- 1Department of Chemistry, Navyug Kanya Mahavidyalaya, University of Lucknow, Lucknow, India
- 2Department of Chemistry, Institute of Science and Research (ISR), IPS Academy, Indore, India
- 3Centre for Applied Chemistry, School of Applied Material Sciences, Central University of Gujarat, Gujarat, India
- 4Department of Life Sciences, Hemchandracharya North Gujarat University, Patan, India
- 5School of Chemical Sciences, Swami Ramanand Teerth Marathwada University, Nanded, India
- 6Department of Chemistry, Medicaps University, Indore, India
- 7Department of Veterinary Clinical Sciences, College of Veterinary Medicine, Iowa State University, Ames, IA, United States
4-Dimensional Printing (4DP) is the latest concept in the pharmacy and biomedical segment with enormous potential in dosage from personalization and medication designing, which adopts time as the fourth dimension, giving printed structures the flexibility to modify their morphology. It can be defined as the fabrication in morphology with the help of smart/intelligent materials like polymers that permit the final object to alter its properties, shape, or function in response to external stimuli such as heat, light, pH, and moisture. The applications of 4DP in biomedicines and healthcare are explored with a focus on tissue engineering, artificial organs, drug delivery, pharmaceutical and biomedical field, etc. In the medical treatments and pharmaceutical field 4DP is paving the way with unlimited potential applications; however, its mainstream use in healthcare and medical treatments is highly dependent on future developments and thorough research findings. Therefore, previous innovations with smart materials are likely to act as precursors of 4DP in many industries. This review highlights the most recent applications of 4DP technology and smart materials in biomedical and healthcare fields which can show a better perspective of 4DP applications in the future. However, in view of the existing limitations, major challenges of this technology must be addressed along with some suggestions for future research. We believe that the application of proper regulatory constraints with 4DP technology would pave the way for the next technological revolution in the biomedical and healthcare sectors.
1 Introduction
The term “4D Printing” (4DP) was coined firstly by Skylar Tibbits in 2014 (Tibbits, 2014) (Raviv D., 2014). Though this is just the inception to explore the potential of 3-Dimensional Printing (3DP) and 4DP in pharmaceuticals and healthcare, innovation is believed to be a non-stop process (Zema et al., 2017; Awad et al., 2018; Trenfield et al., 2018). Advancements are continuously being done in 3DP in overall potential including speed, accuracy, multiple materials, and fabrication accuracy. 3DP though has several applications in the biomedical field but also has some inherent weaknesses as it focuses on the manufacturing of static structures which are highly rigid in nature, retaining the shape of the originally printed structure and performing only a single function (Kuang et al., 2019; Wadher et al., 2021). Biomedical and Pharmaceutical applications require dynamic functions that cannot be fulfilled by 3DP technology. With the progression of biomedical nanotechnology (Gacem et al., 2022; Yadav et al., 2023b), researchers are now focusing on stimuli-responsive materials (smart polymers) which were beneficial in the development of drug delivery systems. These stimuli-responsive materials (smart materials) have the characteristic feature to respond to specific external stimuli when applied. These materials have the unique feature of showing pre-programmed responses and are therefore highly suitable for intelligent applications (Tan et al., 2019).
4DP is found to be the most remarkable transformation in the existing 3DP technique and is an exemplar of the additive manufacturing (AM) technique. 4DP is another smart technology to design flexible and dynamic structures and overcome the limitations of 3DP technology (Khoo et al., 2015). It is crucial to have information about 3DP, its history, and techniques of printing as it will help us to understand the latest concept of smart 4DP and its discovery in a better manner. Many simple and complex materials have been printed recently, by using Computer Aided Design (CAD) through 3DP technique under the overall control of a computer. 3DP has attained great interest among industries and academic institutions due to its high speed, great accuracy, and cheaper cost of production. 3DP is a multidisciplinary approach that collaborates various streams of sciences such as material, engineering science, mechanical & data processing, etc. 3DP is a unique technique by which complex structures can be designed which is not possible by conventional techniques (Melchels et al., 2012; Libonati et al., 2016; Choong et al., 2020; Nishiguchi et al., 2020). Successful discoveries have been done in the fields of remotely actuated robots (Maccurdy et al., 2016), bio-inspired designs (Wegst et al., 2015; Gu et al., 2017), micro-environments and tissues (Zhu et al., 2016) and drug delivery systems. 3DP has proven to be an intelligent and smart technology in the biomedical and other industrial sectors though 4DP is now in its infancy, but it has the super potential to transform the manufacturing industry in future. The basics of 4DP are depicted in Figure 1.
2 4D printing
A research group at MIT first conceptualized the idea of the 4DP of objects (Zhang Z. et al., 2019). The new era is getting more and more advanced due to progressive and continuous growth in different areas of science and technology. 4DP was found to be a dynamic shift in smart manufacturing techniques and is considered to be the most commendable transformation in the 3DP technique. Basically, 4DP can fabricate the 3DP materials by eternal stimuli. 4DP can create those products which can change their morphology in response to an external stimulus such as light, heat, temperature, pH, or water. The basic process involved in 3DP & 4DP techniques are very similar but the variation in 4D printed products is the use of smart materials for the design which is adaptable (Choi et al., 2015). This 4DP process demonstrates a quantum jump in AM smart techniques (André, 2017) which offers a medium to convert notions into reality with functionality that is driven by the performance. With the 4D technique, a large range of materials can be produced which are actively programmable and capable of self-transforming into another shape (Ahmed et al., 2021).
There are three salient features of 4DP that must be satisfied. First is the “selection of composite material” (or intelligent material) which should be responsive to external stimuli, when given. The second is the “external stimulus” which when added to the material makes the object animated. Last is the “time taken by the object to get stimulated” (Pei, 2014). Therefore, the 4DP method is an amazing addition of 3D printers, well-programmed design, and smart materials (Momeni et al., 2017; Shin et al., 2017; Wu et al., 2018). This technique is capable of reducing manufacturing labor costs to the minimum level. Massive products can be manufactured in 3D printers and only be used when exposed to the environment. The industry believes that surgical equipment, biomaterials, and nanotechnology are the most promising healthcare fields in which 4DP are most likely to be implemented (Hu et al., 2021). Latest advancements in the field of material science have produced many smart/intelligent polymeric materials for 4DP techniques that have the potential to self-assemble or deform themselves in response to an external stimulus such as UV light, temperature, magnetic and electric current, pH, etc. (Zhang et al., 2022).
There are many advantages of 4DP technology such as it is highly cost-efficient, time-efficient, less error-prone, highly productive, and sensitive (Tibbits, 2014). There is an increasing interest in studying 4DP technology in responsive structures such as printed actuators, soft robotics in medical devices (Bakarich et al., 2015), smart textiles, and aerospace (Fulcher et al., 2010). 4DP explores the latest branch from AM which explores the future possibilities for both 3DP and 4DP technologies.
3 Important factors required for 4D printing
4DP is highly dependent on five factors these are equipment/3D printers, stimulus-responsive material, stimuli, interaction mechanism, and the types of AM processes (Momeni et al., 2017; Zhang Z. et al., 2019). The AM process does not need any tool and it allows the production of printing material from the command received from the software. There are many types of AM processes such as Selective Laser Sintering (SLS), Selective Laser Melting (SLM), Stereolithography (SLA), Fused Deposition Modeling (FDM), Direct Ink Writing (DIW), Electron Beam Melting (EBM), etc. Most of the processes can print 4D material if, printing is in agreement with the printer. Another factor is the intelligent or smart stimuli-responsive materials used for advanced 4DP. The capability of the smart materials to self-transform themselves against external stimuli showed the strength of the 4DP technique. The next factor is the physical stimuli (temperature, light, moisture, magnetic energy (Yadav et al., 2020; 2023a), electric energy, Ultraviolet light (Modi et al., 2023b), etc., and chemical or biological) that are used in 4DP. The chemical stimuli cover the chemicals, pH level, etc. and the biological stimuli are the enzymes and glucose. Other factors are mathematical modeling and the mechanism of interaction. When an external stimulus is given to a programmable material it has the tendency to undergo the required transformation. It is important to plan the duration of mathematical modeling for which the stimulus will act on the programmable material. In Figure 2 all the factors are depicted which are responsible for 4DP.
4 Promising smart materials used for 4DP
Generally, the materials used for 4DP are called smart or intelligent materials because these are capable to change their properties with time (Leist and Zhou, 2016). Further intelligent materials are not defined universally and can sense the environmental stimulus and produce a useful response. This also includes conventional sensing materials such as piezoelectric materials which several researchers categorize as “intelligent” (Liu K. et al., 2021). Smart materials contribute an important and significant role in 4DP by acquiring, performing, and operating the given stimulus (Haleem et al., 2021). These sustainable materials respond to the given stimulus by performing the actuation, the shape-morphing, or functional modifications resulting in a complete change in the morphology (Invernizzi et al., 2018). Intelligent materials show unquestionable diverse features which can be exploited in products and structures such as responses to external stimuli like self-sensing, self-healing (automated recovery), self-actuating, self-diagnostic, and shape-changing (Liu et al., 2020). Therefore, smart or intelligent materials are those materials that provide a means of attaining an active and intelligent response in a product and have the potential to produce a myriad of increased functionalities. External stimuli are considered to be the biofuel of 4D printing technology (Pingale et al., 2023). A desirable response to external stimuli of the object is acquired by exploiting the physical properties of the printing materials. Therefore, the selection of smart material is highly dependent on the utility and employment of the object printed by 4DP (Ameta et al., 2022). Various smart materials and their applications have been discussed in Table 1 and Figure 3.
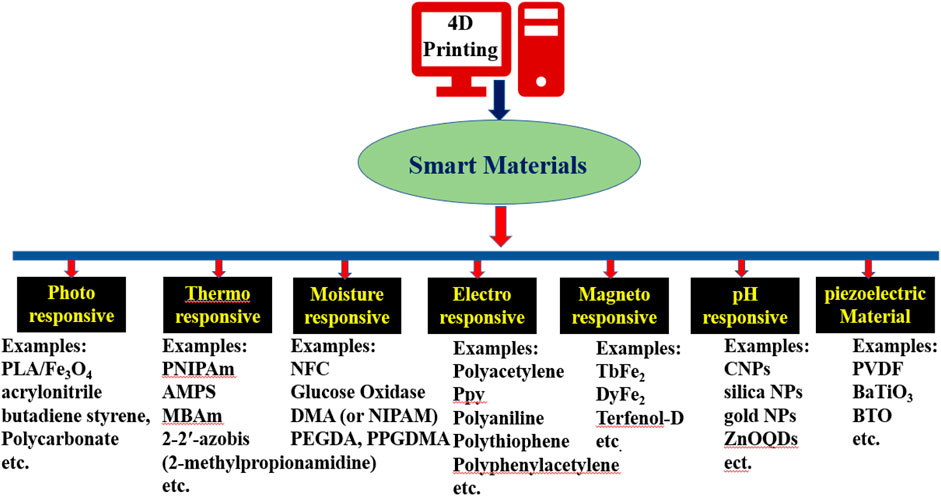
FIGURE 3. Stimuli-responsive smart materials used in 4D printing technology (Sun, 2015; Zhang F. et al., 2019; Farid et al., 2021; Chu et al., 2022). PLA, poly (lactic acid); PNIPAm or NIPAM: Poly (N-isopropylacrylamide); (AMPS), 2-Acrylamido-2-methylpropane sulfonic acid; MBAm, N,N′-Methylenebisacrylamide; NFC, nanofibrillated cellulose; DMA, Dimethylacetamide; PEGDA: poly (ethylene glycol) diacrylate; PPGDMA, poly (propylene dlycol) dimethacrylate;PPy, Polypyrrole; CNPs, Nanostructured carbon nanoparticles; ZNOQDs, surface-modified zinc oxide quantum dots; PVDF, piezoelectric polyvinylidene fluoride; BTO: Barium titanate.
5 4DP applications
Many recent studies have explored a nascent field that integrates therapeutics with 3D and 4D printing. As a result, many formulation concepts and pharmaceutical devices have emerged that can be printed and possibly tailored to an individual. Traditional production is being replaced by rapidly expanding 4DP technology in a wide range of industries that includes medical engineering, healthcare (Yadav et al., 2022), automobile, electronics, textiles, aerospace engineering, defense (Yadav et al., 2023c), advanced 4DP software, etc. (Sahafnejad-Mohammadi et al., 2022). Different applications and a complete overview of 4DP are shown in Figure 4.
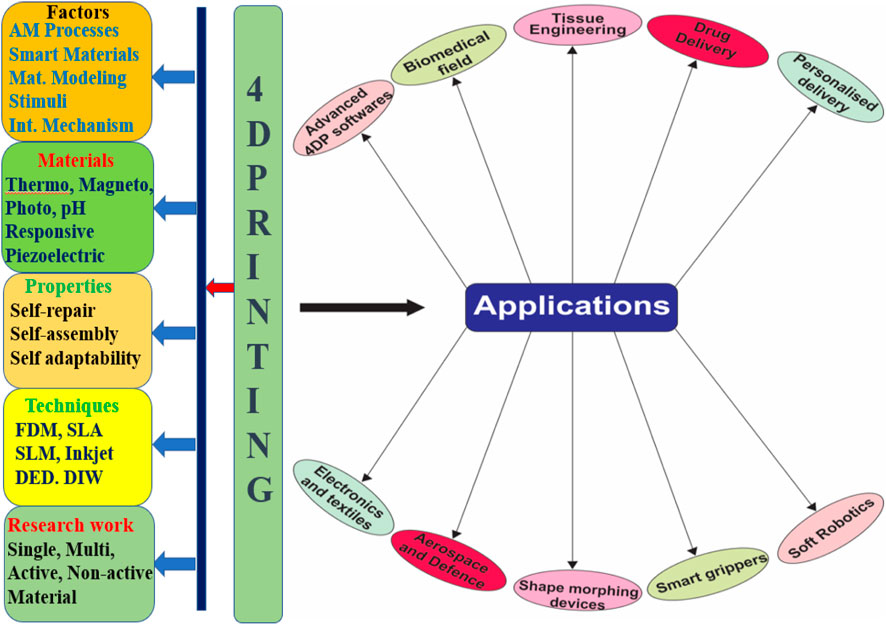
FIGURE 4. An overview of 4D printing technology and its applications. AM, Additive Manufacturing; FDM, Fused Deposition Modeling; SLA, stereolithography apparatus; SLM, Selective laser melting; DED, Direct energy deposition; DIW, Direct-Ink-Writing.
5.1 Opportunities in biomedical and healthcare
In medical devices and healthcare, a growing interest is seen in 4DP which explores a new arena of additive manufacturing research that has the potential to explore both 3DP and 4DP technologies. 4DP has a great advantage for diversified biomedical and healthcare applications, such as tissue engineering, bio-actuators, biosensors, and robotics (Liu B. et al., 2023). The medical and pharmaceutical sectors may be a potential field where 4DP objects can do miracles through biomedical applications of 4D printed objects dependent on chosen printing techniques, parameters, and the desired final usage (Choudhary et al., 2023a). Through a small incision, 4DP stents can be grafted into the body at the desired location, the shape can also be changed with the application of external stimuli and get useful results (Guo et al., 2023). 4D printing is a unique and developing smart technology that may add a novel approach to personalized medication designing and site-specific drug delivery concepts. This technique may take micro-robotics and bio-printing to a higher level and can surely make our healthcare and medical system better and smarter.
Biocompatibility is an important issue in the fabrication of Bio-Medical (BM) devices. Another issue of great importance is fabricating high-resolution structures that remain stable in their temporary as well as permanent spatial arrangements (Wang Q. et al., 2022). Due to the limitations in the physical properties, a single smart material can’t show a perfect structure but these materials are printed by smart 4DP techniques, resulting in their thermo-mechanical behaviors that can be created to promote controlled shape-memory behavior of the desired final printed structure (Ge et al., 2016). Autonomous shape memory and printability in response to an external stimulus are the most important requirements of 4DP materials (Shin et al., 2017). Kokkinis and his co-workers focused their work on 4DP which is magnetically triggered (Kokkinis et al., 2015).
It is said that the 4DP technique works on 3DP fabricating materials made from intelligent materials which are able to fold/unfold themselves. The use of these smart materials can be divided into two mechanisms, i.e., bio-adhesion and encapsulation based on drug delivery. There is a great global concern among scientists about novel drug delivery approaches and 4DP has the potential to offer the new concept of fabrication. In the search for real medical applications, still, high-quality research is needed to apply the technology to consumable medications (Choudhary et al., 2023b).
5.1.1 Bioadhesion devices and drug delivery
An important application of 4D bio-printing is related to pharmaceuticals, bio-adhesion, and drug delivery systems where drugs or cells are encapsulated and then released under the influence of a particular stimulus (Trenfield et al., 2019). Efforts have been directed to develop responsive materials for being used in designing drug delivery systems for localized delivery of drugs and desired kinetics (Gazzaniga et al., 2023). The transdermal drug delivery system has greatly accelerated with microneedles that can penetrate transdermal delivery systems in a painless and convenient manner (Ma and Wu, 2017). A good example of painless drug administration through the skin was given by Liu et al., 2021. which has a hierarchical, limpet tooth-inspired architecture. Many stimuli-responsive systems can be used for targeted drug delivery and for achieving drug release, on demand. The surface properties of stimuli-responsive materials can also be modulated through intrinsic or extrinsic stimuli for enhancing penetration ability and improving cellular uptake (Li et al., 2019).
Bioadhesion devices are capable to initiate drug release by attaching themselves to the intestinal endothelium. According to a group of workers, this application develops a three-layered, mucoadhesive drug delivery system that is designed to work for sustained release formulation (Malachowski et al., 2014). Another bio-adhesive formulation using these materials is well-known multi-fingered thermo-responsive drug removal devices, also known as ‘theragrippers’ (Villar et al., 2011). Theragrippes are multilayered mini/microdevices with sharp microtips, which can latch onto the mucosal tissue in response to different kinds of stimuli. When exposed to temperatures above 32°C according to the predetermined design, it spontaneously grips onto the tissue as soon as introduced into the body at room temperature (Ghosh et al., 2020).
5.1.2 Encapsulation devices
Encapsulation devices can be created for controlled drug delivery due to the unique feature of 4D printed materials to self-fold or unfold themselves. For example, scientists have printed a multisome when exposed to a certain pH; released its inner content and the signal was measured with fluorescence microscopy (Villar et al., 2011). A microrobot device has been created that consisted of a hydrogel bilayer which was fabricated by conventional lithography (Li et al., 2016). Pharmaceutical contents &l living cells can also be printed by 4DP as researchers have fabricated pancreatic beta-cell and fibroblasts onto structures (Azam et al., 2011).
5.1.3 Biosensors, bioactuators and biorobotics
The 3D bioprinting (3DBP) technique is used to design biosensors (sensing devices in biological environments) previously. In recent years, implantable sensors have fetched revolutionary interest in healthcare that can provide continuous information for prolonged periods of time (Zhao et al., 2020). A transition from rigid and bulky devices to miniaturized flexible ones is seen with Hydrogels (as soft materials) to bridge the gap between soft biological systems and hard artificial machines (Ying and Liu, 2021).
A two-photon stereolithography approach has already been used to fabricate biosensors that are microcantilever-based, using polymers that are molecularly imprinted (Gomez et al., 2016) and the stereolithography technique has been used to make cantilevers that contain magnetic nonmaterial’s (Credi et al., 2016). These are useful to study the functions and nature of cells. 4DP bioactuators were investigated and fabricated by alginate hydrogel alginate hydrogels, which are able to withstand different temperatures (Bakarich et al., 2015). Another group printed a temporary shape airway stent which was converted back into a permanent shape by an increase in temperature (Zarek et al., 2017). 4DP has also got a great response in robotics and bioactuators which can be used for further advancement of smart technology. Various non-affordable materials can be fabricated by 4DP and used in the robotic industry. SM polyurethane micro-actuator with tunable properties was fabricated by a group of workers by 4DP (Hearon et al., 2015).
Self-healing pipes and hydrogels, and removing construction errors are some of the important applications of 4DP smart materials that will do wonders in the upcoming time. These 4DP materials are also used as sensors and printed artificial organs (Ahmed et al., 2021). Cells and tissues can be inserted into 4DP materials for providing mechanical forces for bioactuators/biorobots (Stanton et al., 2015). The biorobots can be used in drug delivery systems, difficult surgeries, and as therapeutic agents. In fact, certain difficulties and limitations in recent trials, such as gastric drug delivery systems, vascular stents, and muscle actuators have been worked out with 4DP (Yang et al., 2020). In orthopedics, 4DP can prove highly useable in the treatment of abnormalities due to their self-deformation.
5.1.4 Medical applications
To accomplish biological applications; 3DBP is applied for the substance transfer method to fabricate various living materials. Fully customized, complex structures can be printed by transforming elaborated medical images like X-rays, computed tomography (CT) scans, and magnetic resonance imaging (MRI) scans into 3D Computer-aided design (3D CAD) models for the printing apparatus (Javaid and Haleem, 2019; Wang H. et al., 2022). Recently, bioprinting by 4DP is more adopted by surgeons, in organ transplantation; as a result, this field has developed tremendously (Lu et al., 2022). There are some limitations associated with current tissue engineering which automatically led to the progression of the latest techniques called 3DBP or 4DBP. Another highly approachable biomedical concept used a PCL-diacrylate-based polymer which can be applied to craniomaxillofacial bone defects (Arabiyat et al., 2021).
As compared to conventional tissue engineering; bioprinting has many benefits and most importantly high precision in cell deposing, and fabrication of tissues with high quantities & formed of large tissue-engineered objects. Basically, 4DBP includes a biomaterial and the maturation of the 3DP skeleton using the biomaterial (Kuang et al., 2019). Researchers have fabricated a polymeric grid-patterned grafted bone and glazed it with human nasal inferior turbinate tissue to facilitate graft degradation. Investigations demonstrated the improved osteoinductive and osteoconductive properties of the graft but the lesser mechanical strength of the synthetic graft was a challenge as compared to the natural bones. Therefore, more experiments and improvisation are required before a real-life application (Montgomery et al., 2017).
5.1.5 Tissue engineering
3DP has fetched considerable attention in the biomedical field and tissue engineering (Kokkinis et al., 2015). Different kinds of biological structures (bone, liver tissue, blood vessels (Luan et al., 2022), heart issues, etc.) have been fabricated with 3DBP technologies (Yu et al., 2019). However, due to several limitations of 3DBP, the concept of 4DP has been applied in the field of tissue engineering. 3DP has been revolutionized by 4DP with shape and functional modification over time. The innovative 4DP technique has the potential to fabricate complex multilayer tissue constructs, thereby providing several advantages for tissue engineering and other applications (Miri et al., 2019). For tissue engineering applications a group of workers developed a new polycaprolactone (PCL) based formulation (Diedkova et al., 2023).
4DBP is required to attain such sprightly practice for designing critical and dynamic tissues, hierarchically (such as 4DBP, SM). Recent work presented the application of shape-memory scaffolds in the delivery of functional tissues that is minimally invasive (Gao et al., 2016). Another useful biomedical application of 4DBP in the rebirth of tissue and shipment of cells in a confined area in the body may be envisaged using the in-situ unfolding of 4DBP scaffolds. In the presence of biological moieties, the self-folding process can be done in response to external stimuli (Zhang and Lieber, 2016). To perform the delivery procedures in an accurate and wireless manner; biodegradable and bioelectronic devices can be integrated with scaffolds (Pati et al., 2015; Zhou et al., 2017). Similarly, cell traction forces have been utilized in another research work to make endothelialized tubes for 4D printed structures in a biomimetic way (Kuribayashi-Shigetomi et al., 2012). The potential benefits of 4DBP include high-resolution printing (different types of cells and excellent cell density tissues) and the capability to mass-produce tissue-engineering outputs (Chua and Yeong, 2015).
6 3D versus 4D: a comparative account
The fundamental difference between 3DP and 4DP technologies occurs in the materials that are used to fabricate certain objects. Alternatively, 4DP has added mathematics, stimulus, and interaction parts using smart material, which is entirely different from 3DP. The requirements of dynamic structures and their relevant application such as soft grippers (Georgopoulou et al., 2021), self-assembled space antennae (Momeni et al., 2017), and self-healing polymers (Hager et al., 2010) couldn’t be met by the conventional 3DP technology which can only fabricate static structures from commercial filaments. 4DP can solve this bottleneck issue and offers advantages over 3DP in several aspects, which mainly depend on the fast growth of smart materials and multi-material structures (Jacobsen, 2016). Although 3DP and 4DP have similar advantages, 4DP outperforms 3DP in the dynamic status of produced items, hence, 4DP technology may be revolutionary next-generation of additive manufacturing in this regard (Mohanta, 2018). 4DP offers a change in the printed configuration in response to external stimulus over time, therefore, 4D printed structures should be fully preprogrammed using time as another dimension. Figure 5 summarizes a comparative account between 3DP and 4DP technologies.
7 Limitations of 4DP
Although, 3DBP has tremendous growth due to multiple applications and advantages (especially in medical and healthcare sector) but, it has certain limitations also. 3DPB considers only the original state of the printed structure and considers it to be lifeless (Gao et al., 2016). Still, 4DP is in the initial stage and require more research and development attention to bring the industrial and manufacturing revolution to the medical and pharmaceutical fields. Since 4DP technique is based on smart materials, hence, more production and physical & chemical properties should be studied (Khalid et al., 2022). The challenge and problems related to designing the organs and surgical instruments and their implantations and transformation in the body is really very difficult task. Current 3D printers can’t address fundamental 3DP issues such as simultaneous printing of polymers and metals, avoiding support structures, especially for inaccessible internal structures, high-cost printable materials, and slow print times. Therefore, in order to achieve the aims of 4DP, 3DP must be optimized on the basis of its use in 4DP. To address 3DP challenges, 5-axis 3D printing is currently of high interest (Zhang F. et al., 2019).
The lack of special software for 4DP is another significant limitation because most of the available software (such as design and slicing software) has been prepared for use in 3DP. To meet the requirements of 4DP, more powerful software has to be introduced. There are limitations in selecting smart materials that are used in the biological field like tissue engineering and tissue regeneration because of the limited number of intelligent materials that react to specific stimuli. Limited material availability, slow and inaccurate actuation, etc. are other challenges that need to be addressed. Though, 4DP technology has already proved its efficiency in multiple fields including medical engineering and with the development of inexpensive, high-accuracy printing devices, and most significantly, with the finding of novel smart bio-materials it is predicted to gain its top prospect soon.
8 4DP healthcare market
Technological advancements have brought revolutions in diagnostic and treatment methods. The growing need for advanced technology boosts investments in this field and is further propelling market growth. Consequently, the 4DP healthcare market is growing continuously and it is expected to reach USD 32.8 million in 2028 and CAGR will reach upto 26.7%. Different government and semi-government agencies are working and promoting this smart technology in various developed and developing countries. 4DP has wide applications in different sectors like automobiles, aviation, textiles (Patel et al., 2022), manufacturing industries, military operations, shoes, and fashion industries along with potential applications in healthcare and biomedical fields (Modi et al., 2022).
A driving factor for the use of 4DP in the healthcare field is the growing demand for organ transplants which requires functional implants in the area of tissue engineering and biomaterials. The enormous growth of 4DP is also due to its involvement in complicated cancer chemotherapies and surgical techniques due to the lesser use of surgical techniques (Feng et al., 2023). Also, targeted drug delivery systems use 4DP which could be utilized for delivering the mixture of pharmaceutical drugs in such a way that they act only on a targeted body site. Thus, the 4DP technology has paved the way for the medical field by making new inventions in drug delivery systems and improving the efficiency of medical treatments. It is rapidly replacing the conventional methods of manufacturing medical devices and offering greater functionality and advanced treatment (Melocchi et al., 2021).
9 Conclusion
4DP has grown tremendously since its inception and has proved its influence in different manufacturing & industrial sectors along with healthcare. Despite being a novel technology, 4DP has potential opportunities that are acknowledged by several experts in the field. 4DBP technology has gained lots of attention in biomedical and healthcare applications due to the development of stimulus-responsive biomaterials and tissue regeneration. Further advancement of 4DP will explore the tremendous applications in the biomedical field and would meet the upgraded medical requirements. The latest and highly effective methods of incorporating electronics into smart structures may result in the betterment of novel intelligent devices. Conclusively, 4DBP has an encouraging and hopeful future as a potential technology to copy traditional cellular structures. Therefore, it is expected that 4DP will certainly bring a bright future and revolution into different manufacturing and design industries and a paradigm shift to the plethora of undiscovered bigness that will make our future bright, smarter, and much more convenient. At the same time, more studies are required to resolve the existing challenges before 4DP serves as a powerful and a sustainable technique on a global level.
Author contributions
All authors listed have made a substantial, direct, and intellectual contribution to the work and approved it for publication.
Acknowledgments
We are thankful to the Department of Chemistry, Navyug Kanya Mahavidyalaya, Lucknow and ISR, PS Academy, Indore, INDIA for providing basic facilities. The authors are also thankful to Department of Life Sciences, Hemchandracharya North Gujarat University, Patan, Gujarat, India.
Conflict of interest
The authors declare that the research was conducted in the absence of any commercial or financial relationships that could be construed as a potential conflict of interest.
Publisher’s note
All claims expressed in this article are solely those of the authors and do not necessarily represent those of their affiliated organizations, or those of the publisher, the editors and the reviewers. Any product that may be evaluated in this article, or claim that may be made by its manufacturer, is not guaranteed or endorsed by the publisher.
References
Ahmed, A., Arya, S., Gupta, V., Furukawa, H., and Khosla, A. (2021). 4D printing: fundamentals, materials, applications and challenges. Polym. Guildf. 228, 123926. doi:10.1016/j.polymer.2021.123926
Ali, I., Xudong, L., Xiaoqing, C., Zhiwei, J., Pervaiz, M., Weimin, Y., et al. (2019). A review of electro-stimulated gels and their applications: present state and future perspectives. Mater Sci. Eng. C Mater Biol. Appl. 103, 109852. doi:10.1016/j.msec.2019.109852
Ameta, K. L., Solanki, V. S., Singh, V., Devi, A. P., Chundawat, R. S., and Haque, S. (2022). Critical appraisal and systematic review of 3D & 4D printing in sustainable and environment-friendly smart manufacturing technologies. Sustain. Mater. Technol. 34, e00481. doi:10.1016/j.susmat.2022.e00481
André, J. C. (2017). From additive manufacturing to 3D/4D printing. 3, breakthrough innovations: programmable material, 4D printing and bio-printing. John Wiley & Sons.
Arabiyat, A. S., Pfau, M. R., Grunlan, M. A., and Hahn, M. S. (2021). Intrinsic osteoinductivity of PCL-DA/PLLA semi-IPN shape memory polymer scaffolds. J. Biomed. Mater Res. A 109, 2334–2345. doi:10.1002/jbm.a.37216
Awad, A., Trenfield, S. J., Gaisford, S., and Basit, A. W. (2018). 3D printed medicines: A new branch of digital healthcare. Int. J. Pharm. 548, 586–596. doi:10.1016/j.ijpharm.2018.07.024
Azam, A., Laflin, K. E., Jamal, M., Fernandes, R., and Gracias, D. H. (2011). Self-folding micropatterned polymeric containers. Biomed. Microdevices 13, 51–58. doi:10.1007/s10544-010-9470-x
Bakarich, S. E., Gorkin, R., in het Panhuis, M., and Spinks, G. M. (2015). 4D printing with mechanically robust, thermally actuating hydrogels. Macromol. Rapid Commun. 36, 1211–1217. doi:10.1002/marc.201500079
Behl, M., and Lendlein, A. (2007). Shape-memory polymers. Mater. Today 10, 20–28. doi:10.1016/S1369-7021(07)70047-0
Choi, J., Kwon, O. C., Jo, W., Lee, H. J., and Moon, M. W. (2015). 4D printing technology: A review. 3D Print Addit. Manuf. 2, 159–167. doi:10.1089/3dp.2015.0039
Choong, Y. Y. C., Maleksaeedi, S., Eng, H., Yu, S., Wei, J., and Su, P. C. (2020). High speed 4D printing of shape memory polymers with nanosilica. Appl. Mater Today 18, 100515. doi:10.1016/j.apmt.2019.100515
Choudhary, N., Dhingra, N., Gacem, A., Yadav, V. K., Verma, R. K., Choudhary, M., et al. (2023a). Towards further understanding the applications of endophytes: enriched source of bioactive compounds and bio factories for nanoparticles. Front. Plant Sci. 14, 1193573. doi:10.3389/fpls.2023.1193573
Choudhary, N., Tandi, D., Verma, R. K., Yadav, V. K., Dhingra, N., Ghosh, T., et al. (2023b). Towards further understanding the applications of endophytes: enriched source of bioactive compounds and bio factories for nanoparticles. Front. Plant Sci. 14, 1193573. doi:10.3389/fpls.2023.1193573
Chu, S., Shi, X., Tian, Y., and Gao, F. (2022). pH-responsive polymer nanomaterials for tumor therapy. Front. Oncol. 12, 855019. doi:10.3389/fonc.2022.855019
Chua, C., and Yeong, W. Y. (2015). Bioprinting: Principles and applications. World Scientific Publishing Company. doi:10.1142/9193
Credi, C., Fiorese, A., Tironi, M., Bernasconi, R., Magagnin, L., Levi, M., et al. (2016). 3D printing of cantilever-type microstructures by stereolithography of ferromagnetic photopolymers. ACS Appl. Mater Interfaces 8, 26332–26342. doi:10.1021/acsami.6b08880
Dai, S., Ravi, P., and Tam, K. C. (2008). pH-Responsive polymers: synthesis, properties and applications. Soft Matter 4, 435–449. doi:10.1039/B714741D
Diedkova, K., Pogrebnjak, A. D., Kyrylenko, S., Smyrnova, K., Buranich, V. V., Horodek, P., et al. (2023). Polycaprolactone–MXene nanofibrous scaffolds for tissue engineering. ACS Appl. Mater Interfaces 15, 14033–14047. doi:10.1021/acsami.2c22780
Erol, O., Pantula, A., Liu, W., and Gracias, D. H. (2019). Transformer hydrogels: A review. Adv. Mater Technol. 4, 1900043. doi:10.1002/admt.201900043
Farid, M. I., Wu, W., Liu, X., and Wang, P. (2021). Additive manufacturing landscape and materials perspective in 4D printing. Int. J. Adv. Manuf. Technol. 115, 2973–2988. doi:10.1007/s00170-021-07233-w
Feng, H., Yang, B., Wang, J., Liu, M., Yin, L., Zheng, W., et al. (2023). Identifying malignant breast ultrasound images using ViT-patch. Appl. Sci. Switz. 13, 3489. doi:10.3390/app13063489
Fulcher, J. T., Lu, Y. C., Tandon, G. P., and Foster, D. C. (2010). “Thermomechanical characterization of environmentally conditioned shape memory polymer using nanoindentation,” in Behavior and mechanics of multifunctional materials and composites 2010 (SPIE), 76440F. doi:10.1117/12.846974
Gacem, A., Modi, S., Yadav, V. K., Islam, S., Patel, A., Dawane, V., et al. (2022). Recent advances in methods for synthesis of carbon nanotubes and carbon nanocomposite and their emerging applications: A descriptive review. J. Nanomater 2022, 1–16. doi:10.1155/2022/7238602
Gao, B., Yang, Q., Zhao, X., Jin, G., Ma, Y., and Xu, F. (2016). 4D bioprinting for biomedical applications. Trends Biotechnol. 34, 746–756. doi:10.1016/j.tibtech.2016.03.004
Gazzaniga, A., Foppoli, A., Cerea, M., Palugan, L., Cirilli, M., Moutaharrik, S., et al. (2023). Towards 4D printing in pharmaceutics. Int. J. Pharm. X 5, 100171. doi:10.1016/j.ijpx.2023.100171
Ge, Q., Sakhaei, A. H., Lee, H., Dunn, C. K., Fang, N. X., and Dunn, M. L. (2016). Multimaterial 4D printing with tailorable shape memory polymers. Sci. Rep. 6, 31110. doi:10.1038/srep31110
Georgopoulou, A., Vanderborght, B., and Clemens, F. (2021). Fabrication of a soft robotic gripper with integrated strain sensing elements using multi-material additive manufacturing. Front. Robot. AI 8, 615991. doi:10.3389/frobt.2021.615991
Ghosh, A., Li, L., Xu, L., Dash, R. P., Gupta, N., Lam, J., et al. (2020). Gastrointestinal-resident, shape-changing microdevices extend drug release in vivo. Sci. Adv. 6 (44), eabb4133. doi:10.1126/sciadv.abb4133
Gomez, L. P. C., Spangenberg, A., Ton, X. A., Fuchs, Y., Bokeloh, F., Malval, J. P., et al. (2016). Rapid prototyping of chemical microsensors based on molecularly imprinted polymers synthesized by two-photon stereolithography. Adv. Mater. 28, 5931–5937. doi:10.1002/adma.201600218
Grinberg, D., Siddique, S., Le, M. Q., Liang, R., Capsal, J. F., and Cottinet, P. J. (2019). 4D Printing based piezoelectric composite for medical applications. J. Polym. Sci. B Polym. Phys. 57, 109–115. doi:10.1002/polb.24763
Gu, G. X., Libonati, F., Wettermark, S. D., and Buehler, M. J. (2017). Printing nature: unraveling the role of nacre’s mineral bridges. J. Mech. Behav. Biomed. Mater 76, 135–144. doi:10.1016/j.jmbbm.2017.05.007
Guo, Q., Li, T., Qu, Y., Liang, M., Ha, Y., Zhang, Y., et al. (2023). New research development on trans fatty acids in food: biological effects, analytical methods, formation mechanism, and mitigating measures. Prog. Lipid Res. 89, 101199. doi:10.1016/j.plipres.2022.101199
Habault, D., Zhang, H., and Zhao, Y. (2013). Light-triggered self-healing and shape-memory polymers. Chem. Soc. Rev. 42, 7244–7256. doi:10.1039/C3CS35489J
Hager, M. D., Greil, P., Leyens, C., Van Der Zwaag, S., and Schubert, U. S. (2010). Self-healing materials. Adv. Mater 22, 5424–5430. doi:10.1002/adma201003036
Haleem, A., Javaid, M., Singh, R. P., and Suman, R. (2021). Significant roles of 4D printing using smart materials in the field of manufacturing. Adv. Industrial Eng. Polym. Res. 4, 301–311. doi:10.1016/j.aiepr.2021.05.001
Hearon, K., Wierzbicki, M. A., Nash, L. D., Landsman, T. L., Laramy, C., Lonnecker, A. T., et al. (2015). A processable shape memory polymer system for biomedical applications. Adv. Healthc. Mater 4, 1386–1398. doi:10.1002/adhm.201500156
Hu, F., Shi, X., Wang, H., Nan, N., Wang, K., Wei, S., et al. (2021). Is health contagious? based on empirical evidence from China family panel studies’ data. Front. Public Health 9, 691746. doi:10.3389/fpubh.2021.691746
Invernizzi, M., Turri, S., Levi, M., and Suriano, R. (2018). 4D printed thermally activated self-healing and shape memory polycaprolactone-based polymers. Eur. Polym. J. 101, 169–176. doi:10.1016/j.eurpolymj.2018.02.023
Jacobsen, M. (2016). “Clearing the way for pivotal 21st-century innovation,” in Giftedness and talent in the 21st century: Adapting to the turbulence of globalization. Editors D. Ambrose,, and R. J. Sternberg (Rotterdam: SensePublishers), 163–179. doi:10.1007/978-94-6300-503-6_10
Javaid, M., and Haleem, A. (2019). 4D printing applications in medical field: A brief review. Clin. Epidemiol. Glob. Health 7, 317–321. doi:10.1016/j.cegh.2018.09.007
Jeong, B., and Gutowska, A. (2002). Lessons from nature: stimuli-responsive polymers and their biomedical applications. Trends Biotechnol. 20, 305–311. doi:10.1016/S0167-7799(02)01962-5
Khalid, M. Y., Arif, Z. U., Ahmed, W., Umer, R., Zolfagharian, A., and Bodaghi, M. (2022). 4D printing: technological developments in robotics applications. Sens. Actuators A Phys. 343, 113670. doi:10.1016/j.sna.2022.113670
Khoo, Z. X., Teoh, J. E. M., Liu, Y., Chua, C. K., Yang, S., An, J., et al. (2015). 3D printing of smart materials: A review on recent progresses in 4D printing. Virtual Phys. Prototyp. 10, 103–122. doi:10.1080/17452759.2015.1097054
Kokkinis, D., Schaffner, M., and Studart, A. R. (2015). Multimaterial magnetically assisted 3D printing of composite materials. Nat. Commun. 6, 8643. doi:10.1038/ncomms9643
Kopeček, J., and Yang, J. (2012). Smart self-assembled hybrid hydrogel biomaterials. Angew. Chem. - Int. Ed. 51, 7396–7417. doi:10.1002/anie.201201040
Kuang, X., Roach, D. J., Wu, J., Hamel, C. M., Ding, Z., Wang, T., et al. (2019). Advances in 4D printing: materials and applications. Adv. Funct. Mater 29, 1805290. doi:10.1002/adfm.201805290
Kuribayashi-Shigetomi, K., Onoe, H., and Takeuchi, S. (2012). Cell origami: self-folding of three-dimensional cell-laden microstructures driven by cell traction force. PLoS One 7, e51085. doi:10.1371/journal.pone.0051085
Leist, S. K., and Zhou, J. (2016). Current status of 4D printing technology and the potential of light-reactive smart materials as 4D printable materials. Virtual Phys. Prototyp. 11, 249–262. doi:10.1080/17452759.2016.1198630
Li, C., Wang, J., Wang, Y., Gao, H., Wei, G., Huang, Y., et al. (2019). Recent progress in drug delivery. Acta Pharm. Sin. B 9, 1145–1162. doi:10.1016/j.apsb.2019.08.003
Li, H., Go, G., Ko, S. Y., Park, J. O., and Park, S. (2016). Magnetic actuated pH-responsive hydrogel-based soft micro-robot for targeted drug delivery. Smart Mater Struct. 25, 027001. doi:10.1088/0964-1726/25/2/027001
Libonati, F., Gu, G. X., Qin, Z., Vergani, L., and Buehler, M. J. (2016). Bone-inspired materials by design: toughness amplification observed using 3D printing and testing. Adv. Eng. Mater 18, 1354–1363. doi:10.1002/adem.201600143
Liu, B., Peng, Y., Jin, Z., Wu, X., Gu, H., Wei, D., et al. (2023a). Terahertz ultrasensitive biosensor based on wide-area and intense light-matter interaction supported by QBIC. Chem. Eng. J. 462, 142347. doi:10.1016/j.cej.2023.142347
Liu, C., Wang, Z., Wei, X., Chen, B., and Luo, Y. (2021a). 3D printed hydrogel/PCL core/shell fiber scaffolds with NIR-triggered drug release for cancer therapy and wound healing. Acta Biomater. 131, 314–325. doi:10.1016/j.actbio.2021.07.011
Liu, K., Tebyetekerwa, M., Ji, D., and Ramakrishna, S. (2020). Intelligent materials. Matter 3, 590–593. doi:10.1016/j.matt.2020.07.003
Liu, K., Zhang, Q., Zhou, C., Shi, Y., Sun, C., Sun, H., et al. (2021b). 4D printing of lead zirconate titanate piezoelectric composites transducer based on Direct Ink writing. Front. Mater 8, 659441. doi:10.3389/fmats.2021.659441
Liu, Y., Shaw, B., Dickey, M. D., and Genzer, J. (2023b). Sequential self-folding of polymer sheets. Sci. Adv. 3, e1602417. doi:10.1126/sciadv.1602417
Lu, S., Yang, J., Yang, B., Yin, Z., Liu, M., Yin, L., et al. (2022). Analysis and design of surgical instrument localization algorithm. Comput. Model. Eng. Sci. 137, 669–685. doi:10.32604/cmes.2023.027417
Luan, D., Liu, A., Wang, X., Xie, Y., and Wu, Z. (2022). Robust two-stage location allocation for emergency temporary blood supply in postdisaster. Discrete Dyn. Nat. Soc. 2022, 1–20. doi:10.1155/2022/6184170
Ma, G., and Wu, C. (2017). Microneedle, bio-microneedle and bio-inspired microneedle: A review. J. Control. Release 251, 11–23. doi:10.1016/j.jconrel.2017.02.011
Maccurdy, R., Katzschmann, R., Kim, Y., and Rus, D. (2016). “Printable hydraulics: a method for fabricating robots by 3D Co-printing solids and liquids,” in 2016 IEEE International Conference on Robotics and Automation, Stockholm, Sweden, 16-21 May 2016, 3–9.
Malachowski, K., Breger, J., Kwag, H. R., Wang, M. O., Fisher, J. P., Selaru, F. M., et al. (2014). Stimuli-responsive theragrippers for chemomechanical controlled release. Angew. Chem. - Int. Ed. 53, 8045–8049. doi:10.1002/anie.201311047
Melchels, F. P. W., Domingos, M. A. N., Klein, T. J., Malda, J., Bartolo, P. J., and Hutmacher, D. W. (2012). Additive manufacturing of tissues and organs. Prog. Polym. Sci. 37, 1079–1104. doi:10.1016/j.progpolymsci.2011.11.007
Melocchi, A., Uboldi, M., Cerea, M., Foppoli, A., Maroni, A., Moutaharrik, S., et al. (2021). Shape memory materials and 4D printing in pharmaceutics. Adv. Drug Deliv. Rev. 173, 216–237. doi:10.1016/j.addr.2021.03.013
Miri, A. K., Khalilpour, A., Cecen, B., Maharjan, S., Shin, S. R., and Khademhosseini, A. (2019). Multiscale bioprinting of vascularized models. Biomaterials 198, 204–216. doi:10.1016/j.biomaterials.2018.08.006
Modi, S., Inwati, G. K., Gacem, A., Saquib Abullais, S., Prajapati, R., Yadav, V. K., et al. (2022). Nanostructured antibiotics and their emerging medicinal applications: an overview of nanoantibiotics. Antibiotics 11, 708. doi:10.3390/antibiotics11060708
Modi, S., Yadav, V. K., Amari, A., Alyami, A. Y., Gacem, A., Harharah, H. N., et al. (2023a). Photocatalytic degradation of methylene blue dye from wastewater by using doped zinc oxide nanoparticles. Water (Basel) 15, 2275. doi:10.3390/w15122275
Modi, S., Yadav, V. K., Amari, A., Osman, H., Igwegbe, C. A., and Fulekar, M. H. (2023b). Nanobioremediation: a bacterial consortium-zinc oxide nanoparticle-based approach for the removal of methylene blue dye from wastewater. Environ. Sci. Pollut. Res. 30, 72641–72651. doi:10.1007/s11356-023-27507-y
Mohanta, K. (2018). 4D printing -will it be a game-changer? Res. Dev. Material Sci. 5. doi:10.31031/RDMS.2018.05.000602
Momeni, F., Hassani, S. M. M., Liu, X., and Ni, J. (2017). A review of 4D printing. Mater Des. 122, 42–79. doi:10.1016/J.MATDES.2017.02.068
Montgomery, M., Ahadian, S., Davenport Huyer, L., Lo Rito, M., Civitarese, R. A., Vanderlaan, R. D., et al. (2017). Flexible shape-memory scaffold for minimally invasive delivery of functional tissues. Nat. Mater 16, 1038–1046. doi:10.1038/nmat4956
Nadgorny, M., Xiao, Z., Chen, C., and Connal, L. A. (2016). Three-Dimensional printing of pH-responsive and functional polymers on an affordable desktop printer. ACS Appl. Mater Interfaces 8, 28946–28954. doi:10.1021/acsami.6b07388
Nichterwitz, M., Honnali, S., Kutuzau, M., Guo, S., Zehner, J., Nielsch, K., et al. (2021). Advances in magneto-ionic materials and perspectives for their application. Apl. Mater 9. doi:10.1063/5.0042544
Nishiguchi, A., Zhang, H., Schweizerhof, S., Schulte, M. F., Mourran, A., and Möller, M. (2020). 4D printing of a light-driven soft actuator with programmed printing density. ACS Appl. Mater Interfaces 12, 12176–12185. doi:10.1021/acsami.0c02781
Patel, H., Yadav, V. K., Yadav, K. K., Choudhary, N., Kalasariya, H., Alam, M. M., et al. (2022). A recent and systemic approach towards microbial biodegradation of dyes from textile industries. Water 14, 3163. doi:10.3390/w14193163
Pati, F., Song, T. H., Rijal, G., Jang, J., Kim, S. W., and Cho, D. W. (2015). Ornamenting 3D printed scaffolds with cell-laid extracellular matrix for bone tissue regeneration. Biomaterials 37, 230–241. doi:10.1016/j.biomaterials.2014.10.012
Pei, E. (2014). 4D printing: dawn of an emerging technology cycle. Assem. Autom. 34, 310–314. doi:10.1108/AA-07-2014-062
Pingale, P., Dawre, S., Dhapte-Pawar, V., Dhas, N., and Rajput, A. (2023). Advances in 4D printing: from stimulation to simulation. Drug Deliv. Transl. Res. 13, 164–188. doi:10.1007/s13346-022-01200-y
Sahafnejad-Mohammadi, I., Karamimoghadam, M., Zolfagharian, A., Akrami, M., and Bodaghi, M. (2022). 4D printing technology in medical engineering: a narrative review. J. Braz. Soc. Mech. Sci. Eng. 44, 233. doi:10.1007/s40430-022-03514-x
Schmaljohann, D. (2006). Thermo- and pH-responsive polymers in drug delivery. Adv. Drug Deliv. Rev. 58, 1655–1670. doi:10.1016/j.addr.2006.09.020
Shiblee, M. D. N. I., Ahmed, K., Khosla, A., Kawakami, M., and Furukawa, H. (2018). 3D printing of shape memory hydrogels with tunable mechanical properties. Soft Matter 14, 7809–7817. doi:10.1039/C8SM01156G
Shin, D.-G., Kim, T.-H., and Kim, D.-E. (2017). Review of 4D printing materials and their properties. Int. J. Precis. Eng. Manufacturing-Green Technol. 4, 349–357. doi:10.1007/s40684-017-0040-z
Soppimath, K. S., Aminabhavi, T. M., Dave, A. M., Kumbar, S. G., and Rudzinski, W. E. (2002). Stimulus-responsive “smart” hydrogels as novel drug delivery systems. Drug Dev. Ind. Pharm. 28, 957–974. doi:10.1081/DDC-120006428
Sponchioni, M., Capasso Palmiero, U., and Moscatelli, D. (2019). Thermo-responsive polymers: applications of smart materials in drug delivery and tissue engineering. Mater Sci. Eng. C Mater Biol. Appl. 102, 589–605. doi:10.1016/j.msec.2019.04.069
Stanton, M. M., Trichet-Paredes, C., and Sánchez, S. (2015). Applications of three-dimensional (3D) printing for microswimmers and bio-hybrid robotics. Lab. Chip 15, 1634–1637. doi:10.1039/c5lc90019k
Sun, B.-H. (2015). Smart materials and structures. Cape Town, South Afica: Cape Peninsula Univeresity of Technology.
Tan, D., Nokhodchi, A., and Maniruzzaman, M. (2019). “3D and 4D printing technologies: innovative process engineering and smart additive manufacturing,” in 3D and 4D printing in biomedical applications (Wiley), 25–52. doi:10.1002/9783527813704.ch2
Tibbits, S. (2014). 4D printing: multi-material shape change. Archit. Des. 84, 116–121. doi:10.1002/ad.1710
Trenfield, S. J., Awad, A., Goyanes, A., Gaisford, S., and Basit, A. W. (2018). 3D printing pharmaceuticals: drug development to frontline care. Trends Pharmacol. Sci. 39, 440–451. doi:10.1016/j.tips.2018.02.006
Trenfield, S. J., Awad, A., Madla, C. M., Hatton, G. B., Firth, J., Goyanes, A., et al. (2019). Shaping the future: recent advances of 3D printing in drug delivery and healthcare. Expert Opin. Drug Deliv. 16, 1081–1094. doi:10.1080/17425247.2019.1660318
Ullakko, K. (1996). Magnetically controlled shape memory alloys: A new class of actuator materials. J. Mater Eng. Perform. 5, 405–409. doi:10.1007/BF02649344
Villar, G., Heron, A. J., and Bayley, H. (2011). Formation of droplet networks that function in aqueous environments. Nat. Nanotechnol. 6, 803–808. doi:10.1038/nnano.2011.183
Wadher, K., Trivedi, R., Wankhede, N., Kale, M., and Umekar, M. (2021). 3D printing in pharmaceuticals: an emerging technology full of challenges. Ann. Pharm. Fr. 79, 107–118. doi:10.1016/j.pharma.2020.08.007
Wang, H., Wang, K., Xue, Q., Peng, M., Yin, L., Gu, X., et al. (2022a). Transcranial alternating current stimulation for treating depression: a randomized controlled trial. Brain 145, 83–91. doi:10.1093/brain/awab252
Wang, Q., Guo, Q., Niu, W., Wu, L., Gong, W., Yan, S., et al. (2022b). The pH-responsive phase separation of type-A gelatin and dextran characterized with static multiple light scattering (S-MLS). Food Hydrocoll. 127, 107503. doi:10.1016/j.foodhyd.2022.107503
Wegst, U. G. K., Bai, H., Saiz, E., Tomsia, A. P., and Ritchie, R. O. (2015). Bioinspired structural materials. Nat. Mater 14, 23–36. doi:10.1038/nmat4089
Wu, J.-J., Huang, L.-M., Zhao, Q., and Xie, T. (2018). 4D printing: history and recent progress. Chin. J. Polym. Sci. 36, 563–575. doi:10.1007/s10118-018-2089-8
Yadav, V. K., Amari, A., Gacem, A., Elboughdiri, N., Eltayeb, L. B., and Fulekar, M. H. (2023a). Treatment of fly-ash-contaminated wastewater loaded with heavy metals by using fly-ash-synthesized iron oxide nanoparticles. Water (Basel) 15, 908. doi:10.3390/w15050908
Yadav, V. K., Choudhary, N., Inwati, G. K., Rai, A., Singh, B., Solanki, B., et al. (2023b). Recent trends in the nanozeolites-based oxygen concentrators and their application in respiratory disorders. Front. Med. (Lausanne) 10, 1147373. doi:10.3389/fmed.2023.1147373
Yadav, V. K., Malik, P., Tirth, V., Khan, S. H., Yadav, K. K., Islam, S., et al. (2022). Health and environmental risks of incense smoke: mechanistic insights and cumulative evidence. J. Inflamm. Res. 15, 2665–2693. doi:10.2147/jir.s347489
Yadav, V. K., Modi, T., Alyami, A. Y., Gacem, A., Choudhary, N., Yadav, K. K., et al. (2023c). Emerging trends in the recovery of ferrospheres and plerospheres from coal fly ash waste and their emerging applications in environmental cleanup. Front. Earth Sci. (Lausanne) 11. doi:10.3389/feart.2023.1160448
Yadav, V. K., Yadav, K. K., Gnanamoorthy, G., Choudhary, N., Khan, S. H., Gupta, N., et al. (2020). A novel synthesis and characterization of polyhedral shaped amorphous iron oxide nanoparticles from incense sticks ash waste. Environ. Technol. Innov. 20, 101089. doi:10.1016/j.eti.2020.101089
Yang, Q., Gao, B., and Xu, F. (2020). Recent advances in 4D bioprinting. Biotechnol. J. 15, 1900086. doi:10.1002/biot.201900086
Ying, B., and Liu, X. (2021). Skin-like hydrogel devices for wearable sensing, soft robotics and beyond. iScience 24, 103174. doi:10.1016/j.isci.2021.103174
Yu, C., Ma, X., Zhu, W., Wang, P., Miller, K. L., Stupin, J., et al. (2019). Scanningless and continuous 3D bioprinting of human tissues with decellularized extracellular matrix. Biomaterials 194, 1–13. doi:10.1016/j.biomaterials.2018.12.009
Zarek, M., Mansour, N., Shapira, S., and Cohn, D. (2017). 4D printing of shape memory-based personalized endoluminal medical devices. Macromol. Rapid Commun. 38, 1600628. doi:10.1002/marc.201600628
Zema, L., Melocchi, A., Maroni, A., and Gazzaniga, A. (2017). Three-Dimensional printing of medicinal products and the challenge of personalized therapy. J. Pharm. Sci. 106, 1697–1705. doi:10.1016/j.xphs.2017.03.021
Zhang, A., and Lieber, C. M. (2016). Nano-Bioelectronics. Chem. Rev. 116, 215–257. doi:10.1021/acs.chemrev.5b00608
Zhang, F., Wang, L., Zheng, Z., Liu, Y., and Leng, J. (2019a). Magnetic programming of 4D printed shape memory composite structures. Compos Part A Appl. Sci. Manuf. 125, 105571. doi:10.1016/j.compositesa.2019.105571
Zhang, Z., Demir, K. G., and Gu, G. X. (2019b). Developments in 4D-printing: a review on current smart materials, technologies, and applications. Int. J. Smart Nano Mater 10, 205–224. doi:10.1080/19475411.2019.1591541
Zhang, Z., Wang, L., Zheng, W., Yin, L., Hu, R., and Yang, B. (2022). Endoscope image mosaic based on pyramid ORB. Biomed. Signal Process Control 71, 103261. doi:10.1016/j.bspc.2021.103261
Zhao, Q., Li, C., Shum, H. C., and Du, X. (2020). Shape-adaptable biodevices for wearable and implantable applications. Lab. Chip 20, 4321–4341. doi:10.1039/D0LC00569J
Zhou, W., Dai, X., and Lieber, C. M. (2017). Advances in nanowire bioelectronics. Rep. Prog. Phys. 80, 016701. doi:10.1088/0034-4885/80/1/016701
Keywords: 4-Dimensional Printing, sustainability, biomedical application, smart materials, pharmaceutical applications, healthcare, 4D bioprinting
Citation: Agarwal N, Solanki VS, Ameta KL, Yadav VK, Gupta P, Wanale SG, Shrivastava R, Soni A, Sahoo DK and Patel A (2023) 4-Dimensional printing: exploring current and future capabilities in biomedical and healthcare systems—a Concise review. Front. Bioeng. Biotechnol. 11:1251425. doi: 10.3389/fbioe.2023.1251425
Received: 01 July 2023; Accepted: 10 August 2023;
Published: 22 August 2023.
Edited by:
Sudipto Datta, Indian Institute of Science (IISc), IndiaReviewed by:
Arindam Chakraborty, Indian Institute of Engineering Science and Technology, Shibpur, IndiaRanjit Barua, Indian Institute of Engineering Science and Technology, Shibpur, India
Copyright © 2023 Agarwal, Solanki, Ameta, Yadav, Gupta, Wanale, Shrivastava, Soni, Sahoo and Patel. This is an open-access article distributed under the terms of the Creative Commons Attribution License (CC BY). The use, distribution or reproduction in other forums is permitted, provided the original author(s) and the copyright owner(s) are credited and that the original publication in this journal is cited, in accordance with accepted academic practice. No use, distribution or reproduction is permitted which does not comply with these terms.
*Correspondence: Neha Agarwal, bmVoYWFnYXJ3YWw0MDc0QGdtYWlsLmNvbQ==; Vijendra Singh Solanki, dmlqZW5kcmFzaW5naDAwMThAZ21haWwuY29t; Dipak Kumar Sahoo, ZHNhaG9vQGlhc3RhdGUuZWR1; Ashish Patel, dW5pLmFzaGlzaEBnbWFpbC5jb20=