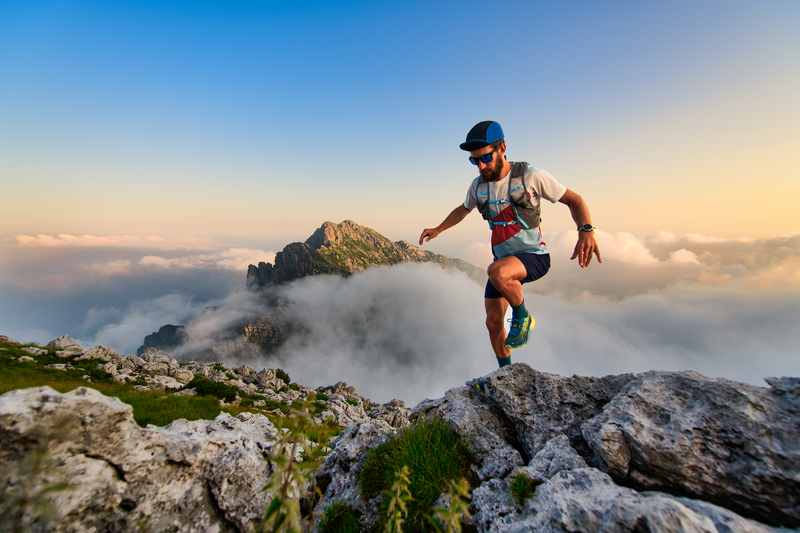
95% of researchers rate our articles as excellent or good
Learn more about the work of our research integrity team to safeguard the quality of each article we publish.
Find out more
REVIEW article
Front. Bioeng. Biotechnol. , 03 April 2024
Sec. Tissue Engineering and Regenerative Medicine
Volume 12 - 2024 | https://doi.org/10.3389/fbioe.2024.1305614
Due to high proliferative capacity, multipotent differentiation, immunomodulatory abilities, and lack of ethical concerns, dental pulp stem cells (DPSCs) are promising candidates for clinical application. Currently, clinical research on DPSCs is in its early stages. The reason for the failure to obtain clinically effective results may be problems with the production process of DPSCs. Due to the different preparation methods and reagent formulations of DPSCs, cell characteristics may be affected and lead to inconsistent experimental results. Preparation of clinical-grade DPSCs is far from ready. To achieve clinical application, it is essential to transit the manufacturing of stem cells from laboratory grade to clinical grade. This review compares and analyzes experimental data on optimizing the preparation methods of DPSCs from extraction to resuscitation, including research articles, invention patents and clinical trials. The advantages and disadvantages of various methods and potential clinical applications are discussed, and factors that could improve the quality of DPSCs for clinical application are proposed. The aim is to summarize the current manufacture of DPSCs in the establishment of a standardized, reliable, safe, and economic method for future preparation of clinical-grade cell products.
DPSCs are derived from the pulp tissue of extracted teeth and have high proliferation, multilineage differentiation capabilities, lower immunogenicity, and immunomodulatory properties compared with other mesenchymal stem cells (MSCs) (Gronthos et al., 2000; Li et al., 2014). Additionally, human-derived DPSCs can be easily isolated from extracted teeth without causing secondary injury, and there are low ethical or legal concerns regarding their therapeutic and medical uses (Bhandi et al., 2021). As a result, both academics and medical professionals are becoming increasingly interested in studying DPSCs (Huang et al., 2009; Egusa et al., 2012). Increasing evidence shows that DPSCs are a highly effective source of MSCs and are promising candidate cells for autologous stem cell therapy in the future (Xiao and Nasu, 2014).
Although the potential therapeutic benefits of DPSCs have been demonstrated in cardiovascular, neural, bone, cartilage, and immune systems in recent years, further research and clinical trials are necessary before DPSCs can be used for disease treatment. According to a 2020 review of clinical trials, only 10 clinical trials using dental pulp-derived cells were found, and they were all used for bone regeneration or dental pulp regeneration (Yamada et al., 2020). A subsequent clinical trial in Japan studied the effectiveness and safety of a single intravenous injection of human DPSCs products in the treatment of ischemic stroke within 48 h of symptom onset (Suda et al., 2022). We searched and summarized the clinical trials using odontogenic stem cells to treat diseases so far through clinicaltrials.gov and the International Clinical Trials Registry Platform. It can be seen that the number of clinical trials has increased significantly. Although most clinical trials are for the treatment of oral diseases, there are also active attempts to treat other diseases (Tables 1, 2). Although clinical research on DPSCs is still in its initial stages, judging from a large number of clinical trials of other stem cells, DPSCs are still very promising for clinical treatment (Horwitz et al., 2002; Clé et al., 2015; Suda et al., 2020).
Table 1. Summary of the number of clinical trials of odontogenic stem cells for disease treatment registered on clinicaltrials.gov.
Table 2. Summary of the number of clinical trials of odontogenic stem cells for disease treatment registered on the International Clinical Trials Registry Platform.
To provide a satisfactory amount of cells for clinical application, DPSCs should be expanded on a large scale while maintaining their functional properties, and it must be demonstrated that DPSCs are safe and effective to be fully functional in humans. About 1–2 × 105 DPSCs can be obtained from a single adult tooth (Gronthos et al., 2011), whereas to achieve desired therapeutic effect 1–10 × 109 stem cells per patient were recommended in clinical application (Migliaccio et al., 2012). Two clinical trials using primary DPSCs that were not expanded in vitro have not observed valid results. Barbier et al. (2018) evaluated the efficacy of autologous primary DPSCs delivered in collagen matrix on alveolar healing after tooth extraction. The measurement results of computed tomography and an advanced display platform showed that there was no significant difference in the degree of bone repair between the control group and the experimental group. A clinical trial in 2023 showed that the periodontal parameters of patients who were simultaneously inoculated with L-PRF and primary DPSCs in the extraction socket of the mandibular third molar were not significantly different from the group inoculated with L-PRF alone (Cubuk et al., 2023). It is a challenge to create such DPSCs in short periods using current culturing methods (Cheung et al., 2021). On the other hand, the medium and agents used in cell culture for basic and translational studies of MSCs may raise safety concerns when it is applied to patients. Worldwide guidelines, such as European Good Manufacturing Practice (EU GMP), currently advise the use of standardized protocols for cell isolation, storage, and growth (Ducret et al., 2015a). However, because most stem cell-based medicinal product manufacturing processes alter the biological characteristics of the cells and the quality of the products produced, they might not be completely satisfying (Menard and Tarte, 2013).
DPSCs undergo multiple steps from extraction to implantation, including expansion, identification, cryopreservation, and resuscitation, and each step is critical for the quality of clinical-grade DPSCs. Successful extraction of primary DPSCs from dental pulp is the prerequisite, identification of DPSCs is the guarantee, and culture and preservation without altering the biological properties of DPSCs is the key and foundation for clinical application (Figure 1). We reviewed articles and patents on optimizing methods from DPSCs extraction to resuscitation processes in the last 30 years. We analyzed the advantages and disadvantages of methodologies, and possible clinical applications, to provide new strategies for establishing standardized, reliable, safe, reproducible, and economic methods.
Figure 1. Schematic representation of optimization studies for the extraction, culture, cryopreservation, and resuscitation of DPSCs. To prepare DPSCs that conform to standards and maintain stem cell characteristics for clinical applications, optimization of many critical steps is essential. The extraction of DPSCs can be achieved using enzymatic digestion, tissue block culture, or a combination of enzymatic digestion and tissue block culture. Culture optimization of DPSCs includes not using fetal bovine serum (FBS), cultivation under low oxygen conditions, transitioning from two-dimensional (2D) culture to three-dimensional (3D) culture that is more suitable for cellular physiological environments, pathway regulation, and gene introduction for immortalization of cells. Cryopreservation optimization of DPSCs includes not using dimethyl sulfoxide (DMSO) or reducing its concentration, not using FBS, using a simpler uncontrolled-rate freezing method, freezing with a 3D scaffold, and directly freezing teeth or dental pulp tissue. Resuscitation optimization of DPSCs includes the addition of growth factors or other additives. Figure 1 was created with BioRender.com.
In 2000, Gronthos et al. (2000) used enzymatic digestion to obtain DPSCs. Pulp tissue from the human third molar was taken and cut into small pieces in a complete culture medium. To digest the pulp tissue and separate the primary DPSCs, 3 mg/mL collagenase type I and 4 mg/mL dispase were added in a 1:1 ratio. There are a variety of digestive techniques that have been proposed for pulp separation, such as those utilized by Woods et al., who substituted neutral protease for dispase and combined type I and type II collagenases (Woods et al., 2009). Furthermore, dental pulp tissue was digested with collagenase type I at 37°C for 30–45 min by Luo et al. to prepare DPSCs (Shijie, 2020). The most popular formulation was 3 mg/mL collagenase type I and 4 mg/mL dispase (Rodas-Junco and Villicana, 2017). Although the enzymatic digestion method has several advantages, such as fast detachment, high effectiveness, and high reproducibility, its low cell adhesion rate, long digestion time, the requirement for technical expertise, and the potential for enzyme-induced cell damage have hindered its application (Ohnuma et al., 2014; Ducret et al., 2015a).
Couble et al. used tissue block culture to obtain DPSCs (Couble et al., 2000). They sized the pulp tissue into small pieces with sterile surgical scissors, fixed the tissue blocks on a glass slide, and cultured them under standard conditions. DPSCs crawled out of the tissue block, proliferated and migrated throughout the culture dish. Tissue block culture method is simple, safe, and does not damage cells. However, it has a low success rate, takes a longer time to obtain a large number of DPSCs, and cannot guarantee consistent experiment results.
The enzymatic digestion method combined with the tissue block method is a combination of the above two methods. Using this method, rapidly growing cell populations emerged after only 1–2 days of primary culture (Raoof et al., 2014). The combination of the aforementioned culture methods provides a fast, effective, and safe cell culture technique.
Some authors believe that both enzymatic digestion and tissue block culture can be used to obtain a suitable population of DPSCs, and there is no significant difference between them (Hilkens et al., 2013; Fujii et al., 2015). The U.S. Food and Drug Administration has, nevertheless, offered suggestions for the minimal operational requirements for businesses that produce human cells, tissues, and cellular and tissue-based products. Processing that does not change the biological properties of the cells or tissues is referred to as minimal modification. Enzymatic digestion, according to the U.S. Food and Drug Administration, does not meet the regulatory criterion of minimal modification. In addition, EU GMP has requirements for the source and quality of enzymes, conditions of use, standardization of digestion procedures and verification of results. The conditions are better met by tissue block culture in contrast (Ducret et al., 2015a). However, there have been two clinical trials in Mexico and China using DPSCs extracted by enzymatic digestion and effectively promoting dental tissue regeneration (ISRCTN12831118, NCT 01814436) (Hernández-Monjaraz et al., 2018; Guo et al., 2021).
The method of extracting DPSCs has a significant impact on the efficiency of in vitro cultivation of DPSCs. Different extraction methods may lead to varying degrees of cell damage and a reduction in cell numbers, thereby affecting cell growth and expansion. Currently, there are relatively few research papers and invention patents focused on optimizing the primary DPSCs extraction methods. Further research is needed to develop extraction methods that meet the clinical requirements for DPSCs.
Different culture mediums can produce MSCs with different stem cell characteristics. Therefore, it is important to choose the best procedure for the proliferation of clinical-grade MSCs. The long-term culture of DPSCs has been linked to several functional changes, including reduced proliferation, altered differentiation propensity, and molecular modifications (Mehrazarin et al., 2011; Alraies et al., 2017). Fetal bovine serum (FBS) is presently added to the culture medium, which is the approach that is most frequently employed for cultivating DPSCs. FBS is a crucial additive for the in vitro culture of cells since it contains elements that can encourage cell adhesion, proliferation, and differentiation, including hormones, nutrients, and growth factors (Huang et al., 2009). Despite its widespread use, FBS has raised serious concerns. In addition to ethical and moral issues associated with the use of animal-derived products, its clinical use is limited because it is an extremely complex mixture containing many components of unknown composition. The use of FBS carries the risk of spreading harmful substances (such as prions, viruses, or zoonotic microorganisms) to the host (Mochizuki and Nakahara, 2018). Secondly, varying quantities of endotoxins, hemoglobin, and other hazardous components that can compromise the safety of a product may be present in serum (van der Valk et al., 2004). Additionally, there are differences in the quality and protein concentration of different batches of serum, which can affect experimental reproducibility (Thirumala et al., 2013). Furthermore, some scientists think that the inclusion of FBS proteins and peptides into cultured cells during the transplantation procedure causes the host to reject the transplanted cells (Spees et al., 2004; Gregory et al., 2006). And FBS is strictly rated by the European Medicines Agency and should be avoided for clinical applications (Wuchter et al., 2016). Therefore, the xenogeneic serum-free culture method for DPSCs is an important research hotspot.
Xenogeneic serum-free medium (XFM) is a type of serum-free medium (SFM), which refers to a medium that does not contain FBS or serum from other animal sources, with some growth factors, cytokines and other essential nutrients added. XFM has shown encouraging outcomes in many experiments that have grown DPSCs. Bakopoulou et al. (2017) found that in SFM, DPSCs had a similar isolation efficiency to that of alveolar bone marrow mesenchymal stem cells. The growth patterns of DPSCs that were passaged and grew continuously in serum-free circumstances were comparable to those in serum-containing conditions. At least in terms of cell growth, By calculating the population doubling time method to evaluate the proliferation ability of DPSCs in different culture media, it was found that DPSCs did not appear to be severely inhibited in two different SFMs. Mochizuki and Nakahara (2018) have shown that XFM for passaging culture exhibits significantly higher proliferative capacity than serum-containing medium. DPSCs maintained in XFM during long-term cultivation exhibited a normal karyotype in vitro and non-tumorigenic potential in vivo. In E8 medium, DPSCs showed decreased apoptosis and more proliferation, pluripotency, and migratory capacity, according to Xiao et al. (2018). Adipocytes, osteoblasts, neurons, and chondrocytes could all be successfully induced from DPSCs cultivated in E8 media. Created by James Thomson’s laboratory, E8 medium is a chemically defined, albumin-free medium originally used to grow induced pluripotent stem cells for use in medicine and research (Chen G. et al., 2011). Abdel Moniem et al. (2019) designed a cocktail of multiple supplements composed of recombinant human basic fibroblast growth factor (bFGF), insulin-transferrin-selenium, ascorbic acid, β-mercaptoethanol, and cholesterol. The proliferation rate of DPSCs cultured in the final cocktail was significantly increased, and the stem cell properties of the cells were not altered. This formulation is a potential and safe substitute for standard animal-derived supplements. A review by Fu et al. (Hengyi and Chenglin, 2022) summarized that there were no significant differences with/without serum on the stemness and differentiation of DPSCs. DPSCs cultured under serum-free situations exhibited typical MSCs characteristics both in vitro and in vivo and were more easily induced to undergo neurogenic differentiation and capillary-like formation. These results suggest that isolating and expanding DPSCs in serum-free conditions is a reliable and safe method.
As to the efficacy of the serum-free culture method, studies have presented conflicting conclusions. Tarle et al. (2011) indicated that SFM reduced the proliferation and differentiation ability of DPSCs compared to FBS culture. Qu et al. (2020) discovered that DPSCs exhibited a more elongated morphology in XFM, a lower proliferation rate following passaging, and a lower count of colony-forming units. In a study by Mochizuki and Nakahara, it was found that excessive proliferation and fusion of DPSCs should be avoided in XFM (Mochizuki and Nakahara, 2018). These cells may be susceptible to exogenous cellular toxicity from agents such as H2O2 and ultraviolet radiation, which can affect stem cell growth to some extent. This could be due to differences in donor characteristics, the composition of the culture media utilized, or variations in culture methods. Moreover, most of the XFM currently used in laboratories to culture DPSCs that comply with EU GMP standards are commercial products, and there are few published data to verify their effects. The feasibility and component improvement of using XFM in DPSCs culture require further extensive and in-depth research.
In recent years, several XFM patents have been disclosed for DPSCs cultivation with different compositions and purposes, as shown in Table 3. From publicly available invention patents, it can be seen that epidermal growth factor (EGF) and platelet-derived growth factor are the most commonly used growth factors, both of which promote cell proliferation and differentiation.
For the growth of stem cells, several human blood derivatives—including autologous or allogeneic human serum, human plasma, platelet lysate (PL), and its released factors—have been suggested as alternatives to FBS. Platelet-rich plasma supplementation aided in the expansion of DPSCs and preserved their stem cell characteristics (Wen et al., 2016; Saeed et al., 2017). After numerous passes, Ma et al. (2021) discovered that the expression of surface markers, chromosomal stability, and expansion rate of DPSCs grown in PL remained unchanged. These cells can influence immunological responses and could develop into neuronal, adipogenic, and osteogenic lineages. Marrazzo et al. (2016) found that low concentrations of PL (1%) could support the growth of DPSCs in vitro and maintain their viability. DPSCs cultured with PL had a higher rate of healing after injury and were less sensitive to the toxicity mediated by exogenous H2O2. In addition, the addition of PL was considered an appropriate strategy to promote the osteogenic and chondrogenic differentiation of DPSCs. Che et al. (Xiaohong and Qinglin, 2020) invented a method for preparing DPSCs using 5% PL and 95% basic culture medium, which achieved good results. Piva et al. (2017) found that DPSCs could be isolated and expanded to a clinical scale in an SFM containing human serum without exogenous growth factors while maintaining their characteristics of dental pulp regeneration and vascularization. Human DPSCs isolated and maintained with plasma rich in growth factors exhibited noticeably better cell stemness, according to research by Anitua et al. (2019). Growth factor-rich plasma is a platelet-rich plasma without white blood cells, which prevents inflammation from being promoted.
Human blood derivatives are considered to be safer than animal-derived products, as they do not carry the risk of animal-related diseases that could impact patient health. Moreover, human blood derivatives can be prepared through simple processes. More importantly, human blood derivatives represented by human PL are considered to comply with EU GMP requirements. However, their preparation requires a large amount of peripheral blood or cord blood, making it more expensive than animal serum. Leukocytes have been removed from human blood derivatives, but the theoretical presence of proteins and cytokines may raise concerns about immune responses, although there is no evidence that internalization of allogeneic PL proteins poses a risk of immune responses (Burnouf et al., 2016). Furthermore, the methods for extracting derivatives from human blood are not standardized, and there is no consensus so far on which specific cytokines play a major role (Astori et al., 2016). Larger animal models are needed to evaluate the therapeutic potential of MSCs cultured from human blood derivatives. Overall, human blood derivatives have several significant advantages as substitutes for animal serum in DPSCs culture. However, some limitations need to be addressed through further improvement and optimization.
The concentration of oxygen affects various cellular activities, such as cellular proliferation and differentiation. Oxygen is a crucial substrate for cellular energy production and metabolism. In vitro, oxygen concentrations of around 20% are used, which do not represent the physiological conditions of stem cell niches. Some studies have indicated that the oxygen content of bone marrow mesenchymal stem cells is between 1% and 7% (Peck et al., 2021). Hard dental tissue encircles the dental pulp tissue, and the only vascular pathway is the apical foramen, indicating that the oxygen partial pressure in the dental pulp tissue is also low (Yu et al., 2002). Even if there are not any precise experimental results to assess the physiological oxygen content in human dental pulp, it has been reported that rat incisor pulp has an oxygen concentration of about 3% (Yu et al., 2002). Hypoxia is a crucial factor in maintaining cell plasticity and self-renewal.
Environmental oxygen concentrations have been linked to many studies’ findings that cultured MSCs suffer from reduced proliferative rates, DNA damage, and early senescence (Estrada et al., 2012; Zayed et al., 2021). Numerous research teams have found that hypoxic culture has positive impacts on MSCs proliferation, differentiation, and apoptosis (Grayson et al., 2007; Hung et al., 2012; Kwon et al., 2017). In DPSCs, low oxygen conditions (5%) have been shown to increase proliferation rates, and stemness properties, and upregulate immune regulatory molecules (Ahmed et al., 2016; Werle et al., 2016; Zayed et al., 2021). Meng et al. (2022) discovered that hypoxia culture decreased both naturally occurring and artificially induced differentiation of human DPSCs while not affecting the morphology, phenotypic, or proliferation activity. Long-term normoxic culture can induce loss of stemness and cellular senescence in human DPSCs, while hypoxic culture could mitigate these effects. Additionally, hypoxia inhibited spontaneous and induced differentiation of human DPSCs. In a study by Zeng et al., a hypoxia-responsive long non-coding RNA was discovered to promote the proliferation and migration of human DPSCs through the PI3K/AKT signaling pathway (Zeng et al., 2022). Additionally, low oxygen environments can reduce the apoptosis rate and expression of reactive oxygen species in DPSCs, and increase colony formation ability and expression of antioxidant enzymes, thus significantly affecting the self-renewal of cells (Liu et al., 2019). Therefore, long-term hypoxic culture is advantageous for maintaining the biological characteristics of DPSCs and is suitable for large-scale expansion.
The most popular technique for producing cell cultures in a two-dimensional (2D) system is adherent culture, in which cells are grown on surfaces. Traditional 2D culture systems are time-consuming, slow, occupy large spaces, and are prone to contamination. Furthermore, they lack the true in vivo cellular microenvironment and may not be suitable for large-scale culture of stem cells, which could lead to the loss of stemness and differentiation potential over time (Whitfield et al., 2013; Bara et al., 2014; Carlos Sepulveda et al., 2014). Three-dimensional (3D) cell culture can imitate the 3D cell network, the interaction between cells and the extracellular matrix (ECM), and the interaction between cells, which more closely resembles the physiological environment of the cells as compared to the 2D adherent cell culture model. This is advantageous for gene expression and signal transduction within the cells. Clustered MSCs exhibit increased levels of various pluripotency markers, such as Oct4, Sox2, and Nanog, in 3D culture, which increases their capacity for self-renewal (Bartosh et al., 2010; Guo et al., 2014; Faruqu et al., 2020; Ylostalo, 2020). Additionally, MSCs have a greater capacity to develop into bone, fat, and nerve cells (Yamaguchi et al., 2014; Petrenko et al., 2017; Song et al., 2018; Kim et al., 2021), and their therapeutic effectiveness is increased by boosting the release of anti-inflammatory cytokines and chemokines, which improves their functional properties (Cui et al., 2017; Sun et al., 2018).
3D cell culture involves seeding cells onto a 3D scaffold or cultivating them into 3D aggregates (Chuhan et al., 2023), including scaffold-based and scaffold-free methods (Figure 2; Table 4).
Figure 2. 3D Culture of DPSCs. The 3D culture of DPSCs includes two methods: scaffold culture and scaffold-free culture. Scaffold culture commonly employs materials such as hydrogels, collagen fibers, alginate, and decellularized matrix. Special techniques used for scaffold-free culture include hanging drop method, magnetic levitation method, spontaneous spheroid formation, and rotational culture method. 3D cell culture can simulate the interactions between cells and the extracellular matrix, as well as cell-to-cell interactions, more closely resembling the physiological environment of cells. This is beneficial for gene expression and signal transduction within the cells and can be used for tissue engineering, organoid construction, drug screening, and other applications. Figure 2 was created with BioRender.com.
Scaffold culture refers to using scaffold materials in 3D space to construct a support structure for cell growth. The scaffold is typically composed of porous or fibrous structures made of polymers, and it is a more traditional approach to 3D cell culture. Scaffolds can provide support structures for cell growth, as well as appropriate growth environments and communication signals between cells. However, there may be issues with biocompatibility and interactions between cells and materials. Scaffold cultivation of DPSCs is more suitable for tissue engineering. Tissue engineering aims to construct artificial tissues and organs that are similar to autologous tissues to achieve medical treatment, and 3D cell culture is a critical step in achieving this goal. Combining MSCs with the right carriers or growth factors can significantly improve the capacity for tissue regeneration (Lee et al., 2019; Lew et al., 2019). The multipotent differentiation potential of DPSCs can be used in the tissue regeneration of various fields.
The culture effects of DPSCs using different scaffolds have been studied. Common materials used include hydrogels, collagen fibers, alginate, and decellularized matrix (Weidong and Runyuan, 2021). Buyuksungur et al. (2021) used a combination of poly(ε-caprolactone) (PCL) and gelatin methacrylate (GelMA) to create a 3D-printed hybrid scaffold loaded with DPSCs. DPSCs were uniformly distributed throughout the hydrogel components and had high cell viability and osteogenic differentiation ability. Salgado et al. (2020) found that DPSCs cultured dynamically in a collagen-nano-hydroxyapatite/phosphoserine biomimetic 3D scaffold were well distributed, exhibited higher activity, and had greater osteogenic differentiation ability. Kong et al. (Lixin et al., 2018) compared the effects of a 1% collagen type I scaffold and 0.25% peptide hydrogel scaffold on DPSCs proliferation and found that both scaffold materials supported good growth and normal morphology of DPSCs. Two clinical trials reported that DPSCs seeded onto collagen scaffolds could induce bone tissue regeneration in bone defects caused by periodontal disease after transplantation (Ferrarotti et al., 2018; Hernández-Monjaraz et al., 2018). Mansouri et al. (2021) prepared a graphene oxide/sodium alginate (GOSA) scaffold, and DPSCs cultured on the 3D porous scaffold showed good proliferative capacity without significant cytotoxicity. Moreover, the toxicity of DPSCs cultured in serum-rich conditions was stronger than in SFM on the scaffold, indicating the potential for DPSCs to be cultured on the scaffold under serum-free conditions for clinical use. Luzuriaga et al. (2022) combined human DPSCs with decellularized ECM scaffolds derived from porcine and human adipose tissue in vitro and processed them into 3D solid foams. The results showed that the human adipose tissue solid foam was a better non-calcifying soft tissue regeneration scaffold, while the porcine adipose tissue solid foam was a more suitable choice for mineralized bone regeneration. These decellularized ECM-derived materials can be used to create customized tissue engineering bioinks thanks to modern 3D bioprinting technologies (Dzobo et al., 2019; Kabirian and Mozafari, 2020). Guo et al. (2021) used decellularized dental matrix combined with human DPSCs aggregates to simulate the developmental microenvironment related to odontogenesis, enabling functional tooth regeneration in patients with tooth avulsion due to trauma, and supporting the continued development of human tooth roots. Foldes et al. (2021) found that cell proliferation rate was 5–10 times higher on two types of microcarriers (non-porous microcarriers: cross-linked dextran with a diameter of 190 μm; porous microcarriers: cellulose structure with a diameter of 230 μm) than those without microspheres. Both porous and non-porous microcarriers were suitable for DPSCs expansion in dynamic culture conditions. The ability to increase the culture volume in 3D space without the requirement for passaging is the use of microcarriers’ greatest benefit.
In recent years, many invention patents for 3D scaffolds used in DPSCs culture have been published, as shown in Table 5.
Scaffold-free culture refers to special culture techniques that allow cells to self-organize into a 3D growth environment without the use of external scaffold materials (Breslin and O'Driscoll, 2013). This is achieved through techniques such as hanging drop method, magnetic levitation method, spontaneous spheroid formation, and rotational culture method which take advantage of cell aggregation tendencies to form aggregates or 3D microspheres. The method is more natural and closer to the in vivo environment, allowing for better maintenance of the self-renewal and differentiation of stem cells. Additionally, it eliminates the need for complex scaffold materials, making it simpler and faster to prepare and avoiding the disadvantages of poor scaffold degradation ability and negative effects on cell growth. For large-scale in vitro development of DPSCs for clinical purposes, this culture approach is more appropriate. However, due to the lack of external scaffold structures, cell growth and differentiation may be more disorderly, requiring more complex culture techniques and conditions.
Many researchers have explored the promoting effects of different types of 3D spheroid culture on the biological characteristics of DPSCs. Pisciotta et al. (2018) found that DPSCs cultured in suspension using SFM supplemented with bFGF and EGF maintained their biological characteristics. Kawashima et al. (2017) cultured DPSCs in a 3D sphere culture system similar to embryonic stem cell suspension culture. The expression of tooth/bone differentiation markers in DPSCs was upregulated, and mineralized nodules were formed, indicating that this 3D sphere culture system may be suitable for inducing hard tissue formation. Chan et al. (2021) demonstrated that DPSCs cultured as magnetic suspension 3D spheres had significantly enhanced tri-lineage differentiation ability. These cells also displayed enhanced expression of genes associated with stemness, adipogenesis, osteogenesis, and angiogenic processes. The apoptotic effects of 3D culture were also improved by activating the MAPK and NF-kB signaling pathways, significantly boosting the therapeutic impact of DPSCs. According to the research by Bu et al., DPSCs cultured in microsphere-forming plates had higher levels of differential gene expression in regeneration-related gene categories and had a stronger capacity for multipotent differentiation than DPSCs cultured in conventional monolayer plates (Bu et al., 2020). Wu et al. (2022) found that 2,3,5,40-tetrahydroxystilbene-2-O-D-glucoside may improve DPSCs stemness when used in a rotating bioreactor. Additionally, the expression of oncogenes and apoptotic genes in DPSCs was markedly decreased. Because the rotating bioreactor overcomes the limitation of surface area, it provides more space for cell yield and can be used for large-scale production and culture of human DPSCs for tissue regeneration. In addition, Itoh et al. (2018) used thermoresponsive hydrogel to create a scaffold-free 3D structure of DPSCs, which has the necessary self-organizing ability as a transplanted tissue. It can differentiate into odontoblasts in vivo and form a vascular-rich dental pulp-like tissue. However, there are relatively few patents related to scaffold-free 3D culture of DPSCs, which poses great difficulties for its actual clinical application.
Currently, 3D-cultured stem cells are widely used in drug screening models, the construction of human organoids, tissue engineering, and other fields, and have shown excellent performance. The 3D culture of DPSCs can be closer to its natural biological environment, which can better preserve the physiological characteristics of cells, and enable DPSCs to grow and expand better. However, the 3D culture of DPSCs requires the use of specialized culture vessels and equipment, which results in relatively high costs. Controlling cell growth and expansion in a 3D environment is more challenging, thus requiring careful monitoring and regulation of the cell growth status. Additionally, several studies have demonstrated that long-term MSCs culture in 3D culture conditions can result in decreased cell proliferation and accelerated rates of cell death because of cellular aging (Mueller-Klieser, 1997; Tsai et al., 2015; Tsai et al., 2019; Son et al., 2021). Dynamic conditions can also generate air bubbles, and cell injury has been linked to the gas-liquid interface (Walls et al., 2017). Overall, DPSCs 3D culture has significant value, but there are also technical and cost-related difficulties that require more extensive and in-depth research.
New targets for controlling stem cell fate and promoting DPSCs stemness in vitro can be found by investigating the signaling pathways involved in proliferation and differentiation. In DPSCs, the Wnt and Notch pathways are critical for controlling self-renewal and differentiation (Scheller et al., 2008; Uribe-Etxebarria et al., 2017). Hanna et al. (2023) found that two small molecule GSK-3 inhibitors (Wnt signaling agonists) can promote the proliferation of human DPSCs, enhance the expression of stem cell markers, and the cells did not undergo apoptosis. Uribe-Etxebarria et al. (2020) stated that simple pharmacological treatment with Wnt-3a (Wnt ligand), perhaps in the presence of FBS, maintained DPSCs for a longer period without compromising their stem cell properties due to spontaneous osteoblast differentiation in vitro. The research group also found that N-[N-(3,5-Difluorophenacetyl)-L-alanyl]-S-phenylglycine t-butyl ester inhibited Notch and reduced the expression of core factors in DPSCs, which decreased their ability to differentiate into fully differentiated osteoblasts and adipocytes (Uribe-Etxebarria et al., 2017). In physiologically generated DPSCs, Wnt activation resulted in a rapid rise in Jagged1 transcription levels. In DPSCs culture, this elevated Jagged1 transcription may ultimately activate the Notch receptor (Uribe-Etxebarria et al., 2017).
The SCF/c-Kit signaling pathway had also been shown to play a role in the self-renewal of human DPSCs and to maintain a long-term pool of human DPSCs (Cucco et al., 2020). Nuclear factor I-C controlled Sox2 to play a role in DPSCs cell proliferation, stem cell niche maintenance, and cell fate determination (Lee et al., 2022). bFGF regulated the self-renewal of DPSCs, maintained pluripotency, inhibited mineralization, and promoted neuronal differentiation mediated by the FGFR and PLCγ pathways (Osathanon et al., 2011). Through certain MAPKs and Smad2/3 signaling pathways, as well as the production of RUNX2, TGF-β is essential for the self-renewal and differentiation of distinct stem cell sources (Park et al., 2019). Recombinant protein factors, however, have proved costly and difficult to make. Smaller molecules that are easier to synthesize play an important role in influencing cellular behavior. Through some cell signaling pathways, including Ras-GAP, ERK1, and mTOR, small compounds can change the features of DPSCs by increasing the expression of genes related to stemness and reducing their propensity to differentiate (Al-Habib et al., 2013) (Table 6).
To fully use the potential of DPSCs, selective stimulation of signaling pathways can govern cell proliferation and improve their attributes. Pathway modulation does not require genetic manipulation and is gentler than cell reprogramming, making it a potentially ideal cellular therapy approach and opening up new possibilities for the use of DPSCs in cell therapy (Uribe-Etxebarria et al., 2023). Indeed, signal pathways are typically complex networks composed of multiple molecules, and the interactions among these molecules are highly intricate. Therefore, more extensive and in-depth research is required to enhance our understanding of signal pathways and apply them to the large-scale in vitro expansion of DPSCs.
Although DPSCs have a high self-renewal capacity, their lifespan is limited in vitro after long-term culture due to the restriction of actual division numbers, ultimately leading to irreversible proliferation arrest, namely replicative senescence, which may result in loss of their stem cell potential (Mokry et al., 2010; Alraies et al., 2017; Zeng et al., 2023). Primary human DPSCs cultured to the seventh generation will enter cell senescence (Galler et al., 2006). According to another study, DPSCs can typically reach a generational height of 13 before reaching full senescence (Wilson et al., 2015). The replicative senescence of stem cells is usually thought to be caused by genomic instability following crucial telomere shortening (Yin et al., 2016). Immortalized stem cells generated through genetic modification or gene introduction have telomere repair ability and at least 200 more divisions of proliferation ability. Compared to general DPSCs, immortalized DPSCs can proliferate indefinitely, have higher biological stability, and exhibit more consistent differentiation ability and phenotype stability.
During cell division, telomerase possesses enzymatic activity to prolong and maintain telomere length. Human telomerase reverse transcriptase (hTERT) plays a crucial role in maintaining telomere length during cell division and regulating the stemness and self-renewal characteristics of stem cells (Venturini et al., 2011; Orimoto et al., 2020b). Some studies and patents have prepared immortalized DPSCs by introducing hTERT alone or in combination with other genes. Egbuniwe et al. (2011) and Egbuniwe et al. (2013) expressed hTERT in DPSCs using a retroviral vector, and the DPSCs were able to maintain the phenotype of odontoblasts and avoid senescence, possibly due to reduced p16 expression. However, some studies suggest that overexpression of hTERT alone may not be sufficient to immortalize cells (Colgin and Reddel, 1999). When Orimoto et al. tried expressing TERT alone, they were unable to produce immortalized DPSCs; instead, they created a new immortalized DPSCs line by co-expressing a mutant version of cyclin-dependent kinase 4, cyclin D1, and TERT, which preserved the original biological properties (Orimoto et al., 2020a). Ueda et al. (Minoru, 2015) provided a method for producing immortalized DPSCs by introducing four genes (TERT, bmi-1, E6, E7). The conditioned medium of immortalized DPSCs was collected and further prepared into a pharmaceutical composition for cancer therapy containing 1.5 times more insulin-like growth factor and vascular endothelial cell proliferation factor. Furthermore, Kornsuthisopon et al. (2022) immortalized human DPSCs by SV40T antigen transfection, extending their lifespan to the 25th passage with better proliferation ability. The immortalized DPSCs were endowed with enhanced proliferation, migration, and apoptotic abilities without tumorigenicity, and they displayed the same stem cell surface markers as the original DPSCs, according to Li et al. (2020) utilization of the iggyBac transposon-mediated method to stably express SV40T-Ag. It is worth noting that the immortalization of DPSCs by this method is reversible, and the cells maintain high biological characteristics after de-immortalization (Table 7).
Immortalized DPSCs can maintain stable proliferation for a long time in vitro, which is useful for long-term research and applications. However, cell immortalization often requires the use of chemical drugs or gene technologies, which may raise ethical concerns. Although some researchers have reported that hTERT-immortalized DPSC lines do not exhibit tumorigenic potential in the short term (Wilson et al., 2015; Inada et al., 2019; Li et al., 2020), long-term safety concerns such as genetic variation and tumorigenesis require continuous monitoring and control. There is a dearth of thorough descriptions of their biological behavior, and in vivo, applicability is still somewhat restricted (Li et al., 2020). Moreover, when immortalized cells gain strong proliferative ability, their differentiation capacity is greatly reduced (Wang et al., 2019; Li et al., 2020). In addition, the clinical effectiveness of Immortalized DPSCs needs to be verified through sufficient clinical trials, and the problems of large-scale production and storage need to be solved. These issues limit the current application of immortalized DPSCs, but they are expected to be resolved in the future.
Due to the temporal discrepancy between the donor and recipient in stem cell transplantation, it is difficult to ensure that the cells can be used immediately. Long-term in vitro culture of stem cells can lead to the introduction of genetic or epigenetic instability, which may alter their characteristics and functional properties, such as reduced differentiation capacity, senescence, and apoptosis, thereby affecting clinical benefits (Safwani et al., 2012; Moll et al., 2014; Turinetto et al., 2016). In addition, long-term in vitro culture is time-consuming and expensive, which highlights the importance of cryopreservation of stem cells. Cryopreservation techniques must be effective and reliable for stem cell-based therapies to be used successfully in clinical settings. Currently, most of the MSCs used clinically have been cultured in vitro and stored at low temperatures.
The cryopreservation process of cells includes dissolving a certain number of cells in cryopreservation solution, aliquoting them into cryovials, and then placing the aliquoted cryovials into −80°C or liquid nitrogen tanks for freezing. A good cryopreservation strategy should be able to overcome problems after thawing, such as poor cell recovery, reduced cell viability, and inhibited cell proliferation. Existing cryopreservation methods can maintain cells for long-term preservation without changing their stemness (Conde et al., 2016; Raik et al., 2020). The standard method for laboratory-based MSCs cryopreservation involves moderate freezing while a cryoprotectant and FBS are present to shield cells against accelerated freezing and thawing cycles (Hanley et al., 2013; Duan et al., 2018; Pilbauerova and Suchanek, 2018). However, this method is an improvement of the cryopreservation method for hematopoietic stem cells and lymphocytes and is not the optimal method for cryopreserving MSCs (Sharma et al., 2014). The optimization of stem cell cryopreservation mainly comes from the challenges of two aspects: ①whether the composition of the cryoprotectants will reduce the vitality and function of stem cells after recovery; ②safety issues (Figure 3).
Figure 3. Optimization of DPSCs Cryopreservation. The traditional method for DPSCs cryopreservation involves programmed freezing in the presence of a cryoprotectant, usually, dimethyl sulfoxide (DMSO), combined with fetal bovine serum (FBS). Optimization methods include reducing the concentration of DMSO to 3% or replacing it with other low-toxicity cryoprotectants, avoiding the use of hazardous FBS, and adopting a convenient and rapid direct freezing method, followed by storage at −80°C or in liquid nitrogen at −196°C. In addition, 3D scaffold cryopreservation can eliminate the long waiting period for patients with acute diseases, while direct cryopreservation of teeth or dental pulp tissue can reduce costs and the risk of tissue contamination. Figure 3 was created with BioRender.com.
Currently, the most commonly used cryopreservation solution for odontogenic stem cells in laboratories is a mixture of dimethyl sulfoxide (DMSO) and FBS (with or without basal culture medium) (Conde et al., 2016; Pilbauerova and Suchanek, 2018). DMSO, the best permeable cell cryoprotectant at the moment, can guard against harm from intracellular ice crystal formation, osmotic pressure fluctuations, and cell structural issues during deep cryopreservation. Cell cryopreservation uses cryoprotectants to lower the freezing point. Cryoprotectants are compounds added to the freezing medium to prevent cell damage during cryopreservation, and they are essential reagents in almost all cell cryopreservation protocols. The effectiveness and stability of cryopreserved stem cell products mainly depend on the cryoprotectants. However, DMSO is also a hazardous chemical reagent that is mostly used in cell recovery and culture operations (Nishigaki et al., 2011), which can impair the ability of frozen MSCs to proliferate and differentiate (Lindemann et al., 2014). Moreover, DMSO, being a “universal solvent,” possesses strong skin penetration and volatilization characteristics, which can pose a risk to the health of operators.
Numerous strategies have been explored to reduce the toxicity of cryoprotectants and minimize cryo-damage, including attempts to lower the concentration of DMSO and utilize other low-toxicity cryoprotectants. Demirci et al. (2014) found that preserving DPSCs in a solution containing 20 μg/mL of borate and 5% DMSO can improve their survival rate. The solution also has no impact on the expression of surface antigens or the ability to differentiate into bone and cartilage. Adding high molecular weight hyaluronic acid to the cryoprotectant can reduce the concentration of DMSO from 10% to 3% without affecting cell viability or MSCs markers, and the proliferation of DPSCs is increased (Pilbauerova et al., 2022). With a magnetic field freezing protocol, the amount of DMSO can be reduced to 3% (Lee et al., 2012a). The presence of a steady magnetic field enhances the effectiveness of DMSO-free DPSCs cryopreservation (Lin et al., 2015). Furthermore, studies have shown that DPSCs cryopreserved with 10% glycerol and 10% ethylene glycol have similar cellular characteristics to those cryopreserved with 10% DMSO (Park et al., 2017).
Furthermore, the presence of FBS greatly limits the clinical application of cryopreserved cell products (Ling et al., 2022). Researchers have also developed xenofree cryopreservation solutions, demonstrating the feasibility of FBS-free cryopreservation (Mochizuki and Nakahara, 2018). Following international standards for drug manufacture, Ducret et al. (2015b) created an innovative in vitro technique for the separation, long-term cryopreservation, and quick expansion of DPSCs. This protocol included enzyme-free cell selection, xenofree products, and SFM. A company has invented a DPSCs cryoprotectant that is free of DMSO and FBS, avoiding the potential for animal serum contamination, reducing cell apoptosis, and enabling cells to recover their activity quickly after cryopreservation (Ying, 2021).
The potential threats posed by cryoprotectants and FBS to the safety and functional characteristics of DPSCs cannot be ignored. Currently, there are many cryopreservation products available for DPSCs. However, it is unclear whether these cryoprotective solutions can be used in clinical research, which greatly hinders the development of stem cells in the therapeutic field. Therefore, it is an important foundation for further clinical application of stem cell products to explore a universal cell cryoprotectant that is free of animal-sourced ingredients and suitable for direct clinical injection, ensuring the safety, timeliness, and scalability of stem cells in clinical applications.
Another important aspect of cryopreservation is the cooling rate. A precise balance between the concentration of cryoprotectants and the cooling rate required for cryopreservation must be maintained (Dissanayake et al., 2010). Osmotic pressure causes cells to dry and shrink when they are cooled too slowly, while intracellular ice production may result from rapid cooling (Chen et al., 2011b; Marquez-Curtis et al., 2015). According to Woods et al. (2009), freezing DPSCs at a pace of −1°C/min in an isopropanol bath before moving them to liquid nitrogen is the best option for preserving them. However, programmed freezing of DPSCs is more complicated and requires higher equipment demands, and uncontrolled rate freezing is a convenient and promising method. DPSCs can survive and maintain their phenotypic characteristics in uncontrolled long-term freezing at −80°C (Raik et al., 2020). DPSCs subjected to uncontrolled freezing remained biologically and functionally stable after 1 year at −80°C, and this was independent of DMSO concentration (Kumar et al., 2015). According to Pilbauerova et al.'s analysis of the effects of uncontrolled rate freezing and 10% DMSO as a cryoprotectant on DPSCs stored for 6 and 12 months, DPSCs were able to withstand stressful situations without losing their stemness (Pilbauerova et al., 2021). The uncontrolled rate freezing method may be a better choice.
Obtaining enough stem cells for tissue engineering applications often requires several months of time-consuming, expensive in vitro growth and multiplication. A well-preserved live-cell biological material structure might therefore be created, eliminating the long waiting period and cutting down on medical expenses.
The elimination of the transplantation preparation process and the ability to obtain standardized cell products as needed are two reasons why low-temperature preservation of cell-microsphere systems is thought to be crucial for clinical translation and commercialization (Gryshkov et al., 2015; Gurruchaga et al., 2018). Human DPSCs contained in gel microspheres made by Yang et al. using an electrostatic microdroplet approach were able to adhere, disseminate, multiply, produce ECM proteins, and have a tendency to inhabit the outer layer (Yang et al., 2021). Furthermore, gradual freezing might be used to typically cryopreserve cells in the GelMA microsphere system. During cryopreservation and thawing, the GelMA microspheres kept their structural integrity, and the thawed cells continued to operate normally. Umemura et al. (Umemura et al., 2011) encapsulated DPSCs in a biocompatible material consisting of alginate, CaCO3, and glucose-d-lactone and stored them in a cryoprotectant containing DMEM, ethylene glycol, sucrose, and polyvinylpyrrolidone. The thawed DPSCs were normal in morphology, maintained multipotency, had high proliferation ability, and expressed MSC-specific markers. 3D scaffolds for cryopreservation of DPSCs hold promising prospects for future applications.
Especially for clinical usage, DPSCs isolation is expensive and time-consuming. Additionally, there is a chance of tissue contamination in operating rooms at hospitals and dentistry clinics since they lack sterile settings. Therefore, cryopreservation of whole teeth or isolated dental pulp tissues has practical value (Malekfar et al., 2016; Wang et al., 2022). By reducing the chance of contamination during surgery after tooth extraction and increasing the likelihood of successfully obtaining healthy DPSCs, this approach also preserves the phenotypic. Furthermore, 70% of DPSCs may be recovered from complete teeth after 1 month of cryopreservation in liquid nitrogen, and high-proliferative DPSCs can be produced from removed teeth kept in PBS at 4°C for up to 120 h (Perry et al., 2008). However, other investigations have revealed that only about 20% of viable DPSCs can be taken from diseased or healthy teeth that have been cryopreserved (Woods et al., 2009; Chen et al. (2011b). From the current evidence, direct cryopreservation of teeth does not yield good results and requires further exploration of feasible options. Moreover, the hard tissue of teeth can hinder the penetration of cryoprotectants into dental pulp, and cryopreservation of dental pulp tissue may be a better method (Takebe et al., 2017). Additionally, the application of a magnetic field during ultralow-temperature preservation can enhance the permeability of cryoprotectants, and magnetic cryopreservation may be a method suitable for the cryopreservation of intact teeth and dental pulp tissues (Lee et al., 2012b).
In recent years, several patents have been published for optimizing DPSCs, tooth, and dental Pulp storage, with varying compositions of added components, as shown in Table 8. From the table, it can be seen that hydroxyethyl starch and mannitol are commonly used cryoprotectants in current optimization schemes, and other components of cryopreservation solutions are mainly chemical substances or plant extracts.
In addition, factors such as freezing temperature, storage density, and storage method also affect the cryopreservation of DPSCs. Cryopreservation of stem cells offers several advantages for clinical use, including flexible treatment planning, improved product quality, and the potential for large-scale cell production (Lee et al., 2018). Furthermore, low-temperature storage can help maintain the genetic stability of cell strains and enable the non-continuous use of cells. However, additional experimental data and further research and clinical trials are needed to ensure the effectiveness and safety of this approach.
The assessment of the survival ability of thawed stem cells is crucial for clinical transplantation. Thawed stem cells often undergo apoptosis due to the activation of the caspase pathway (Desoutter et al., 2019), resulting in poor survival, proliferation, antioxidant capacity, and pluripotency. This may be due to changes in the spatial configuration of cell membrane proteins during the cryopreservation process (Desoutter et al., 2019; Dimas-Gonzalez et al., 2019). Thawing time, washing medium, temperature, centrifugation time, and centrifugation force can all affect the recovery of cells after cryopreservation (Honge et al., 2017). Rapid thawing is generally believed to reduce the chance of damage caused by local re-freezing of cells (Baboo et al., 2019).
However, there are few studies and patents focused on optimizing the recovery methods for DPSCs. Recombinant human bFGF is a type of peptide that can enhance cell proliferation and prolong cell lifespan. According to Luo et al.'s research, 20ng/mL of bFGF can dramatically boost the proliferation of thawed DPSCs by blocking apoptosis, activating the ERK pathway, up-regulating TRPC1, and other mechanisms (Luo et al., 2021). This proliferative advantage can be passed on to subsequent passages while maintaining stemness and pluripotency. Zhang et al. (Jianqiu and Xinhong, 2022) added phospholipids, musk ketone, and irisin to a basal culture medium to create a revival solution that promotes high post-thaw viability and good proliferative activity of cryopreserved DPSCs, allowing for direct use in routine expansion culture. A biotechnology company invented a DPSCs revival medium by adding EGF, transferrin, insulin, α-asarone, N-acetyl-5-methoxytryptamine, corn peptide, casein phosphopeptide, sodium cholate, citric acid zinc, and citronellol to a basal culture medium. Using this revival medium, DPSCs can be rapidly revitalized and easily expanded, facilitating clinical research (Shuang and Jie, 2022). The initial step in using DPSCs in therapeutic settings is to extract them from a cryopreserved cell bank. The effectiveness of the treatments is directly impacted by the quality of the retrieved cells. Therefore, researchers should put this aspect as one of the research priorities.
This article provides a detailed analysis of the optimization research data from the extraction to the cryopreservation process of DPSCs. Based on current evidence, the enzyme-free tissue block culture method is more compliant with international regulatory requirements for primary extraction of DPSCs, although more research is needed to overcome its limitations. Serum-free culture methods, which do not use animal serum, are safer and more suitable for large-scale expansion of DPSCs in vitro. However, the formula for SFM is diverse, and further efforts are needed to standardize it. 3D culture, which is closer to the physiological environment of cells, is more suitable for the growth and expansion of DPSCs. Scaffold culture and scaffold-free culture each have their characteristics and applications. In addition, hypoxic culture, pathway regulation, and cell immortalization all have research and application value and can be used to fully exploit the properties of DPSCs. Regarding the cryopreservation of DPSCs, the safety for clinical application can be improved by reducing the concentration of DMSO in the cryopreservation medium and omitting the addition of FBS. Consistent with SFM, the existing formulas for cryopreservation solutions are diverse, and there is still a long way to go to standardize and normalize them. In addition, an uncontrolled rate freezing method is more convenient, and 3D scaffold cryopreservation can meet clinical emergency needs, while direct freezing of teeth or dental pulp tissue is more suitable for primary medical institutions. Finally, growth factors or other additives can be added to improve the resuscitation effect and maintain the biological characteristics of DPSCs during recovery. In addition to the extraction, culture, freezing, and recovery of DPSCs mentioned above, other factors can affect the efficiency and safety of DPSCs in vitro culture, such as donor age, tooth transportation, short-term storage, immunophenotyping and selection of DPSCs, and culture dish coating.
To realize clinical applications, stem cell therapy should transition from the experimental level in the laboratory to the clinical level, which requires following a series of standards and procedures throughout the manufacturing process. It is also necessary to ensure that the raw materials and equipment used meet regulatory requirements, and have undergone sufficient quality control and validation to ensure that the produced DPSCs meet quality standards and are safe and reliable for clinical use. EU GMP has strict requirements on all aspects of product production, including quality management systems, personnel training, facilities and equipment, raw materials and reagents, process control and documentation. These may be the main obstacles that limit the application of DPSCs to clinical trials, along with the poor translation rate from laboratory to clinic. Although the techniques for isolating, amplifying, and preserving DPSCs have undergone significant advancements, more study is required to create improved techniques and optimized protocols that adhere to standardization requirements, maintain stem cell properties over time, minimize phenotypic variation, and address safety concerns associated with using DPSCs in clinical treatments. There is still much work to be done before DPSCs may be applied to clinical therapies. To solve these restrictions, researchers, physicians, and regulatory organizations must work together to create innovative approaches. It is essential to explore the potential of DPSCs in effective clinical treatments and in improving patients’ wellbeing.
XW: Conceptualization, Writing–original draft. FL: Conceptualization, Writing–original draft. SW: Investigation, Writing–original draft. WX: Investigation, Writing–original draft. JF: Visualization, Writing–original draft. RW: Visualization, Writing–original draft. YH: Writing–review and editing.
The author(s) declare that financial support was received for the research, authorship, and/or publication of this article. This study was supported by the Wuhan University of Science and Technology startup fund (Chu Tian Scholars Program) (grant number X22020024), the open laboratory fund of Hubei Province Key Laboratory of Oral and Maxillofacial Development and Regeneration (grant number 2022kqhm005), and the Hubei Provincial Health and Health Commission Research Project (grant number WJ2023M121).
The authors declare that the research was conducted in the absence of any commercial or financial relationships that could be construed as a potential conflict of interest.
All claims expressed in this article are solely those of the authors and do not necessarily represent those of their affiliated organizations, or those of the publisher, the editors and the reviewers. Any product that may be evaluated in this article, or claim that may be made by its manufacturer, is not guaranteed or endorsed by the publisher.
Abdel Moniem, E. M., El-Batran, M. M., Halawa, A. M., Gomaa, D. H., Eldeen, G. N., and Aly, R. M. (2019). Optimizing a serum-free/xeno-free culture medium for culturing and promoting the proliferation of human dental pulp stem cells. Stem cell Investig. 6, 15. doi:10.21037/sci.2019.06.05
Ahmed, N.E.-M. B., Murakami, M., Kaneko, S., and Nakashima, M. (2016). The effects of hypoxia on the stemness properties of human dental pulp stem cells (DPSCs). Sci. Rep. 6, 35476. doi:10.1038/srep35476
Al-Habib, M., Yu, Z., and Huang, G. T. J. (2013). Small molecules affect human dental pulp stem cell properties via multiple signaling pathways. Stem Cells Dev. 22 (17), 2402–2413. doi:10.1089/scd.2012.0426
Alraies, A., Alaidaroos, N. Y. A., Waddington, R. J., Moseley, R., and Sloan, A. J. (2017). Variation in human dental pulp stem cell ageing profiles reflect contrasting proliferative and regenerative capabilities. Bmc Cell Biol. 18, 12. doi:10.1186/s12860-017-0128-x
Anitua, E., Zalduendo, M., and Troya, M. (2019). Autologous plasma rich in growth factors technology for isolation and ex vivo expansion of human dental pulp stem cells for clinical translation. Regen. Med. 14 (2), 97–111. doi:10.2217/rme-2018-0066
Astori, G., Amati, E., Bambi, F., Bernardi, M., Chieregato, K., Schäfer, R., et al. (2016). Platelet lysate as a substitute for animal serum for the ex-vivo expansion of mesenchymal stem/stromal cells: present and future. Stem Cell Res. Ther. 7 (1), 93. doi:10.1186/s13287-016-0352-x
Baboo, J., Kilbride, P., Delahaye, M., Milne, S., Fonseca, F., Blanco, M., et al. (2019). The impact of varying cooling and thawing rates on the quality of cryopreserved human peripheral blood T cells. Sci. Rep. 9, 3417. doi:10.1038/s41598-019-39957-x
Bakopoulou, A., Apatzidou, D., Aggelidou, E., Gousopoulou, E., Leyhausen, G., Volk, J., et al. (2017). Isolation and prolonged expansion of oral mesenchymal stem cells under clinical-grade, GMP-compliant conditions differentially affects "stemness" properties. Stem Cell Res. Ther. 8, 247. doi:10.1186/s13287-017-0705-0
Baojian, L., and Bin, Q. (2022). Dental pulp stem cell culture medium and culture method. China patent application 202111364561 6.
Bara, J. J., Richards, R. G., Alini, M., and Stoddart, M. J. (2014). Concise review: bone marrow-derived mesenchymal stem cells change phenotype following in vitro culture: implications for basic research and the clinic. Stem Cells 32 (7), 1713–1723. doi:10.1002/stem.1649
Barbier, L., Ramos, E., Mendiola, J., Rodriguez, O., Santamaria, G., Santamaria, J., et al. (2018). Autologous dental pulp mesenchymal stem cells for inferior third molar post-extraction socket healing: a split-mouth randomised clinical trial. Med. Oral, Patol. Oral Y Cirugia Bucal 23 (4), e469–e477. doi:10.4317/medoral.22466
Bartosh, T. J., Ylostalo, J. H., Mohammadipoor, A., Bazhanov, N., Coble, K., Claypool, K., et al. (2010). Aggregation of human mesenchymal stromal cells (MSCs) into 3D spheroids enhances their antiinflammatory properties. Proc. Natl. Acad. Sci. U. S. A. 107 (31), 13724–13729. doi:10.1073/pnas.1008117107
Bhandi, S., Alkahtani, A., Mashyakhy, M., Baeshen, H. A., Mustafa, M., Chohan, H., et al. (2021). Study of optimal conditions for growth and osteogenic differentiation of dental pulp stem cells based on glucose and serum content. Saudi J. Biol. Sci. 28 (11), 6359–6364. doi:10.1016/j.sjbs.2021.06.101
Bingchang, X., Di, W., Xueyu, L., Jia, S., Fang, W., and Degang, S. (2021). Cryopreservation solution for dental pulp or dental pulp stem cells. China patent application 202111077992 4.
Breslin, S., and O'Driscoll, L. (2013). Three-dimensional cell culture: the missing link in drug discovery. Drug Discov. Today 18 (5-6), 240–249. doi:10.1016/j.drudis.2012.10.003
Bu, N.-U., Lee, H.-S., Lee, B.-N., Hwang, Y.-C., Kim, S.-Y., Chang, S. W., et al. (2020). In vitro characterization of dental pulp stem cells cultured in two microsphere-forming culture plates. J. Clin. Med. 9 (1), 242. doi:10.3390/jcm9010242
Burnouf, T., Strunk, D., Koh, M. B. C., and Schallmoser, K. (2016). Human platelet lysate: replacing fetal bovine serum as a gold standard for human cell propagation? Biomaterials 76, 371–387. doi:10.1016/j.biomaterials.2015.10.065
Buyuksungur, S., Hasirci, V., and Hasirci, N. (2021). 3D printed hybrid bone constructs of PCL and dental pulp stem cells loaded GelMA. J. Biomed. Mater. Res. Part A 109 (12), 2425–2437. doi:10.1002/jbm.a.37235
Carlos Sepulveda, J., Tome, M., Eugenia Fernandez, M., Delgado, M., Campisi, J., Bernad, A., et al. (2014). Cell senescence abrogates the therapeutic potential of human mesenchymal stem cells in the lethal endotoxemia model. Stem Cells 32 (7), 1865–1877. doi:10.1002/stem.1654
Celiz, A. D., Vining, K. H., and Mooney, D. J. (2022). Methods and compositions for dental tissue repair and/or regeneration. USA patent application 17545205.
Chan, Y.-H., Lee, Y.-C., Hung, C.-Y., Yang, P.-J., Lai, P.-C., and Feng, S.-W. (2021). Three-dimensional spheroid culture enhances multipotent differentiation and stemness capacities of human dental pulp-derived mesenchymal stem cells by modulating MAPK and NF-kB signaling pathways. Stem Cell Rev. Rep. 17 (5), 1810–1826. doi:10.1007/s12015-021-10172-4
Chen, G., Gulbranson, D. R., Hou, Z., Bolin, J. M., Ruotti, V., Probasco, M. D., et al. (2011a). Chemically defined conditions for human iPSC derivation and culture. Nat. Methods 8 (5), 424–429. doi:10.1038/nmeth.1593
Chen, Y.-K., Huang, A.H.-C., Chan, A.W.-S., Shieh, T.-Y., and Lin, L.-M. (2011b). Human dental pulp stem cells derived from different cryopreservation methods of human dental pulp tissues of diseased teeth. J. Oral Pathology Med. 40 (10), 793–800. doi:10.1111/j.1600-0714.2011.01040.x
Cheung, V. C., Peng, C.-Y., Marinic, M., Sakabe, N. J., Aneas, I., Lynch, V. J., et al. (2021). Pluripotent stem cell-derived endometrial stromal fibroblasts in a cyclic, hormone-responsive, coculture model of human decidua. Cell Rep. 35 (7), 109138. doi:10.1016/j.celrep.2021.109138
Chuhan, Z., Dongmin, Z., and Wenan, X. (2023). Cell co-culture system of dental pulp regenerative tissue engineering. Chin. J. Tissue Eng. Res. 27 (15), 2379–2384. doi:10.12307/2023.376
Clé, D. V., Santana-Lemos, B., Tellechea, M. F., Prata, K. L., Orellana, M. D., Covas, D. T., et al. (2015). Intravenous infusion of allogeneic mesenchymal stromal cells in refractory or relapsed aplastic anemia. Cytotherapy 17 (12), 1696–1705. doi:10.1016/j.jcyt.2015.09.006
Colgin, L. M., and Reddel, R. R. (1999). Telomere maintenance mechanisms and cellular immortalization. Curr. Opin. Genet. Dev. 9 (1), 97–103. doi:10.1016/s0959-437x(99)80014-8
Conde, M. C. M., Chisini, L. A., Grazioli, G., Francia, A., Carvalho, R. V. d., Alcazar, J. C. B., et al. (2016). Does cryopreservation affect the biological properties of stem cells from dental tissues? A systematic review. Braz. Dent. J. 27 (6), 633–640. doi:10.1590/0103-6440201600980
Couble, M. L., Farges, J. C., Bleicher, F., Perrat-Mabillon, B., Boudeulle, M., and Magloire, H. (2000). Odontoblast differentiation of human dental pulp cells in explant cultures. Calcif. Tissue Int. 66 (2), 129–138. doi:10.1007/pl00005833
Cubuk, S., Oduncuoglu, B. F., and Alaaddinoglu, E. E. (2023). The effect of dental pulp stem cells and L-PRF when placed into the extraction sockets of impacted mandibular third molars on the periodontal status of adjacent second molars: a split-mouth, randomized, controlled clinical trial. Oral Maxillofac. Surg. 27 (1), 59–68. doi:10.1007/s10006-022-01045-2
Cucco, C., Zhang, Z., Botero, T. M., Chiego, D. J., Castilho, R. M., and Nor, J. E. (2020). SCF/C-Kit signaling induces self-renewal of dental pulp stem cells. J. Endod. 46 (9), S56–S62. doi:10.1016/j.joen.2020.06.035
Cui, X., Hartanto, Y., and Zhang, H. (2017). Advances in multicellular spheroids formation. J. R. Soc. Interface 14 (127), 20160877. doi:10.1098/rsif.2016.0877
Demirci, S., Dogan, A., Sislii, B., and Sahin, F. (2014). Boron increases the cell viability of mesenchymal stem cells after long-term cryopreservation. Cryobiology 68 (1), 139–146. doi:10.1016/j.cryobiol.2014.01.010
Desoutter, J., Ossart, C., Lacassagne, M.-N., Regnier, A., Marolleau, J. P., and Harrivel, V. (2019). Cryopreservation and thawing of hematopoietic stem cell CD34-induced apoptosis through caspase pathway activation: key role of granulocytes. Cytotherapy 21 (6), 612–618. doi:10.1016/j.jcyt.2019.04.004
Dimas-Gonzalez, J., Nieto-Linares, A., Millan-Rocha, M., Luis Salazar-Bailon, J., Abraham Lorenzo-Moreno, B., and Rojo-Medina, J. (2019). Thawing methods do not affect cell viability of CD45+and CD34+cells, but long-term cryopreservation of umbilical cord blood units generally decreases cell viability. Transfus. Apher. Sci. 58 (2), 196–200. doi:10.1016/j.transci.2019.03.008
Dissanayake, S. C., Che, Z.-M., Choi, S.-H., Lee, S.-J., and Kim, J. (2010). Evaluation of vitrification for cryopreservation of teeth. J. periodontal & implant Sci. 40 (3), 111–118. doi:10.5051/jpis.2010.40.3.111
Duan, W., Lopez, M. J., and Hicok, K. (2018). Adult multipotent stromal cell cryopreservation: pluses and pitfalls. Veterinary Surg. 47 (1), 19–29. doi:10.1111/vsu.12730
Ducret, M., Fabre, H., Degoul, O., Atzeni, G., McGuckin, C., Forraz, N., et al. (2015a). Manufacturing of dental pulp cell-based products from human third molars: current strategies and future investigations. Front. Physiology 6, 213. doi:10.3389/fphys.2015.00213
Ducret, M., Fabre, H., Farges, J.-C., Degoul, O., Atzeni, G., McGuckin, C., et al. (2015b). Production of human dental pulp cells with a medicinal manufacturing approach. J. Endod. 41 (9), 1492–1499. doi:10.1016/j.joen.2015.05.017
Dzobo, K., Motaung, K. S. C. M., and Adesida, A. (2019). Recent trends in decellularized extracellular matrix bioinks for 3D printing: an updated review. Int. J. Mol. Sci. 20 (18), 4628. doi:10.3390/ijms20184628
Egbuniwe, O., Grant, A. D., Renton, T., and Di Silvio, L. (2013). Phenotype-independent effects of retroviral transduction in human dental pulp stem cells. Macromol. Biosci. 13 (7), 851–859. doi:10.1002/mabi.201300020
Egbuniwe, O., Idowu, B. D., Funes, J. M., Grant, A. D., Renton, T., and Di Silvio, L. (2011). P16/p53 expression and telomerase activity in immortalized human dental pulp cells. Cell Cycle 10 (22), 3912–3919. doi:10.4161/cc.10.22.18093
Egusa, H., Sonoyama, W., Nishimura, M., Atsuta, I., and Akiyama, K. (2012). Stem cells in dentistry - Part I: stem cell sources. J. Prosthodont. Res. 56 (3), 151–165. doi:10.1016/j.jpor.2012.06.001
Estrada, J. C., Albo, C., Benguria, A., Dopazo, A., Lopez-Romero, P., Carrera-Quintanar, L., et al. (2012). Culture of human mesenchymal stem cells at low oxygen tension improves growth and genetic stability by activating glycolysis. Cell Death Differ. 19 (5), 743–755. doi:10.1038/cdd.2011.172
Faruqu, F. N., Zhou, S., Sami, N., Gheidari, F., Lu, H., and Al-Jamal, K. T. (2020). Three-dimensional culture of dental pulp pluripotent-like stem cells (DPPSCs) enhances Nanog expression and provides a serum-free condition for exosome isolation. FASEB bioAdvances 2 (7), 419–433. doi:10.1096/fba.2020-00025
Ferrarotti, F., Romano, F., Gamba, M. N., Quirico, A., Giraudi, M., Audagna, M., et al. (2018). Human intrabony defect regeneration with micrografts containing dental pulp stem cells: a randomized controlled clinical trial. J. Clin. Periodontology 45 (7), 841–850. doi:10.1111/jcpe.12931
Foldes, A., Reider, H., Varga, A., Nagy, K. S., Perczel-Kovach, K., Kis-Petik, K., et al. (2021). Culturing and scaling up stem cells of dental pulp origin using microcarriers. Polymers 13 (22), 3951. doi:10.3390/polym13223951
Fujii, S., Fujimoto, K., Goto, N., Kanawa, M., Kawamoto, T., Pan, H., et al. (2015). Characteristic expression of MSX1, MSX2, TBX2 and ENTPD1 in dental pulp cells. Biomed. Rep. 3 (4), 566–572. doi:10.3892/br.2015.456
Galler, K. M., Schweikl, H., Thonemann, B., D'Souza, R. N., and Schmalz, G. (2006). Human pulp-derived cells immortalized with simian virus 40 T-antigen. Eur. J. Oral Sci. 114 (2), 138–146. doi:10.1111/j.1600-0722.2006.00327.x
Gang, C., Haitao, H., Huizhen, Q., Di, X., Jianfeng, C., and Yong, Y. (2020). Regeneration construction method based on pulp-dentin complex and biological scaffold material for constructing pulp-dentin complex structure. China patent application 102017000291125.
Gioventù, S., Frasca, S., Montelatici, E. G. A., Rebulla, P., Andriolo, G., Lazzari, L., et al. (2013). Method for dental pulp cryopreservation, European patent application 11724755.
Grayson, W. L., Zhao, F., Bunnell, B., and Ma, T. (2007). Hypoxia enhances proliferation and tissue formation of human mesenchymal stem cells. Biochem. Biophysical Res. Commun. 358 (3), 948–953. doi:10.1016/j.bbrc.2007.05.054
Gregory, C. A., Reyes, E., Whitney, M. J., and Spees, J. L. (2006). Enhanced engraftment of mesenchymal stem cells in a cutaneous wound model by culture in allogenic species-specific serum and administration in fibrin constructs. Stem Cells 24 (10), 2232–2243. doi:10.1634/stemcells.2005-0612
Gronthos, S., Arthur, A., Bartold, P. M., and Shi, S. (2011). A method to isolate and culture expand human dental pulp stem cells. Methods Mol. Biol. Clift. N.J. 698, 107–121. doi:10.1007/978-1-60761-999-4_9
Gronthos, S., Mankani, M., Brahim, J., Robey, P. G., and Shi, S. (2000). Postnatal human dental pulp stem cells (DPSCs) in vitro and in vivo. Proc. Natl. Acad. Sci. U. S. A. 97 (25), 13625–13630. doi:10.1073/pnas.240309797
Gryshkov, O., Hofmann, N., Lauterboeck, L., Pogozhykh, D., Mueller, T., and Glasmacher, B. (2015). Multipotent stromal cells derived from common marmoset Callithrix jacchus within alginate 3D environment: effect of cryopreservation procedures. Cryobiology 71 (1), 103–111. doi:10.1016/j.cryobiol.2015.05.001
Guo, H., Li, B., Wu, M., Zhao, W., He, X., Sui, B., et al. (2021). Odontogenesis-related developmental microenvironment facilitates deciduous dental pulp stem cell aggregates to revitalize an avulsed tooth. Biomaterials 279, 121223. doi:10.1016/j.biomaterials.2021.121223
Guo, L., Zhou, Y., Wang, S., and Wu, Y. (2014). Epigenetic changes of mesenchymal stem cells in three-dimensional (3D) spheroids. J. Cell. Mol. Med. 18 (10), 2009–2019. doi:10.1111/jcmm.12336
Gurruchaga, H., Saenz del Burgo, L., Hernandez, R. M., Orive, G., Selden, C., Fuller, B., et al. (2018). Advances in the slow freezing cryopreservation of microencapsulated cells. J. Control. Release 281, 119–138. doi:10.1016/j.jconrel.2018.05.016
Haijia, C., Xiaohu, G., Yifei, W., Shuai, L., and Xiaoyan, W. (2017). Dental pulp mesenchymal stem cell preparation and preparation method and application thereof. China Pat. Appl. 201610880924 4.
Haijia, C., Yifei, W., Xiaohu, G., Delong, F., and Yanyan, M. (2018). Cryopreservation liquid for dental pulp and dental pulp stem cells and cryopreservation method thereof. China patent application 201610347793 3.
Han, H., and Qianghui, L. (2021). Dental pulp stem cell culture medium and preparation method thereof. China patent application 202111364286.8.
Hanley, P. J., Mei, Z., Cabreira-Hansen, M. d.G., Klis, M., Li, W., Zhao, Y., et al. (2013). Manufacturing mesenchymal stromal cells for phase I clinical trials. Cytotherapy 15 (4), 416–422. doi:10.1016/j.jcyt.2012.09.007
Hanna, S., Aly, R., Eldeen, G. N., Velasco, A. A., and Alfayate, R. P. (2023). Small molecule GSK-3 inhibitors safely promote the proliferation and viability of human dental pulp stem cells-in vitro. Biomedicines 11 (2), 542. doi:10.3390/biomedicines11020542
Haoyu, Z., Zhenbo, S., Yuanyue, L., Yanchun, L., Ling, Y., Man, X., et al. (2023). Dental pulp mesenchymal stem cell culture medium and application method thereof. China patent application 202111394843.0.
Hengyi, F., and Chenglin, W. (2022). Research progress on serum-free culture methods of human dental pulp stem cells and cell characterization. Int. J. Stomatology 49 (02), 220–226. doi:10.7518/gjkq.2022033
Hernández-Monjaraz, B., Santiago-Osorio, E., Ledesma-Martínez, E., Alcauter-Zavala, A., and Mendoza-Núñez, V. M. (2018). Retrieval of a periodontally compromised tooth by allogeneic grafting of mesenchymal stem cells from dental pulp: a case report. J. Int. Med. Res. 46 (7), 2983–2993. doi:10.1177/0300060518773244
Hilkens, P., Gervois, P., Fanton, Y., Vanormelingen, J., Martens, W., Struys, T., et al. (2013). Effect of isolation methodology on stem cell properties and multilineage differentiation potential of human dental pulp stem cells. Cell Tissue Res. 353 (1), 65–78. doi:10.1007/s00441-013-1630-x
Honge, B. L., Petersen, M. S., Olesen, R., Moller, B. K., and Erikstrup, C. (2017). Optimizing recovery of frozen human peripheral blood mononuclear cells for flow cytometry. Plos One 12 (11), e0187440. doi:10.1371/journal.pone.0187440
Hongwu, D., Tong, L., Haodong, G., Fan, W., Rui, X., and Shiwen, D. (2023). Deciduous tooth pulp stem cell cryopreservation liquid, preparation method, cryopreservation method and application thereof. China patent application 202111321315.2.
Horwitz, E. M., Gordon, P. L., Koo, W. K. K., Marx, J. C., Neel, M. D., McNall, R. Y., et al. (2002). Isolated allogeneic bone marrow-derived mesenchymal cells engraft and stimulate growth in children with osteogenesis imperfecta: implications for cell therapy of bone. Proc. Natl. Acad. Sci. U. S. A. 99 (13), 8932–8937. doi:10.1073/pnas.132252399
Huang, G. T. J., Gronthos, S., and Shi, S. (2009). Mesenchymal stem cells derived from dental tissues vs. Those from other sources: their biology and role in regenerative medicine. J. Dent. Res. 88 (9), 792–806. doi:10.1177/0022034509340867
Hung, S.-P., Ho, J. H., Shih, Y.-R. V., Lo, T., and Lee, O. K. (2012). Hypoxia promotes proliferation and osteogenic differentiation potentials of human mesenchymal stem cells. J. Orthop. Res. 30 (2), 260–266. doi:10.1002/jor.21517
Inada, E., Saitoh, I., Kubota, N., Iwase, Y., Kiyokawa, Y., Shibasaki, S., et al. (2019). piggyBac transposon-based immortalization of human deciduous tooth dental pulp cells with multipotency and non-tumorigenic potential. Int. J. Mol. Sci. 20 (19), 4904. doi:10.3390/ijms20194904
Itoh, Y., Sasaki, J. I., Hashimoto, M., Katata, C., Hayashi, M., and Imazato, S. (2018). Pulp regeneration by 3-dimensional dental pulp stem cell constructs. J. Dent. Res. 97 (10), 1137–1143. doi:10.1177/0022034518772260
Jian, Z., Yuji, W., Bianhong, W., Xuezhu, W., Mengdi, Z., Dengsheng, X., et al. (2021). Degradable hydrogel for regenerating dental pulp and dentin regeneration. China patent application 202110883427.0.
Jianqiu, Z., and Xinhong, F. (2022). Resuscitation method of dental pulp mesenchymal stem cells. China patent application 202210163454.5. .
Jie, L., Junjie, T., and Zhenyi, W. (2023). Preparation method of stem cell three-dimensional culture biological scaffold. China patent application 202310055956.0.
Jingying, Z. (2019). Dental pulp stem cell, preparation method thereof and related bone tissue engineering material. China patent application 201610127648.4. .
Ju, Q., Guoqing, C., and Yan, Y. (2020). Dental mesenchymal stem cell culture medium and activity verification method in dental pulp stem cells. China patent application 202010170861.X.
Junjie, Z., and Yan, F. (2022). Cryopreservation method of dental pulp mesenchymal stem cells. China patent application 202210163428.2. .
Kabirian, F., and Mozafari, M. (2020). Decellularized ECM-derived bioinks: prospects for the future. Methods 171, 108–118. doi:10.1016/j.ymeth.2019.04.019
Kawashima, N., Noda, S., Yamamoto, M., and Okiji, T. (2017). Properties of dental pulp-derived mesenchymal stem cells and the effects of culture conditions. J. Endod. 43 (9), S31–S34. doi:10.1016/j.joen.2017.06.004
Kim, B.-C., Kwack, K. H., Chun, J., and Lee, J.-H. (2021). Comparative transcriptome analysis of human adipose-derived stem cells undergoing osteogenesis in 2D and 3D culture conditions. Int. J. Mol. Sci. 22 (15), 7939. doi:10.3390/ijms22157939
Kornsuthisopon, C., Nantanapiboon, D., Rochanavibhata, S., Nowwarote, N., Namangkalakul, W., and Osathanon, T. (2022). Betaine promotes osteogenic differentiation in immortalized human dental pulp-derived cells. BDJ open 8 (1), 31. doi:10.1038/s41405-022-00123-7
Kui, Y., Huayun, W., Yongjiang, M., Shuilin, X., and Ping, Y. (2019). Dental pulp stem cell separation and culture method. China patent application 201910155437.5.
Kumar, A., Bhattacharyya, S., and Rattan, V. (2015). Effect of uncontrolled freezing on biological characteristics of human dental pulp stem cells. Cell Tissue Bank. 16 (4), 513–522. doi:10.1007/s10561-015-9498-5
Kwon, S. Y., Chun, S. Y., Ha, Y.-S., Kim, D. H., Kim, J., Song, P. H., et al. (2017). Hypoxia enhances cell properties of human mesenchymal stem cells. Tissue Eng. Regen. Med. 14 (5), 595–604. doi:10.1007/s13770-017-0068-8
Lee, D.-S., Song, Y. J., Gug, H. R., Lee, J.-H., Bae, H. S., and Park, J.-C. (2022). Nuclear factor I-C regulates stemness genes and proliferation of stem cells in various mineralized tissue through epithelial-mesenchymal interactions in dental epithelial stem cells. Stem Cells Int. 2022, 1–19. doi:10.1155/2022/1092184
Lee, S.-Y., Huang, G.-W., Shiung, J.-N., Huang, Y.-H., Jeng, J.-H., Kuo, T.-F., et al. (2012a). Magnetic cryopreservation for dental pulp stem cells. Cells Tissues Organs 196 (1), 23–33. doi:10.1159/000331247
Lee, S. Y., Olsen, P., Lee, D. H., Kenoyer, A. L., Budde, L. E., O'Steen, S., et al. (2018). Preclinical optimization of a CD20-specific chimeric antigen receptor vector and culture conditions. J. Immunother. 41 (1), 19–31. doi:10.1097/cji.0000000000000199
Lee, S.-Y. S., Sun, C.-H. B., Kuo, T.-F., Huang, Y.-H., Jeng, J.-H., Yang, J.-C., et al. (2012b). Determination of cryoprotectant for magnetic cryopreservation of dental pulp tissue. Tissue Eng. Part C-Methods 18 (6), 397–407. doi:10.1089/ten.tec.2011.0363
Lee, Y.-C., Chan, Y.-H., Hsieh, S.-C., Lew, W.-Z., and Feng, S.-W. (2019). Comparing the osteogenic potentials and bone regeneration capacities of bone marrow and dental pulp mesenchymal stem cells in a rabbit calvarial bone defect model. Int. J. Mol. Sci. 20 (20), 5015. doi:10.3390/ijms20205015
Lew, W. Z., Feng, S. W., Lin, C. T., and Huang, H. M. (2019). Use of 0.4-Tesla static magnetic field to promote reparative dentine formation of dental pulp stem cells through activation of p38 MAPK signalling pathway. Int. Endod. J. 52 (1), 28–43. doi:10.1111/iej.12962
Li, X., Wang, L., Su, Q., Ye, L., Zhou, X., Song, D., et al. (2020). Highly proliferative immortalized human dental pulp cells retain the odontogenic phenotype when combined with a beta-tricalcium phosphate scaffold and BMP2. Stem cells Int. 2020, 1–18. doi:10.1155/2020/4534128
Li, Z., Jiang, C. M., An, S., Cheng, Q., Huang, Y. F., Wang, Y. T., et al. (2014). Immunomodulatory properties of dental tissue-derived mesenchymal stem cells. Oral Dis. 20 (1), 25–34. doi:10.1111/odi.12086
Lian, C., Yilin, C., Linhua, T., and Shuo, Y. (2015). Injectable tissue engineering building method for regenerating dental pulp dentin. China patent application 201410357932.1. .
Liang, Z., and Qiang, F. (2022). Additive for preserving dental pulp mesenchymal stem cells and application thereof. China patent application 202210163438.6. .
Lin, S.-L., Chang, W.-J., Lin, C.-Y., Hsieh, S.-C., Lee, S.-Y., Fan, K.-H., et al. (2015). Static magnetic field increases survival rate of dental pulp stem cells during DMSO-free cryopreservation. Electromagn. Biol. Med. 34 (4), 302–308. doi:10.3109/15368378.2014.919588
Lindemann, D., Werle, S. B., Steffens, D., Garcia-Godoy, F., Pranke, P., and Casagrande, L. (2014). Effects of cryopreservation on the characteristics of dental pulp stem cells of intact deciduous teeth. Archives Oral Biol. 59 (9), 970–976. doi:10.1016/j.archoralbio.2014.04.008
Ling, L., Yigao, X., Xiutao, Z., Meng, L., and Guojun, L. (2022). Exploration of universal cell cryopreservation solution. Chin. Med. Biotechnol. 17 (04), 347–349. doi:10.3969/j.issn.1673-713X.2022.04.009
Liu, F., Huang, X., Luo, Z., He, J., Haider, F., Son, C., et al. (2019). Hypoxia-activated PI3K/akt inhibits oxidative stress via the regulation of reactive oxygen species in human dental pulp cells. Oxidative Med. Cell. Longev. 2019, 1–10. doi:10.1155/2019/6595189
Lixin, K., Jing, L., Danyang, X., Shengnan, L., Li, A., Yumei, N., et al. (2018). Effects of gel-like scaffold on proliferation of human dental pulp stem cells. Stomatology 38 (07), 593–597. doi:10.13591/j.cnki.kqyx.2018.07.004
Luo, L., Zhang, Y., Chen, H., Hu, F., Wang, X., Xing, Z., et al. (2021). Effects and mechanisms of basic fibroblast growth factor on the proliferation and regenerative profiles of cryopreserved dental pulp stem cells. Cell Prolif. 54 (2), e12969. doi:10.1111/cpr.12969
Luzuriaga, J., Garcia-Gallastegui, P., Garcia-Urkia, N., Pineda, Jr., Irastorza, I., Fernandez-San-Argimiro, F. J., et al. (2022). Osteogenic differentiation of human dental pulp stem cells in decellularised adipose tissue solid foams. Eur. Cells Mater. 43, 112–129. doi:10.22203/ecm.v043a10
Ma, L., Huang, Z., Wu, D., Kou, X., Mao, X., and Shi, S. (2021). CD146 controls the quality of clinical grade mesenchymal stem cells from human dental pulp. Stem Cell Res. Ther. 12 (1), 488. doi:10.1186/s13287-021-02559-4
Malekfar, A., Valli, K. S., Kanafi, M. M., and Bhonde, R. R. (2016). Isolation and characterization of human dental pulp stem cells from cryopreserved pulp tissues obtained from teeth with irreversible pulpitis. J. Endod. 42 (1), 76–81. doi:10.1016/j.joen.2015.10.001
Mansouri, N., Al-Sarawi, S., Losic, D., Mazumdar, J., Clark, J., Gronthos, S., et al. (2021). Biodegradable and biocompatible graphene-based scaffolds for functional neural tissue engineering: a strategy approach using dental pulp stem cells and biomaterials. Biotechnol. Bioeng. 118 (11), 4217–4230. doi:10.1002/bit.27891
Marquez-Curtis, L. A., Janowska-Wieczorek, A., McGann, L. E., and Elliott, J. A. W. (2015). Mesenchymal stromal cells derived from various tissues: biological, clinical and cryopreservation aspects. Cryobiology 71 (2), 181–197. doi:10.1016/j.cryobiol.2015.07.003
Marrazzo, P., Paduano, F., Palmieri, F., Marrelli, M., and Tatullo, M. (2016). Highly efficient in vitro reparative behaviour of dental pulp stem cells cultured with standardised platelet lysate supplementation. Stem Cells Int. 2016, 1–16. doi:10.1155/2016/7230987
Mehrazarin, S., Oh, J. F., Chung, C. L., Chen, W., Kim, R. H., Shi, S., et al. (2011). Impaired odontogenic differentiation of senescent dental mesenchymal stem cells is associated with loss of bmi-1 expression. J. Endod. 37 (5), 662–666. doi:10.1016/j.joen.2011.02.009
Menard, C., and Tarte, K. (2013). Immunoregulatory properties of clinical grade mesenchymal stromal cells: evidence, uncertainties, and clinical application. Stem Cell Res. Ther. 4, 64. doi:10.1186/scrt214
Meng, H., Wei, F., Ge, Z., Jin, J., Wang, H., Wang, L.-s., et al. (2022). Long-term hypoxia inhibits the passage-dependent stemness decrease and senescence increase of human dental pulp stem cells. Tissue & Cell 76, 101819. doi:10.1016/j.tice.2022.101819
Mengyang, Z., and Jianwei, G. (2023). Dental pulp mesenchymal stem cell preserving fluid and application thereof. China patent application 202210163429 7.
Migliaccio, A. R., Whitsett, C., Papayannopoulou, T., and Sadelain, M. (2012). The potential of stem cells as an in vitro source of red blood cells for transfusion. Cell Stem Cell 10 (2), 115–119. doi:10.1016/j.stem.2012.01.001
Mingxin, Z., and Lei, G. (2022). Dental pulp stem cell cryopreservation liquid and cryopreservation method. China patent application 202210163401.3. .
Minoru, U. (2015). Immortalized stem cells, preparation method thereof, and medicinal composition and medicinal preparation comprising product thereof as active ingredient. European patent application 13769656. .
Mochizuki, M., and Nakahara, T. (2018). Establishment of xenogeneic serum-free culture methods for handling human dental pulp stem cells using clinically oriented in-vitro and in-vivo conditions. Stem Cell Res. Ther. 9 (1), 25. doi:10.1186/s13287-017-0761-5
Mokry, J., Soukup, T., Micuda, S., Karbanova, J., Visek, B., Brcakova, E., et al. (2010). Telomere attrition occurs during ex vivo expansion of human dental pulp stem cells. J. Biomed. Biotechnol. 2010, 1–11. doi:10.1155/2010/673513
Moll, G., Alm, J. J., Davies, L. C., von Bahr, L., Heldring, N., Stenbeck-Funke, L., et al. (2014). Do cryopreserved mesenchymal stromal cells display impaired immunomodulatory and therapeutic properties? Stem Cells 32 (9), 2430–2442. doi:10.1002/stem.1729
Mueller-Klieser, W. (1997). Three-dimensional cell cultures: from molecular mechanisms to clinical applications. Am. J. Physiology 273 (4), C1109–C1123. doi:10.1152/ajpcell.1997.273.4.C1109
Nishigaki, T., Teramura, Y., Nasu, A., Takada, K., Toguchida, J., and Iwata, H. (2011). Highly efficient cryopreservation of human induced pluripotent stem cells using a dimethyl sulfoxide-free solution. Int. J. Dev. Biol. 55 (3), 305–311. doi:10.1387/ijdb.103145tn
Ohnuma, K., Fujiki, A., Yanagihara, K., Tachikawa, S., Hayashi, Y., Ito, Y., et al. (2014). Enzyme-free passage of human pluripotent stem cells by controlling divalent cations. Sci. Rep. 4, 4646. doi:10.1038/srep04646
Orimoto, A., Kyakumoto, S., Eitsuka, T., Nakagawa, K., Kiyono, T., and Fukuda, T. (2020a). Efficient immortalization of human dental pulp stem cells with expression of cell cycle regulators with the intact chromosomal condition. PLoS One 15 (3), e0229996. doi:10.1371/journal.pone.0229996
Orimoto, A., Kyakumoto, S., Eitsuka, T., Nakagawa, K., Kiyono, T., and Fukuda, T. (2020b). Efficient immortalization of human dental pulp stem cells with expression of cell cycle regulators with the intact chromosomal condition. Plos One 15 (3), e0229996. doi:10.1371/journal.pone.0229996
Osathanon, T., Nowwarote, N., and Pavasant, P. (2011). Basic fibroblast growth factor inhibits mineralization but induces neuronal differentiation by human dental pulp stem cells through a FGFR and PLCγ signaling pathway. J. Cell. Biochem. 112 (7), 1807–1816. doi:10.1002/jcb.23097
Park, B.-W., Jang, S.-J., Byun, J.-H., Kang, Y.-H., Choi, M.-J., Park, W.-U., et al. (2017). Cryopreservation of human dental follicle tissue for use as a resource of autologous mesenchymal stem cells. J. Tissue Eng. Regen. Med. 11 (2), 489–500. doi:10.1002/term.1945
Park, K.-R., Yun, H.-M., Yeo, I. J., Cho, S., Hong, J. T., and Jeong, Y. S. (2019). Peroxiredoxin 6 inhibits osteogenic differentiation and bone formation through human dental pulp stem cells and induces delayed bone development. Antioxidants Redox Signal. 30 (17), 1969–1982. doi:10.1089/ars.2018.7530
Peck, S. H., Bendigo, J. R., Tobias, J. W., Dodge, G. R., Malhotra, N. R., Mauck, R. L., et al. (2021). Hypoxic preconditioning enhances bone marrow-derived mesenchymal stem cell survival in a low oxygen and nutrient-limited 3D microenvironment. Cartilage 12 (4), 512–525. doi:10.1177/1947603519841675
Perry, B. C., Zhou, D., Wu, X., Yang, F.-C., Byers, M. A., Chu, T. M. G., et al. (2008). Collection, cryopreservation, and characterization of human dental pulp-derived mesenchymal stem cells for banking and clinical use. Tissue Eng. Part C-Methods 14 (2), 149–156. doi:10.1089/ten.tec.2008.0031
Petrenko, Y., Sykova, E., and Kubinova, S. (2017). The therapeutic potential of three-dimensional multipotent mesenchymal stromal cell spheroids. Stem Cell Res. Ther. 8 (1), 94. doi:10.1186/s13287-017-0558-6
Pilbauerova, N., Schmidt, J., Soukup, T., Koberova Ivancakova, R., and Suchanek, J. (2021). The effects of cryogenic storage on human dental pulp stem cells. Int. J. Mol. Sci. 22 (9), 4432. doi:10.3390/ijms22094432
Pilbauerova, N., Schmidt, J., Soukup, T., Prat, T., Nesporova, K., Velebny, V., et al. (2022). Innovative approach in the cryogenic freezing medium for mesenchymal stem cells. Biomolecules 12 (5), 610. doi:10.3390/biom12050610
Pilbauerova, N., and Suchanek, J. (2018). Cryopreservation of dental stem cells. Acta medica (Hradec Kralove) 61 (1), 1–7. doi:10.14712/18059694.2018.16
Pisciotta, A., Bertoni, L., Riccio, M., Mapelli, J., Bigiani, A., La Noce, M., et al. (2018). Use of a 3D floating sphere culture system to maintain the neural crest-related properties of human dental pulp stem cells. Front. Physiology 9, 547. doi:10.3389/fphys.2018.00547
Piva, E., Tarle, S. A., Nor, J. E., Zou, D., Hatfield, E., Guinn, T., et al. (2017). Dental pulp tissue regeneration using dental pulp stem cells isolated and expanded in human serum. J. Endod. 43 (4), 568–574. doi:10.1016/j.joen.2016.11.018
Qingsong, Y., Lihua, L., Yan, H., and Ling, J. (2017). Three-dimensional active complex of dental pulp stem cells for nervous tissue engineering and three-dimensional construction method thereof. China patent application 201710626757.5.
Qingsong, Y., Pingping, Z., Jie, H., Mengting, X., Huaixing, W., and Yan, H. (2019a). Dental pulp tissue serum-free cryopreservation and resuscitation method and cryopreservation solution. China patent application 201910612817.7. .
Qingsong, Y., Pingping, Z., Ting, P., and Jie, H. (2019b). Serum-free culture medium for dental pulp stem cells. China patent application 201910260670.X.
Qingsong, Y., Xingxiang, D., Yan, H., and Wang, L. (2023). Preparation method of dental pulp mesenchymal stem cell lysis buffer with light aging resistance. China patent application 202210181905.8. .
Qu, C., Brohlin, M., Kingham, P. J., and Kelk, P. (2020). Evaluation of growth, stemness, and angiogenic properties of dental pulp stem cells cultured in cGMP xeno-/serum-free medium. Cell Tissue Res. 380 (1), 93–105. doi:10.1007/s00441-019-03160-1
Raik, S., Kumar, A., Rattan, V., Seth, S., Kaur, A., and Bhatta Charyya, S. (2020). Assessment of post-thaw quality of dental mesenchymal stromal cells after long-term cryopreservation by uncontrolled freezing. Appl. Biochem. Biotechnol. 191 (2), 728–743. doi:10.1007/s12010-019-03216-6
Raoof, M., Yaghoobi, M. M., Derakhshani, A., Kamal-Abadi, A. M., Ebrahimi, B., Abbasnejad, M., et al. (2014). A modified efficient method for dental pulp stem cell isolation. Dent. Res. J. 11 (2), 244–250.
Rodas-Junco, B. A., and Villicana, C. (2017). Dental pulp stem cells: current advances in isolation, expansion and preservation. Tissue Eng. Regen. Med. 14 (4), 333–347. doi:10.1007/s13770-017-0036-3
Saeed, M. A., Abd El-Rahman, M., Helal, M. E., Zaher, A. R., and Grawish, M. E. (2017). Efficacy of human platelet rich fibrin exudate vs fetal bovine serum on proliferation and differentiation of dental pulp stem cells. Int. J. Stem Cells 10 (1), 38–47. doi:10.15283/ijsc16067
Safwani, W. K. Z. W., Makpol, S., Sathapan, S., and Chua, K. H. (2012). The impact of long-term in vitro expansion on the senescence-associated markers of human adipose-derived stem cells. Appl. Biochem. Biotechnol. 166 (8), 2101–2113. doi:10.1007/s12010-012-9637-4
Salgado, C. L., Barrias, C. C., and Monteiro, F. J. M. (2020). Clarifying the tooth-derived stem cells behavior in a 3D biomimetic scaffold for bone tissue engineering applications. Front. Bioeng. Biotechnol. 8, 724. doi:10.3389/fbioe.2020.00724
Scheller, E. L., Chang, J., and Wang, C. Y. (2008). Wnt/β-catenin inhibits dental pulp stem cell differentiation. J. Dent. Res. 87 (2), 126–130. doi:10.1177/154405910808700206
Sharma, R. R., Pollock, K., Hubel, A., and McKenna, D. (2014). Mesenchymal stem or stromal cells: a review of clinical applications and manufacturing practices. Transfusion 54 (5), 1418–1437. doi:10.1111/trf.12421
Shijie, L. (2020). Preparation method of dental pulp stem cells. China patent application 202010011360 7.
Shu, J., Yun, Z., Xiluan, J., Shun, Y., and Zhaoxia, L. (2017). Culture medium for human dental pulp stem cells and preparation method for human dental pulp stem cells. China patent application 201710596515.6. .
Shuang, X., and Jie, L. (2022). Dental pulp mesenchymal stem cell resuscitation culture solution, preparation method and resuscitation culture method. China patent application 202011174159.7.
Son, Y.-B., Bharti, D., Kim, S.-B., Jo, C.-H., Bok, E.-Y., Lee, S.-L., et al. (2021). Comparison of pluripotency, differentiation, and mitochondrial metabolism capacity in three-dimensional spheroid formation of dental pulp-derived mesenchymal stem cells. Biomed Res. Int. 2021, 1–10. doi:10.1155/2021/5540877
Song, L., Tsai, A.-C., Yuan, X., Bejoy, J., Sart, S., Ma, T., et al. (2018). Neural differentiation of spheroids derived from human induced pluripotent stem cells-mesenchymal stem cells coculture. Tissue Eng. Part A 24 (11-12), 915–929. doi:10.1089/ten.tea.2017.0403
Songling, W., Lei, H., Donghai, C., and Jian, Z. (2022). Wnt3a-Wnt10a biological tooth root complex and preparation method thereof. China patent application 202211009573.1.
Songtao, S. (2021). Preparation method and application of stem cells. China patent application 202110557156.X.
Spees, J. L., Gregory, C. A., Singh, H., Tucker, H. A., Peister, A., Lynch, P. J., et al. (2004). Internalized antigens must be removed to prepare hypoimmunogenic mesenchymal stem cells for cell and gene therapy. Mol. Ther. J. Am. Soc. Gene Ther. 9 (5), 747–756. doi:10.1016/j.ymthe.2004.02.012
Suda, S., Nito, C., Ihara, M., Iguchi, Y., Urabe, T., Matsumaru, Y., et al. (2022). Randomised placebo-controlled multicentre trial to evaluate the efficacy and safety of JTR-161, allogeneic human dental pulp stem cells, in patients with Acute Ischaemic stRoke (J-REPAIR). BMJ Open 12 (5), e054269. doi:10.1136/bmjopen-2021-054269
Suda, S., Nito, C., Yokobori, S., Sakamoto, Y., Nakajima, M., Sowa, K., et al. (2020). Recent advances in cell-based therapies for ischemic stroke. Int. J. Mol. Sci. 21 (18), 6718. doi:10.3390/ijms21186718
Sun, Y., Wang, Y., Zhou, L., Zou, Y., Huang, G., Gao, G., et al. (2018). Spheroid-cultured human umbilical cord-derived mesenchymal stem cells attenuate hepatic ischemia-reperfusion injury in rats. Sci. Rep. 8, 2518. doi:10.1038/s41598-018-20975-0
Takebe, Y., Tatehara, S., Fukushima, T., Tokuyama-Toda, R., Yasuhara, R., Mishima, K., et al. (2017). Cryopreservation method for the effective collection of dental pulp stem cells. Tissue Eng. Part C-Methods 23 (5), 251–261. doi:10.1089/ten.tec.2016.0519
Tarle, S. A., Shi, S., and Kaigler, D. (2011). Development of a serum-free system to expand dental-derived stem cells: PDLSCs and SHEDs. J. Cell. Physiology 226 (1), 66–73. doi:10.1002/jcp.22304
Thirumala, S., Goebel, W. S., and Woods, E. J. (2013). Manufacturing and banking of mesenchymal stem cells. Expert Opin. Biol. Ther. 13 (5), 673–691. doi:10.1517/14712598.2013.763925
Tsai, A.-C., Liu, Y., Yuan, X., and Ma, T. (2015). Compaction, fusion, and functional activation of three-dimensional human mesenchymal stem cell aggregate. Tissue Eng. Part A 21 (9-10), 1705–1719. doi:10.1089/ten.tea.2014.0314
Tsai, H.-H., Yang, K.-C., Wu, M.-H., Chen, J.-C., and Tseng, C.-L. (2019). The effects of different dynamic culture systems on cell proliferation and osteogenic differentiation in human mesenchymal stem cells. Int. J. Mol. Sci. 20 (16), 4024. doi:10.3390/ijms20164024
Turinetto, V., Vitale, E., and Giachino, C. (2016). Senescence in human mesenchymal stem cells: functional changes and implications in stem cell-based therapy. Int. J. Mol. Sci. 17 (7), 1164. doi:10.3390/ijms17071164
Umemura, E., Yamada, Y., Nakamura, S., Ito, K., Hara, K., and Ueda, M. (2011). Viable cryopreserving tissue-engineered cell-biomaterial for cell banking therapy in an effective cryoprotectant. Tissue Eng. Part C-Methods 17 (8), 799–807. doi:10.1089/ten.tec.2011.0003
Unmentioned (2019). Preparation system of dental pulp mesenchymal stem cell microsphere used for transplantation. China patent application 201811085961 1.
Uribe-Etxebarria, V., Garcia-Gallastegui, P., Perez-Garrastachu, M., Casado-Andres, M., Irastorza, I., Unda, F., et al. (2020). Wnt-3a induces epigenetic remodeling in human dental pulp stem cells. Cells 9 (3), 652. doi:10.3390/cells9030652
Uribe-Etxebarria, V., Luzuriaga, J., Garcia-Gallastegui, P., Agliano, A., Unda, F., and Ibarretxe, G. (2017). Notch/Wnt cross-signalling regulates stemness of dental pulp stem cells through expression of neural crest and core pluripotency factors. Eur. Cells Mater. 34, 249–270. doi:10.22203/eCM.v034a16
Uribe-Etxebarria, V., Pineda, J. R., Garcia-Gallastegi, P., Agliano, A., Unda, F., and Ibarretxe, G. (2023). Notch and Wnt signaling modulation to enhance DPSC stemness and therapeutic potential. Int. J. Mol. Sci. 24 (8), 7389. doi:10.3390/ijms24087389
van der Valk, J., Mellor, D., Brands, R., Fischer, R., Gruber, F., Gstraunthaler, G., et al. (2004). The humane collection of fetal bovine serum and possibilities for serum-free cell and tissue culture. Toxicol. vitro Int. J. Publ. Assoc. BIBRA 18 (1), 1–12. doi:10.1016/j.tiv.2003.08.009
Venturini, L., Daidone, M. G., Motta, R., Collini, P., Spreafico, F., Terenziani, M., et al. (2011). Telomere maintenance in wilms tumors: first evidence for the presence of alternative lengthening of telomeres mechanism. Genes Chromosomes Cancer 50 (10), 823–829. doi:10.1002/gcc.20903
Walls, P. L. L., McRae, O., Natarajan, V., Johnson, C., Antoniou, C., and Bird, J. C. (2017). Quantifying the potential for bursting bubbles to damage suspended cells. Sci. Rep. 7, 15102. doi:10.1038/s41598-017-14531-5
Wang, W., Yan, M., Aarabi, G., Peters, U., Freytag, M., Gosau, M., et al. (2022). Cultivation of cryopreserved human dental pulp stem cells-A new approach to maintaining dental pulp tissue. Int. J. Mol. Sci. 23 (19), 11485. doi:10.3390/ijms231911485
Wang, Y., Chen, S., Yan, Z., and Pei, M. (2019). A prospect of cell immortalization combined with matrix microenvironmental optimization strategy for tissue engineering and regeneration. Cell Biosci. 9, 7. doi:10.1186/s13578-018-0264-9
Weidong, N., and Runyuan, L. (2021). Applications progress of 3D culture of stem cells in the research of dental pulp regeneration. J. Oral Sci. Res. 37 (11), 961–965. doi:10.13701/j.cnki.kqyxyj.2021.11.001
Wen, J., Li, H. T., Li, S. H., Li, X., and Duan, J. M. (2016). Investigation of modified platelet-rich plasma (mPRP) in promoting the proliferation and differentiation of dental pulp stem cells from deciduous teeth. Braz. J. Med. Biol. Res. 49 (10), e5373. doi:10.1590/1414-431x20165373
Werle, S. B., Chagastelles, P., Pranke, P., and Casagrande, L. (2016). The effects of hypoxia on in vitro culture of dental-derived stem cells. Archives Oral Biol. 68, 13–20. doi:10.1016/j.archoralbio.2016.03.011
Whitfield, M. J., Lee, W. C. J., and Van Vliet, K. J. (2013). Onset of heterogeneity in culture-expanded bone marrow stromal cells. Stem Cell Res. 11 (3), 1365–1377. doi:10.1016/j.scr.2013.09.004
Wilson, R., Urraca, N., Skobowiat, C., Hope, K. A., Miravalle, L., Chamberlin, R., et al. (2015). Assessment of the tumorigenic potential of spontaneously immortalized and hTERT-immortalized cultured dental pulp stem cells. Stem Cells Transl. Med. 4 (8), 905–912. doi:10.5966/sctm.2014-0196
Woods, E. J., Perry, B. C., Hockema, J. J., Larson, L., Zhou, D., and Goebel, W. S. (2009). Optimized cryopreservation method for human dental pulp-derived stem cells and their tissues of origin for banking and clinical use. Cryobiology 59 (2), 150–157. doi:10.1016/j.cryobiol.2009.06.005
Wu, Y., Chung, Y.-Y., Chin, Y.-T., Lin, C.-Y., Kuo, P.-J., Chen, T.-Y., et al. (2022). Comparison of 2,3,5,4 '-tetrahydroxystilbene-2-O-b-D-glucoside-induced proliferation and differentiation of dental pulp stem cells in 2D and 3D culture systems-gene analysis. J. Dent. Sci. 17 (1), 14–29. doi:10.1016/j.jds.2021.09.021
Wuchter, P., Vetter, M., Saffrich, R., Diehlmann, A., Bieback, K., Ho, A. D., et al. (2016). Evaluation of GMP-compliant culture media for in vitro expansion of human bone marrow mesenchymal stromal cells. Exp. Hematol. 44 (6), 508–518. doi:10.1016/j.exphem.2016.02.004
Xi, Y., Chengyou, D., and Lujun, Z. (2015). Dental pulp stem cell culture medium. China patent application 201510095430.0.
Xiao, J., Yang, D., Li, Q., Tian, W., and Guo, W. (2018). The establishment of a chemically defined serum-free culture system for human dental pulp stem cells. Stem Cell Res. Ther. 9, 191. doi:10.1186/s13287-018-0928-8
Xiao, L., and Nasu, M. (2014). From regenerative dentistry to regenerative medicine: progress, challenges, and potential applications of oral stem cells. Stem cells cloning Adv. Appl. 7, 89–99. doi:10.2147/sccaa.S51009
Xiaodong, H., Jian, W., Xiang, Z., Huaqiang, X., Caixia, S., Renjie, W., et al. (2018). Human dental pulp stem cell culture medium and preparation method thereof. China patent application 201711117629 4.
Xiaohong, C., and Qinglin, C. (2020). Preparation method of dental pulp stem cells (DPSCs). China patent application 201911129932.5.
Xiaohu, G., Haijia, C., Yifei, W., Delong, F., and Xiaoyan, W. (2017). Tooth specimen preservation liquid and application thereof and tooth specimen preservation method. China patent application 201610881470.2.
Xiaonan, Z., Fangchun, W., Yongquan, G., Xiaoyun, S., Bin, Z., and Liubing, W. (2020). Human dental pulp cell separation culture method. China patent application 202010614171.9.
Xingmei, F., Liren, L., Zhifeng, G., and Dan, H. (2017). Preparation method of dental pulp stem cell and chitosan scaffold complex. China patent application 201610893330.7.
Xiting, M., Boxun, H., Yingying, L., Liang, X., Jianghao, W., Shu, J., et al. (2022). Dental pulp mesenchymal stem cell culture method. China patent application 202211014928.6.
Yachuan, Z., Liwei, Z., Si, W., Xuedong, Z., Xin, X., and Shiqi, G. (2021). VEGF-loaded chitosan temperature-sensitive gel as well as preparation method and application thereof. China patent application 201911263012.2.
Yamada, Y., Nakamura-Yamada, S., Konoki, R., and Baba, S. (2020). Promising advances in clinical trials of dental tissue-derived cell-based regenerative medicine. Stem Cell Res. Ther. 11 (1), 175. doi:10.1186/s13287-020-01683-x
Yamaguchi, Y., Ohno, J., Sato, A., Kido, H., and Fukushima, T. (2014). Mesenchymal stem cell spheroids exhibit enhanced in-vitro and in-vivo osteoregenerative potential. Bmc Biotechnol. 14, 105. doi:10.1186/s12896-014-0105-9
Yang, T., Zhang, Q., Xie, L., Zhang, R., Qian, R., Tian, Y., et al. (2021). hDPSC-laden GelMA microspheres fabricated using electrostatic microdroplet method for endodontic regeneration. Mater. Sci. Eng. C-Materials Biol. Appl. 121, 111850. doi:10.1016/j.msec.2020.111850
Ye, D., and Chen, C. (2022). Low-temperature preserving fluid and preserving method for dental pulp stem cells. China patent application 202210840974.5.
Yelick, P. C., and Khademhosseini, A. (2023). Pulp regeneration compositions and methods of forming and using the same. USA patent application 17969990.
Yifei, W., Haijia, C., Xiaohu, G., Shuai, L., and Xiaoyan, W. (2017). Culture medium and application thereof as well as method for culturing dental pulp stem cells. China patent application 102016000877358.
Yin, Z., Wang, Q., Li, Y., Wei, H., Shi, J., and Li, A. (2016). A novel method for banking stem cells from human exfoliated deciduous teeth: lentiviral TERT immortalization and phenotypical analysis. Stem Cell Res. Ther. 7, 50. doi:10.1186/s13287-016-0309-0
Ying, Y. (2021). Dental pulp mesenchymal stem cell cryopreservation liquid and cryopreservation method. China patent application 202011198474.3.
Yu, C. Y., Boyd, N. M., Cringle, S. J., Alder, V. A., and Yu, D. Y. (2002). Oxygen distribution and consumption in rat lower incisor pulp. Archives oral Biol. 47 (7), 529–536. doi:10.1016/s0003-9969(02)00036-5
Yuansong, Y., Shubo, H., and Juan, L. (2023). Dental pulp mesenchymal stem cells, culture method and application. China patent application 202310398730.0.
Zayed, M., Iohara, K., Watanabe, H., Ishikawa, M., Tominaga, M., and Nakashima, M. (2021). Characterization of stable hypoxia-preconditioned dental pulp stem cells compared with mobilized dental pulp stem cells for application for pulp regenerative therapy. Stem Cell Res. Ther. 12 (1), 302. doi:10.1186/s13287-021-02240-w
Zeng, J., Chen, M., Yang, Y., and Wu, B. (2022). A novel hypoxic lncRNA, HRL-SC, promotes the proliferation and migration of human dental pulp stem cells through the PI3K/AKT signaling pathway. Stem Cell Res. Ther. 13 (1), 286. doi:10.1186/s13287-022-02970-5
Zeng, Y., Liu, L., Huang, D., and Song, D. (2023). Immortalized cell lines derived from dental/odontogenic tissue. Cell Tissue Res. 393 (1), 1–15. doi:10.1007/s00441-023-03767-5
Zhenfeng, Z., and Qingjing, L. (2022). Culture method for extracting dental pulp stem cells from autologous dental pulp. China patent application 202210315633.6.
Zhenhua, G., Yuanyuan, S., Changying, L., Yingxin, W., and Zhaochen, S. (2023). Method for constructing tissue engineering vascularized dental pulp by using acellular matrix and application of tissue engineering vascularized dental pulp. China patent application 202211436970.7.
Keywords: dental pulp stem cells, serum-free culture, 3D cell culture, cryopreservation, stem cell property, clinical application
Citation: Wang X, Li F, Wu S, Xing W, Fu J, Wang R and He Y (2024) Research progress on optimization of in vitro isolation, cultivation and preservation methods of dental pulp stem cells for clinical application. Front. Bioeng. Biotechnol. 12:1305614. doi: 10.3389/fbioe.2024.1305614
Received: 04 October 2023; Accepted: 19 March 2024;
Published: 03 April 2024.
Edited by:
George Alexander Truskey, Duke University, United StatesReviewed by:
Gaskon Ibarretxe, University of the Basque Country, SpainCopyright © 2024 Wang, Li, Wu, Xing, Fu, Wang and He. This is an open-access article distributed under the terms of the Creative Commons Attribution License (CC BY). The use, distribution or reproduction in other forums is permitted, provided the original author(s) and the copyright owner(s) are credited and that the original publication in this journal is cited, in accordance with accepted academic practice. No use, distribution or reproduction is permitted which does not comply with these terms.
*Correspondence: Yan He, aGVsZW4tMTEwMUBob3RtYWlsLmNvbQ==
Disclaimer: All claims expressed in this article are solely those of the authors and do not necessarily represent those of their affiliated organizations, or those of the publisher, the editors and the reviewers. Any product that may be evaluated in this article or claim that may be made by its manufacturer is not guaranteed or endorsed by the publisher.
Research integrity at Frontiers
Learn more about the work of our research integrity team to safeguard the quality of each article we publish.