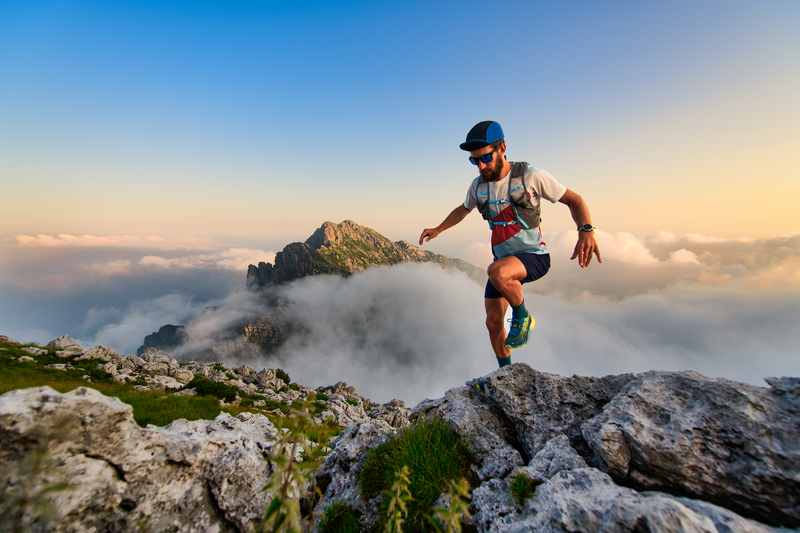
94% of researchers rate our articles as excellent or good
Learn more about the work of our research integrity team to safeguard the quality of each article we publish.
Find out more
REVIEW article
Front. Bioeng. Biotechnol. , 05 January 2024
Sec. Biomaterials
Volume 11 - 2023 | https://doi.org/10.3389/fbioe.2023.1324406
This article is part of the Research Topic Microbe Decoration and Biofabrication for Drug Delivery View all 6 articles
Abdominal aortic aneurysm (AAA) is a severe cardiovascular disease with a high mortality rate. Several screening and diagnostic methods have been developed for AAA early diagnosis. Open surgery and endovascular aortic repair (EVAR) are clinically available for patients who meet the indications for surgery. However, for non-surgical patients, limited drugs exist to inhibit or reverse the progression of aneurysms due to the complex pathogenesis and biological structure of AAA, failing to accumulate precisely on the lesion to achieve sufficient concentrations. The recently developed nanotechnology offers a new strategy to address this problem by developing drug-carrying nanoparticles with enhanced water solubility and targeting capacity, prolonged duration, and reduced side effects. Despite the rising popularity, limited literature is available to highlight the progression of the field. Herein, in this review, we first discuss the pathogenesis of AAA, the methods of diagnosis and treatment that have been applied clinically, followed by the review of research progressions of constructing different drug-loaded nanoparticles for AAA treatment using engineered nanoparticles. In addition, the feasibility of extracellular vesicles (EVs) and EVs-based nanotechnology for AAA treatment in recent years are highlighted, together with the future perspective. We hope this review will provide a clear picture for the scientists and clinicians to find a new solution for AAA clinical management.
The aorta, a sizable blood vessel originating from the heart, exhibits elasticity and undergoes expansion and contraction with each cardiac cycle. If the structural integrity of the aortic wall deteriorates over time, the potential for an aneurysm to develop arises (Baman and Eskandari, 2022). Specifically, aneurysms located in the infrarenal segment of the aorta, measuring at least 1.5 times the diameter of a healthy aorta or exceeding 30 mm in diameter, are classified as abdominal aortic aneurysms (AAA) (Golledge, 2019; Debono et al., 2021). AAA, a severe cardiovascular disease, resulted in 167,200 deaths worldwide in 2017, and 3 million disability-adjusted life years attributed to AAA (Golledge et al., 2023). However, the accuracy of statistical data is affected by the low rates of post-mortems. Nevertheless, these statistics can still serve as valuable references (Virani et al., 2020; Golledge et al., 2023). Notably, the elderly population is particularly susceptible to AAA development. Moreover, when considering gender, the risk of AAA is significantly higher in males (4%–8%) compared to females (0.5%–1.5%) (Klink et al., 2011; Schanzer and Oderich, 2021). Furthermore, prevalence rates of AAA among Black and Asian individuals are lower than those among White individuals (Chan et al., 2020; Schanzer and Oderich, 2021; Summers et al., 2021). Many pathologic factors cause degradation and dilation of the abdominal aortic vessel wall, including elevated matrix metalloproteinase (MMP) (Nosoudi et al., 2015; Bai et al., 2022), degradation of the extracellular matrix (ECM) (Rabkin, 2017), infiltration of inflammatory cells (Schmitz-Rixen et al., 2016), calcification of the vessel wall (Zimmermann, 2018), apoptosis of vascular smooth muscle cells (VSMCs) and oxidative stress (Schmitz-Rixen et al., 2016; Sanchez-Infantes et al., 2021), have been identified contributing to the development and progression of AAA.
The increased size of the aneurysm increases the risk of rupture. Therefore, early screening assumes paramount significance, leading countries like Sweden, the US, and the UK to implement ultrasound screening for high-risk populations (Golledge et al., 2023). In the initial stages, most patients with abdominal aortic aneurysms (AAA) remain undiagnosed until the manifestation of notable clinical indicators, indicating imminent rupture and necessitating elective surgical interventions such as open surgery and EVAR. Elective surgery remains the only option available for the treatment of AAA (Quaye et al., 2022). Based on the guidelines, a patient with an aortic diameter of ≥55 mm (male) or ≥50 mm (female) is suitable for elective surgery (Powell et al., 2007). However, in the case of patients with smaller diameters of AAA, surgical intervention does not necessarily lead to a higher rate of survival and may instead exacerbate the medical and psychological burden on the patient (Toczek et al., 2016). Clinical evidence supports the notion that smoking cessation and effective management of hypertension can be advantageous in delaying the growth and rupture of aneurysms (Sweeting et al., 2012; Benson et al., 2018). Conversely, the use of β-blockers and other antihypertensive medications may aid in reducing cardiovascular risk but have limited efficacy in impeding, inhibiting, or reversing the progression of AAA (Wang et al., 2018; Zhang et al., 2018; Schanzer and Oderich, 2021). This may be related to the formation of multiple layers of thrombus within the pseudolumen, which can prevent the target and release of drugs (Sivaraman et al., 2016).
AAA is induced by a combination of factors that could be targeted therapeutically. Experimental studies performed in small animal studies have shown effectiveness in inhibiting the progression of AAA (Sivaraman et al., 2016; Rabkin, 2017; Senemaud et al., 2017; Yoshimura et al., 2018; Bai et al., 2022). This inhibition was achieved through pathways that inhibit inflammatory cells, modulate the renin-angiotensin system, and reduce ECM degradation via MMP inhibitors. Recent studies have also revealed that enterobacteria and their metabolite butyric acid significantly attenuate AAA progression by reducing aortic wall neutrophil infiltration and NET formation in AAA patients, providing new insights into AAA clinical management (Tian et al., 2022). However, traditional routes of administration have been impacted by inadequate targeting capacity and side effects. As a result, an effective delivery vehicle is of utmost importance (Golledge et al., 2017).
The recent advances in engineered nanoparticles offer new strategies for achieving drug delivery. Nanoparticles, which are particles ranging from 1 to 100 nm in size and much smaller than human cells (Suri et al., 2007; Zhu et al., 2020), are functionally diverse with superior biocompatibility and enhanced permeability. They possess long-circulating potential, which is ideal for long-term therapeutics (Chen et al., 2022). However, it is important to note that engineered nanoparticles constructed too small are easily cleared from the body, have low drug loading, and some materials are toxic. Thus, optimizing the material and size of nanoparticles can improve cellular uptake while avoiding removal by the reticuloendothelial system. The surface modification enables nanoparticles to bind with specific cell receptors, achieving targeted delivery (Hoshyar et al., 2016; Jiang et al., 2017; Zhu et al., 2020). In recent years, research has widely explored engineered nanoparticle drug delivery technologies for the treatment of AAA with encouraging results. This paper summarizes the current status of constructing different drug-loaded nanoparticles to treat AAA using tissue-engineered technology. Additionally, it illustrates the feasibility of emerging EVs and EVs-based nanotechnology for the treatment of AAA. Finally, we discuss the potential application of these new technologies in AAA treatment, shedding light on a new direction for AAA clinical management.
Early research indicated that atherosclerosis plays a pivotal role in the pathogenesis of AAA (Anagnostakos and Lal, 2021). Various methodologies have been employed to investigate the pathogenesis of AAA patients, encompassing the analysis of risk factors associated with AAA diagnosis and progression in population-based screening studies, case-control inquiries, and cohort investigations, as well as the comparison of blood and tissue samples obtained from AAA patients and healthy populations (Golledge, 2019). In addition, risk factors such as age, smoking, race, and gender have a significant effect on the incidence of AAA (Salem et al., 2009; Ullery et al., 2018; Anagnostakos and Lal, 2021). It is mainly believed that AAA is an acquired, immune-driven destruction of the aortic wall (Golledge, 2019). This complex process involves several potential mechanisms that lead to the onset and progression of human disease, and there are three main types: the apoptosis of smooth muscle cells in the vessel wall, infiltration of immune cells, along the disruption and remodeling of the ECM structure (Quintana and Taylor, 2019; Weaver et al., 2022). Recent years have witnessed an increasing amount of evidence that substantiates this perspective (Weaver et al., 2022).
The normal vascular wall of the abdominal aorta is divided into three layers, with the intima formed by endothelial cells and connective tissue, and the media and externa consisting of a complex structure of the ECM, VSMCs and various vascular cells (fibroblasts, etc.) (Li et al., 2022). When arterial damage occurs, particularly affecting the media of the vessel wall, various immune cells such as macrophages (the main infiltrating cell type in the diseased vessel wall), neutrophils, B-cells, and T-cells infiltrate the affected region. This infiltration results in the release of proinflammatory cytokines and reactive oxygen species (ROSs), which subsequently promote the production of MMP and other proteases. Consequently, elastin, collagen (specifically types I and III), matrix cellular proteins, and numerous other proteins within the extracellular matrix (ECM) undergo degradation (Sakalihasan et al., 2018). This phenomenon initiates the disruption of the mid-aortic layer, apoptosis, and dysfunction of VSMCs, as well as the accumulation of additional inflammatory cells. Consequently, a detrimental cycle ensues, resulting in the gradual thinning and weakening of the vessel wall, ultimately culminating in the development of an aneurysm (Figure 1) (Davies, 1998; Nordon et al., 2011; Anagnostakos and Lal, 2021; Dang et al., 2022; Weaver et al., 2022).
FIGURE 1. Etiology and pathogenesis of AAA. Panel 1 reproduced from Ref. (Weaver et al., 2022). Copyright 2022 Elsevier.
In all commonly used rodent models of AAA, the presence of macrophages, neutrophils, B-cells, and T-cells has been observed (Rateri et al., 2011; Alsiraj et al., 2017; Lareyre et al., 2017; Yu et al., 2017; Coscas et al., 2018). Consequently, the downregulation of immune-mediated aortic destruction emerges as a crucial objective to restrict the progression of AAA. Moreover, in human samples of AAA obtained during open surgical repair, a diverse array of natural and acquired immune cells and their products are found within the aortic wall and intraluminal thrombus, providing additional evidence in favor of the inflammation-mediated theory of AAA (Lindeman et al., 2011; Koole et al., 2012; Biros et al., 2014; Zhou et al., 2015). It has also been shown that angiotensin I/II receptors in the aorta are involved in the formation and progression of AAA (Anagnostakos and Lal, 2021). This has potential clinical implications. Presently, numerous studies are being conducted to assess the efficacy of interventions such as doxycycline, tegretol, angiotensinogen-converting enzyme inhibitors (ACEI), and angiotensin receptor blockers (ARB) in mitigating the occurrence and halting the progression of AAA (Anagnostakos and Lal, 2021).
Most people with AAA are asymptomatic and their condition is only detected through relevant imaging tests for other disease diagnoses or incidentally discovered during regular health checks. As rupture of aneurysms results in a very high mortality rate, early screening is crucial to reduce mortality (Oliver-Williams et al., 2018; Sprynger et al., 2019). Ultrasound is recommended as the imaging modality of choice to screen patients with AAA (Chaikof et al., 2018). For individuals with an aneurysm diameter between 30 mm and 39 mm, ultrasound screening is recommended every 3 years. For those with a size between 40 mm and 49 mm, screening should be done annually, while those with an AAA size between 50 mm and 55 mm require screening every 6 months (Table 1) (Chaikof et al., 2018; Sprynger et al., 2019; AORN J, 2020).
The typical AAA patient usually has several risk factors, including family history, being a long-term smoker, being an older male, history of other large vessel aneurysms, and comorbidities (e.g., hypertension, hypercholesterolemia, atherosclerosis) (Ullery et al., 2018). These patients may present symptoms such as abdominal pain, low back pain, back pain, and a pulsating abdominal mass, caused by the aneurysm compressing the surrounding peritoneum and organs. In severe cases, patients may present to the emergency department with hypotension and shock due to AAA rupture. However, not all patients are symptomatic, and some symptoms may present in patients with other diseases (e.g., acute myocardial infarction, kidney stones, gastrointestinal disorders) (Moxon et al., 2010). Precise diagnosis of AAA based on physical symptoms remains a clinical challenge. Patients with suspected AAA require early diagnosis confirmation of the diagnosis. Digital Subtraction Angiography (DSA) is one of the most important imaging methods for diagnosing AAA. DSA imaging has a high accuracy for staging results and morphologic determination of AAA and also allows for intravascular therapeutic manipulation, which is not available with other imaging examinations. However, DSA is an invasive test and is not as fast as computed tomography angiography (CTA), which is first chosen clinically to accurately assess intravascular structures. Recently, CTA has also been combined with artificial intelligence, allowing for automated software analysis, enabling rapid quantitative analysis of intraluminal thrombosis and calcification (Lareyre et al., 2021). Magnetic resonance angiography (MRA) is another method of evaluating AAA that is not usually performed in emergencies and can be used as an adjunct to imaging in patients with contraindications to CT contrast (e.g., renal insufficiency and allergies) (Howell and Rabener, 2016; Chaikof et al., 2018).
Patients with symptomatic AAA and asymptomatic patients with an aneurysm size of ≥55 mm in men and ≥50 mm in women require surgical intervention (Wang et al., 2018). Surgical options for AAA include open repair surgery and EVAR. Open repair surgery is performed under general anesthesia by making a suitable abdominal incision, usually along the anterior midline to expose the aortic aneurysm. The aneurysm is then longitudinally incised to remove the thrombus, followed by implanting an arterial prosthesis into the aorta. EVAR, on the other hand, is performed under local anesthesia, where a stent graft is implanted through the femoral artery into the lesion to close the aneurysm and prevent rupture (Tsilimparis et al., 2017; Chaikof et al., 2018; Anagnostakos and Lal, 2021). In most cases, EVAR is the preferred option for AAA patients. For patients whose vascular anatomy does not meet the requirements of EVAR, open repair surgery can still be performed (Williamson and Babrowski, 2018). Additionally, open repair surgery is often used in cases of intra-aneurysmal leakage or severe graft infection after EVAR (Qrareya and Zuhaili, 2021).
One study compared EVAR with unrepaired patients who were not eligible for open repair and found that EVAR did not improve life expectancy (Sweeting et al., 2017). Several studies have also compared open repair surgery with EVAR in a randomized fashion and found that survival rates in patients with early EVAR are higher than in open repair surgery, but this advantage diminished after 4 years. In the 15-year follow-up, survival rates in patients who underwent EVAR were found to be much lower due to reoperation and secondary aneurysm rupture (Patel et al., 2016). Open surgery is highly invasive and not suitable for some patients with advanced age and severe underlying disease, resulting in higher postoperative complications and mortality. In the long term, EVAR needs to further improve its durability to increase patient survival (Haque and Bhargava, 2022).
The lack of effective drugs for AAA treatment remains a tremendous clinical challenge. The β-adrenergic receptor blocker propranolol was the first drug to treat AAAs in animal models. However, it fails to slow AAA growth in subsequent randomized controlled trials (Propanolol Aneurysm Trial, 2002; Yoshimura et al., 2018). In addition, a few meta-analyses found that statins slow the progression of atherosclerosis, affecting AAA growth and reducing the likelihood of aneurysm rupture (Takagi et al., 2012; Salata et al., 2018; Haque and Bhargava, 2022). Some researchers found a high detection rate of Chlamydia pneumonia at the lesion site in AAA patients and therefore suspected that AAA progression may be related to it. However, the antibiotic treatment did not significantly reduce AAA growth (Juvonen et al., 1997; Yoshimura et al., 2018). Doxycycline, an MMP inhibitor, has not been clinically shown to have a therapeutic effect on AAA growth in randomized testing (Meijer et al., 2013). Anti-hypertensive drugs are more likely to maintain the patient’s blood pressure within a reasonable range, preventing the aneurysm from rupturing. However, some randomized trials indicated that anti-hypertensive drugs are ineffective in treating AAA (Bicknell et al., 2016). A similar conclusion was drawn from the randomized trial of the mast cell inhibitor (Sillesen et al., 2015).
At present, the animal models of AAA are mainly mice and rats. The common methods to induce AAA formation include elastase perfusion, angiotensin II infusion, and calcium chloride (CaCl2) application.
Elastase perfusion is widely employed in the establishment of rodent models for AAA. This intricate procedure necessitates accessing the abdominal cavity and administering elastase via local injection into the infrarenal segment of the abdominal aorta (Busch et al., 2016; Yu et al., 2017; Busch et al., 2018). An obvious advantage of this model is that aneurysm formation occurs only in the portion of the aorta injected with elastase which would result in dilatation of the entire aortic wall. Another advantage is that this model allows for the observation of macrophage infiltration and heightened MMP expression within the aortic wall, closely resembling human pathology (Alan and Cassis, 2004). The limitations of this model are that the surgical procedure is difficult, involves acute injury (the time to form AAA is short), is not suitable for studies of long-term inhibition of AAA growth by drugs, and the size and severity of AAA formed varies greatly (Rd et al., 2001; Wang et al., 2014).
The induction of the AAA model through the injection of angiotensin II is another frequently employed technique (Trachet et al., 2016; Alsiraj et al., 2017). In contrast to the elastase perfusion model, the surgical procedure of this model is easy, allowing for the long-term induction of AAA through the subcutaneous implantation of an osmotic pump containing angiotensin II. Furthermore, the aortic wall exhibits inflammatory cell infiltration, lumen dilatation, and ECM degradation, mirroring the pathological characteristics observed in humans (Senemaud et al., 2017; Fashandi et al., 2018; Golledge, 2019). The limitation of this model is that the formed AAA can appear in the suprarenal portion, which contrasts with the infrarenal location typically observed in human AAA. Furthermore, the primary pathology observed in this model is aortic dissection, whereas non-dissection AAA is predominantly observed in humans (Trachet et al., 2016).
CaCl2 is also frequently used for induction in AAA models. CaCl2 is directly applied to the infrarenal aorta (Chiou et al., 2001), the difficulty of the surgical procedure between the former two models. Moreover, the resulting aortic dilatation in the CaCl2 model is relatively mild. Similar to the elastase perfusion model, the CaCl2 model also involves acute injury and is not suitable for studies of long-term drug inhibition of AAA growth (Rd et al., 2001; Wang et al., 2014). In addition, the severity of AAA observed in this model is not as pronounced as in the two former models.
Every model possesses its own set of merits and demerits, with large animal models being comparatively less utilized within the realm of AAA research. AAA progresses more rapidly in existing animal models, whereas AAA in humans usually forms by slow progression. Consequently, the selection of animal models ought to prioritize the investigation of analogous pathogenic factors to human AAA, as well as the identification of potential therapeutic interventions.
Currently, there is still no drug treatment for AAA in the clinic. The likely reason for this is the complex pathogenesis and biological structure of AAA, which prevents drugs from acting precisely at the site of injury to achieve effective concentrations. The development of engineered nanoparticles offers a new way to address the precise targeting of drugs (Hoshyar et al., 2016; Cheng et al., 2018; Zimmermann, 2018; Yin et al., 2021). The drug-loaded nanoparticles that have been used for AAA testing are summarized in Figure 2 and Table 2. We hope to find an emerging nanotechnology for innovative treatments for AAA.
MMP inhibitors, which are a promising class of drugs for the treatment of AAA, have been shown to attenuate the degradation of ECM, but their use in the clinical treatment of AAA remains unsuccessful. The lack of control of dosage at the lesion resulting in toxicity (high doses) or ineffectiveness (low doses), together with poor water solubility and short circulation time, contributes to the unsatisfactory clinical outcome of drug-based therapy (Ding et al., 2005). By combining nanoparticles and MMP inhibitors, researchers have developed a new drug delivery system that allows aggregation of drug-laden nanoparticles with targeting capacity to the lesion (Bartoli et al., 2006; Yamawaki-Ogata et al., 2010; Nosoudi et al., 2015).
Batimastat is a small molecule MMP inhibitor. Sinha et al. developed an elastin antibody-modified poly (ethylene glycol)-poly (lactic acid) nanoparticles (EL-PEG-PLA) (Figure 3A) (Sinha et al., 2014a), loaded with Batimastat. In the CaCl2-induced rat AAA model, compared to free Batimastat, Batimastat-loaded EL-PEG-PLA nanoparticles effectively inhibited MMP activity, reduced elastin degradation, and prevented aneurysm growth (Sinha et al., 2014a; Nosoudi et al., 2015). These improvements are associated with the degradation of the fibres in the media layer of the AAA abdominal aorta, in which the amorphous elastin core proteins covered by fibres are exposed (Sakai et al., 1986; Sinha et al., 2014a). EL-PEG-PLA nanoparticles can target these proteins, aggregating at the lesion and improving bioavailability.
FIGURE 3. (A) Construct a schematic diagram of EL-PEG-PLA nanoparticles. (B) TEM and schematic diagram of PEG-PLGA nanoparticles loaded with doxycycline. Panel A reproduced from Ref. (Sinha et al., 2014a). Copyright 2014 Elsevier. Panel B reproduced from Ref. (Camardo et al., 2018). Copyright 2017 SpringerLink.
Another popular drug, doxycycline belongs to the group of tetracycline derivatives. It can inhibit the transcriptional process of genes through direct alignment with the catalytic site, thus inhibiting several MMP types (Liu et al., 2003). Even low-dose doxycycline administered systemically can cause significant side effects, so AAA patients do not benefit from clinical application (Ding et al., 2005; Sivaraman and Ramamurthi, 2013; Sylvester et al., 2013). Recent studies have found that localizing doxycycline via mini-osmotic pumps or peri-aortic foams can provide inhibition of MMP activity at very low doses (compared to systemic administration) (Bartoli et al., 2006; Yamawaki-Ogata et al., 2010). Therefore, targeting and slow release are necessary for doxycycline. Ramamurthi et al. reported that cationically functionalized poly (lactic acid-glycolic acid) (PLGA) nanoparticles containing doxycycline were able to release doxycycline locally over a long period to inhibit MMP activity and production (Sivaraman and Ramamurthi, 2013). In recent years, the same team has continued reporting several designs of doxycycline-loaded nanoparticles with a similar targeted therapeutic potential in an elastase-induced AAA rodent model (Sivaraman et al., 2017; Camardo et al., 2018). Particularly, one approach used a didodecyldimethyl ammonium bromide (DMAB) as a cationic surfactant to synthesize PEG-PLGA nanoparticles containing doxycycline through the double-emulsion technique, followed by PEGylation to extend the water solubility and circulating time. Fabricated nanoparticles were able to continuously release doxycycline at an effective dose for a long period (Figure 3B) (Camardo et al., 2018). In addition, another study used PLGA nanoparticles to encapsulate doxycycline and superparamagnetic iron oxide nanoparticles (SPION), which could be precisely directed to the lesion under the action of a magnetic field and release the drug to inhibit MMP activity (Sivaraman et al., 2017). These methods all provide continued release of doxycycline over 2 months, inhibiting MMP activity and promoting the preservation of the elastic matrix. May provide a non-surgical treatment option for AAA patients (Sivaraman et al., 2017; Camardo et al., 2018).
The main location of AAA injury is the elastic layer in the media of the aortic wall. This elastic layer is abundant in elastin. To effectively rehabilitate and safeguard the compromised aortic wall affected by AAA, it is crucial to impede any additional deterioration of elastin, stabilize the remaining elastic layer, and facilitate the regeneration of elastin.
Pentagalloyl glucose (PGG) is a naturally occurring polyphenolic compound with a high affinity for elastin, which protects, stabilizes, and promotes elastin regeneration (Sinha et al., 2014b; Nosoudi et al., 2016a). To utilize PGG for AAA therapeutics, Nosoudi et al. first prepared bovine serum protein (BSA) nanoparticles using the coacervation method, followed by the synthesis of PGG-loaded BSA nanoparticles via the double-emulsion and the incorporation of elastin antibody to increase targeting (EL-BSA). The in vitro results showed that the release of PGG continued for over 10 days. In CaCl2-induced AAA rats, reduced degradation of the elastic layer was observed compared to the control (nanoparticles without PGG), demonstrating a protective effect (Nosoudi et al., 2016a). The team noted during their experiments that this strategy was not effective for the removal of calcareous deposits. Therefore, the team further developed a new strategy for solving the calcification problem in a rat model by loading ethylenediaminetetraacetic acid (EDTA) onto EL-BSA nanoparticles via the reverse emulsion technique (EL-EDTA nanoparticles). In the same animal model, EL-EDTA nanoparticles were first used for 2 weeks to reduce existing calcification deposits, then EL-BSA nanoparticles were continued for 4 weeks, and histological analyses of the animals were performed at week 12, demonstrating that this combination therapy was effective in alleviating the calcification process (Nosoudi et al., 2016b). Dhital et al. similarly developed PGG-loaded nanoparticles and studied their effectiveness in an elastase-induced AAA model in rats. The findings of the study indicate that the examined nanoparticle exhibited inhibitory effects on MMP, while also demonstrating the ability to restore the elastic matrix, stabilize, and regenerate the elastic layer (Dhital and Vyavahare, 2020). These observed outcomes may be attributed to the potential of PGG to stimulate the production of lysyl oxidase (LOX), an enzyme that facilitates the cross-linking process of cohesive elastin. The cross-linking of collagen and elastin, catalyzed by LOX, plays a crucial role in maintaining the stability of the elastin layer (Sylvester et al., 2013).
Hyaluronic acid (HA) is a biocompatible polysaccharide widely found in the ECM, and studies have shown that the tight binding of HA to multifunctional proteoglycans plays a key role in elastogenesis (Bukhari et al., 2018). The fragment size of HA influences cellular function. Particularly, HA oligomers in the 4-mer to 6-mer (756–1,220 Da) range increase elastic fibril production and stability of the elastin matrix (Kothapalli et al., 2009). However, direct administration of HA oligomers has a short half-life in the blood and is unstable in the aneurysmal environment (Fraser et al., 1984; Isnard et al., 2001). To address this issue, Ramamurthi et al. applied a double-emulsion method to develop PLGA nanoparticles loaded with HA oligomers. Administrated nanoparticles could release HA oligomers stably over 30 days, increasing the elastin matrix synthesis and significantly enhancing the activity of LOX, which is important for the stabilization of elastin layer (Sylvester et al., 2013). The team also constructed PLGA nanoparticles loaded with transforming growth factor-β1 (TGF-β1). TGF-β1 regulates cell growth, proliferation, and apoptosis, and is also an elastogenic factor (Ghadami et al., 2000; Gacchina et al., 2011). Co-culture of these nanoparticles with human aortic SMC in vitro showed a significant increase in elastin content and matrix, suggesting that TGF-β1-based PLGA nanoparticles may also be a promising strategy for the treatment of AAA (Venkataraman et al., 2016).
Inflammation is one of the main pathological characteristics of AAA. There are a variety of immune cells infiltrating the AAA vessel wall, in which macrophages are the main type (Sakalihasan et al., 2018; Zhao et al., 2021). Those macrophages are predominantly recruited to the external media of the aortic wall, leading to the release of proinflammatory cytokines. MMP and other proteases are produced, which ultimately lead to the degradation of elastin, collagen, and other proteins in the aortic wall, weakening the vessel wall and contributing to aneurysm development and progression (Raffort et al., 2017).
Rapamycin (RAP) is a potent inhibitor of inflammation and also regulates pathophysiological effects such as cell growth, senescence, and atherosclerosis (Rouer et al., 2014). Limited effectiveness of RAP in reducing the progression of aneurysms was observed in many preclinical trials. The non-specific distribution of RAP, adverse effects after administration, and poor aqueous solubility have contributed to the disappointing outcomes (Lederle, 2013; Cheng et al., 2018). To improve the targeted delivery of RAP, various strategies have been developed, including ROX responsive targeting, since ROS overproduction ROS and oxidative stress are closely associated with AAA formation (Emeto et al., 2016). In one study, Cheng et al. constructed a hierarchical functionalization strategy to develop ROS-responsive atheroma-targeting nanoparticles (oxidation-responsive β-cyclodextrin material (OxbCD)) to enable the delivery of RAP to treat AAA (Figure 4A). The cRGDfK (a cyclopeptide ligand) combined with the nanoparticles, and then coextruded pre-prepared macrophage cell membrane vesicles through a 200 nm polycarbonate membrane and coated onto the core of the nanoparticles to complete the macrophage membrane modification. The fabricated nanoparticles exhibited excellent aneurysm targeting ability due to the specific binding between ligands and macrophage membranes. More importantly, the effective aggregation of the nanoparticles at the lesion resulted in sufficient drug dosage with anti-inflammatory effects in CaCl2-induced AAA rats (Cheng et al., 2018). In another study, Shirasu et al. delivered RAP via Poly (ethylene glycol)-b-poly (γ-benzyl L-glutamate) nanoparticles (PEG-b-PBLG) (Figure 4B). In an elastase-induced AAA rat model, enhanced uptake of nanoparticles by macrophages at the AAA lesion was observed. The nanoparticles can be loaded with a low dose (0.1 mg/kg) of RAP to achieve relief of aneurysm dilatation, whereas the same dose of free RAP does not affect AAA. PEG-b-PBLG nanoparticles loaded with RAP resulted in a reduction of inflammatory cytokine production in the aneurysm wall and inhibited MMP, which contributed to the construction of a drug for the prevention and treatment of AAA (Shirasu et al., 2016).
FIGURE 4. (A) The illustration of ROS-responsive targeting nanoparticles. (B) The illustration of mixing RAP and PEG-b-PBLG. (C) The illustration of mixing pitavastatin and PEG-PLys (FPBA). Panel A reprinted (adapted) from Ref. (Cheng et al., 2018). Copyright 2018 Elsevier. Panel B reproduced from Ref. (Shirasu et al., 2016). Copyright 2016 PLOS ONE. Panel C reproduced from Ref. (Fukuhara et al., 2020). Copyright 2020 MDPI.
Besides RAP, statins are inhibitors of hydroxymethylglutaryl-coenzyme A (HMG-CoA) reductase, which has been widely used for AS treatment, because of their lipid-lowering capacity, anti-inflammatory, and anti-atherosclerotic properties. Many preclinical studies have demonstrated that statins can inhibit the progression of AAA (Steinmetz et al., 2005). However, there is a study claiming that statins are not effective in aneurysms in humans (Itoga et al., 2018), this study was based on oral administration, so it does not negate the possibility of in vivo treatment with nanoparticles loaded with statins. For instance, Fukuhara et al. fabricated poly (ethylene glycol)-b-poly (l-lysine)-phenylboronic acid (PEG-PLys (FPBA)) nanoparticles that contained pitavastatin (Figure 4C). In elastase-induced AAA rats, nanoparticles effectively inhibited AAA expansion by inhibiting macrophage and MMP-9 after intravenous injection, compared to free pitavastatin (Fukuhara et al., 2020). Similarly, Katsuki et al. used PLGA as a drug carrier to synthesize nanoparticles loaded with pitavastatin. In an angiotensin II-induced AAA mice model, intravenous administration of these drug-loaded nanoparticles was found to reduce macrophage accumulation and monocyte chemotactic protein-1 (MCP-1) expression, contributing to the inhibition of AAA formation (Katsuki et al., 2022). Excitingly, the nanoparticles constructed in this study have been tested in Phase I and Phase IIa clinical trials in healthy subjects and patients with severe limb ischemia (clinical trial registry identifier: UMIN000014940 and UMIN000019189). Notably, no significant adverse effects were observed, prompting the initiation of a future large-scale clinical trial. This provides a new strategy for the clinical translation of nanoparticle therapy for AAA (Katsuki et al., 2022). Current drug studies of statins for the prevention and treatment of AAA are limited to pitavastatin, which may be related to its strong anti-inflammatory effect (Aoki et al., 2009).
Tissue-type plasminogen activator (tPA) is a thrombolytic drug that converts plasminogen to plasmin in the blood, thereby rapidly resolving cardiovascular and cerebrovascular thrombotic events, restoring blood supply to organs, and reducing mortality (Fugate and Rabinstein, 2014). Three-quarters of AAA patients have intraluminal thrombosis (ILT) (Sun et al., 2009). However, in AAA, rapid thrombolysis is undesirable because high levels of fibrinolytic enzymes enhance MMP expression, which promotes ECM degradation. In addition, rapid thrombus lysis exposes proteases and inflammatory cells in the ILT to the post-thrombolytic lumen of the aortic wall, resulting in loss of (bio)mechanical barrier of the damaged aortic wall (Kazi et al., 2003; Sun et al., 2009). Slow and controlled thrombolysis, together with regulation of fibrinolytic enzyme production may slow AAA progression and rupture (Sivaraman et al., 2016). As a result, tPA could have the potential to alleviate the AAA. To prove the potential of tPA in AAA management, Sivaraman et al. fabricated PLGA nanoparticles loaded with tPA. Synthesized nanoparticles could slowly lyse fibrin clots in vitro without inducing proteolytic damage. The nanoparticles were further surface functionalized with cationic amphiphiles to bind to anionic fibrin clots. The results showed that slowly released tPA contributed to the gradual dissolution of ILT, which is beneficial to protect the elastic matrix within the adjacent AAA wall from disintegration. Furthermore, the porous structure of ILT resulting from tPA facilitated the penetration of therapeutic agents from the circulatory system into the AAA wall (Sivaraman et al., 2016). In vivo, the environment is more complex and the level of inflammatory activity is significantly higher than in vitro. Platelets and erythrocytes in the ILT may affect nanoparticle binding and thrombolytic effects, without achieving the desired penetration and thrombolysis.
After EVAR surgery for AAA patients, the stent-grafts can open new physical channels for main arterial blood flow and protect the aortic wall from further dilation, but they cannot treat the damage to the vessel wall that has already developed. his can predispose to common complications such as endoleak and aneurysm rupture, which need to be addressed. Drug-coated stent-grafts have been developed to provide local drug therapy, aiming to reduce the potential complications. That being said, the short drug release time at the lesion negatively impacts the therapeutic effect. To solve this issue, Yoshimura et al. have developed a novel drug-targeted stent-graft delivery system with repeated drug administration potential (Yoshimura et al., 2020). The system had two components, a liposome-based biotinylated nanoparticle and a stent-graft coated with biotinylated poly (2-hydroxyethyl methacrylate) (pHEMA) (Figure 5). The drug-loaded nanoparticles can be targeted to the stent-grafts through non-covalent interactions. After further implanted pHEMA stent-grafts into mice, they demonstrated drug release at the target site following systemic injection of drug-laden nanoparticles (Yoshimura et al., 2020). The popularity of stent-grafts provides a new strategy for continued treatment after EVAR.
FIGURE 5. (A) Diagram shows the liposome-based biotinylated drug-loaded nanoparticle. (B) Diagram shows the targeted graft coated with biotin–neutravidin complexes. Panel reprinted (adapted) from Ref. (Yoshimura et al., 2020). Copyright 2020 MDPI.
As mediators of intercellular communication, EVs are heavily involved in the physiological and pathological processes of many cardiovascular diseases (Walker et al., 2019). Many studies have found that EVs are tissue chemotactic. They can deliver biomolecules to recipient cells, and exhibit a good safety profile, which makes them ideal drug delivery vehicles (Dai et al., 2008). In addition, EVs can be harvested from cell culture conditions or biological tissues by extrusion, ultrasound, and other isolate methods. More importantly, they are also very stable in long-term cryopreservation and transportation (Yu et al., 2014; Witwer and Wolfram, 2021).
To date, several studies have explored the potential of EVs in AAA management. For example, Sajeesh et al. found that EVs appear to balance the proteolytic environment of aortic lesions and enhance TIMP activity, a natural inhibitor of MMP, with potential therapeutic value in the early treatment of AAA (Sajeesh et al., 2020). Additional studies have also found that microRNAs (miRNAs) in EVs had different effects on AAA development. Mesenchymal stem cell (MSC)-derived EVs could resolve the inflammatory response and aneurysm progression in AAA through miR-147 (Spinosa et al., 2018). Meanwhile, MiR-106a within EVs could downregulate TIMP-2 expression, restore the production of MMP, and accelerate VSMC apoptosis and ECM degradation (Han et al., 2020), leading to the occurrence of AAA. In addition, overexpression of miR-29b appears to promote AAA progression in rat models. As a result, loading the desirable cargo within EVs could be a promising strategy for AAA treatment.
Despite the many advantages of naturally secreted EVs, their use in therapy is further hampered by disadvantages, including lack of targeting, toxic reactions at high doses, and rapid clearance in vivo by the mononuclear phagocytic system (Wiklander et al., 2015; Wan et al., 2020; Witwer and Wolfram, 2021). To address these issues, natural EVs can be further modified by tissue engineering approaches. For instance, adding targeting ligands (EV display technology), and cell membrane fusion techniques could improve their targeting and circulatory delivery efficiency (Luk and Zhang, 2015; Witwer and Wolfram, 2021; Lu et al., 2022), as shown in Figure 6.
FIGURE 6. (A) Display technology for the construction of engineered EVs. (B) Cell membrane fusion technology for the construction of engineered EVs. Panel reprinted (adapted) from Ref. (Lu et al., 2022). Copyright 2022 Frontiers.
A method of EV display technology is to add targeting ligands by transfecting parent cells with fusion genes for targeting peptides and EV membrane proteins. Overexpression of fusion proteins on the surface of EV membranes moves engineered EVs directly toward the target area. However, there are many problems with EV display technology, for example, the process is very labor-intensive and time-consuming, and the added functional membrane proteins are sometimes degraded by proteases of cells or body fluids, losing their targeting properties. Enhancing the stability and persistence of the targeting peptide is very meaningful for the further development of EV display technology (Lu et al., 2022). The cell membrane fusion technology is also getting more and more attention, which can fuse EV membranes with the cell membranes of many kinds of cells in biomedical applications.
Presently, the clinical management of AAA predominantly revolves around surgical intervention, specifically for individuals at risk of AAA rupture and patients with large AAA. However, no pharmacological alternatives exist for patients who are unable to undergo surgery, individuals with small AAA, and those experiencing ongoing AAA progression. Several studies have reported the advantages of drug-loaded engineered nanoparticles in the treatment of AAA, including the nanoparticles to increase the slow release and targeting of drugs, which can be localized in AAA at lower drug concentrations, showing good efficacy and safety.
However, challenges remain for further clinical translation. Firstly, some materials have high costs and long lead times to construct nanoparticles, and some show potential immunogenicity, or the constructed nanoparticles are so small that they can be quickly cleared in vivo. Secondly, when using antibody proteins to increase nanoparticle targeting, these antibodies are prone to denaturation during the construction process and may be degraded by a variety of enzymes in vivo, with loss of targeting. Finally, preclinical studies on nanoparticle drug delivery for AAA mainly focus on rodent models, which have shown exciting results. However, using only a single model cannot perfectly replace the pathogenic environment of AAA patients (e.g., pressure, flow rate, and composition of internal circulation), and further validation in multiple models is needed before entering clinical practice.
In the future, with the continuous innovation of nanotechnology, the maturation of gene editing technology, and the emergence of new natural nanomaterials such as EVs. Improving the targeting potential, circulation time, and controllable release of drugs by constructing engineered nanoparticles will become more diverse, which will provide new avenues for the next-generation of developing nano-loaded therapeutic AAA.
PD: Conceptualization, Writing–original draft. YH: Data curation, Writing–original draft. CS: Software, Writing–original draft. JG: Writing–original draft. YY: Software, Writing–original draft. JZ: Funding acquisition, Writing–review and editing. XC: Conceptualization, Writing–review and editing. JT: Supervision, Writing–review and editing.
The author(s) declare financial support was received for the research, authorship, and/or publication of this article. This work was supported by the National Natural Science Foundation of China (No. 82222007, 82170281, and U2004203), the Henan Thousand Talents Program (No. ZYQR201912131), Excellent Youth Science Foundation of Henan Province (No. 202300410362), Central Plains Youth Top Talent.
The authors declare that the research was conducted in the absence of any commercial or financial relationships that could be construed as a potential conflict of interest.
The author(s) declared that they were an editorial board member of Frontiers, at the time of submission. This had no impact on the peer review process and the final decision.
All claims expressed in this article are solely those of the authors and do not necessarily represent those of their affiliated organizations, or those of the publisher, the editors and the reviewers. Any product that may be evaluated in this article, or claim that may be made by its manufacturer, is not guaranteed or endorsed by the publisher.
Alan, D., and Cassis, L. A. (2004). Mouse models of abdominal aortic aneurysms. Arterioscler. Thromb. Vasc. Biol. 24, 429–434. doi:10.1161/01.ATV.0000118013.72016.ea
Alsiraj, Y., Thatcher, S. E., Charnigo, R., Chen, K., Blalock, E., Daugherty, A., et al. (2017). Female mice with an XY sex chromosome complement develop severe angiotensin II-induced abdominal aortic aneurysms. Circulation 135 (4), 379–391. doi:10.1161/circulationaha.116.023789
Anagnostakos, J., and Lal, B. K. (2021). Abdominal aortic aneurysms. Prog. Cardiovasc Dis. 65, 34–43. doi:10.1016/j.pcad.2021.03.009
Aoki, T., Kataoka, H., Ishibashi, R., Nakagami, H., Nozaki, K., Morishita, R., et al. (2009). PITAVASTATIN SUPPRESSES FORMATION AND PROGRESSION OF CEREBRAL ANEURYSMS THROUGH INHIBITION OF THE NUCLEAR FACTOR κB PATHWAY. Neurosurgery 64 (2), 357–366. doi:10.1227/01.neu.0000336764.92606.1d
AORN J (2020). Abdominal aortic aneurysm screening. AORN J. 111 (2), 260–262. doi:10.1002/aorn.12922
Bai, L., Ge, L., Zhang, B., Zhang, Y., Gu, J., Liu, L., et al. (2022). CtBP proteins transactivate matrix metalloproteinases and proinflammatory cytokines to mediate the pathogenesis of abdominal aortic aneurysm. Exp. Cell Res. 421, 113386. doi:10.1016/j.yexcr.2022.113386
Baman, J. R., and Eskandari, M. K. (2022). What is an abdominal aortic aneurysm? JAMA 328 (22), 2280. doi:10.1001/jama.2022.18638
Bartoli, M. A., Parodi, F. E., Chu, J., Pagano, M. B., Mao, D., Baxter, B. T., et al. (2006). Localized administration of doxycycline suppresses aortic dilatation in an experimental mouse model of abdominal aortic aneurysm. Aortic Aneurysm 20 (2), 228–236. doi:10.1007/s10016-006-9017-z
Benson, R. A., Meecham, L., Fisher, O., and Loftus, I. M. J. T. B. J. o. R. (2018). Ultrasound screening for abdominal aortic aneurysm: current practice, challenges and controversies. Challenges Controv. 91 (1090), 20170306. doi:10.1259/bjr.20170306
Bicknell, C. D., Kiru, G., Falaschetti, E., Powell, J. T., Poulter, N. R., and Collaborators, A. (2016). An evaluation of the effect of an angiotensin-converting enzyme inhibitor on the growth rate of small abdominal aortic aneurysms: a randomized placebo-controlled trial (AARDVARK). Eur. Heart J. 37 (42), 3213–3221. doi:10.1093/eurheartj/ehw257
Biros, E., Moran, C. S., Rush, C. M., Gäbel, G., Schreurs, C., Lindeman, J. H., et al. (2014). Differential gene expression in the proximal neck of human abdominal aortic aneurysm. Atherosclerosis 233 (1), 211–218. doi:10.1016/j.atherosclerosis.2013.12.017
Bukhari, S. N. A., Roswandi, N. L., Waqas, M., Habib, H., Hussain, F., Khan, S., et al. (2018). Hyaluronic acid, a promising skin rejuvenating biomedicine: a review of recent updates and pre-clinical and clinical investigations on cosmetic and nutricosmetic effects. Int. J. Biol. Macromol. 120 (Pt B), 1682–1695. doi:10.1016/j.ijbiomac.2018.09.188
Busch, A., Holm, A., Otto, C., Wagner, N., Erguen, S., Rosenfeld, M., et al. (2016). Extra- and intraluminal elastase induce morphologically distinct abdominal aortic aneurysms in mice and thus represent specific subtypes of human disease. J. Vasc. Res. 53, 49–57. doi:10.1159/000447263
Busch, A., Chernogubova, E., Jin, H., Meurer, F., Eckstein, H. H., Kim, M., et al. (2018). Four surgical modifications to the classic elastase perfusion aneurysm model enable haemodynamic alterations and extended elastase perfusion. Eur. J. Vasc. Endovasc. Surg. 56 (1), 102–109. doi:10.1016/j.ejvs.2018.03.018
Camardo, A., Seshadri, D., Broekelmann, T., Mecham, R., and Ramamurthi, A. (2018). Multifunctional, JNK-inhibiting nanotherapeutics for augmented elastic matrix regenerative repair in aortic aneurysms. Drug Deliv. Transl. Res. 8 (4), 964–984. doi:10.1007/s13346-017-0419-y
Chaikof, E. L., Dalman, R. L., Eskandari, M. K., Jackson, B. M., Lee, W. A., Mansour, M. A., et al. (2018). The Society for Vascular Surgery practice guidelines on the care of patients with an abdominal aortic aneurysm. J. Vasc. Surg. 67 (1), 2–77 e2. doi:10.1016/j.jvs.2017.10.044
Chan, W. K., Yong, E., Hong, Q., Mmed, L. Z., Lingam, P., Tan, G. W. L., et al. (2020). Systematic review and meta-analysis of the prevalence of abdominal aortic aneurysm in Asian populations. J J. Vasc. Surg. 73, 1069–1074.e1. doi:10.1016/j.jvs.2020.08.140
Chen, C., Ge, J., Gao, Y., Chen, L., Cui, J., Zeng, J., et al. (2022). Ultrasmall superparamagnetic iron oxide nanoparticles: a next generation contrast agent for magnetic resonance imaging. Wiley Interdiscip. Rev. Nanomed Nanobiotechnol 14 (1), e1740. doi:10.1002/wnan.1740
Cheng, J., Zhang, R., Li, C., Tao, H., Dou, Y., Wang, Y., et al. (2018). A targeting nanotherapy for abdominal aortic aneurysms. J. Am. Coll. Cardiol. 72 (21), 2591–2605. doi:10.1016/j.jacc.2018.08.2188
Chiou, A., Chiu, B., and Pearce, W. J. T. (2001). Murine aortic aneurysm produced by periarterial application of calcium chloride. J. Surg. Res. 99 (2), 371–376. doi:10.1006/jsre.2001.6207
Coscas, R., Dupont, S., Mussot, S., Louedec, L., Etienne, H., Morvan, M., et al. (2018). Exploring antibody-dependent adaptive immunity against aortic extracellular matrix components in experimental aortic aneurysms. J. Vasc. Surg. 68 (6s), 60S–71S.e3. doi:10.1016/j.jvs.2017.11.090
Dai, S., Wei, D., Wu, Z., Zhou, X., Wei, X., Huang, H., et al. (2008). Phase I clinical trial of autologous ascites-derived exosomes combined with GM-CSF for colorectal cancer. Mol. Ther. 16 (4), 782–790. doi:10.1038/mt.2008.1
Dang, G., Li, T., Yang, D., Yang, G., Du, X., Yang, J., et al. (2022). T lymphocyte-derived extracellular vesicles aggravate abdominal aortic aneurysm by promoting macrophage lipid peroxidation and migration via pyruvate kinase muscle isozyme 2. Redox Biol. 50, 102257. doi:10.1016/j.redox.2022.102257
Davies, M. J. (1998). Aortic aneurysm formation: lessons from human studies and experimental models. Circulation 98 (3), 193–195. doi:10.1161/01.cir.98.3.193
Debono, S., Nash, J., Tambyraja, A. L., Newby, D. E., and Forsythe, R. O. (2021). Endovascular repair for abdominal aortic aneurysms. Heart 107 (22), 1783–1789. doi:10.1136/heartjnl-2020-318288
Dhital, S., and Vyavahare, N. R. (2020). Nanoparticle-based targeted delivery of pentagalloyl glucose reverses elastase-induced abdominal aortic aneurysm and restores aorta to the healthy state in mice. PLoS One 15 (3), e0227165. doi:10.1371/journal.pone.0227165
Ding, R., McGuinness, C. L., Burnand, K. G., Sullivan, E., and Smith, A. (2005). Matrix metalloproteinases in the aneurysm wall of patients treated with low-dose doxycycline. Vascular 13 (5), 290–297. doi:10.1258/rsmvasc.13.5.290
Emeto, T. I., Moxon, J. V., Au, M. N., and Golledge, J. (2016). Oxidative stress and abdominal aortic aneurysm: potential treatment targets. Clin. Sci. 130 (5), 301–315. doi:10.1042/cs20150547
Fashandi, A. Z., Hawkins, R. B., Salmon, M. D., Spinosa, M. D., Montgomery, W. G., Cullen, J. M., et al. (2018). A novel reproducible model of aortic aneurysm rupture. Surgery 163 (2), 397–403. doi:10.1016/j.surg.2017.10.003
Fraser, J. R. E., Laurent, T. C., Engstromlaurent, A., and Laurent, U. G. B. (1984). ELIMINATION OF HYALURONIC-ACID FROM THE BLOOD STREAM IN THE HUMAN. Clin. Exp. Pharmacol. Physiology 11 (1), 17–25. doi:10.1111/j.1440-1681.1984.tb00235.x
Fugate, J. E., and Rabinstein, A. A. (2014). Update on intravenous recombinant tissue plasminogen activator for acute ischemic stroke. Mayo Clin. Proc. 89 (7), 960–972. doi:10.1016/j.mayocp.2014.03.001
Fukuhara, N., Honda, Y., Ukita, N., Matsui, M., Miura, Y., and Hoshina, K. (2020). Efficient suppression of abdominal aortic aneurysm expansion in rats through systemic administration of statin-loaded nanomedicine. Int. J. Mol. Sci. 21 (22), 8702. doi:10.3390/ijms21228702
Gacchina, C., Brothers, T., and Ramamurthi, A. (2011). Evaluating smooth muscle cells from CaCl2-induced rat aortal expansions as a surrogate culture model for study of elastogenic induction of human aneurysmal cells. Tissue Eng. Part A 17 (15-16), 1945–1958. doi:10.1089/ten.tea.2010.0475
Ghadami, M., Makita, Y., Yoshida, K., Nishimura, G., Fukushima, Y., Wakui, K., et al. (2000). Genetic mapping of the camurati-engelmann disease locus to chromosome 19q13.1-q13.3. Am. J. Hum. Genet. 66 (1), 143–147. doi:10.1086/302728
Golledge, J., Norman, P. E., Murphy, M. P., and Dalman, R. L. (2017). Challenges and opportunities in limiting abdominal aortic aneurysm growth. J. Vasc. Surg. 65 (1), 225–233. doi:10.1016/j.jvs.2016.08.003
Golledge, J., Thanigaimani, S., Powell, J. T., and Tsao, P. S. (2023). Pathogenesis and management of abdominal aortic aneurysm. Eur. Heart J. 44 (29), 2682–2697. doi:10.1093/eurheartj/ehad386
Golledge, J. (2019). Abdominal aortic aneurysm: update on pathogenesis and medical treatments. Nat. Rev. Cardiol. 16 (4), 225–242. doi:10.1038/s41569-018-0114-9
Han, Z. L., Wang, H. Q., Zhang, T. S., He, Y. X., and Zhou, H. (2020). Up-regulation of exosomal miR-106a may play a significant role in abdominal aortic aneurysm by inducing vascular smooth muscle cell apoptosis and targeting TIMP-2, an inhibitor of metallopeptidases that suppresses extracellular matrix degradation. Eur. Rev. Med. Pharmacol. Sci. 24 (15), 8087–8095. doi:10.26355/eurrev_202008_22493
Hoshyar, N., Gray, S., Han, H. B., and Bao, G. (2016). The effect of nanoparticle size on in vivo pharmacokinetics and cellular interaction. Nanomedicine 11 (6), 673–692. doi:10.2217/nnm.16.5
Howell, C. M., and Rabener, M. J. (2016). Abdominal aortic aneurysm: a ticking time bomb. JAAPA 29 (3), 32–36. doi:10.1097/01.jaa.0000480855.07045.50
Isnard, N., Legeais, J. M., Renard, G., and Robert, L. (2001). Effect of hyaluronan on MMP expression and activation. Cell Biol. Int. 25 (8), 735–739. doi:10.1006/cbir.2001.0759
Itoga, N. K., Rothenberg, K. A., Suarez, P., Surgery, T., Mell, M. W., Xu, B., et al. (2018). Metformin prescription status and abdominal aortic aneurysm disease progression in the U.S. veteran population. J. Vasc. Surg. 69, 710–716. doi:10.1016/j.jvs.2018.06.194
Jiang, W., Rutherford, D., Vuong, T., and Liu, H. (2017). Nanomaterials for treating cardiovascular diseases: a review. Bioact. Mater 2 (4), 185–198. doi:10.1016/j.bioactmat.2017.11.002
Juvonen, J. J., Tuvonen, T., Laurila, A., Alakarppa, H., Lounatmaa, K., Surcel, H. M., et al. (1997). Demonstration of Chlamydia pneumoniae in the walls of abdominal aortic aneurysms. J. Vasc. Surg. 25 (3), 499–505. doi:10.1016/s0741-5214(97)70260-x
Katsuki, S., Koga, J. I., Matoba, T., Umezu, R., Nakashiro, S., Nakano, K., et al. (2022). Nanoparticle-mediated delivery of pitavastatin to monocytes/macrophages inhibits angiotensin II-induced abdominal aortic aneurysm formation in apoe(-/-) mice. J. Atheroscler. Thromb. 29 (1), 111–125. doi:10.5551/jat.54379
Kazi, M., Thyberg, J., Religa, P., Roy, J., Eriksson, P., Hedin, U., et al. (2003). Influence of intraluminal thrombus on structural and cellular composition of abdominal aortic aneurysm wall. J. Vasc. Surg. 38 (6), 1283–1292. doi:10.1016/s0741-5214(03)00791-2
Klink, A., Hyafil, F., Rudd, J., Faries, P., Fuster, V., Mallat, Z., et al. (2011). Diagnostic and therapeutic strategies for small abdominal aortic aneurysms. Nat. Rev. Cardiol. 8 (6), 338–347. doi:10.1038/nrcardio.2011.1
Koole, D., Hurks, R., Schoneveld, A., Vink, A., Golledge, J., Moran, C. S., et al. (2012). Osteoprotegerin is associated with aneurysm diameter and proteolysis in abdominal aortic aneurysm disease. Arterioscler. Thromb. Vasc. Biol. 32 (6), 1497–1504. doi:10.1161/atvbaha.111.243592
Kothapalli, C. R., Taylor, P. M., Smolenski, R. T., Yacoub, M. H., and Ramamurthi, A. (2009). Transforming growth factor beta 1 and hyaluronan oligomers synergistically enhance elastin matrix regeneration by vascular smooth muscle cells. Tissue Eng. Part A 15 (3), 501–511. doi:10.1089/ten.tea.2008.0040
Lareyre, F., Clément, M., Raffort, J., Pohlod, S., Patel, M., Esposito, B., et al. (2017). TGFβ (transforming growth factor-β) blockade induces a human-like disease in a nondissecting mouse model of abdominal aortic aneurysm. Arterioscler. Thromb. Vasc. Biol. 37 (11), 2171–2181. doi:10.1161/atvbaha.117.309999
Lareyre, F., Adam, C., Carrier, M., and Raffort, J. (2021). Artificial intelligence and automatic segmentation of abdominal aortic aneurysm: past, present, and future. J. Vasc. Surg. 74 (1), 347–348. doi:10.1016/j.jvs.2021.01.073
Lederle, F. A. (2013). Abdominal aortic aneurysm: still No pill. Ann. Intern. Med. 159 (12), 852–853. doi:10.7326/0003-4819-159-12-201312170-00012
Li, Z., Cong, X., and Kong, W. (2022). Matricellular proteins: potential biomarkers and mechanistic factors in aortic aneurysms. J. Mol. Cell Cardiol. 169, 41–56. doi:10.1016/j.yjmcc.2022.05.001
Lindeman, J. H. N., Rabelink, T. J., and van Bockel, J. H. (2011). Immunosuppression and the abdominal aortic aneurysm. Circulation 124 (18), e463–e465. doi:10.1161/circulationaha.110.008573
Liu, J., Xiong, W., Baca-Regen, L., Nagase, H., and Baxter, B. T. (2003). Mechanism of inhibition of matrix metalloproteinase-2 expression by doxycycline in human aortic smooth muscle cells. J. Vasc. Surg. 38 (6), 1376–1383. doi:10.1016/s0741-5214(03)01022-x
Lu, S., Wang, R., Fu, W., and Si, Y. (2022). Applications of extracellular vesicles in abdominal aortic aneurysm. Front. Cardiovasc Med. 9, 927542. doi:10.3389/fcvm.2022.927542
Luk, B. T., and Zhang, L. F. (2015). Cell membrane-camouflaged nanoparticles for drug delivery. J. Control. Release 220, 600–607. doi:10.1016/j.jconrel.2015.07.019
Meijer, C. A., Stijnen, T., Wasser, M., Hamming, J. F., van Bockel, H., and Lindeman, J. H. N. (2013). Doxycycline for stabilization of abdominal aortic aneurysms: a randomized trial. Ann. Intern. Med. 159 (12), 815–+. doi:10.7326/0003-4819-159-12-201312170-00007
Moxon, J. V., Parr, A., Emeto, T. I., Walker, P., Norman, P. E., and Golledge, J. (2010). Diagnosis and monitoring of abdominal aortic aneurysm: current status and future prospects. Curr. Probl. Cardiol. 35 (10), 512–548. doi:10.1016/j.cpcardiol.2010.08.004
Nordon, I. M., Hinchliffe, R. J., Loftus, I. M., and Thompson, M. M. (2011). Pathophysiology and epidemiology of abdominal aortic aneurysms. Nat. Rev. Cardiol. 8 (2), 92–102. doi:10.1038/nrcardio.2010.180
Nosoudi, N., Nahar-Gohad, P., Sinha, A., Chowdhury, A., Gerard, P., Carsten, C. G., et al. (2015). Prevention of abdominal aortic aneurysm progression by targeted inhibition of matrix metalloproteinase activity with batimastat-loaded nanoparticles. Circ. Res. 117 (11), e80–e89. doi:10.1161/circresaha.115.307207
Nosoudi, N., Chowdhury, A., Siclari, S., Parasaram, V., Karamched, S., and Vyavahare, N. (2016a). Systemic delivery of nanoparticles loaded with pentagalloyl glucose protects elastic lamina and prevents abdominal aortic aneurysm in rats. J. Cardiovasc Transl. Res. 9 (5-6), 445–455. doi:10.1007/s12265-016-9709-x
Nosoudi, N., Chowdhury, A., Siclari, S., Karamched, S., Parasaram, V., Parrish, J., et al. (2016b). Reversal of vascular calcification and aneurysms in a rat model using dual targeted therapy with EDTA- and PGG-loaded nanoparticles. Theranostics 6 (11), 1975–1987. doi:10.7150/thno.16547
Oliver-Williams, C., Sweeting, M. J., Turton, G., Parkin, D., Cooper, D., Rodd, C., et al. (2018). Lessons learned about prevalence and growth rates of abdominal aortic aneurysms from a 25-year ultrasound population screening programme. Br. J. Surg. 105 (1), 68–74. doi:10.1002/bjs.10715
Patel, R., Sweeting, M. J., Powell, J. T., and Greenhalgh, R. M. (2016). Endovascular versus open repair of abdominal aortic aneurysm in 15-years’ follow-up of the UK endovascular aneurysm repair trial 1 (EVAR trial 1): a randomised controlled trial. Lancet 388 (10058), 2366–2374. doi:10.1016/s0140-6736(16)31135-7
Powell, J. T., Brown, L. C., Forbes, J. F., Fowkes, F. G., Greenhalgh, R. M., Ruckley, C. V., et al. (2007). Final 12-year follow-up of surgery versus surveillance in the UK small aneurysm trial. Br. J. Surg. 94 (6), 702–708. doi:10.1002/bjs.5778
Propanolol Aneurysm Trial, I. (2002). Propranolol for small abdominal aortic aneurysms: results of a randomized trial. J. Vasc. Surg. 35 (1), 72–79. doi:10.1067/mva.2002.121308
Qrareya, M., and Zuhaili, B. (2021). Management of postoperative complications following endovascular aortic aneurysm repair. Surg. Clin. North Am. 101 (5), 785–798. doi:10.1016/j.suc.2021.05.020
Quaye, K. B., Pack, N., Wilson-Byrne, T., and Long, C. A. (2022). Contemporary management of abdominal aortic aneurysms. Curr. Cardiol. Rep. 24 (4), 431–438. doi:10.1007/s11886-022-01662-z
Quintana, R. A., and Taylor, W. R. (2019). Cellular mechanisms of aortic aneurysm formation. Circulation Res. 124 (4), 607–618. doi:10.1161/circresaha.118.313187
Rabkin, S. W. (2017). The role matrix metalloproteinases in the production of aortic aneurysm. Prog. Mol. Biol. Transl. Sci. 147, 239–265. doi:10.1016/bs.pmbts.2017.02.002
Raffort, J., Lareyre, F., Clement, M., Hassen-Khodja, R., Chinetti, G., and Mallat, Z. (2017). Monocytes and macrophages in abdominal aortic aneurysm. Nat. Rev. Cardiol. 14 (8), 457–471. doi:10.1038/nrcardio.2017.52
Rateri, D. L., Howatt, D. A., Moorleghen, J. J., Charnigo, R., Cassis, L. A., and Daugherty, A. (2011). Prolonged infusion of angiotensin II in apoE(-/-) mice promotes macrophage recruitment with continued expansion of abdominal aortic aneurysm. Am. J. Pathology 179 (3), 1542–1548. doi:10.1016/j.ajpath.2011.05.049
Rd, C. C., Calton, W. C., Johanning, J. M., Armstrong, P. J., Franklin, D. P., Carey, D. J., et al. (2001). Elastase is not sufficient to induce experimental abdominal aortic aneurysms. J. Vasc. Surg. 33, 33. doi:10.1067/mva.2001.112706
Rouer, M., Xu, B. H., Xuan, H. J., Tanaka, H., Fujimura, N., Glover, K. J., et al. (2014). Rapamycin limits the growth of established experimental abdominal aortic aneurysms. Eur. J. Vasc. Endovasc. Surg. 47 (5), 493–500. doi:10.1016/j.ejvs.2014.02.006
Sajeesh, S., Broekelman, T., Mecham, R. P., and Ramamurthi, A. (2020). Stem cell derived extracellular vesicles for vascular elastic matrix regenerative repair. Acta Biomater. 113, 267–278. doi:10.1016/j.actbio.2020.07.002
Sakai, L. Y., Keene, D. R., Engvall, E., and Fibrillin, A. (1986). Fibrillin, a new 350-kD glycoprotein, is a component of extracellular microfibrils. J. Cell Biol. 103 (6), 2499–2509. doi:10.1083/jcb.103.6.2499
Sakalihasan, N., Michel, J.-B., Katsargyris, A., Kuivaniemi, H., Defraigne, J.-O., Nchimi, A., et al. (2018). Abdominal aortic aneurysms. Nat. Rev. Dis. Prim. 4 (1), 34. doi:10.1038/s41572-018-0030-7
Salata, K., Syed, M., Hussain, M. A., de Mestral, C., Greco, E., Mamdani, M., et al. (2018). Statins reduce abdominal aortic aneurysm growth, rupture, and perioperative mortality: a systematic review and meta-analysis. J. Am. Heart Assoc. 7 (19), e008657. doi:10.1161/jaha.118.008657
Salem, M. K., Rayt, H. S., Hussey, G., Rafelt, S., Nelson, C. P., Sayers, R. D., et al. (2009). Should Asian men be included in abdominal aortic aneurysm screening programmes? Eur. J. Vasc. Endovasc. Surg. 38 (6), 748–749. doi:10.1016/j.ejvs.2009.07.012
Sanchez-Infantes, D., Nus, M., Navas-Madronal, M., Fite, J., Perez, B., Barros-Membrilla, A. J., et al. (2021). Oxidative stress and inflammatory markers in abdominal aortic aneurysm. Antioxidants (Basel) 10 (4), 602. doi:10.3390/antiox10040602
Schanzer, A., and Oderich, G. S. (2021). Management of abdominal aortic aneurysms. N. Engl. J. Med. 385 (18), 1690–1698. doi:10.1056/nejmcp2108504
Schmitz-Rixen, T., Keese, M., Hakimi, M., Peters, A., Bockler, D., Nelson, K., et al. (2016). Ruptured abdominal aortic aneurysm-epidemiology, predisposing factors, and biology. Langenbecks Arch. Surg. 401 (3), 275–288. doi:10.1007/s00423-016-1401-8
Senemaud, J., Caligiuri, G., Etienne, H., Delbosc, S., Michel, J. B., and Coscas, R. (2017). Translational relevance and recent advances of animal models of abdominal aortic aneurysm. Arterioscler. Thromb. Vasc. Biol. 37 (3), 401–410. doi:10.1161/atvbaha.116.308534
Shirasu, T., Koyama, H., Miura, Y., Hoshina, K., Kataoka, K., and Watanabe, T. (2016). Nanoparticles effectively target rapamycin delivery to sites of experimental aortic aneurysm in rats. PLoS One 11 (6), e0157813. doi:10.1371/journal.pone.0157813
Sillesen, H., Eldrup, N., Hultgren, R., Lindeman, J., Bredahl, K., Thompson, M., et al. (2015). Randomized clinical trial of mast cell inhibition in patients with a medium-sized abdominal aortic aneurysm. Br. J. Surg. 102 (8), 1295–1901. doi:10.1002/bjs.9917
Sinha, A., Shaporev, A., Nosoudi, N., Lei, Y., Vertegel, A., Lessner, S., et al. (2014a). Nanoparticle targeting to diseased vasculature for imaging and therapy. Nanomedicine 10 (5), 1003–1012. doi:10.1016/j.nano.2014.02.002
Sinha, A., Nosoudi, N., and Vyavahare, N. (2014b). Elasto-regenerative properties of polyphenols. Biochem. Biophys. Res. Commun. 444 (2), 205–211. doi:10.1016/j.bbrc.2014.01.027
Sivaraman, B., and Ramamurthi, A. (2013). Multifunctional nanoparticles for doxycycline delivery towards localized elastic matrix stabilization and regenerative repair. Acta Biomater. 9 (5), 6511–6525. doi:10.1016/j.actbio.2013.01.023
Sivaraman, B., Sylvester, A., and Ramamurthi, A. (2016). Fibrinolytic PLGA nanoparticles for slow clot lysis within abdominal aortic aneurysms attenuate proteolytic loss of vascular elastic matrix. Mater Sci. Eng. C Mater Biol. Appl. 59, 145–156. doi:10.1016/j.msec.2015.09.056
Sivaraman, B., Swaminathan, G., Moore, L., Fox, J., Seshadri, D., Dahal, S., et al. (2017). Magnetically-responsive, multifunctional drug delivery nanoparticles for elastic matrix regenerative repair. Acta Biomater. 52, 171–186. doi:10.1016/j.actbio.2016.11.048
Spinosa, M., Lu, G. Y., Su, G., Bontha, S. V., Gehrau, R., Salmon, M. D., et al. (2018). Human mesenchymal stromal cell-derived extracellular vesicles attenuate aortic aneurysm formation and macrophage activation via microRNA-147. Faseb J. 32 (11), 6038–6050. doi:10.1096/fj.201701138rr
Sprynger, M., Willems, M., Van Damme, H., Drieghe, B., Wautrecht, J. C., and Moonen, M. (2019). Screening Program of abdominal aortic aneurysm. Angiology 70 (5), 407–413. doi:10.1177/0003319718824940
Steinmetz, E. F., Buckley, C., Shames, M. L., Ennis, T. L., Vanvickle-Chavez, S. J., Mao, D. L., et al. (2005). Treatment with simvastatin suppresses the development of experimental abdominal aortic aneurysms in normal and hypercholesterolemic mice. Ann. Surg. 241 (1), 92–101. doi:10.1097/01.sla.0000150258.36236.e0
Summers, K. L. K., Sheahan, E. K., Sheahan, C. M., and Malachi, G. (2021). Evaluating the prevalence of abdominal aortic aneurysms in the United States through a national screening database. J J. Vasc. Surg. 73 (1), 61–68. doi:10.1016/j.jvs.2020.03.046
Sun, N., Leung, J. H., Wood, N. B., Hughes, A. D., Thom, S. A., Cheshire, N. J., et al. (2009). Computational analysis of oxygen transport in a patient-specific model of abdominal aortic aneurysm with intraluminal thrombus. Br. J. Radiol. 82, S18–S23. doi:10.1259/bjr/89466318
Suri, S. S., Fenniri, H., and Singh, B. (2007). Nanotechnology-based drug delivery systems. J. Occup. Med. Toxicol. 2, 16. doi:10.1186/1745-6673-2-16
Sweeting, M. J., Thompson, S. G., Brown, L. C., Powell, J. T., and collaborators, R. (2012). Meta-analysis of individual patient data to examine factors affecting growth and rupture of small abdominal aortic aneurysms. Br. J. Surg. 99 (5), 655–665. doi:10.1002/bjs.8707
Sweeting, M. J., Patel, R., Powell, J. T., and Greenhalgh, R. M. (2017). Endovascular repair of abdominal aortic aneurysm in patients physically ineligible for open repair: very long-term follow-up in the EVAR-2 randomized controlled trial. Ann. Surg. 266, 713–719. doi:10.1097/SLA.0000000000002392
Sylvester, A., Sivaraman, B., Deb, P., and Ramamurthi, A. (2013). Nanoparticles for localized delivery of hyaluronan oligomers towards regenerative repair of elastic matrix. Acta Biomater. 9 (12), 9292–9302. doi:10.1016/j.actbio.2013.07.032
Takagi, H., Yamamoto, H., Iwata, K., Goto, S., Umemoto, T., and Group, A. (2012). Effects of statin therapy on abdominal aortic aneurysm growth: a meta-analysis and meta-regression of observational comparative studies. Eur. J. Vasc. Endovasc. Surg. 44 (3), 287–292. doi:10.1016/j.ejvs.2012.06.021
Tian, Z., Zhang, Y., Zheng, Z., Zhang, M., Zhang, T., Jin, J., et al. (2022). Gut microbiome dysbiosis contributes to abdominal aortic aneurysm by promoting neutrophil extracellular trap formation. Cell Host Microbe 30 (10), 1450–1463 e8. doi:10.1016/j.chom.2022.09.004
Toczek, J., Meadows, J. L., and Sadeghi, M. M. (2016). Novel molecular imaging approaches to abdominal aortic aneurysm risk stratification. Circ. Cardiovasc Imaging 9 (1), e003023. doi:10.1161/circimaging.115.003023
Trachet, B., Piersigilli, A., Fraga-Silva, R., Aslanidou, L., Sordet-Dessimoz, J., Astolfo, A., et al. (2016). Ascending aortic aneurysm in angiotensin II-infused mice: formation, progression, and the role of focal dissections. Arterioscler. Thromb. Vasc. Biol. 36 (4), 673–681. doi:10.1161/atvbaha.116.307211
Tsilimparis, N., Heidemann, F., Rohlffs, F., Diener, H., Wipper, S., Debus, E. S., et al. (2017). Outcome of surgeon-modified fenestrated/branched stent-grafts for symptomatic complex aortic pathologies or contained rupture. J. Endovasc. Ther. 24 (6), 825–832. doi:10.1177/1526602817729673
Ullery, B. W., Hallett, R. L., and Fleischmann, D. (2018). Epidemiology and contemporary management of abdominal aortic aneurysms. Abdom. Radiol. (NY) 43 (5), 1032–1043. doi:10.1007/s00261-017-1450-7
Venkataraman, L., Sivaraman, B., Vaidya, P., and Ramamurthi, A. (2016). Nanoparticulate delivery of agents for induced elastogenesis in three-dimensional collagenous matrices. J. Tissue Eng. Regen. Med. 10 (12), 1041–1056. doi:10.1002/term.1889
Virani, S. S., Alonso, A., Benjamin, E. J., Bittencourt, M. S., Callaway, C. W., Carson, A. P., et al. (2020). Heart disease and stroke statistics—2020 update: a report from the American heart association. Circulation 141 (9), e139–e596. doi:10.1161/cir.0000000000000757
Walker, S., Busatto, S., Pham, A., Tian, M., Suh, A., Carson, K., et al. (2019). Extracellular vesicle-based drug delivery systems for cancer treatment. Theranostics 9 (26), 8001–8017. doi:10.7150/thno.37097
Wan, Z., Zhao, L. B., Lu, F., Gao, X. T., Dong, Y., Zhao, Y. X., et al. (2020). Mononuclear phagocyte system blockade improves therapeutic exosome delivery to the myocardium. Theranostics 10 (1), 218–230. doi:10.7150/thno.38198
Wang, Y., Krishna, S., Moxon, J., Dinh, T., Jose, R., Yu, H., et al. (2014). Influence of apolipoprotein E, age and aortic site on calcium phosphate induced abdominal aortic aneurysm in mice. Atherosclerosis 235 (1), 204–212. doi:10.1016/j.atherosclerosis.2014.04.033
Wang, Y. D., Liu, Z. J., Ren, J., and Xiang, M. X. (2018). Pharmacological therapy of abdominal aortic aneurysm: an update. Curr. Vasc. Pharmacol. 16 (2), 114–124. doi:10.2174/1570161115666170413145705
Weaver, L. M., Loftin, C. D., and Zhan, C. G. (2022). Development of pharmacotherapies for abdominal aortic aneurysms. Biomed. Pharmacother. 153, 113340. doi:10.1016/j.biopha.2022.113340
Wiklander, O. P. B., Nordin, J. Z., O’Loughlin, A., Gustafsson, Y., Corso, G., Mager, I., et al. (2015). Extracellular vesicle in vivo biodistribution is determined by cell source, route of administration and targeting. J. Extracell. vesicles 4, 26316. doi:10.3402/jev.v4.26316
Williamson, A. J., and Babrowski, T. (2018). Current endovascular management of complex pararenal aneurysms. J. Cardiovasc Surg. (Torino) 59 (3), 336–341. doi:10.23736/s0021-9509.18.10408-3
Witwer, K. W., and Wolfram, J. (2021). Extracellular vesicles versus synthetic nanoparticles for drug delivery. Nat. Rev. Mater 6 (2), 103–106. doi:10.1038/s41578-020-00277-6
Yamawaki-Ogata, A., Hashizume, R., Satake, M., Kaneko, H., Mizutani, S., Moritan, T., et al. (2010). A doxycycline loaded, controlled-release, biodegradable fiber for the treatment of aortic aneurysms. Biodegrad. fiber Treat. aortic aneurysms 31 (36), 9554–9564. doi:10.1016/j.biomaterials.2010.08.069
Yin, L., Zhang, K. J., Sun, Y. T., and Liu, Z. J. (2021). Nanoparticle-assisted diagnosis and treatment for abdominal aortic aneurysm. Front. Med. 8, 665846. doi:10.3389/fmed.2021.665846
Yoshimura, K., Morikage, N., Nishino-Fujimoto, S., Furutani, A., Shirasawa, B., and Hamano, K. (2018). Current status and perspectives on pharmacologic therapy for abdominal aortic aneurysm. Curr. Drug Targets 19 (11), 1265–1275. doi:10.2174/1389450119666171227223331
Yoshimura, K., Aoki, H., Teruyama, C., Iijima, M., Tsutsumi, H., Kuroda, S., et al. (2020). A novel hybrid drug delivery system for treatment of aortic aneurysms. Int. J. Mol. Sci. 21 (15), 5538. doi:10.3390/ijms21155538
Yu, B., Zhang, X. M., and Li, X. R. (2014). Exosomes derived from mesenchymal stem cells. Int. J. Mol. Sci. 15 (3), 4142–4157. doi:10.3390/ijms15034142
Yu, M., Chen, C., Cao, Y., and Qi, R. (2017). Inhibitory effects of doxycycline on the onset and progression of abdominal aortic aneurysm and its related mechanisms. Eur. J. Pharmacol. 811, 101–109. doi:10.1016/j.ejphar.2017.05.041
Zhang, S. L., Du, X., Chen, Y. Q., Tan, Y. S., and Liu, L. (2018). Potential medication treatment according to pathological mechanisms in abdominal aortic aneurysm. J. Cardiovasc Pharmacol. 71 (1), 46–57. doi:10.1097/fjc.0000000000000540
Zhao, G. Z., Lu, H. C., Chang, Z. Y., Zhao, Y., Zhu, T. Q., Chang, L., et al. (2021). Single-cell RNA sequencing reveals the cellular heterogeneity of aneurysmal infrarenal abdominal aorta. Cardiovasc. Res. 117 (5), 1402–1416. doi:10.1093/cvr/cvaa214
Zhou, Y., Wu, W., Lindholt, J. S., Sukhova, G. K., Libby, P., Yu, X., et al. (2015). Regulatory T cells in human and angiotensin II-induced mouse abdominal aortic aneurysms. Cardiovasc Res. 107 (1), 98–107. doi:10.1093/cvr/cvv119
Zhu, S., Zhu, K., Li, J., Lai, H., and Wang, C. (2020). Nano-biomaterials for the delivery of therapeutic and monitoring cues for aortic diseases. Front. Bioeng. Biotechnol. 8, 583879. doi:10.3389/fbioe.2020.583879
Keywords: abdominal aortic aneurysm, engineered nanoparticles, screening, extracellular vesicles, treatment
Citation: Du P, Hou Y, Su C, Gao J, Yang Y, Zhang J, Cui X and Tang J (2024) The future for the therapeutics of abdominal aortic aneurysm: engineered nanoparticles drug delivery for abdominal aortic aneurysm. Front. Bioeng. Biotechnol. 11:1324406. doi: 10.3389/fbioe.2023.1324406
Received: 19 October 2023; Accepted: 12 December 2023;
Published: 05 January 2024.
Edited by:
Jun Liao, University of Texas at Arlington, United StatesReviewed by:
Ling Tang, Mayo Clinic Arizona, United StatesCopyright © 2024 Du, Hou, Su, Gao, Yang, Zhang, Cui and Tang. This is an open-access article distributed under the terms of the Creative Commons Attribution License (CC BY). The use, distribution or reproduction in other forums is permitted, provided the original author(s) and the copyright owner(s) are credited and that the original publication in this journal is cited, in accordance with accepted academic practice. No use, distribution or reproduction is permitted which does not comply with these terms.
*Correspondence: Junnan Tang, ZmNjdGFuZ2puQHp6dS5lZHUuY24=; Xiaolin Cui, c3RldmVuY3VpQGN1aGsuZWR1LmNu; Jinying Zhang, anl6aGFuZ0B6enUuZWR1LmNu
Disclaimer: All claims expressed in this article are solely those of the authors and do not necessarily represent those of their affiliated organizations, or those of the publisher, the editors and the reviewers. Any product that may be evaluated in this article or claim that may be made by its manufacturer is not guaranteed or endorsed by the publisher.
Research integrity at Frontiers
Learn more about the work of our research integrity team to safeguard the quality of each article we publish.