- 1Department of Oral and Maxillofacial Surgery, School of Stomatology, Shandong First Medical University, Jinan, Shandong, China
- 2The CONVERSATIONALIST Club and Department of Stomatological Technology, School of Stomatology, Shandong First Medical University, Jinan, Shandong, China
- 3Department and Research Institute of Dental Biomaterials and Bioengineering, Yonsei University College of Dentistry, Seoul, Republic of Korea
- 4School of Basic Medicine, Shandong First Medical University, Jinan, Shandong, China
Bioactive glasses (BGs) are ideal biomaterials in the field of bio-restoration due to their excellent biocompatibility. Titanium alloys are widely used as a bone graft substitute material because of their excellent corrosion resistance and mechanical properties; however, their biological inertness makes them prone to clinical failure. Surface modification of titanium alloys with bioactive glass can effectively combine the superior mechanical properties of the substrate with the biological properties of the coating material. In this review, the relevant articles published from 2013 to the present were searched in four databases, namely, Web of Science, PubMed, Embase, and Scopus, and after screening, 49 studies were included. We systematically reviewed the basic information and the study types of the included studies, which comprise in vitro experiments, animal tests, and clinical trials. In addition, we summarized the applied coating technologies, which include pulsed laser deposition (PLD), electrophoretic deposition, dip coating, and magnetron sputtering deposition. The superior biocompatibility of the materials in terms of cytotoxicity, cell activity, hemocompatibility, anti-inflammatory properties, bioactivity, and their good bioactivity in terms of osseointegration, osteogenesis, angiogenesis, and soft tissue adhesion are discussed. We also analyzed the advantages of the existing materials and the prospects for further research. Even though the current research status is not extensive enough, it is still believed that BG-coated Ti implants have great clinical application prospects.
1 Introduction
Bioactive glass (BG) can repair, restore, replace, and help regenerate tissue through the combination of tissues and materials. BG has uniform particle size and great adhesion with irregular sizes and shapes. Furthermore, its inherent biocompatibility and high strength make it an ideal biomaterial (Manam et al., 2017). After contact with biological fluids, some ions are released on the surface of the BG particles, which can regulate the osmotic pressure and pH value around the implant, thereby damaging the cell wall structure of bacteria and inducing antibacterial activity (Allan et al., 2002; Coraça-Huber et al., 2014; Drago et al., 2018). BGs also have appropriate biodegradability and particles are easily absorbed. In addition, this material has good bioactivity, which can promote osteoinduction and thus rapidly form bone-like structures. However, when compared to human bone tissue, BG is more brittle. Due to its poor mechanical properties, BG is not suitable for the load-bearing areas (Cannillo et al., 2009; Yanovska et al., 2011). The mentioned properties make it ideal for use in toothpaste, bone grafts, scaffolds, drug delivery systems, soft tissue engineering, and biomaterial coatings (Hench, 2006; Asif et al., 2014).
Titanium (Ti) alloys have better biocompatibility than other metal implants. Ti does not cause rejection after direct contact with bone tissue nor does it have negative effects or other bioelectronic reactions on biological organs. When compared with stainless steel and cobalt-based metals, Ti has lower modulus and better corrosion resistance while the corrosion resistance of metals and the biocompatibility of corrosion products can reflect metal’s own biocompatibility (Long and Rack, 1998). Their inertness made them virtually unreactive to the surrounding tissue environment, resulting in low cytotoxicity. The hydroxyapatite (HA) layer is often coated on the Ti alloy surface, which leads to the combination with the host collagen fiber, demonstrating Ti alloy’s osteointegration. The oxide layer on their surface is equipped with excellent corrosion resistance (Escalas et al., 1976). For mechanical property aspects, Young’s modulus of Ti alloy is much smaller than that of other metallic biomaterials, such as stainless steel, thereby reducing the stress shielding effect (Niinomi, 1998). Due to their excellent biocompatibility, corrosion resistance, and mechanical properties, Ti alloys can be applied as orthopedic and stomatological implants for arthroplasty and implantology. However, due to their intrinsic inertness, Ti alloys cannot form a close connection at the interface between the implant and host tissue. Meanwhile, the low wear resistance causes implants to loosen (Long and Rack, 1998). The inability of Ti alloys to achieve both shear strength and ductility greatly limits their application as implant materials in joint replacement (Chen and Thouas, 2015). The physical and chemical properties of the implant surface are crucial and play an important role in the osseointegration process between the bone and the implant. Therefore, surface modification of titanium implants can greatly reduce their limitations in clinical application (Geng et al., 2021a).
The surface modification of Ti using BG can improve osteointegration and osteogenesis (Gomez-Vega et al., 2001; López et al., 2016), which combines the substrates’ excellent mechanical properties with BG coatings’ biological properties (Solai et al., 2011). In fact, HA is still a common material for titanium alloy coatings. Apatite has the same inorganic composition as bone tissue and has been widely used in the field of bone transplantation and studied as a coating material (Geng et al., 2021b). Both BG and HA have good biological properties and excellent osteoconductivity. However, BG has better osteogenesis properties than HA coating materials (Dhinasekaran et al., 2021). BG coatings lead to apatite layer formation on the surface and thus improve close integration with both human hard and soft tissues (Sanz-Herrera and Boccaccini, 2011) and help in bone growth (Moreira et al., 2018; Vuornos et al., 2019). Furthermore, the ions released by BGs in bodily fluids can stimulate angiogenesis and wound healing (Cohrs et al., 2019; Zhang et al., 2019). Multiple manufacturing technologies have been investigated to coat Ti alloys with BG, such as electrophoretic deposition (Estrada-Cabrera et al., 2019), electrochemical deposition (Balamurugan et al., 2009), pulsed laser deposition (PLD) (Wang et al., 2018), dip coating deposition (Safaee et al., 2021), magnetron sputtering (Berbecaru et al., 2010), thermal spraying (Herman et al., 2000), and laser cladding (Comesana et al., 2010). Doping different ions in BG can improve the specific properties of the coating, such as magnesium ions (Mg2+), zinc ions (Zn2+), and strontium ions (Sr2+). Many recent studies have shown that Sr2+ can promote bone formation and inhibit osteoclast absorption; therefore, this element is often doped into implants or their coatings to improve the osteogenic performance of the implants (Geng et al., 2021a; Geng et al., 2022). Besides, BG coatings, when combined with other biomaterials, can improve biological properties. Drug-loaded chitosan BG coatings exhibit good cellular activity, antimicrobial capacity, and osteogenic activity (Patel et al., 2012).
In a previously published review relevant to BG coatings, Oliver et al. (2019) summarized the performance improvement of BG coatings on medical metallic implants. Maximov et al. (2021) listed different methods of BG preparation as well as coating technologies. Baino and Verné (2017) specifically discussed the different clinical areas of application of BG coatings on biomedical implants. In this systematic review, the authors have searched and screened relevant articles and summarized and analyzed the characteristics of the included studies, the manufacturing technologies of BG coatings on Ti implants, and the properties of BG coatings. Previous research status that included study types is also included in this review. This systematic review aims to evaluate the properties of BG-coated Ti implants versus bare Ti implants and systematically adds up previous coating technology and relevant parameters as the influencing factors for BG coatings on Ti implants, providing a theoretical basis for future studies.
2 Materials and methods
2.1 Inclusion and exclusion criteria
The inclusion and exclusion criteria were framed based on the PICOS model. In vivo studies and clinical trials, and in vitro studies that investigated both biocompatibility and bioactivity were included in the assessment. Studies applying Ti or its alloys coated with bioactive glasses or the composite coatings on the Ti implant containing bioactive glasses were included in this review. The outcome indicators discussed in this review contain biocompatibility, bioactivity, and antibacterial properties, which are shown in Figure 1. Biocompatibility refers to 1) cytotoxicity and cell activity, 2) hemocompatibility, 3) anti-inflammatory properties, and 4) bioactivity. Bioactivity comprises 1) osteointegration, 2) osteogenesis, 3) angiogenesis, and 4) soft tissue adhesion.
Articles published in the last 10 years were included. There are no restrictions on the research type. Articles not published in English and whose full texts were unavailable were excluded.
2.2 Literature search and screening
Four databases, namely, PubMed, Embase, Scopus, and Web of Science, were searched in this study. The search strategy is shown in Table 1.
Duplicates were removed using Endnote X9.3.2. The first screening was performed by filtering the title and abstract and inclusion of studies was determined after reading the full texts. The screening was done following the inclusion and exclusion criteria and was performed by two independent authors. Any conflict was resolved by a third author.
2.3 Data extraction
Data were collected using Microsoft Excel. The extracted data included substance material and samples’ shapes and sizes, glass models and composites, experimental subjects, manufacturing methods, and special process parameters. Other included information can be seen in detail in the following contents.
The data were extracted independently by two researchers, and any problems were solved through discussion and a third author's help.
3 Characteristics of included studies
3.1 Basic information
The process of literature screening is shown in Figure 2. After screening, 49 articles were included in this systematic review. The characteristics of the included studies are shown in Table 2.
Ti-6Al-4V, which has been widely applied in orthopedic prostheses and dental implants, is the most widely applied alloy in the included studies. Pure Ti has also been investigated. Bioactive glasses 45S5, S35P5 (Massera et al., 2012), and 58S (Sepulveda et al., 2002) are the most commonly studied basic bioactive glass.
3.2 Study type
3.2.1 In vitro studies
In vitro experiments were introduced in 43 studies, and the cell types tested are listed in Table 3. Among all in vitro studies, human osteosarcoma cells, pre-osteoblast MC3T3-E1 cells, mesenchymal matrix cells, stem cells, and fibroblasts have been widely used.
Being derived from malignant bone tumors, various types of osteosarcoma cell lines were isolated due to bone tumor categories, for example, U2OS, Saos-2, and MG-63 (Matter et al., 2021). According to their properties of having a higher capacity to help matrix mineralization, being easier to culture, having a more stable phenotype (Rau et al., 2020), and having a faster proliferation rate (Abushahba et al., 2020), they were frequently used for fabricating the osteoblast models. The pre-osteoblast MC3T3-E1 cell line is generated from mouse primary osteoblast culture (Nesabi et al., 2021), which displays similar behavior toward primary osteoblasts and, thus, shows better osteogenic differentiation (Ye et al., 2017). Mesenchymal matrix or stem cells, which are derived from a variety of tissues such as the bone marrow and adipose tissue, are multipotent adult cells (Zhang et al., 2016) and can differentiate into different cell lines. Adipose-derived mesenchymal stem cells (MSCs) are more frequently used because they are abundant and the collection process is simpler and does not cause great trauma (Chen et al., 2014), and this leads to their wide application prospects in regenerative medicine (Palangadan et al., 2014). Such MSCs can also be used to study the osteogenic differentiation potential of glass materials and to study the ability to synthesize certain specific proteins (Rau et al., 2020). Involved in granulation tissue formation, fibroblasts are connective tissue cells that synthesize collagen fibers and matrix components and are essential in the wound healing process (Avcu et al., 2018) and mediation of soft tissue integration (Patel et al., 2019). The test on fibroblasts can illustrate a material's potential application in soft tissue repair.
3.2.2 In vivo studies
For animal tests, the characteristics of the in vivo studies that were included are listed in Table 4. Many included studies have chosen New Zealand rabbits, and the included surgical sites were the femur, rabbit tibia, and mandible for dogs or pigs. Rabbits are the most commonly used animals due to their size and growth speed. Less soft tissue is found around rabbits' tibia, which is easy to operate on, while the femur has sufficient bone marrow cavity and is, thus, suitable for studying internal fixation of fractures (Guimarães et al., 2020). However, the small size of the bone has led to a reduction in the number and size of implants and, consequently, their style types are also reduced. Pig mandibles have a similar regeneration rate, morphology, and masticatory mechanics to that of humans, and human-sized dental implants are allowed (Zarghami et al., 2020). Even though mini pigs can overcome the problem of being overweight, they are very aggressive and difficult to tame. Dog mandibles are commonly used in dental implant models for assessing bone regeneration around implants (Zarghami et al., 2021). We can use human-sized dental implants in larger dogs that can actively cooperate with rehabilitation treatment programs (Guimarães et al., 2020). However, ethical issues deserve further discussion due to the harm of medical experiments to dogs.
3.2.3 Clinical studies
For clinical trials, BG-coated implants are mainly applied in orthopedics and stomatology, and the relative information is shown in Table 5. To date, clinical trials have revealed the effectiveness of BG-coated implants in total hip arthroplasty and dental implantation. In the 10-year retrospective studies carried out by Orita et al. (2022) through clinical evaluation and radiographic assessment after hip arthroplasty surgery, BG-coated implants were proven to have a better survival rate and wear resistance. In the prospective studies by Mistry et al. (2016), by comparing the osteogenesis around dental implants, the BG-coated dental implants contributed to new bone generation.
4 Coating manufacturing technology for BGs on Ti implant surface
4.1 Substrate pretreatment
Substrate pretreatment plays an important role in bio-interaction (Pattanaik et al., 2012), which can improve corrosion resistance (Kim et al., 1996) and osteointegration (Buser et al., 2012; Fischer and Stenberg, 2012). Surface roughness can also be increased as well as the adhesion between substrate and coating. Sandblasting is a simple, low-cost method (Zhou et al., 2016). Aluminum oxide and silicon carbon can be injected onto the substrate using high-speed compressed air, which improves the surface roughness. Sandblasting combined with acid etching forms a microporous structure and removes the residual abrasive particles (Pattanaik et al., 2012). Chemical pretreatments are also performed. Alkali and heat-treated implants, which apply hydroxide at high temperatures, form a titanate layer on the Ti surface. This improves the connection between the bone tissue and the implant and enables higher implant stability (Nishiguchi et al., 2003). The porous nanostructures can also increase the bond strength between coatings and substrates (Nesabi et al., 2021). Surface topology is an important surface structure that can effectively regulate the behavior of cells. Numerous studies have shown that rough or micro-/nano-sized topological structures can effectively improve cell behavior, thereby enhancing the integration ability between implants and bone interfaces (Geng et al., 2021a). Micro-arc oxidation can generate a uniform, rough, and porous oxide layer, which contributes to a tighter connection between substrate and coatings (Ma et al., 2016). Among the different coating technologies, polishing and sandblasting the titanium substrate to increase its surface roughness, followed by cleaning the substrate with distilled water, acetone, or ethanol, are the more common pretreatment procedures.
4.2 Pulsed laser deposition
PLD is performed under confined conditions. The schematic diagram is shown in Figure 3A. The pulsed molecular laser source can be used to sinter bioactive glass. In a physical coating preparation process, the precise ratio of the coating components can be guaranteed, and the prepared coatings are more uniformly attached. To ensure uniform adhesion and prevent laser single-point substrate surface corrosion, the target is usually coated in a rotary manner (Ma et al., 2016; Wang et al., 2018; Wang et al., 2020).
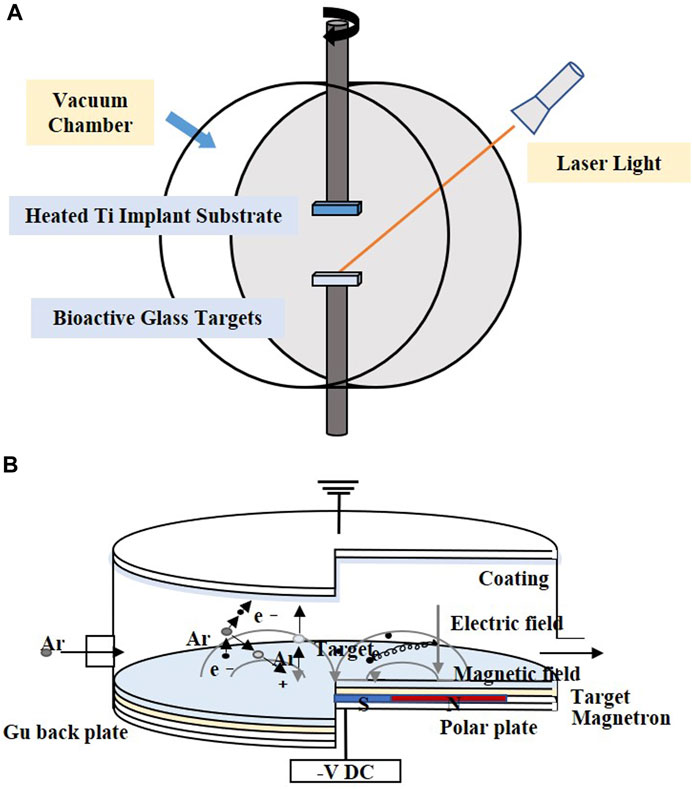
FIGURE 3. Coating manufacturing technologies of (A) PLD and (B) magnetron sputtering. The figures were redesigned based on other studies (Torrisi et al., 2007; Calderon Velasco et al., 2016).
Table 6 shows the process parameters of the included studies. In the included studies, PLD was usually performed under an environmental temperature of 200°C–800°C. The substrate temperature mainly regulates crystal composition and physiochemical and biological properties by changing the alignments of the target material on the surface and the bonding level of the coating (Figure 4) (Serra et al., 2004; Wang et al., 2020). When the substrate temperature reaches 200°C, the BG coating gains the best mechanical properties and surface appearance and can best bond with the substrates (Serra et al., 2004; Zhao et al., 2008). However, another study has proven that coatings formed under 700°C have the best biological properties (Dhinasekaran et al., 2021). To reduce the overlap between the laser and vapor that it generates, the angle of incidence of the laser projection on the substrate is maintained at 45° (Shaikh et al., 2019).
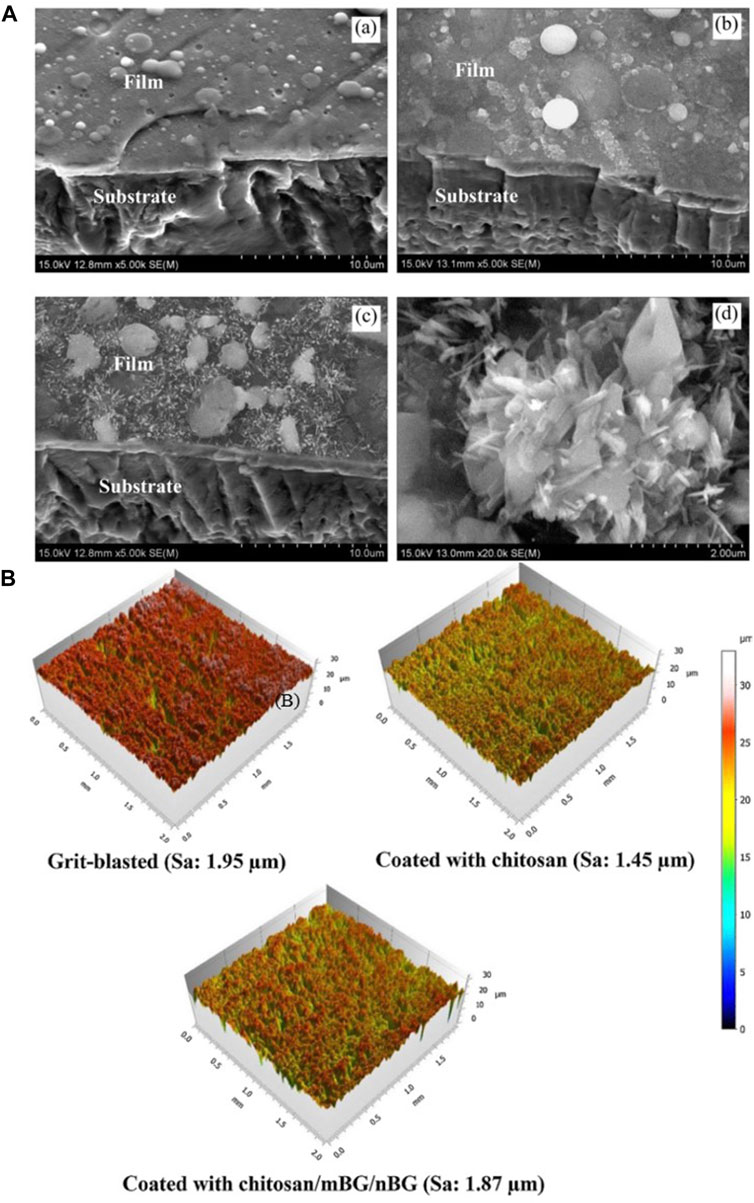
FIGURE 4. (A) SEM morphologies of films under different PLD process parameters: (a) 600°C; (b) 800°C; (c,d) 600°C + 800°C. (B) 3D surface topographies of the substrate and coatings. The figures were obtained with permission from Avcu et al. (2018) and Wang et al. (2020).
Coatings formed by PLD have a dense surface, a better chemical composition ratio of BG (Ledda et al., 2016), and a microsphere (Palangadan et al., 2014). The nanostructure on the surface can help in implant osteointegration (Rau et al., 2020). PLD mixed with micro-arc oxidation enables the porous morphology of coatings, which results in a better surface appearance and structure (Ma et al., 2016).
4.3 Magnetron sputtering
Magnetron sputtering is usually performed under low-pressure conditions, where the deposition chamber is filled with gas, which usually comprises argon (Ar) (Figure 3B). Ar atoms are ionized to produce Ar+ and new electrons, with the electronic field accelerating the electrons that bombard the targets and sputter the ions from the target atoms. After deposition, the substrate is heated to optimize the coatings (Shi et al., 2008).
The distance between the substrate and target may influence the deposition rate. A closer distance means a higher deposition rate. However, the increased momentum of the charged particles leads to an increase in the substrate temperature (Maximov et al., 2021). The acceleration voltage, heat treatment time, vacuum pressure, and filling gas also have an impact on the process (Wolke et al., 2005; Popa et al., 2017).
Magnetron sputtering makes the thickness of the coating uniform and adjustable and results in higher adhesion and purity of the coating (Shi et al., 2008), which makes it suitable for covering large areas of the substrate, and the technology is easily scalable to the industrial level under alternating current conditions (Popa et al., 2015).
4.4 Dip coating
Dip coating technology is usually combined with sol–gel, which is a process of preparing bioactive glass (Figure 5A). The glass precursor is obtained from a solution of metal alkoxides and nitrates in ethanol, which is subjected to sufficient hydrolysis and condensation reactions by stirring (Catauro et al., 2016), and the substrates are then immersed in gel. In other cases, the prepared glass is mixed into the solution and infiltrates the substrates. After dipping, drying and calcination are performed to remove excess material and stabilize the coating.
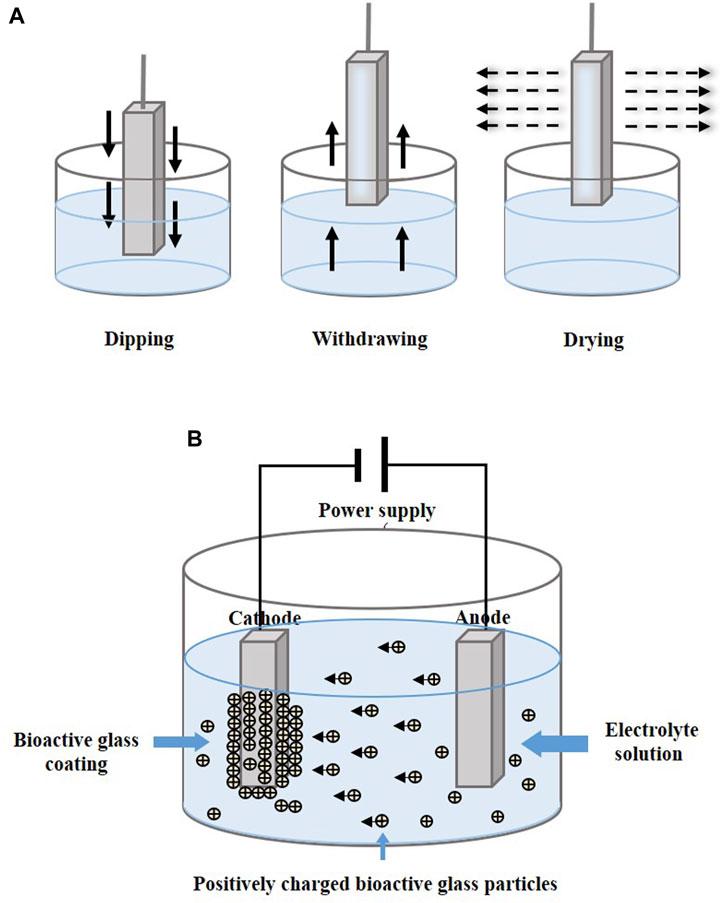
FIGURE 5. Coating manufacturing technologies of (A) dip coating and (B) EPD. The figures were redesigned based on other studies (Bohner and Lemaitre, 2009; Boccaccini et al., 2010; Bakhshandeh and Amin Yavari, 2018; Sultana et al., 2021).
Various conditions such as the extraction speed, dipping times, viscosity of sol, and process of drying influence the coating formation (Brown, 1989). The extraction speed is directly related to the coating thickness. The slower the speed, the thinner the coating and the more the original shape of the substrate can be maintained.
When compared with others, dip coating can be performed under lower temperatures, and the process is cheaper and easier. The coatings are more uniform and have higher purity, which stabilizes the substrate's shape (Fu et al., 2011; Catauro et al., 2016; Fu et al., 2017a). Other components, such as nanoparticles, mesoporous agents, and antimicrobial agents added to the solution, may lead to complications in the coating structure and composition (Tian et al., 2016; Rivadeneira and Gorustovich, 2017).
4.5 Electrophoretic deposition
Electrophoretic deposition (EPD) forms a coating by applying a direct current or alternating current electric field between two electrodes, causing charged particles to be dispersed in suspension and move in the direction of the substrate electrode (Figure 5B). The particles are deposited in an orderly manner on the substrate (Wang et al., 2014; Sultana et al., 2021). Heat treatment at 800°C–900°C makes the coatings denser and more stable (Ananth et al., 2013; Khanmohammadi et al., 2020).
Table 7 shows the parameters in the process of EPD in the included studies. The factors affecting the properties of coatings prepared via EPD can be concluded as solutions’ stability, conductivity (Zhitomirsky et al., 2009), and BG powder concentration. The distance between the electrodes, applied electric field voltage, deposition time, temperature, and pH all influence the deposited coatings. Changes in the BG concentration in the solution may increase the electrophoretic mobility, and adjustments made in the deposition parameters can make the coatings denser and more uniform (Jugowiec et al., 2017).
4.6 Hydrothermal deposition
Hydrothermal deposition is a multiphase reaction of dissolution and recrystallization of materials under high temperature and pressure with an aqueous solution as the reaction system in a closed autoclave, leading to the formation of precipitates (Pore et al., 2021). This method can be used for the preparation of powders and as a coating technique for materials.
The process essentially involves attaching thermocouples and pressure sensors to the reactor assembly, setting the parameters, and heating the reactor. Subsequently, pressure builds up and the coating is deposited on the sample in a supercritical environment (Ali et al., 2018).
The method is simple and inexpensive and can be used to synthesize coatings with uniform thickness, orientation, and shape directly in an aqueous solution (Valanezhad et al., 2015). The coating structure can be controlled by changing the synthesis parameters. However, with this method, it is not easy to control the crystal structure formed, and due to the environmental requirements of high temperature and high pressure, it is more dependent on the experimental equipment.
5 Biocompatibility and antibacterial properties
5.1 Cytotoxicity and cell activity
Cytotoxicity is the killing of cells by chemicals without involving the cellular mechanisms of apoptosis or necrosis. After implantology, ions that reach the cytotoxic concentration leach out of the glass coating and interact with the cells (Al-Noaman et al., 2012). As an important indicator to assess the safety of biological materials, cytotoxicity is assessed by in vitro studies that simulate the survival environment of cells. A lower cell survival rate indicates higher cytotoxicity, indicating clinical risk (Wataha et al., 1994). Cell viability above 70% is usually considered non-cytotoxic (Wei and Ding, 2017). The release of large amounts of alkaline ions can have adverse effects on living cells. The increase in pH at the implantation site is accompanied by the dissolution of bioactive glass, which increases by-products and ultimately leads to toxic effects on the surrounding tissues (Jones, 2013). The adverse effect causes tissue reactions such as inflammation, necrosis, induction of immunity, and carcinogenesis (Costa, 1991).
Cytotoxicity is influenced by the substance and doped content in the glass coating. BG doped with silver ions, cobalt oxide, and titanium dioxide has shown that the doping of cobalt oxide causes higher cytotoxicity than that of silver and titanium dioxide (Lung et al., 2021). This is mainly because cobalt ions induce oxidative stress and activate intracellular nicotinamide adenine dinucleotide phosphate (NADPH) oxidase to produce ROS, which causes oxidative damage to cells (Chattopadhyay et al., 2015) and affects cell morphology and viability (Fleury et al., 2006). A high level of silver in the coating increases the level of released nitrate and improves its cytotoxicity (Catauro et al., 2015).
Cell activity refers to the ability of cells to maintain or resume normal physiological activities, such as cell adhesion, proliferation, migration, differentiation, and metabolism (Patel et al., 2019), which is affected by environmental factors, such as cell culture parameters, attached drugs, and growth factors. Cell adhesion refers to the cellular ability to contact and bind to adjacent cells or the extracellular matrix (ECM) (Humphries et al., 2009). Cell spreading is the behavior of cells on the surface of a biomaterial, which is influenced by certain protein molecules (Cuvelier et al., 2007). Cell proliferation is the process of cell division by DNA replication and other reactions under the action of cycle regulators, resulting in an increase in cell numbers, which is the basis for normal tissue development and maintenance (Xynos et al., 2001). Cell migration is the movement of cells after receiving a certain signal or concentration gradient of a substance and is essential for proper immune response and wound repair (Trepat et al., 2012). The morphological and functional changes that occur because of the selective expression of cellular genes are defined as cell differentiation (Ponzetti and Rucci, 2021).
BG 45S5 is generally regarded as the gold standard for bioactive glass, and its ionic lysate induces adhesion and proliferation of cells (Abushahba et al., 2020). Calcium silicate-based materials release calcium and silicate ions, which induce osteoblast proliferation by gene activation (Catauro et al., 2016). Uniform coatings give the best cell metabolic, whereas inhomogeneous coatings, where some cells are in direct contact with the Ti matrix, are less biocompatible (Catauro et al., 2016). However, studies have shown that, when compared with HA coatings, BG-coated samples lead to the rupture and contraction of cells (Dhinasekaran et al., 2021). The surface roughness and profile of the coatings influence cell adhesion and proliferation (Gaweda et al., 2018). Fluorescence staining was performed on cells to observe the effect of BG coatings on the distribution of cytoskeleton, a typical sub-apical localization of the cytoskeleton around the cell membrane can be found. This phenomenon confirms the adhesion and proliferation ability of the cells and demonstrates that BG coatings contribute to the differentiation of the Caco-2 cell line [Figure 6A(a,b)] (Ledda et al., 2015). Doping zinc oxide into BG 45S5 stimulates osteoblasts proliferation and, thus, improves the combination between the implants and bone tissue (Ishikawa et al., 2002; Oki et al., 2004). BG-coated implants doped with Ag2O increase cell viability. A large number of cells can be observed on the surface with Ag2O in 0.008 % mol/mol, which may have resulted from the release of nitrate ions (Catauro et al., 2015). On the surface of composite coatings comprising chitosan and BG, more cell adhesion, a higher rate of proliferation, and an extended and expanded cytoskeleton can be observed (Patel et al., 2019; Zarghami et al., 2021). On the surface of yttria-stabilized zirconia (YSZ)-BG composite coatings, a large coverage of osteoblasts can be observed, with many filamentous adhesions between the cells and visible nodule formations, which is an early feature of cell differentiation [Figure 6A(c,d)]. However, increasing the relative content of YSZ in the coating decreases the cell activity, as more yttria Y3+ ions are released (Ananth et al., 2013).
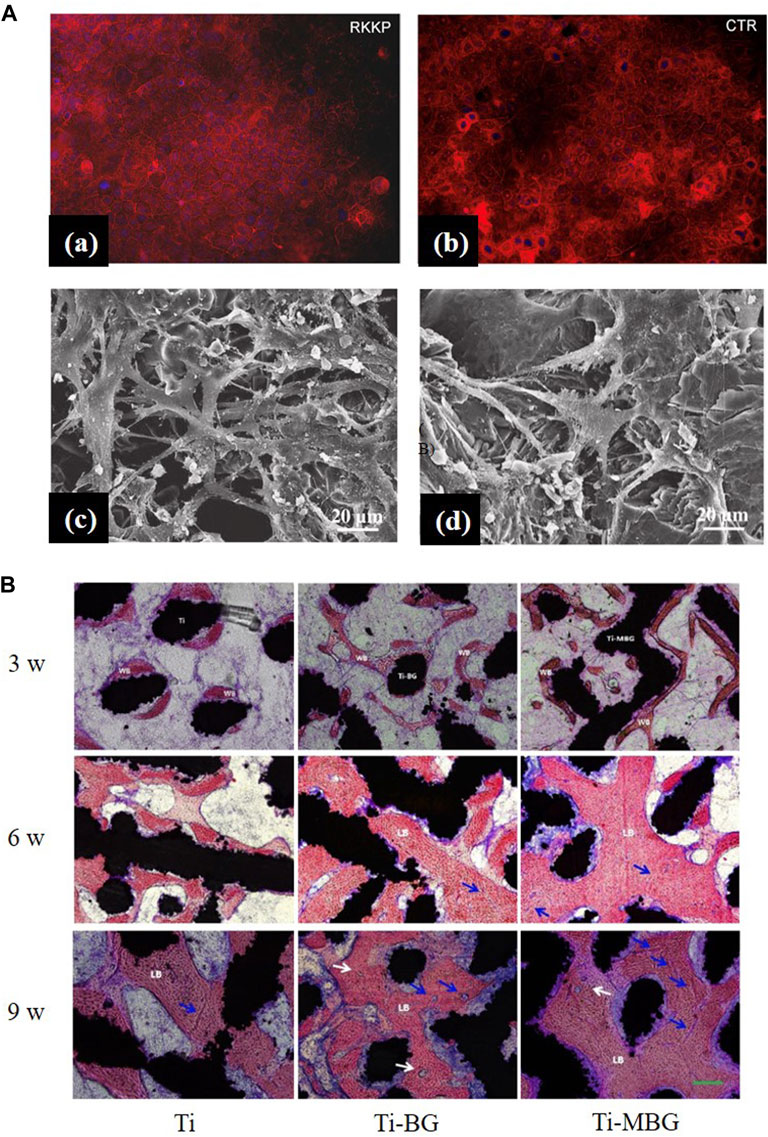
FIGURE 6. (A), (a,b) Actin distribution in the Caco-2 cell line: cells seeded on the RKKP film surfaces and plastic Petri dishes (CTR: critical temperature resister, a semiconductor ceramic material). (c, d) Morphological aspects of MG-63 osteoblast cells cultured on YSZ-BG-coated Ti6Al4V. (c) Cells on 1YSZ-2BG are similar, but the osteoblasts are well spread and present cytoplasmic extensions forming a continuous surface layer. (d) Cell morphology on 2YSZ-2BG coating is similar to (c), but filopodial extensions are fewer. The figures were obtained with permission from Ananth et al. (2013) and Ledda et al. (2015). (B) Representative histological images of non-decalcified sections are obtained by methylene blue acid fuchsin staining. Blue arrows, neovascularization; white arrows, the Haversian system; WB, woven bone; LB, lamellar bone. The figures were obtained with permission from Zhang et al. (2021).
In in vivo experiments, after the formation of HA on the surface, five stages of biological events occurred: 1) growth factors adsorption, 2) bone progenitor cells adhesion, 3) proliferation, 4) differentiation, and 5) production of the extracellular matrix, which enhances bone healing (Popa et al., 2015). The surface roughness contributes to a larger cell attachment area. It has been observed that cell density and bone healing in implants with rough surfaces are significantly better than in those with smooth surfaces (Klyui et al., 2021). Dissolved ions such as Ca, Mg, and Si can activate the expression of bone-related genes by regulating bone-related cell growth and metabolism (Taguchi et al., 2019; Zhang et al., 2021). The wettability and hydrophilicity of the surface also promote cell proliferation (Soares et al., 2018).
5.2 Hemocompatibility analysis
Hemocompatibility is the ability of blood to tolerate a material without causing significant adverse blood reactions when the material is in contact with blood (Nalezinkova, 2020). The main adverse blood reactions involve thrombosis. The absorbance of blood proteins on the surface of the materials triggers a series of cascade reactions, resulting in thrombosis, and the coagulation cascade spreads rapidly, leading to death in severe cases (Manivasagam et al., 2021). Hemolysis, which reflects hemocompatibility, is caused by adverse reactions to any toxic substance that comes in contact with blood (Dhinasekaran et al., 2021). The percentage of hemolysis (hemolysis%) is calculated on the basis of the following formula:
If the hemolysis% of a sample is <2%, it is non-hemolytic; a hemolysis% between 2% and 5% indicates that it is slightly hemolytic; if the hemolysis% >5%, it is considered hemolytic. Materials that are blood compatible are considered to have a hemolysis% less than 5%.
Studies have demonstrated that the hemolysis% of BG powders in all concentrations is lower than that of HA at the same concentrations, while more red blood cells (RBCs) are ruptured with BG coating. This may be due to the release of sodium ions from the BG coating, causing RBCs to rupture and, thus, exhibit hematotoxicity, which is corrected by washing after coating (Durgalakshmi et al., 2020; Dhinasekaran et al., 2021). Bargavi et al. (2022) found that BG coatings doped with various concentrations of alumina (Al) exhibited non-hemolytic properties and improved hemocompatibility when compared to pure BG coatings, where BG coatings doped with 10% Al had the best hemocompatibility. The hemocompatibility of BG coatings doped with zirconia (Zr) has also been investigated. With an increase in Zr concentration, the hemolysis% of the coating slightly decreased; while BG coatings doped with 5% and 10% Zr showed non-hemolysis, BG coatings doped with 15% Zr showed slight hemolysis (hemolysis % <2.5%) (Bargavi et al., 2020). Generally, BG-coated implants show great hemocompatibility.
5.3 Anti-inflammatory properties
Inflammation is an immune response of the body to resist harmful irritation, which helps maintain tissue homeostasis during injury or infection (Medzhitov, 2010; Wu et al., 2019; Chang and Xiong, 2020); however, excessive inflammatory responses form fibrous capsules that prevent implants' osteointegration. Therefore, superior anti-inflammatory property is critical for implant success.
There is no significant difference in the expression of anti-inflammatory factors in human amniotic mesenchymal stromal cells (hAMSCs) on RKKP glass-ceramic coating when compared with the control group, which indicates that the coating did not affect the expression of hAMSCs' anti-inflammatory factors (Ledda et al., 2016). Wu et al. (2014) found that bioactive Sr2MgSi2O7 (SMS) ceramic coatings exhibited superior anti-inflammatory effects compared to HAp coatings, and their mechanism of inhibiting the inflammatory response may be due to the 1) inhibition of the Wnt5A/Ca2+ pathway, which enhances the inflammatory response by decreasing the Ca2+ concentration or 2) inhibition of inflammatory cytokine expressions by the Toll-like receptor (TLR) pathway, which induces an immune response by the release of Mg2+ and Sr2+ (Figures 7A a–c).
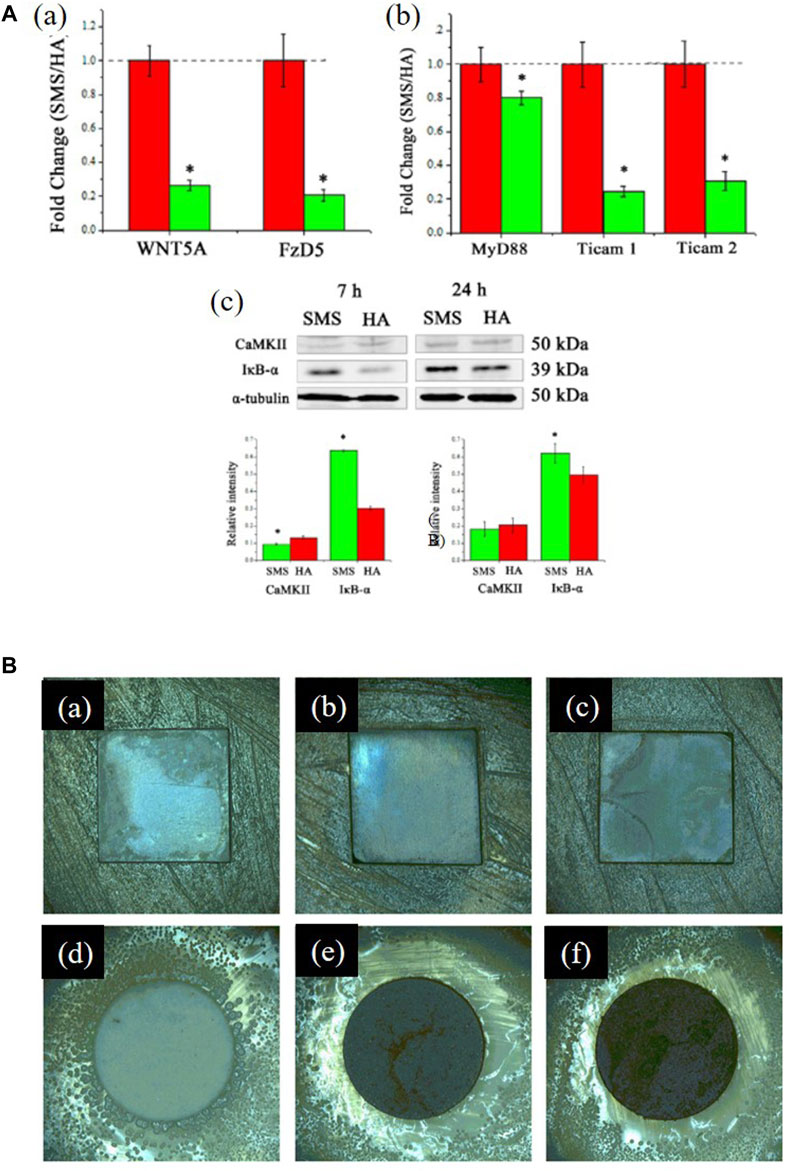
FIGURE 7. (A), (a) Fold changes of WNT5A/Ca2+ pathway-related genes: WNT5A and Fz5. (b) Fold changes of Toll-like pathway-related genes: MyD88, TICAM1, and TICAM2. (c) Western blotting analysis of CaMKII and IκB-α expression. *significant difference by comparing RAW 264.7 cells cultured in SMS coating with HA (p < 0.05). The figures were obtained with permission from Wu et al. (2014). (B) Typical optical images of antibacterial test results of the coated samples against Staphylococcus aureus: (a) 0 Ag, (b) 5 Ag, (c) 10 Ag, and (d–f) their corresponding glass wafer samples: 0 Ag, 5 Ag, and 10 Ag: Ag/Ca atomic ratios of 0%, 5%, and 10%, respectively. The figures were obtained with permission from Fu et al. (2017).
For in vivo experiments, the inflammatory response of the host to the implant is a normal bodily reaction, often manifested as a local inflammatory response and vascular congestion, which disappears after some time (Taguchi et al., 2019). The gingival index determines the inflammatory status by observing the gingival condition, while the periodontal pocket is a manifestation of the pathological inflammatory response (Löe, 1967; Donos, 2018). In clinical trials, in follow-up survey statistics, the gingival index and depth of periodontal pockets were smaller in BG-coated groups, which showed a higher success rate of implantology (Mistry et al., 2016).
5.4 Bioactivity properties
5.4.1 Osteointegration
Osteointegration, also known as osseointegration, mainly describes the level of direct connection between an artificial implant and bone tissue without an intermediate fibrous connective tissue layer (Brånemark et al., 1977; Goriainov et al., 2014). A good interface between the implant and bone is an important factor in the formation of dense new bone and, thus, for the osseointegration of both (Agarwal et al., 2015; Hu et al., 2019; Sang et al., 2022). The wettability and surface energy of a material can change the binding of implants to osteoblasts after implantation (Chen et al., 2011).
In animal experiments, BG coating improved implant wettability and enhanced cell viability in the early stages of bone healing, thus significantly increasing bone-to-implant contact (BIC) and bone mineral density (BMD) (Soares et al., 2018), with new bones being formed around the implant and closely combined with the bone tissue (Taguchi et al., 2019). Strontium-substituted bioactive glass (SrBG) coating can stimulate bone formation by releasing dissolved products, showing a superior bone fixation effect (Newman et al., 2014). Reparative osteogenesis formed around the BG/HA/TCP composite coating implants (Klyui et al., 2021), and a perfect fusion with the bone tissue could be observed around the HA/BG/wollastonite (WS) composite coating implant (Wang et al., 2020). However, some studies have also found that in HA/BG coatings, when the concentration of BG is increased, a faster dissolution rate of BG leads to new bone damage and limits the combination of implants and bone tissue (Wang et al., 2018). Only BG containing a certain weight percentage, that is, 40–60wt% SiO2, can promote osteogenesis (van Oirschot et al., 2014).
In clinical trials, good bone ingrowth can be found near the implants in BG-coated hip implants (Orita et al., 2022). The bone regeneration around the oral implants is better, with osteoid formation and increased mineralization, which is specifically reflected in the higher median interface density (MID), discrete interface density (DID), and interface radiodensity (IFD) observed at 6 months (Mistry et al., 2016).
5.4.2 Osteogenesis
Osteogenesis refers to bone tissue formation, which is a complex procedure of osteo-development. Bone matrix mineralization and secretion are eternal procedures controlled by osteoblasts (Popa et al., 2015). Based on the included studies, osteogenesis mainly reflects on apatite formation in simulated body fluid (SBF), positive osteoblasts response, and rapid increase in new bone formation and mineralization in vivo.
5.4.2.1 Apatite formation in SBF
BG and doped ions in the coating significantly affect apatite formation in SBF. Phosphate in BG can promote apatite formation in SBF (Li et al., 2021). Mesoporous bioactive glass (MBG) coatings with an ordered mesoporous structure exhibit more evident apatite deposition than BG coatings (Zhang et al., 2016).
Ananth et al. (2013) observed more calcium phosphate particle deposition by increasing the relative content of BG in YSZ-BG composite coatings, which may be due to the promotion of apatite nucleation by the Si–OH group in the BG coating (Figure 8A). Increasing the BG content in chitosan/BG composite coatings also enhances the osteo-biological activity of the coating (Avcu et al., 2018). A high SiO2 content reduces the dissolution rate of BG and influences surface apatite formation, which indicates that the formation of surface apatite can be improved by reducing the content of SiO2 and doping an appropriate amount of Ag and Co (Lung et al., 2021). However, Ag+ is smaller in size than Ca2+ and binds more firmly with unbridged oxygen, so high Ag content is not conducive to the formation of HA (Catauro et al., 2015). The BG-Al composite coating was created using the sol–gel method, and with an increase in Al concentration, the growth rate of apatite accelerated (Bargavi et al., 2022).
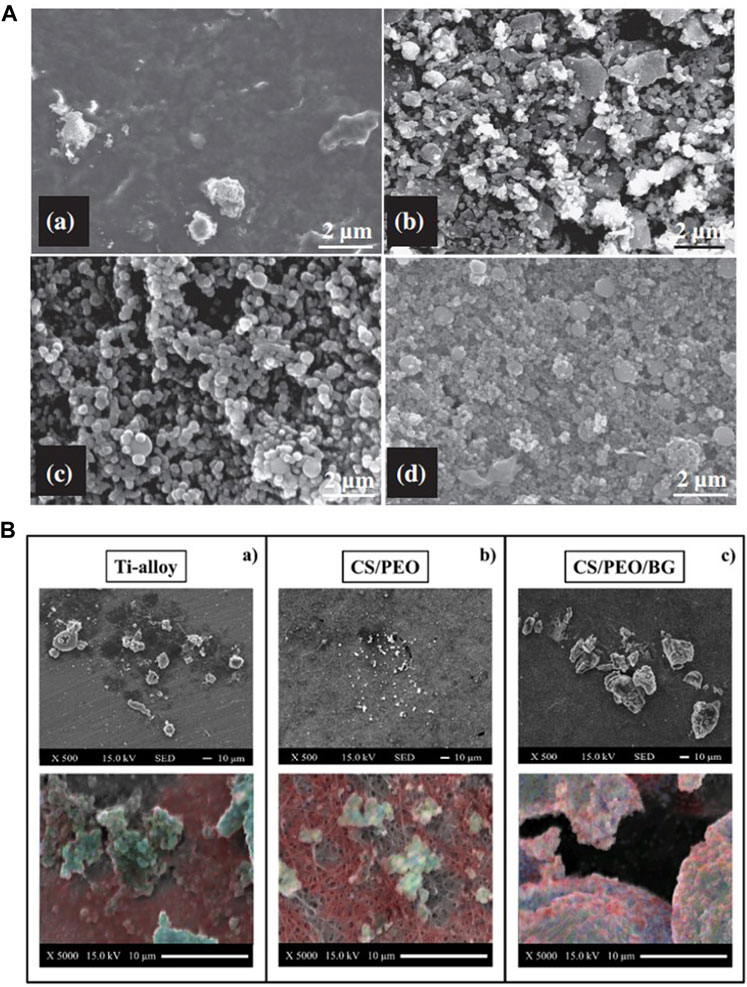
FIGURE 8. (A) SEM images of the 1YSZ-2BG–coated Ti6Al4V alloy, which was immersed in SBF for various time durations (days): (a) 3, (b) 7, (c) 14, and (d) 21. (B) SEM images of bone formation on the three substrates and EDX elemental maps labeled calcium (blue), carbon (red), and phosphorous (blue) to identify the presence of the mineral. The figures were obtained with permission from Ananth et al. (2013) and Boschetto et al. (2020).
5.4.2.2 Osteocyte experiments
A material's influence on cellular behavior is essential in osteogenesis. The connection between the bone in vivo has two steps: first, the generation of a carbonate HA layer on the glass surface, to which the osteogenesis-related cells will subsequently respond. The dissolution products of BG modulate the proliferation and differentiation of cells to accelerate the integration of bone (Crush et al., 2021). In vitro cellular experiments reveal the production of intracellular osteogenic markers and assess the osteogenic activity of BG. The maturation of osteoblasts is categorized into proliferation, differentiation, and mineralization (Owen et al., 1990). In the proliferative stage, cells express genes that regulate the cell cycle and growth and form the ECM. Alkaline phosphatase (ALP), which is expressed in the initial differentiation stage, and osteopontin (OPN), which is expressed in the initial mineralization stage, induce matrix maturation and mineralization, and their increased expressions in osteoblast markers promote mineral deposition (Saino et al., 2010).
Ions released by BG dissolution can stimulate gene expression (Hench et al., 2020), which indicates that glass components have a significant impact on cells’ proliferation and differentiation. Si and Ca groups play a more important role in osteo-associated cells’ differentiation and proliferation than P and Na groups (Su et al., 2019). Cell inoculation on BG-coated surface significantly increased the content of mineralized matrix deposition (Figure 8B) (Boschetto et al., 2020), markers of early differentiation such as ALP and RUNX2, and expression of osteocalcin (OCL), which is expressed in late differentiation (Ledda et al., 2016). It has also been shown that the mesoporous physical structure of glass promotes the effective release of Ca and Si ions, leading to a higher level of cell differentiation (Zhang et al., 2016; Ye et al., 2017). When compared with uncoated Ti and BG-coated implants, more collagens were released in osteoblasts co-cultured with zirconia-containing BG-coated implants because the BG-Zr composite mediates collagen synthesis by changing the pH through an ion-release mechanism (Bargavi et al., 2020). Meanwhile, a higher pH also favors bone formation processes, which include the cross-linking of collagen chains and subsequent deposition of HA (Wang et al., 2011; Bargavi et al., 2020).
5.4.2.3 In vivo trials
Osteogenesis in clinical trials contributes to the complete restoration and biomechanical properties of natural bone (Santos et al., 2009). Mechanical stabilization and appropriate host response are essential to ensure osteogenesis (Lavenus et al., 2015).
The intrinsic mechanism of osteogenesis is inextricably linked to the ion release process of the BG coating. The silica-rich layer on BG coatings leaches into the local tissue fluid, regulating the osteoblast cycle and allowing rapid osteogenesis and mineralization, which increases peri-implant osteogenesis and mineralization (Xynos et al., 2000; Hench and Greenspan, 2013). Ion dissolution products containing Ca, Mg, and Si bioactive glasses activate the expression of bone-related genes such as bone morphogenetic proteins (BMPs) and vascular endothelial growth factor (VEGF), stimulating osteogenesis and angiogenesis (Huang et al., 2009; Sun et al., 2009; Gu et al., 2011; Hoppe et al., 2011; Saffarian Tousi et al., 2013; Zhai et al., 2013; Henstock et al., 2015; Shamsi et al., 2017; Naseri et al., 2018; O’Neill et al., 2018; Zafar et al., 2019; Zhou et al., 2019).
In animal tests, BG-coated implants show active areas on the surface that serve as the origin of osteogenesis, forming bones with better morphology, maturity, quantity, and thickness than in control groups, and with more rapid and effective osteogenesis (Figure 6B) (Taguchi et al., 2019; Zhang et al., 2021). It has also been shown that a more mature new bone was observed around BG-coated implants in the early stages of bone healing, but as the healing time increased, the advantage of BG-coated implants was no longer evident, since surface roughness affects the adhesion, migration, and differentiation of osteoblasts and thereby the osteogenesis process (Soares et al., 2018).
Poly (co-glycolide propionate)/BG (PBG) nanocomposite coatings induce more than 85% in bone formations. On HA/BG/WS composite coatings, the formation of surface bone tissue has been observed (Wang et al., 2020), while BG composite coatings with 30 wt% HA (pure) and 20 wt% TCP (tricalcium phosphate) show a larger area of bone formation around the implant (Klyui et al., 2021). Furthermore, the peri-implant osteogenic capacity may be related to the implant site and animal species, and the bone quality and quantity at the implant site may interfere with possible significant differences between experimental groups (van Oirschot et al., 2014).
5.4.2.4 Clinical trials
In clinical trials, BG-coated groups show a higher success potential: the least marginal bone loss (MBL), the highest IFD in both low- and high-density bone tissue, and a better deposition and mineralization of new bone tissue around the implant (Mistry et al., 2016). Furthermore, BG-coated hip implants have better bone growth conditions with excellent survival rates and wear resistance (Orita et al., 2022).
5.4.3 Angiogenesis
Angiogenesis, also known as vascular regeneration, is the sprouting and remodeling of neovascularization in the original vascular network (Huang and Nan, 2019), which is essential for tissue repair after implantation (Mehdikhani-Nahrkhalaji et al., 2015; Nowak-Sliwinska et al., 2018; Klyui et al., 2021). The formation of vessels helps transport oxygen, nutrients, minerals, and osteoprogenitor cells over long distances, contributing to bone healing (Zhang et al., 2021). Cerium oxide and BG doped with 2% Zn nanocomposite coatings exhibit superior pro-vascular regenerative capacity and the mechanism is related to the regulation of the hypoxic response and structural reorganization of cells (Matter et al., 2021). Increasing the porosity of BG-coated porous scaffold, reducing the thickness of the coating, and improving the surface roughness all provide space for new bone formation, contributing to angiogenesis (Zhang et al., 2021).
5.4.4 Soft tissue adhesion
After implantation, soft tissue reaction leads to the formation of a fibrous capsule, which contacts the implant without adhesion, allowing relative movement between the implant and the surrounding tissue. Appropriate soft tissue adhesion holds the implant stably in the surrounding tissue (Lee et al., 2010), which limits hematoma and abscess formation and prevents infection (Lee et al., 2010; Zigterman et al., 2019).
Surface roughness, coating composition, and structural design of the implants are the main factors affecting soft tissue adhesion (Lee et al., 2010; Zigterman et al., 2019). The ceria and BG doped with 2% Zn nanocomposite coatings reduce the biomineralization behavior to adapt to soft tissue while inducing the generation of vascular endothelial cells without cytotoxicity to gingival fibroblasts. It can promote rapid wound healing and exhibit superior soft tissue regeneration abilities in subsequent scratch assays (Matter et al., 2021). During oral implantology, the surrounding blood clot adheres firmly to BG-coated implants, indicating that BG-coated implants have higher wettability and stronger adhesion to the surrounding soft tissue than machined bare titanium implants (Mistry et al., 2016).
5.5 Antibacterial properties
Antibacterial properties are an essential characteristic in grafts implanted clinically, which refers to the grafts’ ability to reduce microbial growth on their surfaces. The formation of a biofilm is the first stage of bacterial growth, which also inhibits the proliferation of osteoblasts.
Better antibacterial properties are reflected in larger bacterial inhibition zones, lower reduction rates, fewer colony-forming units, and lower minimum inhibitory concentrations. A study has shown that as the content of silver ions in the coating increased, the number of bacteria on the sample surface decreased significantly and the bacterial inhibition area on the surface became larger [Figures 7B(a–f)] (Fu et al., 2017b). The cobalt- and Ti-doped glass coatings have better antibacterial properties than traditional 58S glass (Lung et al., 2021). As more zirconium oxide is added to samples, they exhibit a higher ability to inhibit bacterial growth (Bargavi et al., 2020), whereas borate-based glass exhibits worse antibacterial properties (Rau et al., 2020). Glass composited with drugs can help eradicate bacteria (Zarghami et al., 2021). By observing bacteria in saliva on the implant surface, Costa et al. (2020) found that fewer pathogenic bacteria were observed on the surface.
The antibacterial property is mainly increased by doping silver and other metallic ions in the manufacturing of chitosan composted coatings and in combination with antibiotics like tetracycline and vancomycin. The inhibition of bacteria by silver ions is mainly due to direct contact, which results in the deformation of cell membranes (Lung et al., 2021) and ROS in bacteria (Fu et al., 2017b). The electrostatic effects leading to changes in cell membrane permeability, thereby influencing cell signal transduction and production of ROS, are the main reasons for antibacterial properties caused by metallic compounds (Lung et al., 2021). Due to the slow-release behavior, BG containing chitosan and vancomycin has higher bactericidal effects (Zarghami et al., 2020). Chitosan increases cell membrane permeability, leading to the release of intracellular substances and thus causing cell death (Ordikhani et al., 2016). The composite coating of bioactive glass as a drug carrier can also significantly improve antimicrobial properties. BG has a rougher surface (Matter et al., 2021) and creates a more alkaline biological environment (Echezarreta-López and Landin, 2013; Brauer, 2015) through dissolution and ion release, where bacteria grow poorly.
In in vivo experiments, the plaque index and gingival recession were assessed to evaluate the degree of oral hygiene (Silness and Löe, 1964; Kassab and Cohen, 2003). A clinical trial proved that patients with BG-coated implants have reduced plaque index and gingival recession and, therefore, reduced occurrence of oral disease (Mistry et al., 2016).
6 Conclusion and future prospects
Bioactive glasses are widely researched because of their good biological properties; however, poor mechanical properties limit their clinical applications. Suitable coating technologies are essential for the performance of glass coatings’ relevant properties. This work systematically reviews the coating technology of BG on the surface of Ti and its alloys and summarizes the principles of the technology, relevant parameters, and their relative advantages, providing a reliable basis for coating technology selection. BG coatings exhibit excellent cell compatibility, antibacterial and anti-inflammatory properties, and higher levels of osseointegration and osteogenesis, which indicate that BG coatings on Ti and its alloys have excellent biocompatibility and bioactivity. The doping of ions and compounding with other substances significantly improve the coatings’ performances.
However, BG-coated Ti and its alloy implants face many challenges nowadays. Adding antibacterial ions, such as Ag and Co, and compositing with drugs, such as tetracycline and vancomycin, can improve the antibacterial properties of metal implants. However, the overuse of metal ions may cause cytotoxicity and limit cell metabolism, which leads to negative tissue reactions. Therefore, the balance of antibacterial properties and cytotoxicity requires further study for coating optimization. In terms of physical properties, there are significant differences in the coefficients of thermal expansion between metals and glass materials that can lead to cracking and failure of coatings. Improving the compatibility of substrates and coatings also relies on further research.
In vitro experiments can filter suitable implant biomaterials in the first stage, which prevents excessive euthanization of laboratory animals and simplifies the quantification of experimental results. However, cellular metabolites cause peripheral tissue reactions, and in vitro studies cannot test the subsequent biological effects. Animal experiments can assess the influence of coated implants on the surrounding and distant organs while controlling different pathological models and loading conditions, making them important pre-clinical research. For BG-coated implants, advanced clinical applications require more animal experiments and clinical trials.
Data availability statement
The original contributions presented in the study are included in the article/Supplementary Material; further inquiries can be directed to the corresponding authors.
Author contributions
JL: conceptualization, data curation, resources, visualization, manuscript writing–original draft, and manuscript writing–review and editing; XL: Methodology, resources, software, visualization, and manuscript writing–original draft; XZ: methodology, resources, software, visualization, and manuscript writing–original draft; YL: formal analysis, investigation, resources, and manuscript writing–original draft; XG: methodology, resources, visualization, and manuscript writing–original draft; KS: investigation, resources, visualization, and manuscript writing–original draft; HC: investigation, resources, and manuscript writing–original draft; QJ: investigation, resources, and manuscript writing–original draft; HJ: conceptualization, supervision, validation, and manuscript writing–review and editing; KL: conceptualization, supervision, and validation.
Funding
The author(s) declare financial support was received for the research, authorship, and/or publication of this article. This research was funded by [Shandong Provincial key research and development program] grant number [2019GSF108259].
Conflict of interest
The authors declare that the research was conducted in the absence of any commercial or financial relationships that could be construed as a potential conflict of interest.
Publisher’s note
All claims expressed in this article are solely those of the authors and do not necessarily represent those of their affiliated organizations, or those of the publisher, editors, and reviewers. Any product that may be evaluated in this article, or claim that may be made by its manufacturer, is not guaranteed or endorsed by the publisher.
References
Abushahba, F., Tuukkanen, J., Aalto-Setala, L., Miinalainen, I., Hupa, L., and Narhi, T. O. (2020). Effect of bioactive glass air-abrasion on the wettability and osteoblast proliferation on sandblasted and acid-etched titanium surfaces. Eur. J. Oral Sci. 128 (2), 160–169. doi:10.1111/eos.12683
Agarwal, R., Gonzalez-Garcia, C., Torstrick, B., Guldberg, R. E., Salmeron-Sanchez, M., and Garcia, A. J. (2015). Simple coating with fibronectin fragment enhances stainless steel screw osseointegration in healthy and osteoporotic rats. Biomaterials. 63 (2015) 137–145. doi:10.1016/j.biomaterials.2015.06.025
Al-Noaman, A., Rawlinson, S. C. F., and Hill, R. G. (2012). The influence of CaF2 content on the physical properties and apatite formation of bioactive glass coatings for dental implants. J. NON-CRYSTALLINE SOLIDS 358 (15), 1850–1858. doi:10.1016/j.jnoncrysol.2012.05.039
Ali, A., Iqbal, F., Ahmad, A., Ikram, F., Nawaz, A., Chaudhry, A. A., et al. (2018). Hydrothermal deposition of high strength calcium phosphate coatings on magnesium alloy for biomedical applications. Surf. Coatings Technol. 357, 716–727. doi:10.1016/j.surfcoat.2018.09.016
Allan, I., Wilson, M., and Newman, H. (2002). Particulate Bioglass® reduces the viability of bacterial biofilms formed on its surface in an in vitro model. Clin. Oral Implants Res. 13 (1), 53–58. doi:10.1034/j.1600-0501.2002.130106.x
Ananth, K. P., Suganya, S., Mangalaraj, D., Ferreira, J. M. F., and Balamurugan, A. (2013). Electrophoretic bilayer deposition of zirconia and reinforced bioglass system on Ti6Al4V for implant applications: an in vitro investigation. Mater. Sci. Eng. C-Materials Biol. Appl. 33 (7), 4160–4166. doi:10.1016/j.msec.2013.06.010
Asif, I. M., Shelton, R. M., Cooper, P. R., Addison, O., and Martin, R. A. (2014). In vitro bioactivity of titanium-doped bioglass. J. Mater. Sci. Mater. Med. 25 (8), 1865–1873. doi:10.1007/s10856-014-5230-4
Avcu, E., Avcu, Y. Y., Bastan, F. E., Rehman, M. A. U., Ustel, F., and Boccaccini, A. R. (2018). Tailoring the surface characteristics of electrophoretically deposited chitosan-based bioactive glass composite coatings on titanium implants via grit blasting. Prog. Org. Coatings 123, 362–373. doi:10.1016/j.porgcoat.2018.07.021
Baino, F., and Verné, E. (2017). Glass-based coatings on biomedical implants: A state-of-the-art review. Biomed. Glas. 3 (1), 1–17. doi:10.1515/bglass-2017-0001
Bakhshandeh, S., and Amin Yavari, S. (2018). Electrophoretic deposition: a versatile tool against biomaterial associated infections. J. Mater Chem. B 6 (8), 1128–1148. doi:10.1039/c7tb02445b
Balamurugan, A., Balossier, G., Michel, J., and Ferreira, J. M. F. (2009). Electrochemical and structural evaluation of functionally graded bioglass-apatite composites electrophoretically deposited onto Ti6Al4V alloy. Electrochimica Acta 54 (4), 1192–1198. doi:10.1016/j.electacta.2008.08.055
Bargavi, P., Chandran, R. R., Durgalakshmi, D., Rajashree, P., Ramya, R., and Balakumar, S. (2022). Drug infused Al2O3-bioactive glass coatings toward the cure of orthopedic infection. Prog. Biomater. 11 (1), 79–94. doi:10.1007/s40204-022-00181-y
Bargavi, P., Chitra, S., Durgalakshmi, D., Radha, G., and Balakumar, S. (2020). Zirconia reinforced bio-active glass coating by spray pyrolysis: structure, surface topography, in-vitro biological evaluation and antibacterial activities. Mater. Today Commun. 25, 101253. doi:10.1016/j.mtcomm.2020.101253
Berbecaru, C., Alexandru, H. V., Stan, G. E., Marcov, D. A., Pasuk, I., and Ianculescu, A. (2010). First stages of bioactivity of glass-ceramics thin films prepared by magnetron sputtering technique. Mater. Sci. Eng. B 169 (1-3), 101–105. doi:10.1016/j.mseb.2010.01.007
Boccaccini, A. R., Keim, S., Ma, R., Li, Y., and Zhitomirsky, I. (2010). Electrophoretic deposition of biomaterials. J. R. Soc. Interface 7 (5), S581–S613. doi:10.1098/rsif.2010.0156.focus
Bohner, M., and Lemaitre, J. (2009). Can bioactivity be tested in vitro with SBF solution? Biomaterials 30 (12), 2175–2179. doi:10.1016/j.biomaterials.2009.01.008
Boschetto, F., Doan, H. N., Vo, P. P., Zanocco, M., Zhu, W. L., Sakai, W., et al. (2020). Antibacterial and osteoconductive effects of chitosan/polyethylene oxide (PEO)/Bioactive glass nanofibers for orthopedic applications. Appl. Sciences-Basel 10 (7), 2360. doi:10.3390/app10072360
Brånemark, P. I., Hansson, B. O., Adell, R., Breine, U., Lindström, J., Hallén, O., et al. (1977). Osseointegrated implants in the treatment of the edentulous jaw. Experience from a 10-year period. Scand. J. Plast. Reconstr. Surg. Suppl. 16, 1–132.
Brauer, D. S. (2015). Bioactive glasses—structure and properties. Angew. Chem. Int. Ed. 54 (14), 4160–4181. doi:10.1002/anie.201405310
Brown, P. (1989). Sol-gel technology for thin films, fibers, preforms, electronics, and specialty shapes: Edited by Lisa C Klein. Noyes Publications.
Buser, D., Janner, S. F. M., Wittneben, J. G., Bragger, U., Ramseier, C. A., and Salvi, G. E. (2012). 10-Year survival and success rates of 511 titanium implants with a sandblasted and acid-etched surface: a retrospective study in 303 partially edentulous patients. Clin. IMPLANT Dent. Relat. Res. 14 (6), 839–851. doi:10.1111/j.1708-8208.2012.00456.x
Calderon Velasco, S., Cavaleiro, A., and Carvalho, S. (2016). Functional properties of ceramic-Ag nanocomposite coatings produced by magnetron sputtering. Prog. Mater. Sci. 84, 158–191. doi:10.1016/j.pmatsci.2016.09.005
Cannillo, V., Colmenares-Angulo, J., Lusvarghi, L., Pierli, F., and Sampath, S. (2009). In vitro characterisation of plasma-sprayed apatite/wollastonite glass–ceramic biocoatings on titanium alloys. J. Eur. Ceram. Soc. 29 (9), 1665–1677. doi:10.1016/j.jeurceramsoc.2008.09.022
Catauro, M., Bollino, F., Papale, F., and Ciprioti, S. V. (2015). Investigation on bioactivity, biocompatibility, thermal behavior and antibacterial properties of calcium silicate glass coatings containing Ag. J. Non-Crystalline Solids 422, 16–22. doi:10.1016/j.jnoncrysol.2015.04.037
Catauro, M., Papale, F., and Bollino, F. (2016). Coatings of titanium substrates with xCaO·(1 − x)SiO 2 sol–gel materials: characterization, bioactivity and biocompatibility evaluation. Mater. Sci. Eng. C-Materials Biol. Appl. 58, 846–851. doi:10.1016/j.msec.2015.09.033
Chang, M. X., and Xiong, F. (2020). Astaxanthin and its effects in inflammatory responses and inflammation-associated diseases: recent advances and future directions. Molecules 25 (22), 5342. doi:10.3390/molecules25225342
Chattopadhyay, S., Dash, S. K., Tripathy, S., Das, B., Mandal, D., Pramanik, P., et al. (2015). Toxicity of cobalt oxide nanoparticles to normal cells; an in vitro and in vivo study. Chemico-Biological Interact. 226, 58–71. doi:10.1016/j.cbi.2014.11.016
Chen, L., McCrate, J. M., Lee, J. C. M., and Li, H. (2011). The role of surface charge on the uptake and biocompatibility of hydroxyapatite nanoparticles with osteoblast cells. Nanotechnology 22 (10), 105708. doi:10.1088/0957-4484/22/10/105708
Chen, Q., and Thouas, G. A. (2015). Metallic implant biomaterials. Mater. Sci. Eng. R Rep. 87, 1–57. doi:10.1016/j.mser.2014.10.001
Chen, X. C., Zhang, M. J., Pu, X. M., Yin, G. F., Liao, X. M., Huang, Z. B., et al. (2014). Characteristics of heat-treated plasma-sprayed CaO-MgO-SiO2-based bioactive glass-ceramic coatings on Ti-6Al-4V alloy. Surf. Coatings Technol. 249, 97–103. doi:10.1016/j.surfcoat.2014.03.056
Cohrs, N. H., Schulz-Schonhagen, K., Mohn, D., Wolint, P., Meier Burgisser, G., Stark, W. J., et al. (2019). Modification of silicone elastomers with Bioglass 45S5® increases in ovo tissue biointegration. J. Biomed. Mater Res. B Appl. Biomater. 107 (4), 1180–1188. doi:10.1002/jbm.b.34211
Comesana, R., Quintero, F., Lusquinos, F., Pascual, M. J., Boutinguiza, M., Duran, A., et al. (2010). Laser cladding of bioactive glass coatings. Acta Biomater. 6 (3), 953–961. doi:10.1016/j.actbio.2009.08.010
Coraça-Huber, D. C., Fille, M., Hausdorfer, J., Putzer, D., and Nogler, M. (2014). Efficacy of antibacterial bioactive glass S53P4 against S. aureus biofilms grown on titanium discs in vitro. J. Orthop. Res. 32 (1), 175–177. doi:10.1002/jor.22463
Costa, M. (1991). Molecular mechanisms of nickel carcinogenesis. Annu. Rev. Pharmacol. Toxicol. 31, 321–337. doi:10.1146/annurev.pa.31.040191.001541
Costa, R. C., Souza, J. G. S., Cordeiro, J. M., Bertolini, M., de Avila, E. D., Landers, R., et al. (2020). Synthesis of bioactive glass-based coating by plasma electrolytic oxidation: untangling a new deposition pathway toward titanium implant surfaces. J. Colloid Interface Sci. 579, 680–698. doi:10.1016/j.jcis.2020.06.102
Crush, J., Hussain, A., Seah, K. T. M., and Khan, W. S. (2021). Bioactive glass: methods for assessing angiogenesis and osteogenesis. Front. Cell. Dev. Biol. 9, 643781. doi:10.3389/fcell.2021.643781
Cuvelier, D., Théry, M., Chu, Y. S., Dufour, S., Thiéry, J. P., Bornens, M., et al. (2007). The universal dynamics of cell spreading. Curr. Biol. 17 (8), 694–699. doi:10.1016/j.cub.2007.02.058
Dhinasekaran, D., Kaliaraj, G. S., Jagannathan, M., Rajendran, A. R., Prakasarao, A., Ganesan, S., et al. (2021). Pulsed laser deposition of nanostructured bioactive glass and hydroxyapatite coatings: microstructural and electrochemical characterization. Mater. Sci. Eng. C-Materials Biol. Appl. 130, 112459. doi:10.1016/j.msec.2021.112459
Drago, L., Toscano, M., and Bottagisio, M. (2018). Recent evidence on bioactive glass antimicrobial and antibiofilm activity: a mini-review. Materials 11 (2), 326. doi:10.3390/ma11020326
Durgalakshmi, D., Rakkesh, R. A., Aruna, P., Ganesan, S., and Balakumar, S. (2020). Bioactivity and hemocompatibility of sol–gel bioactive glass synthesized under different catalytic conditions. New J. Chem. 44 (48), 21026–21037. doi:10.1039/D0NJ02445G
Echezarreta-López, M. M., and Landin, M. (2013). Using machine learning for improving knowledge on antibacterial effect of bioactive glass. Int. J. Pharm. 453 (2), 641–647. doi:10.1016/j.ijpharm.2013.06.036
Escalas, F., Galante, J., Rostoker, W., and Coogan, P. (1976). Biocompatibility of materials for total joint replacement. J. Biomed. Mater. Res. 10 (2), 175–195. doi:10.1002/jbm.820100203
Estrada-Cabrera, E., Torres-Ferrer, L. R., Aztatzi-Aguilar, O. G., De Vizcaya-Ruiz, A., Meraz-Rios, M. A., Zarate-Triviño, D. G., et al. (2019). Chitosan-bioglass coatings on partially nanostructured anodized Ti-6Al-4V alloy for biomedical applications. Surf. Coatings Technol. 375, 468–476. doi:10.1016/j.surfcoat.2019.07.002
Fischer, K., and Stenberg, T. (2012). Prospective 10-year cohort study based on a randomized controlled trial (RCT) on implant-supported full-arch maxillary prostheses. Part 1: sandblasted and acid-etched implants and mucosal tissue. Clin. IMPLANT Dent. Relat. Res. 14 (6), 808–815. doi:10.1111/j.1708-8208.2011.00389.x
Fleury, C., Petit, A., Mwale, F., Antoniou, J., Zukor, D. J., Tabrizian, M., et al. (2006). Effect of cobalt and chromium ions on human MG-63 osteoblasts in vitro: morphology, cytotoxicity, and oxidative stress. Biomaterials 27 (18), 3351–3360. doi:10.1016/j.biomaterials.2006.01.035
Fu, T., Alajmi, Z., Shen, Y. G., Wang, L. J., Yang, S. Y., and Zhang, M. (2017b). Sol-gel preparation and properties of Ag-containing bioactive glass films on titanium. Int. J. Appl. Ceram. Technol. 14 (6), 1117–1124. doi:10.1111/ijac.12713
Fu, T., Liu, B. G., Zhou, Y. M., and Wu, X. M. (2011). Sol-gel titania coating on NiTi alloy with a porous titania film as interlayer. J. SOL-GEL Sci. Technol. 58 (1), 307–311. doi:10.1007/s10971-010-2392-5
Fu, T., Sun, J. M., Zhao, Y. T., Wang, L. J., Zhou, Y. C., and Ma, X. (2017a). Hydrothermally crystallized Sr-containing bioactive glass film and its cytocompatibility. Ceram. Int. 43 (16), 13689–13695. doi:10.1016/j.ceramint.2017.07.080
Gaweda, M., Jelen, P., Dlugon, E., Wajda, A., Lesniak, M., Simka, W., et al. (2018). Bioactive layers based on black glasses on titanium substrates. J. Am. Ceram. Soc. 101 (2), 590–601. doi:10.1111/jace.15202
Gebhardt, F., Seuss, S., Turhan, M. C., Hornberger, H., Virtanen, S., and Boccaccini, A. R. (2012). Characterization of electrophoretic chitosan coatings on stainless steel. Mater. Lett. 66 (1), 302–304. doi:10.1016/j.matlet.2011.08.088
Geng, Z., Ji, L., Li, Z., Wang, J., He, H., Cui, Z., et al. (2021b). Nano-needle strontium-substituted apatite coating enhances osteoporotic osseointegration through promoting osteogenesis and inhibiting osteoclastogenesis. Bioact. Mater. 6 (2021), 905–915. doi:10.1016/j.bioactmat.2020.09.024
Geng, Z., Li, X., Ji, L., Li, Z., Zhu, S., Cui, Z., et al. (2021a). A novel snail-inspired bionic design of titanium with strontium-substituted hydroxyapatite coating for promoting osseointegration. J. Mater. Sci. Technol. 79(2021) 35–45. doi:10.1016/j.jmst.2020.11.041
Geng, Z., Sang, S., Wang, S., Meng, F., Li, Z., Zhu, S., et al. (2022). Optimizing the strontium content to achieve an ideal osseointegration through balancing apatite-forming ability and osteogenic activity. Biomater. Adv. 133(2022) 112647. doi:10.1016/j.msec.2022.112647
Gomez-Vega, J. M., Hozumi, A., Saiz, E., Tomsia, A. P., Sugimura, H., and Takai, O. (2001). Bioactive glass–mesoporous silica coatings on Ti6Al4V through enameling and triblock-copolymer-templated sol-gel processing. J. Biomed. Mater. Res. 56(3): 382–389. doi:10.1002/1097-4636(20010905)56:3<382::AID-JBM1107>3.0
Goriainov, V., Cook, R., Latham, J. M., Dunlop, D. G., and Oreffo, R. O. C. (2014). Bone and metal: an orthopaedic perspective on osseointegration of metals. Acta Biomater. 10 (10), 4043–4057. doi:10.1016/j.actbio.2014.06.004
Gu, H., Guo, F., Zhou, X., Gong, L., Zhang, Y., Zhai, W., et al. (2011). The stimulation of osteogenic differentiation of human adipose-derived stem cells by ionic products from akermanite dissolution via activation of the ERK pathway. Biomaterials 32 (29), 7023–7033. doi:10.1016/j.biomaterials.2011.06.003
Guimarães, R. P., Xavier, L. G. O., Maltos, K. L. M., Sá, A. F., Domingues, R. Z., Carvalho, V. E., et al. (2020). Koh group influence on titanium surfaces and pure sol-gel silica for enhanced osteogenic activity. J. Biomater. Appl. 35 (3), 405–421. doi:10.1177/0885328220934323
He, D. H., Wang, P., Liu, P., Liu, X. K., Chen, X. H., Li, W., et al. (2019). Anodic voltage dependence of Ti-6Al-4V substrates and hydroxyapatite coating. J. Nanosci. Nanotechnol. 19 (9), 5700–5706. doi:10.1166/jnn.2019.16530
Hench, L. L., and Greenspan, D. (2013). Interactions between bioactive glass and collagen: a review and new perspectives. J. Aust. Ceram. Soc. 49 (2), 1–40.
Hench, L. L. (2006). The story of Bioglass®. J. Mater. Sci. Mater. Med. 17(11): 967–978. doi:10.1007/s10856-006-0432-z
Hench, L. L., Xynos, I. D., Edgar, A. J., Buttery, L. D. K., and Polak, J. M. (2020). Gene activating glasses. Phys. Chem. GLASSES-EUROPEAN J. GLASS Sci. Technol. PART B 61 (4), 155–162. doi:10.13036/17533562.61.4.Hench
Henstock, J. R., Canham, L. T., and Anderson, S. I. (2015). Silicon: the evolution of its use in biomaterials. Acta Biomater. 11, 17–26. doi:10.1016/j.actbio.2014.09.025
Herman, H., Sampath, S., and McCune, R. (2000). Thermal spray: current status and future trends. MRS Bull. 25 (7), 17–25. doi:10.1557/mrs2000.119
Hoppe, A., Güldal, N. S., and Boccaccini, A. R. (2011). A review of the biological response to ionic dissolution products from bioactive glasses and glass-ceramics. Biomaterials 32 (11), 2757–2774. doi:10.1016/j.biomaterials.2011.01.004
Hu, C., Ashok, D., Nisbet, D. R., and Gautam, V. (2019). Bioinspired surface modification of orthopedic implants for bone tissue engineering. Biomaterials 219, 119366. doi:10.1016/j.biomaterials.2019.119366
Huang, Y.-J., and Nan, G.-X. (2019). Oxidative stress-induced angiogenesis. J. Clin. Neurosci. 63, 13–16. doi:10.1016/j.jocn.2019.02.019
Huang, Y., Jin, X., Zhang, X., Sun, H., Tu, J., Tang, T., et al. (2009). In vitro and in vivo evaluation of akermanite bioceramics for bone regeneration. Biomaterials 30 (28), 5041–5048. doi:10.1016/j.biomaterials.2009.05.077
Humphries, M. J., Even-Ram, S., and Artym, V. (2009). Extracellular matrix protocols. Second Edition. Totowa, NJ: Humana Press, 203–210. doi:10.1007/978-1-59745-413-1_14Cell adhesion assays
Ishikawa, K., Miyamoto, Y., Yuasa, T., Ito, A., Nagayama, M., and Suzuki, K. (2002). Fabrication of Zn containing apatite cement and its initial evaluation using human osteoblastic cells. Biomaterials 23 (2), 423–428. doi:10.1016/S0142-9612(01)00121-1
Jones, J. R. (2013). Review of bioactive glass: from Hench to hybrids. Acta Biomater. 9 (1), 4457–4486. doi:10.1016/j.actbio.2012.08.023
Jugowiec, D., Lukaszczyk, A., Cieniek, L., Kot, M., Reczynska, K., Cholewa-Kowalska, K., et al. (2017). Electrophoretic, deposition and characterization of composite chitosan-based coatings incorporating bioglass and sol-gel glass particles on the Ti-13Nb-13Zr alloy. Surf. Coatings Technol. 319, 33–46. doi:10.1016/j.surfcoat.2017.03.067
Kargozar, S., Montazerian, M., Fiume, E., and Baino, F. (2019). Multiple and promising applications of strontium (Sr)-Containing bioactive glasses in bone tissue engineering. Front. Bioeng. Biotechnol. 7, 161. doi:10.3389/fbioe.2019.00161
Kassab, M. M., and Cohen, R. E. (2003). The etiology and prevalence of gingival recession. J. Am. Dent. Assoc. 134 (2), 220–225. doi:10.14219/jada.archive.2003.0137
Khanmohammadi, S., Ojaghi-Ilkhchi, M., and Farrokhi-Rad, M. (2020). Evaluation of bioglass and hydroxyapatite based nanocomposite coatings obtained by electrophoretic deposition. Ceram. Int. 46 (16), 26069–26077. doi:10.1016/j.ceramint.2020.07.100
Kim, H. M., Miyaji, F., Kokubo, T., and Nakamura, T. (1996). Preparation of functionally graded bioactive titanium and its alloys by chemical treatment. J. Biomed. Mater Res. 32 (3), 1102–1107. doi:10.2320/jinstmet1952.62.11_1102
Klyui, N. I., Chornyi, V. S., Zatovsky, I. V., Tsabiy, L. I., Buryanov, A. A., Protsenko, V. V., et al. (2021). Properties of gas detonation ceramic coatings and their effect on the osseointegration of titanium implants for bone defect replacement. Ceram. Int. 47 (18), 25425–25439. doi:10.1016/j.ceramint.2021.05.265
Lavenus, S., Poxson, D. J., Ogievetsky, N., Dordick, J. S., and Siegel, R. W. (2015). Stem cell behavior on tailored porous oxide surface coatings. Biomaterials 55, 96–109. doi:10.1016/j.biomaterials.2015.03.033
Ledda, M., De Bonis, A., Bertani, F. R., Cacciotti, I., Teghil, R., Lolli, M. G., et al. (2015). Interdisciplinary approach to cell-biomaterial interactions: biocompatibility and cell friendly characteristics of RKKP glass-ceramic coatings on titanium. Biomed. Mater. 10 (3), 035005. doi:10.1088/1748-6041/10/3/035005
Ledda, M., Fosca, M., De Bonis, A., Curcio, M., Teghil, R., Lolli, M. G., et al. (2016). Placenta derived mesenchymal stem cells hosted on RKKP glass-ceramic: a tissue engineering strategy for bone regenerative medicine applications. Biomed Res. Int. 2016, 1–11. doi:10.1155/2016/3657906
Lee, S., Goh, B. T., Wolke, J., Tideman, H., Stoelinga, P., and Jansen, J. (2010). Soft tissue adaptation to modified titanium surfaces. J. Biomed. Mater. Res. Part A 95A (2), 543–549. doi:10.1002/jbm.a.32849
Li, B., Li, Y., Li, J., Fu, X., Li, H., Wang, H., et al. (2014). Influence of nanostructures on the biological properties of Ti implants after anodic oxidation. J. Mater Sci. Mater Med. 25 (1), 199–205. doi:10.1007/s10856-013-5064-5
Li, Y., Chen, L., Chen, X., Hill, R., Zou, S., Wang, M., et al. (2021). High phosphate content in bioactive glasses promotes osteogenesis in vitro and in vivo. Dent. Mater 37 (2), 272–283. doi:10.1016/j.dental.2020.11.017
Löe, H. (1967). The gingival index, the plaque index and the retention index systems. J. Periodontology 38 (6), 610–616. doi:10.1902/jop.1967.38.6_part2.610
Long, M., and Rack, H. J. (1998). Titanium alloys in total joint replacement—A materials science perspective. Biomaterials 19 (18), 1621–1639. doi:10.1016/S0142-9612(97)00146-4
López, M. M. M., Fauré, J., Cabrera, M. I. E., and García, M. E. C. (2016). Structural characterization and electrochemical behavior of 45S5 bioglass coating on Ti6Al4V alloy for dental applications. Mater. Sci. Eng. B 206, 30–38. doi:10.1016/j.mseb.2015.09.003
Lung, C. Y. K., Abdalla, M. M., Chu, C. H., Yin, I., Got, S. R., and Matinlinna, J. P. (2021). A multi-element-doped porous bioactive glass coating for implant applications. Materials 14 (4), 961. doi:10.3390/ma14040961
Ma, J., Wang, C. Z., Ban, C. L., Chen, C. Z., and Zhang, H. M. (2016). Pulsed laser deposition of magnesium-containing bioactive glass film on porous Ti-6Al-4V substrate pretreated by micro-arc oxidation. Vacuum 125, 48–55. doi:10.1016/j.vacuum.2015.12.005
Mahlooji, E., Atapour, M., and Labbaf, S. (2019). Electrophoretic deposition of Bioactive glass - chitosan nanocomposite coatings on Ti-6Al-4V for orthopedic applications. Carbohydr. Polym. 226, 115299. doi:10.1016/j.carbpol.2019.115299
Manam, N. S., Harun, W. S. W., Awang Shri, D. N., Bin Che Ghani, S., Kurniawan, T., Ismail, M., et al. (2017). Study of corrosion in biocompatible metals for implants: a review. J. Alloys Compd. 701, 698–715. doi:10.1016/j.jallcom.2017.01.196
Manivasagam, V. K., Sabino, R. M., Kantam, P., and Popat, K. C. (2021). Surface modification strategies to improve titanium hemocompatibility: a comprehensive review. Mater Adv. 2 (18), 5824–5842. doi:10.1039/d1ma00367d
Marques, I. D., Barao, V. A., da Cruz, N. C., Yuan, J. C., Mesquita, M. F., Ricomini-Filho, A. P., et al. (2015). Electrochemical behavior of bioactive coatings on cp-Ti surface for dental application. Corros. Sci. 100, 133–146. doi:10.1016/j.corsci.2015.07.019
Massera, J., Fagerlund, S., Hupa, L., and Hupa, M. (2012). Crystallization mechanism of the bioactive glasses, 45S5 and S53P4. J. Am. Ceram. Soc. 95 (2), 607–613. doi:10.1111/j.1551-2916.2011.05012.x
Matter, M. T., Maliqi, L., Keevend, K., Guimond, S., Ng, J., Armagan, E., et al. (2021). One-Step synthesis of versatile antimicrobial nano-architected implant coatings for hard and soft tissue healing. Acs Appl. Mater. Interfaces 13 (28), 33300–33310. doi:10.1021/acsami.1c10121
Maximov, M., Maximov, O.-C., Craciun, L., Ficai, D., Ficai, A., and Andronescu, E. (2021). Bioactive glass—an extensive study of the preparation and coating methods. Coatings 11 (11), 1386. doi:10.3390/coatings11111386
Medzhitov, R. (2010). Inflammation 2010: new adventures of an old flame. Cell. 140 (6), 771–776. doi:10.1016/j.cell.2010.03.006
Mehdikhani-Nahrkhalaji, M., Fathi, M. H., Mortazavi, V., Mousavi, S. B., Akhavan, A., Haghighat, A., et al. (2015). Biodegradable nanocomposite coatings accelerate bone healing: in vivo evaluation. Dent. Res. J. (Isfahan). 12 (1), 89–99. doi:10.4103/1735-3327.150342
Mistry, S., Roy, R., Kundu, B., Datta, S., Kumar, M., Chanda, A., et al. (2016). Clinical outcome of hydroxyapatite coated, bioactive glass coated, and machined Ti6Al4V threaded dental implant in human jaws: a short-term comparative study. Implant Dent. 25 (2), 252–260. doi:10.1097/ID.0000000000000376
Moreira, C. D. F., Carvalho, S. M., Sousa, R. G., Mansur, H. S., and Pereira, M. M. (2018). Nanostructured chitosan/gelatin/bioactive glass in situ forming hydrogel composites as a potential injectable matrix for bone tissue engineering. Mater. Chem. Phys. 218, 304–316. doi:10.1016/j.matchemphys.2018.07.039
Nalezinkova, M. (2020). In vitro hemocompatibility testing of medical devices. Thromb. Res. 195, 146–150. doi:10.1016/j.thromres.2020.07.027
Naseri, S., Nazhat, S. N., and Ylänen, H. (2018). Bioactive glasses. Second Edition. Woodhead Publishing, 381–405. doi:10.1016/B978-0-08-100936-9.00019-814 - bioactive and soluble glasses for wound-healing applications
Nesabi, M., Valanezhad, A., Safaee, S., Odatsu, T., Abe, S., and Watanabe, I. (2021). A novel multi-structural reinforced treatment on Ti implant utilizing a combination of alkali solution and bioactive glass sol. J. Mech. Behav. Biomed. Mater. 124, 104837. doi:10.1016/j.jmbbm.2021.104837
Newman, S. D., Lotfibakhshaiesh, N., O'Donnell, M., Walboomers, X. F., Horwood, N., Jansen, J. A., et al. (2014). Enhanced osseous implant fixation with strontium-substituted bioactive glass coating. Tissue Eng. Part A 20 (13-14), 1850–1857. doi:10.1089/ten.TEA.2013.0304
Niinomi, M. (1998). Mechanical properties of biomedical titanium alloys. Mater. Sci. Eng. A 243 (1), 231–236. doi:10.1016/S0921-5093(97)00806-X
Nishiguchi, S., Fujibayashi, S., Kim, H. M., Kokubo, T., and Nakamura, T. (2003). Biology of alkali- and heat-treated titanium implants. J. Biomed. Mater Res. A 67 (1), 26–35. doi:10.1002/jbm.a.10540
Nowak-Sliwinska, P., Alitalo, K., Allen, E., Anisimov, A., Aplin, A. C., Auerbach, R., et al. (2018). Consensus guidelines for the use and interpretation of angiogenesis assays. Angiogenesis 21 (3), 425–532. doi:10.1007/s10456-018-9613-x
Oki, A., Parveen, B., Hossain, S., Adeniji, S., and Donahue, H. (2004). Preparation and in vitro bioactivity of zinc containing sol-gel–derived bioglass materials. J. Biomed. Mater. Res. Part A 69A (2), 216–221. doi:10.1002/jbm.a.20070
Oliver, J. N., Su, Y., Lu, X., Kuo, P. H., Du, J., and Zhu, D. (2019). Bioactive glass coatings on metallic implants for biomedical applications. Bioact. Mater 4, 261–270. doi:10.1016/j.bioactmat.2019.09.002
O’Neill, E., Awale, G., Daneshmandi, L., Umerah, O., and Lo, K. W. H. (2018). The roles of ions on bone regeneration. Drug Discov. Today 23 (4), 879–890. doi:10.1016/j.drudis.2018.01.049
Ordikhani, F., and Simchi, A. (2014). Long-term antibiotic delivery by chitosan-based composite coatings with bone regenerative potential. Appl. Surf. Sci. 317, 56–66. doi:10.1016/j.apsusc.2014.07.197
Ordikhani, F., Zustiak, S. P., and Simchi, A. (2016). Surface modifications of titanium implants by multilayer bioactive coatings with drug delivery potential: antimicrobial, biological, and drug release studies. Jom 68 (4), 1100–1108. doi:10.1007/s11837-016-1840-2
Orita, K., Goto, K., Kuroda, Y., Kawai, T., Okuzu, Y., Takaoka, Y., et al. (2022). Long-term outcome of primary total hip arthroplasty with cementless bioactive glass ceramic bottom-coated implants and highly cross-linked polyethylene: A minimum 10-year analysis. J. Orthop. Sci. 28 (2), 385–390. doi:10.1016/j.jos.2021.12.019
Owen, T. A., Aronow, M., Shalhoub, V., Barone, L. M., Wilming, L., Tassinari, M. S., et al. (1990). Progressive development of the rat osteoblast phenotype in vitro: reciprocal relationships in expression of genes associated with osteoblast proliferation and differentiation during formation of the bone extracellular matrix. J. Cell. Physiol. 143 (3), 420–430. doi:10.1002/jcp.1041430304
Palangadan, R., Sukumaran, A., Fernandez, F. B., John, A., and Varma, H. (2014). Pulsed laser deposition and in vitro characteristics of triphasic - HASi composition on titanium. J. Biomaterials Appl. 28 (6), 849–858. doi:10.1177/0885328213484545
Pantulap, U., Arango-Ospina, M., and Boccaccini, A. R. (2021). Bioactive glasses incorporating less-common ions to improve biological and physical properties. J. Mater Sci. Mater Med. 33 (1), 3. doi:10.1007/s10856-021-06626-3
Park Ridge, N. J. (1988). Price: $72.00 ISBN 0-8155-1154-X. Mater. Res. Bull. 24(3): 389. doi:10.1016/0025-5408(89)90225-0
Patel, K. D., Buitrago, J. O., Parthiban, S. P., Lee, J. H., Singh, R. K., Knowles, J. C., et al. (2019). Combined effects of nanoroughness and ions produced by electrodeposition of mesoporous bioglass nanoparticle for bone regeneration. ACS Appl. Bio Mater 2 (11), 5190–5203. doi:10.1021/acsabm.9b00859
Patel, K. D., El-Fiqi, A., Lee, H.-Y., Singh, R. K., Kim, D.-A., Lee, H.-H., et al. (2012). Chitosan–nanobioactive glass electrophoretic coatings with bone regenerative and drug delivering potential. J. Mater. Chem. 22 (47), 24945–24956. doi:10.1039/c2jm33830k
Pattanaik, B., Pawar, S., and Pattanaik, S. (2012). Biocompatible implant surface treatments. Indian J. Dent. Res. 23 (3), 398–406. doi:10.4103/0970-9290.102240
Pishbin, F., Mourino, V., Gilchrist, J. B., McComb, D. W., Kreppel, S., Salih, V., et al. (2013). Single-step electrochemical deposition of antimicrobial orthopaedic coatings based on a bioactive glass/chitosan/nano-silver composite system. Acta Biomater. 9 (7), 7469–7479. doi:10.1016/j.actbio.2013.03.006
Pishbin, F., Simchi, A., Ryan, M. P., and Boccaccini, A. R. (2011). Electrophoretic deposition of chitosan/45S5 Bioglass® composite coatings for orthopaedic applications. Surf. Coatings Technol. 205 (23-24), 5260–5268. doi:10.1016/j.surfcoat.2011.05.026
Ponzetti, M., and Rucci, N. (2021). Osteoblast differentiation and signaling: established concepts and emerging topics. Int. J. Mol. Sci. 22 (13), 6651. doi:10.3390/ijms22136651
Popa, A. C., Stan, G. E., Enculescu, M., Tanase, C., Tulyaganov, D. U., and Ferreira, J. M. (2015). Superior biofunctionality of dental implant fixtures uniformly coated with durable bioglass films by magnetron sputtering. J. Mech. Behav. Biomed. Mater 51, 313–327. doi:10.1016/j.jmbbm.2015.07.028
Popa, A. C., Stan, G. E., Husanu, M. A., Mercioniu, I., Santos, L. F., Fernandes, H. R., et al. (2017). Bioglass implant-coating interactions in synthetic physiological fluids with varying degrees of biomimicry. Int. J. Nanomedicine 12, 683–707. doi:10.2147/IJN.S123236
Pore, O. C., Fulari, A. V., Shejwal, R. V., Fulari, V. J., and Lohar, G. M. (2021). Review on recent progress in hydrothermally synthesized MCo2O4/rGO composite for energy storage devices. Chem. Eng. J. 426, 131544. doi:10.1016/j.cej.2021.131544
Rastegari, S., and Salahinejad, E. (2019). Surface modification of Ti-6Al-4V alloy for osseointegration by alkaline treatment and chitosan-matrix glass-reinforced nanocomposite coating. Carbohydr. Polym. 205, 302–311. doi:10.1016/j.carbpol.2018.10.082
Rau, J. V., De Bonis, A., Curcio, M., Schuhladen, K., Barbaro, K., De Bellis, G., et al. (2020). Borate and silicate bioactive glass coatings prepared by nanosecond pulsed laser deposition. Coatings 10 (11), 1105. doi:10.3390/coatings10111105
Rivadeneira, J., and Gorustovich, A. (2017). Bioactive glasses as delivery systems for antimicrobial agents. J. Appl. Microbiol. 122 (1365-2672), 1424–1437. (Electronic). doi:10.1111/jam.13393
Rizwan, M., Alias, R., Zaidi, U. Z., Mahmoodian, R., and Hamdi, M. (2018). Surface modification of valve metals using plasma electrolytic oxidation for antibacterial applications: a review. J. Biomed. Mater Res. A 106 (2), 590–605. doi:10.1002/jbm.a.36259
Safaee, S., Valanezhad, A., Nesabi, M., Jafarnia, S., Sano, H., Shahabi, S., et al. (2021). Fabrication of bioactive glass coating on pure titanium by sol-dip method: dental applications. Dent. Mater J. 40 (4), 949–956. doi:10.4012/dmj.2020-323
Saffarian Tousi, N., Velten, M. F., Bishop, T. J., Leong, K. K., Barkhordar, N. S., Marshall, G. W., et al. (2013). Combinatorial effect of Si4+, Ca2+, and Mg2+ released from bioactive glasses on osteoblast osteocalcin expression and biomineralization. Mater. Sci. Eng. C 33 (5), 2757–2765. doi:10.1016/j.msec.2013.02.044
Saino, E., Maliardi, V., Quartarone, E., Fassina, L., Benedetti, L., De Angelis, M. G., et al. (2010). In vitro enhancement of SAOS-2 cell calcified matrix deposition onto radio frequency magnetron sputtered bioglass-coated titanium scaffolds. Tissue Eng. Part A 16 (3), 995–1008. doi:10.1089/ten.TEA.2009.0051
Sang, S., Wang, S., Yang, C., Geng, Z., and Zhang, X. (2022). Sponge-inspired sulfonated polyetheretherketone loaded with polydopamine-protected osthole nanoparticles and berberine enhances osteogenic activity and prevents implant-related infections. Chem. Eng. J. 437 (2022), 135255. doi:10.1016/j.cej.2022.135255
Santos, M. I., Unger, R. E., Sousa, R. A., Reis, R. L., and Kirkpatrick, C. J. (2009). Crosstalk between osteoblasts and endothelial cells co-cultured on a polycaprolactone–starch scaffold and the in vitro development of vascularization. Biomaterials 30 (26), 4407–4415. doi:10.1016/j.biomaterials.2009.05.004
Sanz-Herrera, J. A., and Boccaccini, A. R. (2011). Modelling bioactivity and degradation of bioactive glass based tissue engineering scaffolds. Int. J. Solids Struct. 48 (2), 257–268. doi:10.1016/j.ijsolstr.2010.09.025
Sepulveda, P., Jones, J. R., and Hench, L. L. (2002). In vitro dissolution of melt-derived 45S5 and sol-gel derived 58S bioactive glasses. J. Biomed. Mater. Res. 61 (2), 301–311. doi:10.1002/jbm.10207
Serra, J., Liste, S., González, P., Serra, C., Borrajo, J. P., Chiussi, S., et al. (2004). The role of the temperature and laser fluence on the properties of PLD bioactive glass films. Appl. Phys. A 79 (4), 983–986. doi:10.1007/s00339-004-2610-5
Shaikh, S., Kedia, S., Majumdar, A. G., Subramanian, M., and Sinha, S. (2019). 45S5 bioactive glass coating on Ti6Al4V alloy using pulsed laser deposition technique. Mater. Res. Express 6 (12), 125428. doi:10.1088/2053-1591/ab7568
Shamsi, M., Karimi, M., Ghollasi, M., Nezafati, N., Shahrousvand, M., Kamali, M., et al. (2017). In vitro proliferation and differentiation of human bone marrow mesenchymal stem cells into osteoblasts on nanocomposite scaffolds based on bioactive glass (64SiO2-31CaO-5P2O5)-poly-l-lactic acid nanofibers fabricated by electrospinning method. Mater. Sci. Eng. C 78, 114–123. doi:10.1016/j.msec.2017.02.165
Shi, J. Z., Chen, C. Z., Yu, H. J., and Zhang, S. J. (2008). The effect of process conditions on the properties of bioactive films prepared by magnetron sputtering. Vacuum 83 (2), 249–256. doi:10.1016/j.vacuum.2008.05.019
Silness, J., and Löe, H. (1964). Periodontal disease in pregnancy II. Correlation between oral hygiene and periodontal condition. Acta Odontol. Scand. 22 (1), 121–135. doi:10.3109/00016356408993968
Soares, P. B. F., Moura, C. C. G., Chinaglia, C. R., Zanotto, E. D., Zanetta-Barbosa, D., and Stavropoulos, A. (2018). Effect of titanium surface functionalization with bioactive glass on osseointegration: an experimental study in dogs. Clin. Oral Implants Res. 29 (11), 1120–1125. doi:10.1111/clr.13375
Sola, A., Bellucci, D., Cannillo, V., and Cattini, A. (2011). Bioactive glass coatings: A review. Surf. Eng. 27 (8), 560–572. doi:10.1179/1743294410y.0000000008
Su, T. R., Chu, Y. H., Yang, H. W., Huang, Y. F., and Ding, S. J. (2019). Component effects of bioactive glass on corrosion resistance and in vitro biological properties of apatite-matrix coatings. Bio-Medical Mater. Eng. 30 (2), 207–218. doi:10.3233/bme-191045
Sultana, A., Zare, M., Luo, H., and Ramakrishna, S. (2021). Surface engineering strategies to enhance the in situ performance of medical devices including atomic scale engineering. Int. J. Mol. Sci. 22 (21), 11788. doi:10.3390/ijms222111788
Sun, J., Li, J., Liu, X., Wei, L., Wang, G., and Meng, F. (2009). Proliferation and gene expression of osteoblasts cultured in DMEM containing the ionic products of dicalcium silicate coating. Biomed. Pharmacother. 63 (9), 650–657. doi:10.1016/j.biopha.2009.01.007
Taguchi, T., Yanagi, Y., Yoshimaru, K., Zhang, X.-Y., Matsuura, T., Nakayama, K., et al. (2019). Regenerative medicine using stem cells from human exfoliated deciduous teeth (SHED): a promising new treatment in pediatric surgery. Surg. Today 49 (4), 316–322. doi:10.1007/s00595-019-01783-z
Tian, B., Chen, W., Dong, Y. F., Marymont, J. V., Lei, Y., Ke, Q. F., et al. (2016). Silver nanoparticle-loaded hydroxyapatite coating: structure, antibacterial properties, and capacity for osteogenic induction in vitro. RSC Adv. 6 (11), 8549–8562. doi:10.1039/c5ra25391h
Torrisi, L., Borrielli, A., and Margarone, D. (2007). Study on the ablation threshold induced by pulsed lasers at different wavelengths. Nucl. Instrum. Methods Phys. Res. Sect. B Beam Interact. Mater. Atoms 255 (2), 373–379. doi:10.1016/j.nimb.2006.12.144
Trepat, X., Chen, Z., and Jacobson, K. (2012). Cell migration. Compr. Physiol. 2 (4), 2369–2392. doi:10.1002/cphy.c110012
Valanezhad, A., Tsuru, K., and Ishikawa, K. (2015). Fabrication of strongly attached hydroxyapatite coating on titanium by hydrothermal treatment of Ti–Zn–PO4 coated titanium in CaCl2 solution. J. Mater. Sci. Mater. Med. 26 (7), 212. doi:10.1007/s10856-015-5548-6
van Oirschot, B. A., Alghamdi, H. S., Närhi, T. O., Anil, S., Al Farraj Aldosari, A., van den Beucken, J. J., et al. (2014). In vivo evaluation of bioactive glass-based coatings on dental implants in a dog implantation model. Clin. Oral Implants Res. 25 (1), 21–28. doi:10.1111/clr.12060
van Oirschot, B. A., Meijer, G. J., Bronkhorst, E. M., Närhi, T., Jansen, J. A., and van den Beucken, J. J. (2016). Comparison of different surface modifications for titanium implants installed into the goat iliac crest. Clin. Oral Implants Res. 27 (2), e57–e67. doi:10.1111/clr.12529
van Oirschot, B., Eman, R. M., Habibovic, P., Leeuwenburgh, S. C. G., Tahmasebi, Z., Weinans, H., et al. (2016). Osteophilic properties of bone implant surface modifications in a cassette model on a decorticated goat spinal transverse process. Acta Biomater. 37, 195–205. doi:10.1016/j.actbio.2016.03.037
Vuornos, K., Ojansivu, M., Koivisto, J. T., Hakkanen, H., Belay, B., Montonen, T., et al. (2019). Bioactive glass ions induce efficient osteogenic differentiation of human adipose stem cells encapsulated in gellan gum and collagen type I hydrogels. Mater Sci. Eng. C Mater Biol. Appl. 99, 905–918. doi:10.1016/j.msec.2019.02.035
Wang, D. G., Chen, C. Z., Yang, X. X., Ming, X. C., and Zhang, W. L. (2018). Effect of bioglass addition on the properties of HA/BG composite films fabricated by pulsed laser deposition. Ceram. Int. 44 (12), 14528–14533. doi:10.1016/j.ceramint.2018.05.069
Wang, D. G., Xiao, F. H., Li, Y., Ming, X. C., Zhai, J. Q., and Chen, C. Z. (2020). Properties of HA-based composite films fabricated by pulsed laser deposition with an in-situ heat treatment. Surf. Coatings Technol. 394, 125863. doi:10.1016/j.surfcoat.2020.125863
Wang, G., Liu, X., Zreiqat, H., and Ding, C. (2011). Enhanced effects of nano-scale topography on the bioactivity and osteoblast behaviors of micron rough ZrO2 coatings. Colloids Surf. B Biointerfaces 86 (2), 267–274. doi:10.1016/j.colsurfb.2011.04.006
Wang, Z., Zhang, X., Gu, J., Yang, H., Nie, J., and Ma, G. (2014). Electrodeposition of alginate/chitosan layer-by-layer composite coatings on titanium substrates. Carbohydr. Polym. 103, 38–45. doi:10.1016/j.carbpol.2013.12.007
Wataha, J. C., Hanks, C. T., Strawn, S. E., and Fat, J. C. (1994). Cytotoxicity of components of resins and other dental restorative materials. J. Oral Rehabil. 21 (4), 453–462. doi:10.1111/j.1365-2842.1994.tb01159.x
Wei, C. K., and Ding, S. J. (2017). Dual-functional bone implants with antibacterial ability and osteogenic activity. J. Mater. Chem. B 5 (10), 1943–1953. doi:10.1039/c7tb00173h
Wolke, J. G. C., Vandenbulcke, E., van Oirschot, B., and Jansen, J. A. (2005). A study to the surface characteristics of RF magnetron sputtered bioglass - and calcium phosphate coatings. Key Eng. Mater. 284-286, 187–190. doi:10.4028/www.scientific.net/kem.284-286.187
Wu, C. T., Chen, Z. T., Yi, D. H., Chang, J., and Xiao, Y. (2014). Multidirectional effects of Sr-Mg-and Si-containing bioceramic coatings with high bonding strength on inflammation, osteoclastogenesis, and osteogenesis. Acs Appl. Mater. Interfaces 6 (6), 4264–4276. doi:10.1021/am4060035
Wu, M.-S., Aquino, L. B. B., Barbaza, M. Y. U., Hsieh, C.-L., De Castro-Cruz, K. A., Yang, L.-L., et al. (2019). Anti-inflammatory and anticancer properties of bioactive compounds from Sesamum indicum L.—a review. Molecules 24 (24), 4426. doi:10.3390/molecules24244426
Xynos, I. D., Edgar, A. J., Buttery, L. D. K., Hench, L. L., and Polak, J. M. (2001). Gene-expression profiling of human osteoblasts following treatment with the ionic products of Bioglass® 45S5 dissolution. J. Biomed. Mater. Res. 55 (2), 151–157. doi:10.1002/1097-4636(200105)55:2<151::aid-jbm1001>3.0.co;2-d
Xynos, I. D., Edgar, A. J., Buttery, L. D. K., Hench, L. L., and Polak, J. M. (2000). Ionic products of bioactive glass dissolution increase proliferation of human osteoblasts and induce insulin-like growth factor II mRNA expression and protein synthesis. Biochem. Biophysical Res. Commun. 276 (2), 461–465. doi:10.1006/bbrc.2000.3503
Yanovska, A., Kuznetsov, V., Stanislavov, A., Danilchenko, S., and Sukhodub, L. (2011). Synthesis and characterization of hydroxyapatite-based coatings for medical implants obtained on chemically modified Ti6Al4V substrates. Surf. Coatings Technol. 205 (23), 5324–5329. doi:10.1016/j.surfcoat.2011.05.040
Ye, X., Leeflang, S., Wu, C., Chang, J., Zhou, J., and Huan, Z. (2017). Mesoporous bioactive glass functionalized 3D Ti-6Al-4V scaffolds with improved surface bioactivity. Mater. (Basel) 10 (11), 1244. doi:10.3390/ma10111244
Zafar, M. S., Farooq, I., Awais, M., Najeeb, S., Khurshid, Z., Zohaib, S., et al. (2019). Chapter 11 - bioactive surface coatings for enhancing osseointegration of dental implants. Biomedical, therapeutic and clinical applications of bioactive glasses. Woodhead Publishing, 313–329. doi:10.1016/B978-0-08-102196-5.00011-2
Zarghami, V., Ghorbani, M., Bagheri, K. P., and Shokrgozar, M. A. (2021). Prevention the formation of biofilm on orthopedic implants by melittin thin layer on chitosan/bioactive glass/vancomycin coatings. J. Mater. Science-Materials Med. 32 (7), 75. doi:10.1007/s10856-021-06551-5
Zarghami, V., Ghorbani, M., Bagheri, K. P., and Shokrgozar, M. A. (2020). Prolongation of bactericidal efficiency of chitosan - bioactive glass coating by drug controlled release. Prog. Org. Coatings 139, 105440. doi:10.1016/j.porgcoat.2019.105440
Zhai, W., Lu, H., Wu, C., Chen, L., Lin, X., Naoki, K., et al. (2013). Stimulatory effects of the ionic products from Ca–Mg–Si bioceramics on both osteogenesis and angiogenesis in vitro. Acta Biomater. 9 (8), 8004–8014. doi:10.1016/j.actbio.2013.04.024
Zhang, G., Zhao, P., Lin, L., Qin, L., Huan, Z., Leeflang, S., et al. (2021). Surface-treated 3D printed Ti-6Al-4V scaffolds with enhanced bone regeneration performance: an in vivo study. Ann. Transl. Med. 9 (1), 39. doi:10.21037/atm-20-3829
Zhang, J., Lynch, R. J. M., Watson, T. F., and Banerjee, A. (2019). Chitosan-bioglass complexes promote subsurface remineralisation of incipient human carious enamel lesions. J. Dent. 84, 67–75. doi:10.1016/j.jdent.2019.03.006
Zhang, Y. L., Chen, L., Shi, M. C., Zhai, D., Zhu, H. Y., Chang, J., et al. (2016). Mesoporous bioactive glass nanolayer-modified zirconia coatings on Ti-6Al-4V with improved in vitro bioactivity. Int. J. Appl. Glass Sci. 7 (2), 216–228. doi:10.1111/ijag.12210
Zhao, Y., Song, M., Chen, C., and Liu, J. (2008). The role of the pressure in pulsed laser deposition of bioactive glass films. J. Non-Crystalline Solids 354 (33), 4000–4004. doi:10.1016/j.jnoncrysol.2008.05.019
Zhitomirsky, D., Roether, J. A., Boccaccini, A. R., and Zhitomirsky, I. (2009). Electrophoretic deposition of bioactive glass/polymer composite coatings with and without HA nanoparticle inclusions for biomedical applications. J. Mater. Process. Technol. 209 (4), 1853–1860. doi:10.1016/j.jmatprotec.2008.04.034
Zhou, J. C., Yang, Y. Y., Frank, M. A., Detsch, R., Boccaccini, A. R., and Virtanen, S. (2016). Accelerated degradation behavior and cytocompatibility of pure iron treated with sandblasting. ACS Appl. Mater. INTERFACES 8 (40), 26482–26492. doi:10.1021/acsami.6b07068
Zhou, Y., Wu, C., and Chang, J. (2019). Bioceramics to regulate stem cells and their microenvironment for tissue regeneration. Mater. Today 24, 41–56. doi:10.1016/j.mattod.2018.07.016
Keywords: bone regeneration, surface modification, biomaterials, tissue engineering, bioglass, bioactive materials
Citation: Liang J, Lu X, Zheng X, Li YR, Geng X, Sun K, Cai H, Jia Q, Jiang HB and Liu K (2023) Modification of titanium orthopedic implants with bioactive glass: a systematic review of in vivo and in vitro studies. Front. Bioeng. Biotechnol. 11:1269223. doi: 10.3389/fbioe.2023.1269223
Received: 29 July 2023; Accepted: 18 September 2023;
Published: 15 November 2023.
Edited by:
Xin Liu, Shanghai Jiao Tong University School of Medicine, ChinaReviewed by:
Baiyan Sui, Shanghai Jiao Tong University School of Medicine, ChinaZhen Geng, Shanghai University, China
Copyright © 2023 Liang, Lu, Zheng, Li, Geng, Sun, Cai, Jia, Jiang and Liu. This is an open-access article distributed under the terms of the Creative Commons Attribution License (CC BY). The use, distribution or reproduction in other forums is permitted, provided the original author(s) and the copyright owner(s) are credited and that the original publication in this journal is cited, in accordance with accepted academic practice. No use, distribution or reproduction is permitted which does not comply with these terms.
*Correspondence: Heng Bo Jiang, aGVuZ2JvamlhbmdAdmlwLnFxLmNvbQ==; Kai Liu, bGl1a2FpZmFuY3lAMTYzLmNvbQ==
†These authors have contributed equally to this work