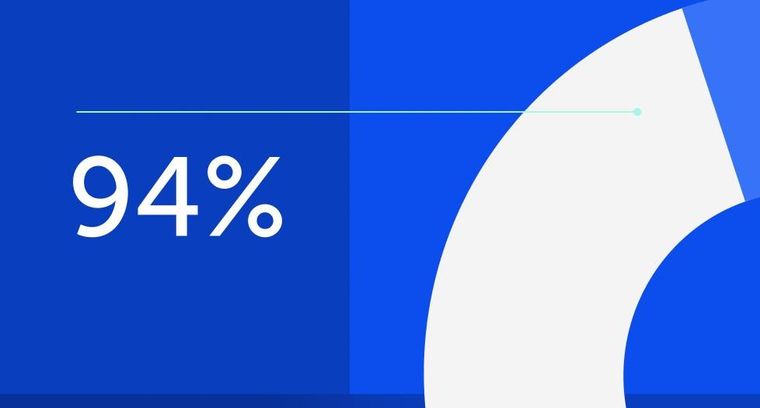
94% of researchers rate our articles as excellent or good
Learn more about the work of our research integrity team to safeguard the quality of each article we publish.
Find out more
MINI REVIEW article
Front. Bioeng. Biotechnol., 07 December 2023
Sec. Tissue Engineering and Regenerative Medicine
Volume 11 - 2023 | https://doi.org/10.3389/fbioe.2023.1308761
Peripheral nerve injury (PNI) is a neurological disorder caused by trauma that is frequently induced by accidents, war, and surgical complications, which is of global significance. The severity of the injury determines the potential for lifelong disability in patients. Artificial nerve scaffolds have been investigated as a powerful tool for promoting optimal regeneration of nerve defects. Over the past few decades, bionic scaffolds have been successfully developed to provide guidance and biological cues to facilitate Schwann cell myelination and orientated axonal growth. Numerous assessment techniques have been employed to investigate the therapeutic efficacy of nerve scaffolds in promoting the growth of Schwann cells and axons upon the bioactivities of distinct scaffolds, which have encouraged a greater understanding of the biological mechanisms involved in peripheral nerve development and regeneration. However, it is still difficult to compare the results from different labs due to the diversity of protocols and the availability of innovative technologies when evaluating the effectiveness of novel artificial scaffolds. Meanwhile, due to the complicated process of peripheral nerve regeneration, several evaluation methods are usually combined in studies on peripheral nerve repair. Herein, we have provided an overview of the evaluation methods used to study the outcomes of scaffold-based therapies for PNI in experimental animal models and especially focus on Schwann cell functions and axonal growth within the regenerated nerve.
Peripheral nerve injury (PNI) is a traumatic nervous disease in clinical settings with significant severity worldwide, which is commonly caused by accidents, war, and surgical complications (Houshyar et al., 2019; Meena et al., 2021; Chen et al., 2022; Hercher et al., 2022; Hui et al., 2022). It has been reported that peripheral nerve injuries affect approximately 2.8% of trauma patients annually, causing a serious health problem (Taylor et al., 2008; Vijayavenkataraman, 2020; Acheta et al., 2021). PNI can lead to lifelong disability in patients with neuropathic pain, depending on the severity of the injury (Yi et al., 2019; Davoli-Ferreira et al., 2020; Idrisova et al., 2022; Sharifi et al., 2023; Xiong et al., 2023). As the regeneration rate of damaged peripheral nerves is approximately 1 mm per day, the strategies and outcomes of clinical treatment on PNI usually depend on the different types of injury and the distances of the gaps (Seddon et al., 1943; Sunderland, 1947; Juckett et al., 2022; Zhang et al., 2023a; Choe et al., 2023). Moreover, patients may need multiple surgeries and extended hospitalizations, which places a financial burden on the healthcare system (Padovano et al., 2022; Dong et al., 2023).
Nowadays, the standard clinical intervention for bridging millimeter-scaled nerve gaps often relies on a tension-free end-to-end suture (Lopez-Cebral et al., 2017; Kou et al., 2019; Leite et al., 2019). However, for longer-gapped PNI, the distal nerve and end tissue may undergo atrophy over time, which can cause permanent sensory and motor dysfunction (Tang et al., 2021). The gold standard for treating long-gapped PNI is to bridge the nerve defect using an autologous nerve graft in the clinic (Heath and Rutkowski, 1998). Nevertheless, secondary injury, mismatch on the size of donor and recipient nerve tissues, and peripheral neurofibroma formation usually limit the therapeutic efficiency and outcome of autologous nerve grafts, which can result in poor sensory and functional recovery (Bombeiro et al., 2016; He et al., 2020; Manto et al., 2021; Tang et al., 2022). Thus, artificial nerve scaffolds have been explored to facilitate the desirable regeneration of nerves after injuries. In recent decades, artificial scaffolds, with effective guidance and biological cues to facilitate Schwann cell (SC) myelination and axonal growth, have emerged along with a deeper understanding of biological mechanisms in peripheral nerve development and regeneration (Jiang et al., 2022a; Zhang et al., 2022a; Huang et al., 2022; Smith et al., 2022; Thibodeau et al., 2022; Zhang et al., 2023b). However, when evaluating the effectiveness of novel artificial scaffolds, it is difficult to compare the results from different labs due to the diversity of protocols and the availability of innovative technologies. Therefore, in this review, we have explored the useful methods applied in evaluating the outcomes of scaffold-based therapies for PNI in experimental animal models and especially focus on Schwann cell functions and axonal growth within the regenerated nerve.
Schwann cells are the most well-known and abundant cell type of peripheral glial cells, which have been well-studied in peripheral nerve repair (Jessen and Arthur-Farraj, 2019; Wang et al., 2019; Wang et al., 2023a). With deeper understanding about the biological functions of Schwann cells in nerve repair, distinct populations of SCs have been revealed. Generally, SCs are divided into myelinating and non-myelinating cell types. Myelinating SCs ensheath axons to facilitate the conduction of electric impulses during nervous activity, while non-myelinating SCs, such as Remak SCs, surround axons of small caliber and provide trophic support to unmyelinated axons (Brifault et al., 2020; Bosch-Queralt et al., 2023; Procacci et al., 2023). In addition to forming myelin sheaths around axons and secreting neurotrophic factors, SCs can also regulate sensory perception and facilitate cell communications between synapses (Faroni et al., 2014). Moreover, it has been found that SCs also play a significant role in neuroinflammation and neuropathic pain (Wang et al., 2022; Adam et al., 2023; Chen et al., 2023).
All types of SCs can promote peripheral nerve regeneration through their cell differentiation and functional alteration after PNI. Soon after injury, demyelination of injured peripheral nerves occurs and axons break into debris in the distal stump via Wallerian degeneration (Zou et al., 2022). Then, SCs are activated in the repair of PNI. Initially, SCs segment the debris of injured peripheral nerves and induce macrophage recruitment to clean the debris to enable successful outgrowth of axons in the proximal stump. During the process of axonal regrowth, activated SCs differentiate into mature SCs and highly express myelination-associated genes, such as peripheral myelin protein 22 (PMP22), myelin basic protein (MBP), and myelin-associated glycoprotein (MAG). Meanwhile, mature SCs secrete various neurotrophic factors, such as nerve growth factor (NGF) and brain-derived neurotrophic factor (BDNF), to provide a supportive microenvironment for encouraging nerve growth. Moreover, SCs can also guide axonal regrowth to the distal stump by forming Büngner bands (Scheme 1) (Cristob and Lee, 2022; Talsma et al., 2022; Yuan et al., 2022).
SCHEME 1. Schwann cells in the repair of peripheral nerve injury. After injury, the debris of injured axons in the distal stump is segmented and cleaned by Schwann cells and by recruited macrophages. Once the myelin debris is cleared, Schwann cells release a series of neurotrophic factors and guide axonal regrowth to the distal stump by forming Büngner bands.
Several studies and molecular mechanisms have demonstrated that effective modulation of Schwann cell and axon behavior is a promising strategy for PNI repair (Liu et al., 2019; Xuan et al., 2020; Huang et al., 2021). Recently, hydrogel conduits have been regarded as powerful tools for promoting PNI repair by mimicking the extracellular matrix (ECM) of nerve tissue and serving as carriers for delivering drugs, growth factors, and cells of various types (Liu et al., 2021; Rao et al., 2022; Arif et al., 2023). The ways to crosslink the polymer chains within hydrogel conduits determine the properties of conduits, such as stability, biodegradability, and release of bioactive molecules for modulating nerve regeneration. Normally, chemical crosslinking and physical crosslinking are the two main ways of fabricating hydrogel conduits. Chemical crosslinking methods include Schiff base, click chemistry, condensation reaction, or photo-crosslinking, which can equip the hydrogel conduits with improved mechanical strength and stable immobilization of bioactive molecules to match the mechanical properties of nerve tissue and achieve long-term bioactivity. On the other hand, physical crosslinking methods, such as electrostatic associations, π–π stacking, hydrogen bonds, and hydrophobic interactions, avoid the use and toxicity of crosslinkers in chemical crosslinked hydrogel conduits and draw much attention in the field of delivering growth factors for promoting nerve growth (Solomevich et al., 2023) (Scheme 2).
SCHEME 2. Chemical and physical crosslinking methods of fabrication of hydrogel conduits for promoting nerve growth.
To better enhance the therapeutic efficacy of nerve scaffolds, natural polymer-based hydrogels with ECM-associated proteins or peptides have been engineered to provide similar mechanical properties and bioactivities as the native tissues to maintain the biological functions of the SCs and accelerate axon growth (Joshi et al., 2023; Valentino et al., 2023). Aligned patterns have been created on the nerve scaffolds to guide the orientated growth and myelination of SCs (Wang et al., 2020; Gu et al., 2021). To encourage the growth of Schwann cells based on the aligned patterns, inducing newborn axonal sprouts from the proximal stump to the distal stump, poly(D,L-lactide-co-caprolactone) (PLCL) films with linear micropatterns of graphene oxide- or bioactive peptide-modified microscaled ridges and grooves have been developed to promote the aligned migration of Schwann cells to facilitate peripheral nerve regeneration (Zhang et al., 2020; Zhang et al., 2022b). Furthermore, the aligned electrospun fiber-based scaffolds containing anisotropic topological cues at the micro–nanoscale have also been fabricated to preciously mimic the microstructure of peripheral nerve tissue to improve the guidance and biological cues for supporting nerve regeneration (Yi et al., 2020; Cavanaugh et al., 2022; Chen et al., 2022) (Scheme 3).
SCHEME 3. Aligned electrospun fiber-based scaffolds containing anisotropic topological cues that promote peripheral nerve regeneration by mimicking the microstructure of nerve tissue. (A) Schematic illustration of preparation of well-aligned electrospun fibers. (B) SEM images of aligned fibers. Scale bar = 10 μm. (C) The topology of aligned fibers facilitated the differentiation of Schwann cells by activating YAP nuclear translocation. (D) Aligned fiber scaffolds benefit orientated axon growth. (Figures reproduced from Chen et al., 2022).
Various evaluation methods have been used to study the therapeutic effect of nerve scaffolds on facilitating the growth of SCs and axons in vitro and in vivo, depending on the biological functions of different scaffolds. Among them, evaluations of the locomotor behavior of animals, electroneurography of nerves, weight loss of muscles, and histological examination of regenerated nerve tissue have been considered gold standards for analyzing the in vivo therapeutic effect of bioscaffolds on promoting nerve regeneration and functional recovery in current studies (Table 1) (Liu et al., 2022; Duan et al., 2023; Fang et al., 2023; Gao et al., 2023; Jin et al., 2023; Li et al., 2023; Semmler et al., 2023; Wu et al., 2023; Yan et al., 2023; Yang et al., 2023). These studies demonstrated proper methods to assess the degree of nerve regeneration and events occurring during the nerve repair process. These experimental studies allow us to determine the effects of biomaterial-based therapies and compare different strategies for promoting PNI repair. Benefiting from well-designed evaluation methods, specific questions regarding bioscaffold-promoted nerve regeneration can gradually be answered via a combination of various methods, and a better way can be found to design improved and multi-functional bioscaffolds from the appropriate information (Scheme 4).
SCHEME 4. In vivo evaluation methods on nerve regeneration and functional recovery in sciatic nerve defect rats treated by artificial bioscaffolds, including electrophysiological recovery analysis of the muscular response to electrical nerve stimulation, histological examination of regenerated nerve tissue, and morphometric analysis of the regenerated nerve by TEM. (Figures reproduced from Chen et al., 2022; Tang et al., 2021).
Walking track analysis is usually conducted to evaluate the nerve functional recovery of a rat model after conduit implantation. During the experiment, rats are made to walk, and their footprints are recorded at the CatWalk system. For example, Wang et al., 2023 developed CYIGSR functionalized conduits to bridge 10-mm rat sciatic nerve defects, and the nerve function recovery of rats was evaluated by footprint analysis at 2 weeks post-treatment. The sciatic nerve function index (SFI) of rats in the CYIGSR functionalized conduit group was higher than that in the chitosan control group, indicating that immobilization of CYIGSR on nerve scaffolds can significantly promote nerve function recovery in PNI (Wang et al., 2023b).
Measurement of active ankle angle at the terminal stance (ATS) is another way to assess the nerve functional recovery after bioscaffold-based treatment. Lee et al. measured the ATS of rats every 3 weeks during 12 weeks post-surgery and photographed them with a 60 Hz digital camera positioned at a distance of 1 m to the animal. Rats treated with poly(lactide-co-ε-caprolactone) conduits containing NGF-loaded gelatin hydrogel showed gradual recovery of nerve function and exhibited improved ATS during 12 weeks, which was comparable to the autograft group, indicating the NGF sustainedly released from the conduits can accelerate nerve regeneration in vivo (Lee et al., 2022).
Although a large number of surgical interventions performed on patients are for the upper limb level in the clinic, sciatic nerve defect in the rat model is still dominant in experimental research. Therefore, most of the methods have been developed to assess the nerve function restoration of hindlimbs for evaluating the therapeutic effect of bioscaffolds. Walking track and ankle angle analyses have long been used in the assessment of sciatic functional nerve recovery. However, the validity of the sciatic functional index has been questioned, and it is important to find new ways to more accurately evaluate nerve functional recovery in rodents.
The restoration of electrical nerve stimulation from motor neurons to the muscles is essential for repair of nerve tissue. Thus, the muscular response to the electrical nerve stimulation is usually tested by electrophysiological recordings before the animals are sacrificed for histological analysis. Motor unit number estimation (MUNE) is a non-invasive electrophysiologic method to monitor the muscular response to electrical nerve stimulation by McComas et al. (1971). In the study by Cicero et al., electrophysiological recordings were conducted to assess the muscular response to electrical nerve stimulation at 120 days after implantation of nerve conduit in rats with a pair of mono-polar recording needle electrodes placed at the belly and the tibialis anterior (TA) and the gastrocnemious (GA) medialis muscles. The sciatic nerve was stimulated with square-wave pulses of 0.1-ms duration, and the compound muscle action potential (CMAP) response was recorded. Compared to the contralateral uninjured nerve, a significant decrease in MUNE in the operated limb was found. Meanwhile, the recovery of MUNE in the TA muscle of rats was much better in the poly-butylene succinate scaffold group compared to the untreated group, which was comparable to the healthy limb muscle, indicating that the poly-butylene succinate scaffolds effectively guided peroneal fibers (Cicero et al., 2022).
In PNI, the denervated muscle undergoes atrophy and loss of muscle mass. When the muscle is innervated, the degeneration of the muscle can be interrupted and the muscle can recover its tropism (Ai et al., 2019; Rahmati et al., 2021). Therefore, the evaluation of the wet weight of the innervated gastrocnemius muscle reflects the index of nerve regeneration. Gastrocnemius muscles are usually harvested, and the wet weight of the muscle at the surgical site is compared with that at the non-surgical site. In Zaminy et al. (2021), 7-mm sciatic nerve defect in rats was bridged by using a decellularized nerve xenograft, and the recovery rate of the wet weight of the gastrocnemius muscle was significantly higher in the nerve autograft and acellular nerve xenograft groups without a significant difference at 8 weeks after surgery, demonstrating the promising capacity of acellular nerve xenografts on repairing sciatic nerve defects in vivo.
Immunofluorescence staining is a typical method to effectively visualize myelination, angiogenesis, axonal extension, and immune state within regenerated nerve tissue for evaluating bioscaffold-promoted nerve regeneration. S100β expression is a marker commonly used to assess glial cells in nerve repair, such as SCs in peripheral nerves; NF200 is a marker protein for assessment of nerve filaments. Dong et al. developed an aligned P(MMD-co-LA) fibrous membrane conduit loaded with deferoxamine (A_PDPLA/DFO) to bridge a 10-mm sciatic nerve defect in rats. Nerve paraffin sections were performed to detect axonal regeneration via immunofluorescence staining with a double staining of NF200 and S100β. At 24 weeks post-surgery, an identical nerve structure was visualized via the fluorescence of NF200 and S100β in the A_PDPLA/DFO group, and the positive areas of NF200 and S100β within the regenerated nerve were comparable to the autograft group, indicating that the release of deferoxamine from the A_PDPLA/DFO conduit successfully accelerated the process of nerve repair by promoting cell migration and guiding polarization of the vasculature (Dong et al., 2022).
The expression level of Ki67 in the regenerated nerve can reflect the proliferation state of axons, and the apoptotic state of axons can be evaluated using immunofluorescence staining of C-caspase3 in nerve tissues. Jiang et al., by immunofluorescent staining of Ki67 and C-caspase3, found that the protein level of Ki67 in the regenerated nerve tissue within the multilayered melatonin/reduced graphene oxide/polycaprolactone (MLT/RGO/PCL) conduit was significantly higher than that in the autograft and the protein level of C-caspase-3 was very low in the MLT/RGO/PCL group, indicating that the combination of MLT and RGO enhanced the axonal regrowth and reduced cell apoptosis in PNI repair (Jiang et al., 2022b).
The inflammatory response during the process of PNI repair greatly affects the outcome of bioscaffold-based treatment. To assess the infiltration of inflammatory cells and demyelination within the nerve tissues, Jahromi et al. collected the sciatic nerve tissues from 10-mm sciatic nerve defects in rats treated with nerve conduits containing Schwann cells and curcumin at 12 weeks post-surgery and stained them with hematoxylin and eosin (H&E) and Luxol fast blue (LFB). The histopathologic evaluation of nerve tissues indicated that incorporating Schwann cells and curcumin in nerve conduits significantly promoted sciatic nerve regeneration and neovascularization with minimal inflammatory responses (Jahromi et al., 2020)
However, there are some limitations in histopathologically evaluating regenerated nerve tissue via immunostaining and histochemical staining. As the aim of each experiment is different, certain dyes must be chosen carefully for single or even multiple tracing according to the interest of researchers in specific regeneration events. The results are largely based on the fixation methods and working conditions such as temperature and humidity. Moreover, extreme care must be taken for the quantitative analysis of nerve fibers and protein expression in the regenerated nerve tissue through immunofluorescence staining assays. Appropriate quantification in immunofluorescence-based methods usually depends on image acquisition and comparing the results to appropriate positive and negative controls to eliminate the errors.
Morphometric analysis of ultrathin sections of the regenerated nerve is another effective way to determine the diameter of myelinated nerve fibers and the thickness of myelin sheaths. Zhu et al. cut tissue sections from the middle part of the regenerated nerves and stained them with uranyl acetate and lead citrate. By using transmission electron microscopy (TEM) evaluation, they found compact and uniformly structured myelinated nerve fibers in the ECM scaffold. The myelin sheathes of myelinated nerve fibers were relatively dense with intact basal membranes, indicating that ECM scaffolds with microchannels held a strong capacity for guiding nerve regeneration and prompting functional recovery (Zhu et al., 2019). In addition to analyzing the mean diameter of myelinated nerve fibers and myelin sheath thickness, Amini et al. (2020) also imaged cross-sections of the nerve conduit after implantation by TEM and calculated the G-ratio by comparing the inner axonal diameter to the total outer diameter for determining the speed of fiber conduction. Thicker axonal diameter of myelinated nerve fibers was found in regenerated nerves with a G-ratio of 0.6 in PCL with 15% of the lignin nanoparticle conduit group, indicating the dominant role of lignin in nerve repair (Amini et al., 2020).
SCs can create a favorable microenvironment for axonal growth by releasing neurotrophic factors, which plays an important role in PNI recovery. Therefore, it is of great importance to evaluate the capacity of bioengineered nerve conduits in promoting neurotrophic factor release from SCs in vivo. In Yang et al.’s study, the sciatic nerve tissue in conduits was harvested and transferred immediately into liquid nitrogen for further RNA extraction, and the gene expression of neurotrophic factors, vascular endothelial growth factor (VEGF), and myelin genes was evaluated by using the qRT-PCR assay. It was found that rats in peptide hydrogel groups exhibited significantly enhanced gene expression of NGF, BDNF, VEGF, and PMP22, indicating that dual-functionalized peptide hydrogels of RAD/IKV/RGI promoted SC myelination and development to facilitate growth factor secretion for nerve regeneration (Yang et al., 2020). Mao et al. assessed the changes in key factors (NGF and VEGF) that contribute to nerve regeneration in regenerated nerve tissues using Western blotting. Results showed that the conduit with piezoelectric stimulation effectively promoted NGF and VEGF expression in nerve tissues at 28 days post-surgery, indicating that piezoelectric stimulation of the conduit had a promising therapeutic effect on PNI repair by enhancing neurotrophic factor secretion (Mao et al., 2022).
Overall, the treatment for large-gapped PNI is still suboptimal, which affects patients’ quality of life and causes significant healthcare costs. The artificial scaffolds can modulate Schwann cell behavior and encourage axonal regrowth in nerve regeneration by providing various physical and biological cues to create a favorable microenvironment, which have emerged as alternative tools for prompting PNI recovery. In this review, we have focused on discussing experimental methods for evaluating Schwann cell behavior and axonal extension regulated by bioscaffolds in PNI repair. Since the process of peripheral nerve regeneration is very complicated and involves various cell types, such as SCs, neurons, fibroblasts, macrophages, and even T cells, there is no single evaluation method that can comprehensively answer all the questions in bioscaffold-based therapy. Therefore, it is important to combine appropriate evaluation methods to study the therapeutic effect of novel biomaterials in terms of animal functional behavior, morphological and histopathologic evaluation of regenerated nerves, and analyses of growth factors at the biomolecular level.
JL: conceptualization, funding acquisition, project administration, resources, supervision, visualization, writing–original draft, and writing–review and editing. CH: data curation, formal analysis, software, validation, visualization, and writing–original draft. SZ: data curation, formal analysis, software, validation, visualization, and writing–original draft. YZ: formal analysis, software, validation, visualization, and writing–original draft. MZ: software, validation, visualization, and writing–original draft. XT: software, validation, visualization, and writing–original draft. QL: software, validation, visualization, and writing–original draft. LX: conceptualization, funding acquisition, resources, supervision, validation, writing–original draft, and writing–review and editing. YY: conceptualization, funding acquisition, resources, software, writing–original draft, and writing–review and editing.
The authors declare that financial support was received for the research, authorship, and/or publication of this article. This work was financially supported by the National Natural Science Foundation of China (Project No. 32230057), the Natural Science Foundation of Jiangsu Province (Project No. BE2022766), the Natural Science Foundation of the Higher Education Institutions of Jiangsu Province (22KJA310003), and Jiangsu Provincial Key Medical Center.
The authors declare that the research was conducted in the absence of any commercial or financial relationships that could be construed as a potential conflict of interest.
All claims expressed in this article are solely those of the authors and do not necessarily represent those of their affiliated organizations, or those of the publisher, the editors, and the reviewers. Any product that may be evaluated in this article, or claim that may be made by its manufacturer, is not guaranteed or endorsed by the publisher.
Acheta, J., Stephens, S. B. Z., Belin, S., and Poitelon, Y. (2021). Therapeutic low-intensity ultrasound for peripheral nerve regeneration - a Schwann cell perspective. Front. Cell. Neurosci. 15, 812588. doi:10.3389/fncel.2021.812588
Adam, M., Lin, L., Makin, A., Zhang, X. F., Zhou, L. X., Liao, X. Y., et al. (2023). Glial cell line-derived neurotrophic factor and brain-derived neurotrophic factor regulate the interaction between astrocytes and Schwann cells at the trigeminal root entry zone. Neural Regen. Res. 18 (6), 1364–1370. doi:10.4103/1673-5374.354517
Ai, A., Behforouz, A., Ehterami, A., Sadeghvaziri, N., Jalali, S., Farzamfar, S., et al. (2019). Sciatic nerve regeneration with collagen type I hydrogel containing chitosan nanoparticle loaded by insulin. Int. J. Polym. Mater. Polym. Biomaterials 68 (18), 1133–1141. doi:10.1080/00914037.2018.1534114
Amini, S., Saudi, A., Amirpour, N., Jahromi, M., Najafabadi, S. S., Kazemi, M., et al. (2020). Application of electrospun polycaprolactone fibers embedding lignin nanoparticle for peripheral nerve regeneration: in vitro and in vivo study. Int. J. Biol. Macromol. 159, 154–173. doi:10.1016/j.ijbiomac.2020.05.073
Arif, Z. U., Khalid, M. Y., Noroozi, R., Hossain, M., Shi, H. H., Tariq, A., et al. (2023). Additive manufacturing of sustainable biomaterials for biomedical applications. Asian J. Pharm. Sci. 18 (3), 100812. doi:10.1016/j.ajps.2023.100812
Bombeiro, A. L., Santini, J. C., Thome, R., Ferreira, E. R. L., Nunes, S. L. O., Moreira, B. M., et al. (2016). Enhanced immune response in immunodeficient mice improves peripheral nerve regeneration following axotomy. Front. Cell. Neurosci. 10, 151. doi:10.3389/fncel.2016.00151
Bosch-Queralt, M., Fledrich, R., and Stassart, R. M. (2023). Schwann cell functions in peripheral nerve development and repair. Neurobiol. Dis. 176, 105952. doi:10.1016/j.nbd.2022.105952
Brifault, C., Romero, H., Van-Enoo, A., Pizzo, D., Azmoon, P., Kwon, H., et al. (2020). Deletion of the gene encoding the NMDA receptor GluN1 subunit in Schwann cells causes ultrastructural changes in Remak bundles and hypersensitivity in pain processing. J. Neurosci. 40 (47), 9121–9136. doi:10.1523/jneurosci.0663-20.2020
Cavanaugh, M., Asheghali, D., Motta, C. M., Silantyeva, E., Nikam, S. P., Becker, M. L., et al. (2022). Influence of touch-spun nanofiber diameter on contact guidance during peripheral nerve repair. Biomacromolecules 23 (6), 2635–2646. doi:10.1021/acs.biomac.2c00379
Chen, S. H., Gu, X. P., Li, R. D., An, S. H., and Wang, Z. J. (2023). Genome-wide analysis of histone H3 lysine 27 trimethylation profiles in sciatic nerve of chronic constriction injury rats. Neurochem. Res. 48 (6), 1945–1957. doi:10.1007/s11064-023-03879-y
Chen, X., Tang, X., Wang, Y., Gu, X., Huang, T., Yang, Y., et al. (2022). Silk-inspired fiber implant with multi-cues enhanced bionic microenvironment for promoting peripheral nerve repair. Biomater. Adv. 135, 112674. doi:10.1016/j.msec.2022.112674
Choe, G., Han, U. G., Ye, S., Kang, S., Yoo, J., Cho, Y. S., et al. (2023). Effect of electrical stimulation on nerve-guided facial nerve regeneration. Acs Biomaterials Sci. Eng. 9 (6), 3512–3521. doi:10.1021/acsbiomaterials.3c00222
Cicero, L., Licciardi, M., Cirincione, R., Puleio, R., Giammona, G., Giglia, G., et al. (2022). Polybutylene succinate artificial scaffold for peripheral nerve regeneration. J. Biomed. Mater. Res. Part B-Applied Biomaterials 110 (1), 125–134. doi:10.1002/jbm.b.34896
Cristob, C. D., and Lee, H. K. (2022). Development of myelinating glia: an overview. Glia 70 (12), 2237–2259. doi:10.1002/glia.24238
Davoli-Ferreira, M., de Lima, K. A., Fonseca, M. M., Guimaraes, R. M., Gomes, F. I., Cavallini, M. C., et al. (2020). Regulatory T cells counteract neuropathic pain through inhibition of the Th1 response at the site of peripheral nerve injury. Pain 161 (8), 1730–1743. doi:10.1097/j.pain.0000000000001879
Dong, Q., Yang, X., Liang, X., Liu, J., Wang, B., Zhao, Y., et al. (2023). Composite hydrogel conduit incorporated with platelet-rich plasma improved the regenerative microenvironment for peripheral nerve repair. Acs Appl. Mater. Interfaces 15 (20), 24120–24133. doi:10.1021/acsami.3c02548
Dong, X., Wu, P., Yan, L., Liu, K., Wei, W., Cheng, Q., et al. (2022). Oriented nanofibrous P(MMD-co-LA)/Deferoxamine nerve scaffold facilitates peripheral nerve regeneration by regulating macrophage phenotype and revascularization. Biomaterials 280, 121288. doi:10.1016/j.biomaterials.2021.121288
Duan, G., Li, C., Yan, X., Yang, S., Wang, S., Sun, X., et al. (2023). Construction of a mineralized collagen nerve conduit for peripheral nerve injury repair. Regen. Biomater. 10, rbac089. doi:10.1093/rb/rbac089
Fang, Y., Wang, C., Liu, Z., Ko, J., Chen, L., Zhang, T., et al. (2023). 3D printed conductive multiscale nerve guidance conduit with hierarchical fibers for peripheral nerve regeneration. Adv. Sci. (Weinh) 10 (12), e2205744. doi:10.1002/advs.202205744
Faroni, A., Castelnovo, L. F., Procacci, P., Caffino, L., Fumagalli, F., Melfi, S., et al. (2014). Deletion of GABA-B receptor in Schwann cells regulates Remak bundles and small nociceptive C-fibers. Glia 62 (4), 548–565. doi:10.1002/glia.22625
Gao, S., Tang, Y., Sun, W., Liu, Z., Zhao, T., Li, X., et al. (2023). 3D-bioprinted GelMA nerve guidance conduits promoted peripheral nerve regeneration by inducing trans-differentiation of MSCs into SCLCs via PIEZO1/YAP axis. Mater. Today Adv. 17, 100325. doi:10.1016/j.mtadv.2022.100325
Gu, X. Y., Chen, X. L., Tang, X. X., Zhou, Z. H., Huang, T. T., Yang, Y. M., et al. (2021). Pure-silk fibroin hydrogel with stable aligned micropattern toward peripheral nerve regeneration. Nanotechnol. Rev. 10 (1), 10–19. doi:10.1515/ntrev-2021-0002
He, L. M., Xiao, Q., Zhao, Y. Y., Li, J., Reddy, S., Shi, X. S., et al. (2020). Engineering an injectable electroactive nanohybrid hydrogel for boosting peripheral nerve growth and myelination in combination with electrical stimulation. Acs Appl. Mater. Interfaces 12 (47), 53150–53163. doi:10.1021/acsami.0c16885
Heath, C. A., and Rutkowski, G. E. (1998). The development of bioartificial nerve grafts for peripheral-nerve regeneration. Trends Biotechnol. 16 (4), 163–168. doi:10.1016/s0167-7799(97)01165-7
Hercher, D., Nguyen, M. Q., and Dworak, H. (2022). Extracellular vesicles and their role in peripheral nerve regeneration. Exp. Neurol. 350, 113968. doi:10.1016/j.expneurol.2021.113968
Houshyar, S., Bhattacharyya, A., and Shanks, R. (2019). Peripheral nerve conduit: materials and structures. Acs Chem. Neurosci. 10 (8), 3349–3365. doi:10.1021/acschemneuro.9b00203
Huang, Q., Cai, Y. T., Zhang, X., Liu, J. C., Liu, Z. J., Li, B., et al. (2021). Aligned graphene mesh-supported double network natural hydrogel conduit loaded with netrin-1 for peripheral nerve regeneration. Acs Appl. Mater. Interfaces 13 (1), 112–122. doi:10.1021/acsami.0c16391
Huang, W. C., Lin, C. C., Chiu, T. W., and Chen, S. Y. (2022). 3D gradient and linearly aligned magnetic microcapsules in nerve guidance conduits with remotely spatiotemporally controlled release to enhance peripheral nerve repair. Acs Appl. Mater. Interfaces 14 (41), 46188–46200. doi:10.1021/acsami.2c11362
Hui, Y., Yan, Z., Yang, H., Xu, X., Yuan, W.-E., and Qian, Y. (2022). Graphene family nanomaterials for stem cell neurogenic differentiation and peripheral nerve regeneration. ACS Appl. bio Mater. 5 (10), 4741–4759. doi:10.1021/acsabm.2c00663
Idrisova, K. F., Zeinalova, A. K., Masgutova, G. A., Bogov, A. A., Allegrucci, C., Syromiatnikova, V. Y., et al. (2022). Application of neurotrophic and proangiogenic factors as therapy after peripheral nervous system injury. Neural Regen. Res. 17 (6), 1240–1247. doi:10.4103/1673-5374.327329
Jahromi, H. K., Farzin, A., Hasanzadeh, E., Barough, S. E., Mahmoodi, N., Najafabadi, M. R. H., et al. (2020). Enhanced sciatic nerve regeneration by poly-L-lactic acid/multi-wall carbon nanotube neural guidance conduit containing Schwann cells and curcumin encapsulated chitosan nanoparticles in rat. Mater. Sci. Eng. C-Materials Biol. Appl. 10, 110564. doi:10.1016/j.msec.2019.110564
Jessen, K. R., and Arthur-Farraj, P. (2019). Repair Schwann cell update: adaptive reprogramming, EMT, and stemness in regenerating nerves. Glia 67 (3), 421–437. doi:10.1002/glia.23532
Jiang, H., Wang, X., Li, X., Jin, Y., Yan, Z., Yao, X., et al. (2022b). A multifunctional ATP-generating system by reduced graphene oxide-based scaffold repairs neuronal injury by improving mitochondrial function and restoring bioelectricity conduction. Mater. Today Bio 13, 100211. doi:10.1016/j.mtbio.2022.100211
Jiang, Y. H., Tang, X. X., Li, T., Ling, J., and Yang, Y. M. (2022a). The success of biomaterial-based tissue engineering strategies for peripheral nerve regeneration. Front. Bioeng. Biotechnol. 10, 1039777. doi:10.3389/fbioe.2022.1039777
Jin, B., Yu, Y., Chen, X., Yang, Y., Xiong, Y., Im, Y. J., et al. (2023). Microtubes with gradient decellularized porcine sciatic nerve matrix from microfluidics for sciatic nerve regeneration. Bioact. Mater. 21, 511–519. doi:10.1016/j.bioactmat.2022.08.027
Joshi, A., Choudhury, S., Baghel, V. S., Ghosh, S., Gupta, S., Lahiri, D., et al. (2023). 4D printed programmable shape-morphing hydrogels as intraoperative self-folding nerve conduits for sutureless neurorrhaphy. Adv. Healthc. Mater. 12, e2300701. doi:10.1002/adhm.202300701
Juckett, L., Saffari, T. M., Ormseth, B., Senger, J. L., and Moore, A. M. (2022). The effect of electrical stimulation on nerve regeneration following peripheral nerve injury. Biomolecules 12 (12), 1856. doi:10.3390/biom12121856
Kou, Y. H., Yu, Y. L., Zhang, Y. J., Han, N., Yin, X. F., Yuan, Y. S., et al. (2019). Repair of peripheral nerve defects by nerve transposition using small gap bio-sleeve suture with different inner diameters at both ends. Neural Regen. Res. 14 (4), 706–712. doi:10.4103/1673-5374.247475
Lee, H. S., Jeon, E. Y., Nam, J. J., Park, J. H., Choi, I. C., Kim, S. H., et al. (2022). Development of a regenerative porous PLCL nerve guidance conduit with swellable hydrogel-based microgrooved surface pattern via 3D printing. Acta Biomater. 141, 219–232. doi:10.1016/j.actbio.2022.01.042
Leite, A. P. S., Pinto, C. G., Tiburcio, F. C., Sartori, A. A., de Castro Rodrigues, A., Barraviera, B., et al. (2019). Heterologous fibrin sealant potentiates axonal regeneration after peripheral nerve injury with reduction in the number of suture points. Injury 50 (4), 834–847. doi:10.1016/j.injury.2019.03.027
Li, Y., Chen, Z., Zhou, J., Guan, Y., Xing, J., Niu, Z., et al. (2023). Combining chitin biological conduits with injectable adipose tissue-derived decellularised matrix hydrogels loaded with adipose-derived mesenchymal stem cells for the repair of peripheral nerve defects in rats. Colloids Surfaces A Physicochem. Eng. Aspects, 130743. doi:10.1016/j.colsurfa.2022.130743
Liu, S., Rao, Z., Zou, J., Chen, S., Zhu, Q., Liu, X., et al. (2021). Properties regulation and biological applications of decellularized peripheral nerve matrix hydrogel. ACS Appl. bio Mater. 4 (8), 6473–6487. doi:10.1021/acsabm.1c00616
Liu, Y. J., Chen, X. F., Zhou, L. P., Rao, F., Zhang, D. Y., and Wang, Y. H. (2022). A nerve conduit filled with Wnt5a-loaded fibrin hydrogels promotes peripheral nerve regeneration. CNS Neurosci. Ther. 28 (1), 145–157. doi:10.1111/cns.13752
Liu, Y. M., Yu, F. L., Zhang, B. B., Zhou, M., Bei, Y., Zhang, Y. F., et al. (2019). Improving the protective effects of aFGF for peripheral nerve injury repair using sulfated chitooligosaccharides. Asian J. Pharm. Sci. 14 (5), 511–520. doi:10.1016/j.ajps.2018.09.007
Lopez-Cebral, R., Silva-Correia, J., Reis, R. L., Silva, T. H., and Oliveira, J. M. (2017). Peripheral nerve injury: current challenges, conventional treatment approaches, and new trends in biomaterials-based regenerative strategies. Acs Biomaterials Sci. Eng. 3 (12), 3098–3122. doi:10.1021/acsbiomaterials.7b00655
Manto, K. M., Govindappa, P. K., Parisi, D., Karuman, Z., Martinazzi, B., Hegarty, J. P., et al. (2021). (4-Aminopyridine)-PLGA-PEG as a novel thermosensitive and locally injectable treatment for acute peripheral nerve injury. ACS Appl. bio Mater. 4 (5), 4140–4151. doi:10.1021/acsabm.0c01566
Mao, R., Yu, B., Cui, J., Wang, Z., Huang, X., Yu, H., et al. (2022). Piezoelectric stimulation from electrospun composite nanofibers for rapid peripheral nerve regeneration. Nano Energy 98, 107322. doi:10.1016/j.nanoen.2022.107322
McComas, A. J., Fawcett, P. R., Campbell, M. J., and Sica, R. E. (1971). Electrophysiological estimation of the number of motor units within a human muscle. J. neurology, Neurosurg. psychiatry 34 (2), 121–131. doi:10.1136/jnnp.34.2.121
Meena, P., Kakkar, A., Kumar, M., Khatri, N., Nagar, R. K., Singh, A., et al. (2021). Advances and clinical challenges for translating nerve conduit technology from bench to bed side for peripheral nerve repair. Cell Tissue Res. 383 (2), 617–644. doi:10.1007/s00441-020-03301-x
Padovano, W. M., Dengler, J., Patterson, M. M., Yee, A., Snyder-Warwick, A. K., Wood, M. D., et al. (2022). Incidence of nerve injury after extremity trauma in the United States. Hand (N Y) 17 (4), 615–623. doi:10.1177/1558944720963895
Procacci, N. M., Hastings, R. L., Aziz, A. A., Christiansen, N. M., Zhao, J., DeAngeli, C., et al. (2023). Kir4.1 is specifically expressed and active in non-myelinating Schwann cells. Glia 71 (4), 926–944. doi:10.1002/glia.24315
Rahmati, M., Ehterami, A., Saberani, R., Abbaszadeh-Goudarzi, G., Rezaei Kolarijani, N., Khastar, H., et al. (2021). Improving sciatic nerve regeneration by using alginate/chitosan hydrogel containing berberine. Drug Deliv. Transl. Res. 11 (5), 1983–1993. doi:10.1007/s13346-020-00860-y
Rao, Z. L., Lin, Z. D., Song, P. P., Quan, D. P., and Bai, Y. (2022). Biomaterial-based Schwann cell transplantation and Schwann cell-derived biomaterials for nerve regeneration. Front. Cell. Neurosci. 16, 926222. doi:10.3389/fncel.2022.926222
Seddon, H. J., Medawar, P. B., and Smith, H. (1943). Rate of regeneration of peripheral nerves in man. J. physiology 102 (2), 191–215. doi:10.1113/jphysiol.1943.sp004027
Semmler, L., Naghilou, A., Millesi, F., Wolf, S., Mann, A., Stadlmayr, S., et al. (2023). Silk-in-Silk nerve guidance conduits enhance regeneration in a rat sciatic nerve injury model. Adv. Healthc. Mater. 12 (11), e2203237. doi:10.1002/adhm.202203237
Sharifi, M., Farahani, M. K., Salehi, M., Atashi, A., Alizadeh, M., Kheradmandi, R., et al. (2023). Exploring the physicochemical, electroactive, and biodelivery properties of metal nanoparticles on peripheral nerve regeneration. Acs Biomaterials Sci. Eng. 9 (1), 106–138. doi:10.1021/acsbiomaterials.2c01216
Smith, D. H., Burrell, J. C., Browne, K. D., Katiyar, K. S., Ezra, M. I., Dutton, J. L., et al. (2022). Tissue-engineered grafts exploit axon-facilitated axon regeneration and pathway protection to enable recovery after 5-cm nerve defects in pigs. Sci. Adv. 8 (44), eabm3291. doi:10.1126/sciadv.abm3291
Solomevich, S. O., Oranges, C. M., Kalbermatten, D. F., Schwendeman, A., and Madduri, S. (2023). Natural polysaccharides and their derivatives as potential medical materials and drug delivery systems for the treatment of peripheral nerve injuries. Carbohydr. Polym. 315, 120934. doi:10.1016/j.carbpol.2023.120934
Sunderland, S. (1947). Rate of regeneration in human peripheral nerves; analysis of the interval between injury and onset of recovery. Archives neurology psychiatry 58 (3), 251–295. doi:10.1001/archneurpsyc.1947.02300320002001
Talsma, A. D., Niemi, J. P., Pachter, J. S., and Zigmond, R. E. (2022). The primary macrophage chemokine, CCL2, is not necessary after a peripheral nerve injury for macrophage recruitment and activation or for conditioning lesion enhanced peripheral regeneration. J. Neuroinflammation 19 (1), 179. doi:10.1186/s12974-022-02497-9
Tang, X. X., Gu, X. Y., Huang, T. T., Chen, X. L., Zhou, Z. H., Yang, Y. M., et al. (2021). Anisotropic silk-inspired nerve conduit with peptides improved the microenvironment for long-distance peripheral nerve regeneration. Acs Macro Lett. 10 (12), 1501–1509. doi:10.1021/acsmacrolett.1c00533
Tang, X. X., Li, Q. Y., Huang, T. T., Zhang, H., Chen, X. L., Ling, J., et al. (2022). Regenerative role of T cells in nerve repair and functional recovery. Front. Immunol. 13, 923152. doi:10.3389/fimmu.2022.923152
Taylor, C. A., Braza, D., Rice, J. B., and Dillingham, T. (2008). The incidence of peripheral nerve injury in extremity trauma. Am. J. Phys. Med. Rehabilitation 87 (5), 381–385. doi:10.1097/phm.0b013e31815e6370
Thibodeau, A., Galbraith, T., Fauvel, C. M., Khuong, H. T., and Berthod, F. (2022). Repair of peripheral nerve injuries using a prevascularized cell-based tissue-engineered nerve conduit. Biomaterials, 121269. doi:10.1016/j.biomaterials.2021.121269
Valentino, C., Vigani, B., Zucca, G., Ruggeri, M., Marrubini, G., Boselli, C., et al. (2023). Design of novel mechanically resistant and biodegradable multichannel platforms for the treatment of peripheral nerve injuries. Biomacromolecules 24 (4), 1731–1743. doi:10.1021/acs.biomac.2c01498
Vijayavenkataraman, S. (2020). Nerve guide conduits for peripheral nerve injury repair: a review on design, materials and fabrication methods. Acta Biomater. 106, 54–69. doi:10.1016/j.actbio.2020.02.003
Wang, J., Xiong, H., Zhu, T. H., Liu, Y., Pan, H. B., Fan, C. Y., et al. (2020). Bioinspired multichannel nerve guidance conduit based on shape memory nanofibers for potential application in peripheral nerve repair. Acs Nano 14 (10), 12579–12595. doi:10.1021/acsnano.0c03570
Wang, J., Zheng, W., Chen, L., Zhu, T. H., Shen, W., Fan, C. Y., et al. (2019). Enhancement of Schwann cells function using graphene-oxide-modified nanofiber scaffolds for peripheral nerve regeneration. Acs Biomaterials Sci. Eng. 5 (5), 2444–2456. doi:10.1021/acsbiomaterials.8b01564
Wang, J. Y., Chen, H. F., Hou, W. L., Han, Q. J., and Wang, Z. Y. (2023a). Hippo pathway in Schwann cells and regeneration of peripheral nervous system. Dev. Neurosci. 45, 276–289. doi:10.1159/000530621
Wang, Q., Li, H. Y., Ling, Z. M., Chen, G., and Wei, Z. Y. (2022). Inhibition of Schwann cell pannexin 1 attenuates neuropathic pain through the suppression of inflammatory responses. J. Neuroinflammation 19 (1), 244–316. doi:10.1186/s12974-022-02603-x
Wang, Y., Zhu, L., Wei, L., Zhou, Y., Yang, Y., and Zhang, L. (2023b). A bio-orthogonally functionalized chitosan scaffold with esterase-activatable release for nerve regeneration. Int. J. Biol. Macromol. 229, 146–157. doi:10.1016/j.ijbiomac.2022.12.113
Wu, W., Dong, Y., Liu, H., Jiang, X., Yang, L., Luo, J., et al. (2023). 3D printed elastic hydrogel conduits with 7,8-dihydroxyflavone release for peripheral nerve repair. Mater. Today Bio 20, 100652. doi:10.1016/j.mtbio.2023.100652
Xiong, F., Wei, S., Wu, S., Jiang, W., Li, B., Xuan, H., et al. (2023). Aligned electroactive electrospun fibrous scaffolds for peripheral nerve regeneration. ACS Appl. Mater. interfaces 15 (35), 41385–41402. doi:10.1021/acsami.3c09237
Xuan, H., Tang, X., Zhu, Y., Ling, J., and Yang, Y. (2020). Freestanding hyaluronic acid/silk-based self-healing coating toward tissue repair with antibacterial surface. ACS Appl. bio Mater. 3 (3), 1628–1635. doi:10.1021/acsabm.9b01196
Yan, Z., Ye, T., Yang, L., Jiang, H., Chen, C., Chen, S., et al. (2023). Nanobiology dependent therapeutic convergence between biocompatibility and bioeffectiveness of graphene oxide quantum dot scaffold for immuno-inductive angiogenesis and nerve regeneration. Adv. Funct. Mater. 33 (9), 2211709. doi:10.1002/adfm.202211709
Yang, J., Hsu, C. C., Cao, T. T., Ye, H., Chen, J., and Li, Y. Q. (2023). A hyaluronic acid granular hydrogel nerve guidance conduit promotes regeneration and functional recovery of injured sciatic nerves in rats. Neural Regen. Res. 18 (3), 657–663. doi:10.4103/1673-5374.350212
Yang, S., Wang, C., Zhu, J., Lu, C., Li, H., Chen, F., et al. (2020). Self-assembling peptide hydrogels functionalized with LN- and BDNF- mimicking epitopes synergistically enhance peripheral nerve regeneration. Theranostics 10 (18), 8227–8249. doi:10.7150/thno.44276
Yi, B. C., Shen, Y. B., Tang, H., Wang, X. L., and Zhang, Y. Z. (2020). Stiffness of the aligned fibers affects structural and functional integrity of the oriented endothelial cells. Acta Biomater. 108, 237–249. doi:10.1016/j.actbio.2020.03.022
Yi, S., Xu, L., and Gu, X. (2019). Scaffolds for peripheral nerve repair and reconstruction. Exp. Neurol. 319, 112761. doi:10.1016/j.expneurol.2018.05.016
Yuan, Y. M., Wang, Y., Wu, S. H., and Zhao, M. Y. (2022). Review: myelin clearance is critical for regeneration after peripheral nerve injury. Front. Neurology 13, 908148. doi:10.3389/fneur.2022.908148
Zaminy, A., Sayad-Fathi, S., Kasmaie, F. M., Jahromi, Z., and Zendedel, A. (2021). Decellularized peripheral nerve grafts by a modified protocol for repair of rat sciatic nerve injury. Neural Regen. Res. 16 (6), 1086–1092. doi:10.4103/1673-5374.300449
Zhang, D., Li, Z., Shi, H., Yao, Y., Du, W., Lu, P., et al. (2022b). Micropatterns and peptide gradient on the inner surface of a guidance conduit synergistically promotes nerve regeneration in vivo. Bioact. Mater. 9, 134–146. doi:10.1016/j.bioactmat.2021.07.010
Zhang, D., Yao, Y., Duan, Y., Yu, X., Shi, H., Nakkala, J. R., et al. (2020). Surface-anchored graphene oxide nanosheets on cell-scale micropatterned poly(d,l-lactide-co-caprolactone) conduits promote peripheral nerve regeneration. ACS Appl. Mater. Interfaces 12 (7), 7915–7930. doi:10.1021/acsami.9b20321
Zhang, H., Guo, J. H., Wang, Y., Shang, L. R., Chai, R. J., and Zhao, Y. J. (2022a). Natural polymer-derived bioscaffolds for peripheral nerve regeneration. Adv. Funct. Mater. 32 (41), 2203829. doi:10.1002/adfm.202203829
Zhang, H., Lan, D., Wu, B., Chen, X., Li, X., Li, Z., et al. (2023a). Electrospun piezoelectric scaffold with external mechanical stimulation for promoting regeneration of peripheral nerve injury. Biomacromolecules 24 (7), 3268–3282. doi:10.1021/acs.biomac.3c00311
Zhang, M., An, H., Gu, Z., Huang, Z., Zhang, F., Wen, Y., et al. (2023b). Mimosa-inspired stimuli-responsive curling bioadhesive tape promotes peripheral nerve regeneration. Adv. Mater. 35, e2212015. doi:10.1002/adma.202212015
Zhu, M., Li, W., Dong, X., Yuan, X., Midgley, A. C., Chang, H., et al. (2019). In vivo engineered extracellular matrix scaffolds with instructive niches for oriented tissue regeneration. Nat. Commun. 10, 4620. doi:10.1038/s41467-019-12545-3
Keywords: peripheral nerve injury, nerve regeneration, natural polymer, Schwann cell, neurotrophic factors
Citation: Ling J, He C, Zhang S, Zhao Y, Zhu M, Tang X, Li Q, Xu L and Yang Y (2023) Progress in methods for evaluating Schwann cell myelination and axonal growth in peripheral nerve regeneration via scaffolds. Front. Bioeng. Biotechnol. 11:1308761. doi: 10.3389/fbioe.2023.1308761
Received: 09 October 2023; Accepted: 20 November 2023;
Published: 07 December 2023.
Edited by:
Bin Li, Soochow University, ChinaReviewed by:
Yikai Xu, Queen’s University Belfast, United KingdomCopyright © 2023 Ling, He, Zhang, Zhao, Zhu, Tang, Li, Xu and Yang. This is an open-access article distributed under the terms of the Creative Commons Attribution License (CC BY). The use, distribution or reproduction in other forums is permitted, provided the original author(s) and the copyright owner(s) are credited and that the original publication in this journal is cited, in accordance with accepted academic practice. No use, distribution or reproduction is permitted which does not comply with these terms.
*Correspondence: Liming Xu, eHVsaW1pbmdAbmlmZGMub3JnLmNu; Yumin Yang, eWFuZ3ltQG50dS5lZHUuY24=
Disclaimer: All claims expressed in this article are solely those of the authors and do not necessarily represent those of their affiliated organizations, or those of the publisher, the editors and the reviewers. Any product that may be evaluated in this article or claim that may be made by its manufacturer is not guaranteed or endorsed by the publisher.
Research integrity at Frontiers
Learn more about the work of our research integrity team to safeguard the quality of each article we publish.