L., and Musumeci, G. (2014). Expression of CHI3L1 and CHIT1 in osteoarthritic rat cartilage model. A morphological study. Eur. J. Histochem 58, 2423. doi:10.4081/ejh.2014.2423
Dominici, M., Le Blanc, K., Mueller, I., Slaper-Cortenbach, I., Marini, F., Krause, D., et al. (2006). Minimal criteria for defining multipotent mesenchymal stromal cells. The International Society for Cellular Therapy position statement. Cytotherapy 8, 315–317. doi:10.1080/14653240600855905
Dong, J., Li, L., Fang, X., and Zang, M. (2021). Exosome-encapsulated microRNA-127-3p released from bone marrow-derived mesenchymal stem cells alleviates osteoarthritis through regulating CDH11-mediated wnt/β-catenin pathway. J. Pain Res. 14, 297–310. doi:10.2147/jpr.s291472
Doyle, L. M., and Wang, M. Z. (2019). Overview of extracellular vesicles, their origin, composition, purpose, and methods for exosome isolation and analysis. Cells 8, 727. doi:10.3390/cells8070727
Eibl, R., Kaiser, S., Lombriser, R., and Eibl, D. (2010). Disposable bioreactors: the current state-of-the-art and recommended applications in Biotechnology. Appl. Microbiol. Biotechnol. 86, 41–49. doi:10.1007/s00253-009-2422-9
Eom, Y. W., Lee, J. E., Yang, M. S., Jang, I. K., Kim, H. E., Lee, D. H., et al. (2011). Rapid isolation of adipose tissue-derived stem cells by the storage of lipoaspirates. Yonsei Med. J. 52, 999–1007. doi:10.3349/ymj.2011.52.6.999
Eydt, C., Geburek, F., Schröck, C., Hambruch, N., Rohn, K., Pfarrer, C., et al. (2016). Sternal bone marrow derived equine multipotent mesenchymal stromal cells (MSCs): investigations considering the sampling site and the use of different culture media. Veterinary Med. Sci. 2, 200–210. doi:10.1002/vms3.36
Fahey, M., Bennett, M., Thomas, M., Montney, K., Vivancos-Koopman, I., Pugliese, B., et al. (2022). Mesenchymal stromal cells donate mitochondria to articular chondrocytes exposed to mitochondrial, environmental, and mechanical stress. Sci. Rep. 12, 21525. doi:10.1038/s41598-022-25844-5
Fang, Y., Ni, J., Wang, Y. S., Zhao, Y., Jiang, L. Q., Chen, C., et al. (2023). Exosomes as biomarkers and therapeutic delivery for autoimmune diseases: opportunities and challenges. Autoimmun. Rev. 22, 103260. doi:10.1016/j.autrev.2022.103260
Fortunato, D., Mladenović, D., Criscuoli, M., Loria, F., Veiman, K. L., Zocco, D., et al. (2021). Opportunities and pitfalls of fluorescent labeling methodologies for extracellular vesicle profiling on high-resolution single-particle platforms. Int. J. Mol. Sci. 22, 10510. doi:10.3390/ijms221910510
Gittel, C., Brehm, W., Burk, J., Juelke, H., Staszyk, C., and Ribitsch, I. (2013). Isolation of equine multipotent mesenchymal stromal cells by enzymatic tissue digestion or explant technique: comparison of cellular properties. BMC Veterinary Res. 9, 221. doi:10.1186/1746-6148-9-221
Gurung, S., Perocheau, D., Touramanidou, L., and Baruteau, J. (2021). The exosome journey: from biogenesis to uptake and intracellular signalling. Cell Commun. Signal 19, 47. doi:10.1186/s12964-021-00730-1
Harding, C., Heuser, J., and Stahl, P. (1983). Receptor-mediated endocytosis of transferrin and recycling of the transferrin receptor in rat reticulocytes. J. Cell Biol. 97, 329–339. doi:10.1083/jcb.97.2.329
Harrell, C. R., Jovicic, N., Djonov, V., and Volarevic, V. (2020). Therapeutic use of mesenchymal stem cell-derived exosomes: from basic science to clinics. Pharmaceutics 12, 474. doi:10.3390/pharmaceutics12050474
Hass, R., Kasper, C., Böhm, S., and Jacobs, R. (2011). Different populations and sources of human mesenchymal stem cells (MSC): a comparison of adult and neonatal tissue-derived MSC. Cell Commun. Signal 9, 12. doi:10.1186/1478-811x-9-12
He, L., Chen, Y., Ke, Z., Pang, M., Yang, B., Feng, F., et al. (2020). Exosomes derived from miRNA-210 overexpressing bone marrow mesenchymal stem cells protect lipopolysaccharide induced chondrocytes injury via the NF-κB pathway. Gene 751, 144764. doi:10.1016/j.gene.2020.144764
Henne, W. M., Buchkovich, N. J., and Emr, S. D. (2011). The ESCRT pathway. Dev. Cell 21, 77–91. doi:10.1016/j.devcel.2011.05.015
Hickey, D. G., Frenkel, S. R., and Di Cesare, P. E. (2003). Clinical applications of growth factors for articular cartilage repair. Am. J. Orthop. (Belle Mead NJ) 32, 70–76.
Hunter, D. J., and Bierma-Zeinstra, S. (2019). Osteoarthritis. Lancet 393, 1745–1759. doi:10.1016/s0140-6736(19)30417-9
Jha, S., Kumar, D., and Kaul, J. M. (2016). Efficacy of immediate, 24 hours versus 48 hours culture method in cytogenetic study of pediatric acute lymphoblastic leukemia. Int. J. Anat. Res. 4, 3175–3178. doi:10.16965/ijar.2016.432
Jiang, T., Wang, Z., and Sun, J. (2020). Human bone marrow mesenchymal stem cell-derived exosomes stimulate cutaneous wound healing mediates through TGF-β/Smad signaling pathway. Stem Cell Res. Ther. 11, 198. doi:10.1186/s13287-020-01723-6
Jin, Z., Ren, J., and Qi, S. (2020). Exosomal miR-9-5p secreted by bone marrow-derived mesenchymal stem cells alleviates osteoarthritis by inhibiting syndecan-1. Cell Tissue Res. 381, 99–114. doi:10.1007/s00441-020-03193-x
Juan, T., and Fürthauer, M. (2018). Biogenesis and function of ESCRT-dependent extracellular vesicles. Semin. Cell Dev. Biol. 74, 66–77. doi:10.1016/j.semcdb.2017.08.022
Kim, M., Yun, H.-W., Park, D. Y., Choi, B. H., and Min, B. H. (2018). Three-dimensional spheroid culture increases exosome secretion from mesenchymal stem cells. Tissue Eng. Regen. Med. 15, 427–436. doi:10.1007/s13770-018-0139-5
Koh, Y. X., Almughlliq, F. B., Vaswani, K., Peiris, H. N., and Mitchell, M. D. (2018). Exosome enrichment by ultracentrifugation and size exclusion Chromatography. Front. Bioscience-Scholar 23, 865–874. doi:10.2741/4621
Komaki, M., Numata, Y., Morioka, C., Honda, I., Tooi, M., Yokoyama, N., et al. (2017). Exosomes of human placenta-derived mesenchymal stem cells stimulate angiogenesis. Stem Cell Res. Ther. 8, 219. doi:10.1186/s13287-017-0660-9
Kong, R., Zhang, J., Ji, L., Yu, Y., Gao, J., and Zhao, D. (2023). Synovial mesenchymal stem cell-derived exosomal microRNA-320c facilitates cartilage damage repair by targeting ADAM19-dependent Wnt signalling in osteoarthritis rats. Inflammopharmacology 31, 915–926. doi:10.1007/s10787-023-01142-y
Kowal, J., Arras, G., Colombo, M., Jouve, M., Morath, J. P., Primdal-Bengtson, B., et al. (2016). Proteomic comparison defines novel markers to characterize heterogeneous populations of extracellular vesicle subtypes. Proc. Natl. Acad. Sci. U. S. A. 113, E968–E977. doi:10.1073/pnas.1521230113
Lai, R. C., Arslan, F., Lee, M. M., Sze, N. S., Choo, A., Chen, T. S., et al. (2010). Exosome secreted by MSC reduces myocardial ischemia/reperfusion injury. Stem Cell Res. 4, 214–222. doi:10.1016/j.scr.2009.12.003
Le, H., Xu, W., Zhuang, X., Chang, F., Wang, Y., and Ding, J. (2020). Mesenchymal stem cells for cartilage regeneration. J. Tissue Eng. 11, 204173142094383. doi:10.1177/2041731420943839
Leisheng, Z., Xiaowei, G., Shixun, M., Miao, Y., Xianghong, X., Yuanguang, Z., et al. (2023). “MSC-derived exosomes for tissue engineering and disease intervention,” in Exosomes. Editor S. SHERIN (Rijeka: IntechOpen).
Li, Y. J., Wu, J. Y., Liu, J., Xu, W., Qiu, X., Huang, S., et al. (2021). Artificial exosomes for translational nanomedicine. J. Nanobiotechnology 19, 242. doi:10.1186/s12951-021-00986-2
Liang, Y., Li, J., Wang, Y., He, J., Chen, L., Chu, J., et al. (2022). Platelet rich plasma in the repair of articular cartilage injury: a narrative review. Cartilage 13, 194760352211184. doi:10.1177/19476035221118419
Liang, Y., Xu, X., Li, X., Xiong, J., Li, B., Duan, L., et al. (2020). Chondrocyte-targeted MicroRNA delivery by engineered exosomes toward a cell-free osteoarthritis therapy. ACS Appl. Mater Interfaces 12, 36938–36947. doi:10.1021/acsami.0c10458
Li, F., Xu, Z., Xie, Z., Sun, X., Li, C., Chen, Y., et al. (2023). Adipose mesenchymal stem cells-derived exosomes alleviate osteoarthritis by transporting microRNA -376c-3p and targeting the WNT-beta-catenin signaling axis. Apoptosis 28, 362–378. doi:10.1007/s10495-022-01787-0
Li, M., Jiang, Y., Hou, Q., Zhao, Y., Zhong, L., and Fu, X. (2022). Potential pre-activation strategies for improving therapeutic efficacy of mesenchymal stem cells: current status and future prospects. Stem Cell Res. Ther. 13, 146. doi:10.1186/s13287-022-02822-2
Liu, S., Fan, M., Xu, J. X., Yang, L. J., Qi, C. C., Xia, Q. R., et al. (2022). Exosomes derived from bone-marrow mesenchymal stem cells alleviate cognitive decline in AD-like mice by improving BDNF-related neuropathology. J. Neuroinflammation 19, 35. doi:10.1186/s12974-022-02393-2
Liu, Y., Zou, R., Wang, Z., Wen, C., Zhang, F., and Lin, F. (2018). Exosomal KLF3-AS1 from hMSCs promoted cartilage repair and chondrocyte proliferation in osteoarthritis. Biochem. J. 475, 3629–3638. doi:10.1042/bcj20180675
Liu, Z., Hu, X., Man, Z., Zhang, J., Jiang, Y., and Ao, Y. (2016). A novel rabbit model of early osteoarthritis exhibits gradual cartilage degeneration after medial collateral ligament transection outside the joint capsule. Sci. Rep. 6, 34423. doi:10.1038/srep34423
Li, Z., and Zhang, Y. (2019). Efficacy of bone marrow stimulation in arthroscopic repair of full thickness rotator cuff tears: a meta-analysis. J. Orthop. Surg. Res. 14, 36. doi:10.1186/s13018-019-1072-6
Lu, P. J., Zhou, X. Z., Shen, M., and Lu, K. P. (1999). Function of WW domains as phosphoserine- or phosphothreonine-binding modules. Science 283, 1325–1328. doi:10.1126/science.283.5406.1325
Mao, G., Xu, Y., Long, D., Sun, H., Li, H., Xin, R., et al. (2021). Exosome-transported circRNA_0001236 enhances chondrogenesis and suppress cartilage degradation via the miR-3677-3p/Sox9 axis. Stem Cell Res. Ther. 12, 389. doi:10.1186/s13287-021-02431-5
Meldolesi, J. (2018). Exosomes and ectosomes in intercellular communication. Curr. Biol. 28, R435–R444. doi:10.1016/j.cub.2018.01.059
Mendt, M., Rezvani, K., and Shpall, E. (2019). Mesenchymal stem cell-derived exosomes for clinical use. Bone Marrow Transplant. 54, 789–792. doi:10.1038/s41409-019-0616-z
Minciacchi, V. R., Freeman, M. R., and Di Vizio, D. (2015). Extracellular vesicles in cancer: exosomes, microvesicles and the emerging role of large oncosomes. Semin. Cell Dev. Biol. 40, 41–51. doi:10.1016/j.semcdb.2015.02.010
Mobasheri, A., Vannucci, S. J., Bondy, C. A., Carter, S. D., Innes, J. F., Arteaga, M. F., et al. (2002). Glucose transport and metabolism in chondrocytes: a key to understanding chondrogenesis, skeletal development and cartilage degradation in osteoarthritis. Histol. Histopathol. 17, 1239–1267. doi:10.14670/HH-17.1239
Mukhamedshina, Y., Shulman, I., Ogurcov, S., Kostennikov, A., Zakirova, E., Rogozhin, A., et al. (2019). Mesenchymal stem cell therapy for spinal cord contusion: a comparative study on small and large animal models. Biomolecules 9, 811. doi:10.3390/biom9120811
Ni, Z., Kuang, L., Chen, H., Xie, Y., Zhang, B., Ouyang, J., et al. (2019). The exosome-like vesicles from osteoarthritic chondrocyte enhanced mature IL-1β production of macrophages and aggravated synovitis in osteoarthritis. Cell Death Dis. 10, 522. doi:10.1038/s41419-019-1739-2
Okamura, A., Yoshioka, Y., Saito, Y., and Ochiya, T. (2023). Can extracellular vesicles as drug delivery systems Be a game changer in cardiac disease? Pharm. Res. 40, 889–908. doi:10.1007/s11095-022-03463-z
Oses, C., Olivares, B., Ezquer, M., Acosta, C., Bosch, P., Donoso, M., et al. (2017). Preconditioning of adipose tissue-derived mesenchymal stem cells with deferoxamine increases the production of pro-angiogenic, neuroprotective and anti-inflammatory factors: potential application in the treatment of diabetic neuropathy. PLoS One 12, e0178011. doi:10.1371/journal.pone.0178011
Patel, J. M., Saleh, K. S., Burdick, J. A., and Mauck, R. L. (2019). Bioactive factors for cartilage repair and regeneration: improving delivery, retention, and activity. Acta Biomater. 93, 222–238. doi:10.1016/j.actbio.2019.01.061
Qiu, B., Xu, X., Yi, P., and Hao, Y. (2020). Curcumin reinforces MSC-derived exosomes in attenuating osteoarthritis via modulating the miR-124/NF-kB and miR-143/ROCK1/TLR9 signalling pathways. J. Cell Mol. Med. 24, 10855–10865. doi:10.1111/jcmm.15714
Qiu, M., Liu, D., and Fu, Q. (2021). MiR-129-5p shuttled by human synovial mesenchymal stem cell-derived exosomes relieves IL-1β induced osteoarthritis via targeting HMGB1. Life Sci. 269, 118987. doi:10.1016/j.lfs.2020.118987
Quicke, J. G., Conaghan, P. G., Corp, N., and Peat, G. (2022). Osteoarthritis year in review 2021: epidemiology & therapy. Osteoarthr. Cartil. 30, 196–206. doi:10.1016/j.joca.2021.10.003
Rahbaran, M., Zekiy, A. O., Bahramali, M., Jahangir, M., Mardasi, M., Sakhaei, D., et al. (2022). Therapeutic utility of mesenchymal stromal cell (MSC)-based approaches in chronic neurodegeneration: a glimpse into underlying mechanisms, current status, and prospects. Cell Mol. Biol. Lett. 27, 56. doi:10.1186/s11658-022-00359-z
Rehman, F. U., Liu, Y., Zheng, M., and Shi, B. (2023). Exosomes based strategies for brain drug delivery. Biomaterials 293, 121949. doi:10.1016/j.biomaterials.2022.121949
Ruhlen, R., and Marberry, K. (2014). The chondrocyte primary cilium. Osteoarthr. Cartil. 22, 1071–1076. doi:10.1016/j.joca.2014.05.011
Sahu, R., Kaushik, S., Clement, C. C., Cannizzo, E. S., Scharf, B., Follenzi, A., et al. (2011). Microautophagy of cytosolic proteins by late endosomes. Dev. Cell 20, 131–139. doi:10.1016/j.devcel.2010.12.003
Schiller, L. T., Lemus-Diaz, N., Rinaldi Ferreira, R., Böker, K. O., and Gruber, J. (2018). Enhanced production of exosome-associated AAV by overexpression of the tetraspanin CD9. Mol. Ther. Methods Clin. Dev. 9, 278–287. doi:10.1016/j.omtm.2018.03.008
Sekiya, I., Ojima, M., Suzuki, S., Yamaga, M., Horie, M., Koga, H., et al. (2012). Human mesenchymal stem cells in synovial fluid increase in the knee with degenerated cartilage and osteoarthritis. J. Orthop. Res. 30, 943–949. doi:10.1002/jor.22029
Shao, L. T., Luo, L., Qiu, J. H., and Deng, D. Y. B. (2022). PTH (1-34) enhances the therapeutic effect of bone marrow mesenchymal stem cell-derived exosomes by inhibiting proinflammatory cytokines expression on OA chondrocyte repair in vitro. Arthritis Res. Ther. 24, 96. doi:10.1186/s13075-022-02778-x
Sharma, A. R., Jagga, S., Lee, S. S., and Nam, J. S. (2013). Interplay between cartilage and subchondral bone contributing to pathogenesis of osteoarthritis. Int. J. Mol. Sci. 14, 19805–19830. doi:10.3390/ijms141019805
Shen, B., Liu, J., Zhang, F., Wang, Y., Qin, Y., Zhou, Z., et al. (2016). CCR2 positive exosome released by mesenchymal stem cells suppresses macrophage functions and alleviates ischemia/reperfusion-induced renal injury. Stem Cells Int. 2016, 1240301–1240309. doi:10.1155/2016/1240301
Skotland, T., Hessvik, N. P., Sandvig, K., and Llorente, A. (2019). Exosomal lipid composition and the role of ether lipids and phosphoinositides in exosome biology. J. Lipid Res. 60, 9–18. doi:10.1194/jlr.r084343
Smith, R. J. P., Faroni, A., Barrow, J. R., Soul, J., and Reid, A. J. (2021). The angiogenic potential of CD271+ human adipose tissue-derived mesenchymal stem cells. Stem Cell Res. Ther. 12, 160. doi:10.1186/s13287-021-02177-0
Sterzenbach, U., Putz, U., Low, L. H., Silke, J., Tan, S. S., and Howitt, J. (2017). Engineered exosomes as vehicles for biologically active proteins. Mol. Ther. 25, 1269–1278. doi:10.1016/j.ymthe.2017.03.030
Sukho, P., Kirpensteijn, J., Hesselink, J. W., Van Osch, G. J., Verseijden, F., and Bastiaansen-Jenniskens, Y. M. (2017). Effect of cell seeding density and inflammatory cytokines on adipose tissue-derived stem cells: an in vitro study. Stem Cell Rev. Rep. 13, 267–277. doi:10.1007/s12015-017-9719-3
Sun, A. R., Wu, X., Liu, B., Chen, Y., Armitage, C. W., Kollipara, A., et al. (2019). Pro-resolving lipid mediator ameliorates obesity induced osteoarthritis by regulating synovial macrophage polarisation. Sci. Rep. 9, 426. doi:10.1038/s41598-018-36909-9
Tan, S. S., Yin, Y., Lee, T., Lai, R. C., Yeo, R. W., Zhang, B., et al. (2013). Therapeutic MSC exosomes are derived from lipid raft microdomains in the plasma membrane. J. Extracell. Vesicles 2. doi:10.3402/jev.v2i0.22614
Tao, S. C., Tan, Y., Zhang, Y.-L., Yin, W., Guo, S., and Zhang, C. (2017). Exosomes derived from miR-140-5p-overexpressing human synovial mesenchymal stem cells enhance cartilage tissue regeneration and prevent osteoarthritis of the knee in a rat model. Theranostics 7, 180–195. doi:10.7150/thno.17133
Tao, Y., Zhou, J., Wang, Z., Tao, H., Bai, J., Ge, G., et al. (2021). Human bone mesenchymal stem cells-derived exosomal miRNA-361-5p alleviates osteoarthritis by downregulating DDX20 and inactivating the NF-κB signaling pathway. Bioorg Chem. 113, 104978. doi:10.1016/j.bioorg.2021.104978
Théry, C., Zitvogel, L., and Amigorena, S. (2002). Exosomes: composition, biogenesis and function. Nat. Rev. Immunol. 2, 569–579. doi:10.1038/nri855
To, K., Zhang, B., Romain, K., Mak, C., and Khan, W. (2019). Synovium-derived mesenchymal stem cell transplantation in cartilage regeneration: a prisma review of in vivo studies. Front. Bioeng. Biotechnol. 7, 314. doi:10.3389/fbioe.2019.00314
Trams, E. G., Lauter, C. J., Salem, N., and Heine, U. (1981). Exfoliation of membrane ecto-enzymes in the form of micro-vesicles. Biochim. Biophys. Acta 645, 63–70. doi:10.1016/0005-2736(81)90512-5
Wakitani, S., Mitsuoka, T., Nakamura, N., Toritsuka, Y., Nakamura, Y., and Horibe, S. (2004). Autologous bone marrow stromal cell transplantation for repair of full-thickness articular cartilage defects in human patellae: two case reports. Cell Transpl. 13, 595–600. doi:10.3727/000000004783983747
Wang, R., and Xu, B. (2021). TGF-β1-modified MSC-derived exosomal miR-135b attenuates cartilage injury via promoting M2 synovial macrophage polarization by targeting MAPK6. Cell Tissue Res. 384, 113–127. doi:10.1007/s00441-020-03319-1
Wang, R., and Xu, B. (2022). TGFβ1-modified MSC-derived exosome attenuates osteoarthritis by inhibiting PDGF-BB secretion and H-type vessel activity in the subchondral bone. Acta histochem. 124, 151933. doi:10.1016/j.acthis.2022.151933
Wang, R., Xu, B., and Xu, H. (2018). TGF-β1 promoted chondrocyte proliferation by regulating Sp1 through MSC-exosomes derived miR-135b. Cell Cycle 17, 2756–2765. doi:10.1080/15384101.2018.1556063
Wan, T., Zhong, J., Pan, Q., Zhou, T., Yuan, P., and Liu, X. (2022). Exosome-mediated delivery of Cas9 ribonucleoprotein complexes for tissue-specific gene therapy of liver diseases. Sci. Adv. 8, eabp9435. doi:10.1126/sciadv.abp9435
Wen, C., Lin, L., Zou, R., Lin, F., and Liu, Y. (2022). Mesenchymal stem cell-derived exosome mediated long non-coding RNA KLF3-AS1 represses autophagy and apoptosis of chondrocytes in osteoarthritis. Cell Cycle 21, 289–303. doi:10.1080/15384101.2021.2019411
Wiklander, O. P., Nordin, J. Z., O'Loughlin, A., Gustafsson, Y., Corso, G., Mäger, I., et al. (2015). Extracellular vesicle in vivo biodistribution is determined by cell source, route of administration and targeting. J. Extracell. Vesicles 4, 26316. doi:10.3402/jev.v4.26316
Wu, J., Kuang, L., Chen, C., Yang, J., Zeng, W. N., Li, T., et al. (2019). miR-100-5p-abundant exosomes derived from infrapatellar fat pad MSCs protect articular cartilage and ameliorate gait abnormalities via inhibition of mTOR in osteoarthritis. Biomaterials 206, 87–100. doi:10.1016/j.biomaterials.2019.03.022
Xu, L., Golshirazian, I., Asbury, B. J., and Li, Y. (2014). Induction of high temperature requirement A1, a serine protease, by TGF-beta1 in articular chondrocytes of mouse models of OA. Histol. Histopathol. 29, 609–618. doi:10.14670/HH-29.10.609
Xu, X., Liang, Y., Li, X., Ouyang, K., Wang, M., Cao, T., et al. (2021). Exosome-mediated delivery of kartogenin for chondrogenesis of synovial fluid-derived mesenchymal stem cells and cartilage regeneration. Biomaterials 269, 120539. doi:10.1016/j.biomaterials.2020.120539
Yaghoubi, Y., Movassaghpour, A., Zamani, M., Talebi, M., Mehdizadeh, A., and Yousefi, M. (2019). Human umbilical cord mesenchymal stem cells derived-exosomes in diseases treatment. Life Sci. 233, 116733. doi:10.1016/j.lfs.2019.116733
Yan, C., Wang, Y., Shen, X. Y., Yang, G., Jian, J., Wang, H. S., et al. (2011). MicroRNA regulation associated chondrogenesis of mouse MSCs grown on polyhydroxyalkanoates. Biomaterials 32, 6435–6444. doi:10.1016/j.biomaterials.2011.05.031
Zeng, J., Sun, P., Zhao, Y., Fang, X., Wu, Z., and Qi, X. (2023). Bone mesenchymal stem cell-derived exosomes involved co-delivery and synergism effect with icariin via mussel-inspired multifunctional hydrogel for cartilage protection. Asian J. Pharm. Sci. 18, 100799. doi:10.1016/j.ajps.2023.100799
Zhang, F. X., Liu, P., Ding, W., Meng, Q. B., Su, D. H., Zhang, Q. C., et al. (2021). Injectable Mussel-Inspired highly adhesive hydrogel with exosomes for endogenous cell recruitment and cartilage defect regeneration. Biomaterials 278, 121169. doi:10.1016/j.biomaterials.2021.121169
Zhang, K., Zhao, X., Chen, X., Wei, Y., Du, W., Wang, Y., et al. (2018a). Enhanced therapeutic effects of mesenchymal stem cell-derived exosomes with an injectable hydrogel for hindlimb ischemia treatment. ACS Appl. Mater Interfaces 10, 30081–30091. doi:10.1021/acsami.8b08449
Zhang, S., Chuah, S. J., Lai, R. C., Hui, J. H. P., Lim, S. K., and Toh, W. S. (2018b). MSC exosomes mediate cartilage repair by enhancing proliferation, attenuating apoptosis and modulating immune reactivity. Biomaterials 156, 16–27. doi:10.1016/j.biomaterials.2017.11.028
Zhang, Z., Zhao, S., Sun, Z., Zhai, C., Xia, J., Wen, C., et al. (2023). Enhancement of the therapeutic efficacy of mesenchymal stem cell-derived exosomes in osteoarthritis. Cell Mol. Biol. Lett. 28, 75. doi:10.1186/s11658-023-00485-2
Zhao, J., Li, X., Hu, J., Chen, F., Qiao, S., Sun, X., et al. (2019). Mesenchymal stromal cell-derived exosomes attenuate myocardial ischaemia-reperfusion injury through miR-182-regulated macrophage polarization. Cardiovasc Res. 115, 1205–1216. doi:10.1093/cvr/cvz040
Zhu, Y., Wang, Y., Zhao, B., Niu, X., Hu, B., Li, Q., et al. (2017). Comparison of exosomes secreted by induced pluripotent stem cell-derived mesenchymal stem cells and synovial membrane-derived mesenchymal stem cells for the treatment of osteoarthritis. Stem Cell Res. Ther. 8, 64. doi:10.1186/s13287-017-0510-9
Keywords: exosome, mesenchymal stem cell, osteoarthritis, drug delivery, engineered exosome
Citation: Wen S, Huang X, Ma J, Zhao G, Ma T, Chen K, Huang G, Chen J, Shi J and Wang S (2024) Exosomes derived from MSC as drug system in osteoarthritis therapy. Front. Bioeng. Biotechnol. 12:1331218. doi: 10.3389/fbioe.2024.1331218
Received: 31 October 2023; Accepted: 08 March 2024;
Published: 20 March 2024.
Edited by:
Hasan Uludag, University of Alberta, CanadaReviewed by:
Uday Chintapula, University of Pennsylvania, United StatesMadhan Jeyaraman, Dr. MGR Educational and Research Institute, India
Copyright © 2024 Wen, Huang, Ma, Zhao, Ma, Chen, Huang, Chen, Shi and Wang. This is an open-access article distributed under the terms of the Creative Commons Attribution License (CC BY). The use, distribution or reproduction in other forums is permitted, provided the original author(s) and the copyright owner(s) are credited and that the original publication in this journal is cited, in accordance with accepted academic practice. No use, distribution or reproduction is permitted which does not comply with these terms.
*Correspondence: Siqun Wang, d3NxdW42NkBzaW5hLmNvbQ==
†These authors have contributed equally to this work and share first authorship