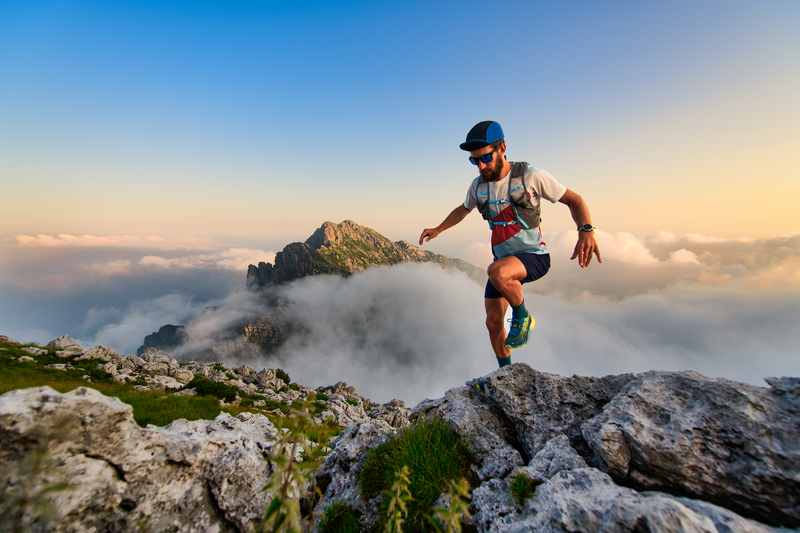
95% of researchers rate our articles as excellent or good
Learn more about the work of our research integrity team to safeguard the quality of each article we publish.
Find out more
MINI REVIEW article
Front. Bioeng. Biotechnol. , 16 November 2023
Sec. Synthetic Biology
Volume 11 - 2023 | https://doi.org/10.3389/fbioe.2023.1244377
Human transcriptome can undergo RNA mis-splicing due to spliceopathies contributing to the increasing number of genetic diseases including muscular dystrophy (MD), Alzheimer disease (AD), Huntington disease (HD), myelodysplastic syndromes (MDS). Intron retention (IR) is a major inducer of spliceopathies where two or more introns remain in the final mature mRNA and account for many intronic expansion diseases. Potential removal of such introns for therapeutic purposes can be feasible when utilizing bioinformatics, catalytic RNAs, and nano-drug delivery systems. Overcoming delivery challenges of catalytic RNAs was discussed in this review as a future perspective highlighting the significance of utilizing synthetic biology in addition to high throughput deep sequencing and computational approaches for the treatment of mis-spliced transcripts.
Various pathogenesis could result from spliceopathies, in which pre-mRNA undergoes a dysregulated-splicing process. Spliceosomes are the largest ribonucleoproteins that assemble around newly synthesized RNA transcripts in order to perform two distinctive trans-esterification reactions, which contribute to the precise removal of the intervening non-coding sequences (introns) followed by joining the coding regions (exons) during the transcription maturation step (Valadkhan and Manley, 2001). Along with the two-post transcriptional modification; 5′ capping and 3′ poly A tailing, splicing occurs in a highly coordinated manner to produce a fully functional messenger RNA (mRNA) ready to be translated into the protein of interest via the cytoplasmic ribosomes. Mammalian spliceosomes consist of five essential uridine-rich small nuclear RNAs (U1, U2, U4, U5 and U6 snRNAs), which recruit a massive number of auxiliary splicing factors (∼200 proteins) needed for initiating the splicing reaction (Rappsilber et al., 2002; Valadkhan et al., 2009; Suñé-Pou et al., 2020). Spliceosomes drive the alternative splicing (AS), which is a critical regulatory process contributing to the vast diversity of translated proteins in mammalian cells. For a given transcript, there are five major AS events that might take place; constitutive splicing, exon skipping pattern, mutually exclusive exons, alternative 5′ or 3′ splice site events (Graveley, 2001; Modrek and Lee, 2002; Yeo et al., 2005) and intron retention (IR) (Galante et al., 2004; Edwards et al., 2016; Rekosh and Hammarskjold, 2018).
Within the IR event, transcripts harbor two or more introns that failed to be spliced out from the mature mRNA due to spliceosomal dysfunction leading to spliceopathies (Monteuuis et al., 2019). Among all AS events, IR received a minimal attention and considered to be a rare event in which hydrolysis often occurs via the cellular degradation pathway. However, growing number of reports suggest that IR could affect 80% of the coding genes (Middleton et al., 2017), especially those involved in cell differentiation (Llorian et al., 2016) and cell cycling (Braunschweig et al., 2014; Llorian et al., 2016; Middleton et al., 2017). Per the cancer genomic atlas (TSGA) and the transcriptomic cancer studies, IR is considered the common AS mode among all cancer types as it accounts for the wide diversities in cancer transcriptomes (Supek et al., 2014; Dvinge and Bradley, 2015).
Interestingly, spliceosomal dysfunction is often caused by errors in the transesterification reactions of the cis-acting elements and/or trans-acting factors during the spliceosomal assembly. Chemically, transesterification is a type of SN2 nucleophilic substitution reactions where synchronously one of the ester bonds is broken and another ester bond is formed. In a typical splicing reaction, two consecutive reaction takes place in the nucleus (nuclear splicing) as follows: first, nucleophilic attack of the hydroxyl group at 2′ carbon atom of the branched adenosine located in the introns will results in releasing the first free 5′exon and 2′-5′ unusual phosphodiester bond formation between the hydroxyl group of the branched adenosine and 5′ phosphoryl group of the 5′ end of intron to form partial lariat structure in step commonly known as branching. Second step known as ligation which involves the cleavage at 3′ splice site done by the attack of the 3′ hydroxyl group of the 5′ exon and leads to joining of the exons together and release the intron (Horowitz and Abelson, 1993; Shi et al., 2018).
Consequently, mutations in both cis-acting elements and trans-acting factors could inevitably influence the functionality of spliceosome machinery leading to spliceosomopathies. For instance, alteration in the cis-acting elements such as enhancers and silencers significantly affects the catalytic reaction leading to mis-splicing (Scotti and Swanson, 2016; Anna and Monika, 2018). IR that results from mutations in the trans-acting factors can disrupt the activity of spliceosomes as well. For example, mutations occur in the most important component factors expressed in spliceosome (PRPF31, PRPF3 and PRPF8) lead to hereditary disease in the eye called retinitis pigmentosa (RP) that dramatically delayed the spliceosome assembly affecting the pre-mRNA splicing. Such mutations decreased the removal of ∼9% of the introns from coding genes not only from the retina of the eye, but other tissues such as lymphoblast (Tanackovic et al., 2011). Other mutations affecting multiple E/A splicing complex, namely, U2AF35, ZRSR2, SRSF2 and SF3B1, lead to myelodysplastic syndromes, which is a heterogeneous group of myeloid neoplasms that manifests bone marrow failure leading to acute myeloid leukemia (Yoshida et al., 2011). Another example of mutations in three important spliceosomal maintenance proteins (TDP-43, FUS/TLS, and SMN) cause profound loss of the spliceosomal integrity and lead to amyotrophic lateral sclerosis (ALS) and spinal muscular atrophy (SMA) (Tsuiji et al., 2013). Even though it is widely accepted that exons skipping during the splicing process is considered the most common patterns of AS and account for ∼60% of spliced transcripts (Sugnet et al., 2003; Dvinge and Bradley, 2015) it was reported in multiple cancer genomic studies that IRs were predominant in all analyzed cancer transcripts (Dvinge and Bradley, 2015). Four decades ago, when IR is discovered, it revealed new insights of its role in regulation of gene expression, pathogenesis, and treatment approaches (Kumari et al., 2022). Researchers have been captivated in carrying out pre-clinical and clinical trials on new drug molecules to either interfere, inhibit, or alter the spliceosome itself or the splicing reactions to treat various spliceopathies (Rupaimoole and Slack, 2017; Bonnal et al., 2020; Desterro et al., 2020; Steensma et al., 2021; Childs-Disney et al., 2022; Murphy et al., 2022; Qin et al., 2022; Suresh et al., 2022; Zhu et al., 2022; Velema and Lu, 2023). It is worth noting that IR can be used as a diagnostic biomarker for the intronic expansion disorders in addition to its applications for therapeutic purposes (Sznajder et al., 2018). Yet, the field of IR is still emerging and there is more to explore (Vanichkina et al., 2018).
Mammalian systems exert diverse regulatory processes to control the fate of IR-containing mRNA transcripts (IR-mRNAs) (Figure 1), which are often subjected to nuclear retention accompanied with nuclear degradation via the exosomal degradation pathway (Gudipati et al., 2012). However, a novel class of introns termed Detained Introns (DIs) was discovered recently in which introns are retained within the nucleus and protected from degradation, yet exhibiting a slower splicing process than other introns within the same gene (Figure 1) (Boutz et al., 2015; Mauger et al., 2016; Naro et al., 2017). Interestingly, incomplete transcripts might be coupled with exporting proteins and translocated to the cytoplasm to form the Cytoplasmic Intron Retaining Transcripts (CIRTs) (Figure 1) (Yap et al., 2012; Buckley et al., 2014). CIRTs can be degraded in the cytoplasm via the mRNA surveillance pathways and considered to be important check points to remove mis-spliced mRNAs (Powers et al., 2020). Surveillance pathways include 1) the non-sense mediated decay (NMD) pathway, which is triggered by the presence of the premature termination codon (PTC) in CIRTs (Lejeune and Maquat, 2005; Jaillon et al., 2008; Wong et al., 2013; Rekosh and Hammarskjold, 2018), 2) the no-go decay pathway activated in the presence of stalled ribosomes (Passos et al., 2009), and 3) the non-stop decay pathway targeting the degradation of transcripts that lack PTC (Van Hoof et al., 2002; Vasudevan et al., 2002). Evidently, CIRTs can escape these mRNA surveillance pathways and proceed to produce novel protein isoforms (Gontijo et al., 2011; Yap et al., 2012; Nasif et al., 2018). Experimental validation showed that miR-128 has the ability to suppress the NMD factors (UPF1 and MLN51) leading to IR-mRNAs escape followed by the production of protein isoforms (Bruno et al., 2011). Strikingly, the ability of CIRTs to avoid the NMD pathway depends on the cellular micro-environmental conditions such as hypoxia, infection, and the lack of nutrients (Karam et al., 2013; Hug et al., 2015; Li et al., 2017; Nasif et al., 2018). Those conditions of cellular microenvironment are well-established hallmarks for a wide range of inflammatory-based diseases ranging from cancer to neuropathies, which emphasize the significance of IR in pathological states (Brady et al., 2017; Farina et al., 2020; Massonneau et al., 2020; Tan et al., 2020).
FIGURE 1. Different Fates of Pre-mRNA transcripts. A). Nuclear degradation B). Detained Introns (DIs) C). Cytoplasmic intron retaining transcripts (CIRTs).
The survival of IR-mRNAs from the cellular regulatory control becomes more apparent due to the advancement in IR-mRNA detection methods such as deep sequencing (Zheng et al., 2020). In a similar manner, the biological role of IR-mRNAs in both physiological and pathological states appears of high importance owing to the advancement of computational analyses (Jacob and Smith, 2017; Grabski et al., 2021). The power of computational technology and bioinformatics has been employed to develop programs capable to spot the intron retaining transcripts with high speed, accuracy, and sensitivity while building a huge IR library database that could be used as a reference for future IR studies as summarized in Table 1 (Bai et al., 2015; Middleton et al., 2017; Li et al., 2020). Apparently, current algorithms that detect AS in general might be tweaked to specifically detect IR (Zheng et al., 2020). Recent evolution of the deep tech and artificial intelligence have enormously improved the outcome for the precise and accurate detection of intronic sequences among transcripts to assist in the diagnosis of intronic abnormalities and aberrant splicing events (Zheng et al., 2020). For instance, Sun et al. (2023) employ the Intron Retention Index (IRI), an IRtools that provides IR analysis reads from RNA sequences collected from patients with systemic lupus erythematosus (SLE). The study reported dysregulation in IR as a hallmark of SLE disorder, which can be incorporate to enhance the accuracy of the IR detection. DeepRetention has the ability to predict the depth in intronic regions through pattern modelling, take the sequence depth into account as its main input to provide more detailed and accurate detection data like the intron length and the likelihood of retained introns (Wu et al., 2023).
In fact, the current algorithms lack the ability to annotate IR-containing mRNAs hindering the build up of a database parallel to that of intron-containing genomic DNAs, along with eliminating the differential expressions of IR-containing mRNAs. To overcome such limitations, advanced in vivo cell-imaging techniques have been successfully implemented to detect the presence and the expression levels of IR-containing mRNAs where non-invasive bioluminescence reporters are used to screen the IR splicing events while offering real time quantification (Shi et al., 2018; Zheng et al., 2019; Xie et al., 2020). Combining in silico computational methods with in vivo imaging techniques could provide accurate and reliable outcomes to ensure greater impacts in terms of detection and visualization. While detection techniques can bring the scientific community one step closer to rescuing patients from the deleterious effects of IR mis-spliced transcripts, they need to be coupled with therapeutic interventions to strengthen the battle against the intron-causing diseases. The field of manipulating and treating IR is still in its infancy since the number of related studies is limited, which warrants the need for extensive investigations.
The increased demands to treat spliceopathies have ignited the development of innovative therapeutic approaches such as spliceosome-mediated RNA trans-splicing (SMaRT) (Wally et al., 2012), splice switching oligonucleotides (SSO) (Havens and Hastings, 2016), CRISPR/Cas9 (Yuan et al., 2018) and nanomedicine (Garcia-Blanco, 2003; Havens et al., 2013; Suñé-Pou et al., 2017; Suñé-Pou et al., 2020). For decades, scientists have been in route to develop treatments for mis-spliced transcripts using different re-engineered genetic tools such as group I introns ribozymes. In vitro studies showed that these ribozymes can be re-engineered to employ the trans-splicing type of reaction in order to repair the mis-spliced transcripts and generate a functioning protein having high specificity and fidelity (Sullenger and Cech, 1994; Watanabe and Sullenger, 2000; Ryu et al., 2003; Song and Lee, 2006). Nonetheless, ribozymes can recognize one splicing site and replace the defective part at either 5′ or 3’ ends. Amini et al investigated the development of a ribozyme that resembles human spliceosomes in recognizing two splicing sites, excising introns, and joining the two flanking exons. This novel spliceozyme showed a significant removal of 100 nucleotides from the intron of interest followed by the production of a functioning protein with high accuracy (Amini et al., 2014; Amini and Müller, 2015), which is potentially feasible for a wide range of therapeutic applications.
Valadkhan et al. developed a small spliceozyme to perform splicing reactions by using the mammalian catalytic core of the spliceosomes, U2 and U6 snRNA, to efficiently catalyze an in vitro intron removal via resembling the first two trans-esterification splicing reactions in the nucleus. The results showed the successful removal of introns and subsequent ligation of exons from synthetic oligonucleotides constructs forming IR-free RNA products (Valadkhan and Manley, 2001; Valadkhan et al., 2009). This could unleash the potential of protein-free catalytic RNAs as artificial spliceozymes in hopes to expedite their translation into clinics via acquiring engineered delivery systems to enhance their efficacy in vivo. Many pitfalls and challenges will need to be overcome prior to the in vivo testing of spliceozymes including preserving the stability of the protein-free catalytic RNAs against the degradative enzymes present in serum, minimizing immunogenicity, overcoming biological membranes, and maximizing the efficacy of the splicing reaction upon introduction to the target cells carrying mis-spliced transcripts.
Synthetic biology has served the scientific community via enabling the construction of RNA riboswitches and aptamers to treat splicing mutations, regulate mammalian gene expression, or interfere with the splicing process as reported in the literature (An et al., 2006; Beilstein et al., 2015; Berens et al., 2015; Mathur et al., 2017; Vogel et al., 2018; Mol et al., 2019; Spöring et al., 2020). Alternatively, targeting the spliceosomes, their components, and/or their mechanisms of action can be a potential treatment approach (Eymin, 2020). Designing various types of synthetic RNA-based nanodevices along with their current progression and applications as post-transcriptional modulators were discussed in a recent review (Kawasaki et al., 2020). However, these studies collectively dealt with different types of splicing patterns while neglecting the significance and complexity of the IR defects.
RNA-based therapeutic platforms offer great potential in the treatment of various diseases including cancer (Lin et al., 2020). However, such platforms are still falling behind as clinical trials remain pending owing to the short life span of RNAs, their sensitivity to enzymatic degradation, and obstructed cellular internalization as a result of having a highly negatively charged backbone (Reischl and Zimmer, 2009). Scientists have extensively investigated possible routes to tackle those challenges and enhance the delivery of such therapies (Figure 2). Viral vectors are one of the potential carrier systems to deliver nucleic acid therapies providing both accuracy and protection against any enzymatic degradation of the loaded genetic materials. Despite their great properties, there are major drawbacks associated with the use of viral-based systems including their insufficiency in delivering the therapeutic agents to specific organs, which might provoke the immune system and possibly cause carcinogenesis among many other safety concerns (Lukashev and Zamyatnin, 2016; Zhou et al., 2020).
Alternatively, a more biologically feasible and safer route utilizing non-viral vectors has been used to overcome such drawbacks. Diverse types of non-viral vectors like lipid-based nanocarriers (Tagami et al., 2011; Xue et al., 2015), plasmid DNAs (Charoenphol and Bermudez, 2014; Hu et al., 2018), scaffolds (Chen et al., 2018; Kelly et al., 2019) and AuNPs (Guo et al., 2015) have been extensively studied to load and deliver RNA to the desired tissues. Lipid-based nanocarriers have numerous types but the most studied one is liposomes owing to their advantageous properties like biocompatibility, simple preparation, ease of surface modification to increase tissue targeting, and high RNA encapsulation efficiency when employing positively charged lipids. Inoh et al. (2011) successfully loaded siRNA into liposomes containing vectors and observed rapid and direct delivery to cytosol, minimal cytotoxicity, effective gene silencing, and less risk in triggering the immune response. Polymers have also attained a great attention as candidate carriers for RNA therapeutics due to their interesting properties in terms of biodegradation, cellular internalization, and the ability to control the release of loaded materials. Potential RNA polymeric carriers are countless and fully discussed in a recently published review (Ulkoski et al., 2019). Interestingly, DNA nanostructures such as DNA origami have emerged as a promising technology for delivering various bioactive molecules owing to their disintegrated internal aqueous nature allowing the delivery of different hydrophilic cargos like RNA molecules with reduced immune response and increased cellular accumulation. Lee et al. (2012) were able to construct DNA tetrahedra loaded with therapeutic siRNA allowing for ultimate and efficient therapeutic delivery. When incubated with human cervical cancer HeLa cells, gene silencing and tumor size reduction were both observed.
Following the pioneer studies conducted on DNA nanotechnology, scientists have been attracted to RNA nanotechnology via designing and building RNA nanostructures that can be applicable in the field of nanobiomedicine. RNA scaffolds emerge upon folding RNA strands into desired structures (Afonin et al., 2010; Afonin et al., 2012; Afonin et al., 2014; Sachdeva et al., 2014; Myhrvold and Silver, 2015; Bui et al., 2017; Ohno et al., 2019) as successfully did so Høiberg et al. (2019) with entrapping intrinsic siRNAs for efficient gene knockdown. Recently, metal-organic frameworks (MOFs) have been developed as nanoscale carrier systems for RNA therapies. The conducted study developed a unique UiO-NMOF exhibiting a characteristic surface morphology for co-delivering chemotherapeutic agent cisplatin and siRNA, the results shows a promising MDR gene silencing in ovarian cancer as well as the enhancement of cisplatin efficacy (He et al., 2014). Among all nanocarriers, AuNPs have been the stellar candidate for various biomedical and clinical applications including RNA therapeutics. They possess distinctive physiochemical, biological, and optical detection properties. In addition to their unique surface plasmon resonance, reduced cytotoxicity upon surface modifications with targeting ligands provides a selective and effective delivery system (Pissuwan et al., 2011; Conde et al., 2012; Guo et al., 2016; Graczyk et al., 2020). Intriguingly, developed a novel nanozyme system in which gold nanoparticles (AuNPs) were coupled with two different enzymes; natural (ligating) RNA ligase (RtcB) used to join exons after cleavage and synthetic (cleaving) DNAzyme to recognize the intron and initiate the splicing reaction. Results showed that the nanozyme selectively spliced 19 nucleotides out of RNA with 10% yield. Moreover, increased splicing reaction up to 66% was observed upon the addition of an excess amount of RNA ligase. However, the low RtcB copy number on individual AuNPs limits the splicing efficiency (Petree et al., 2018).
Nanotechnology can be a powerful weapon to secure healthy aging via repairing DNA and RNA damages. Life extension of patients suffering from muscular dystrophy (MD), Alzheimer disease (AD), Huntington disease (HD), or myelodysplastic syndromes (MDS) can be feasible through nanotechnology by which engineered nanorobots can perform cellular level surgeries such as splicing with high precision. To the best of our knowledge, no reports have shown artificial in vivo splicing in humans or even in animal models despite the advancement in performing in vivo RNA therapies in humans. Revolutionized gene editing tools like RNAi modalities (e.g., siRNA and miRNA) and CRISPR-Cas9 were encapsulated inside nanocarrier systems like lipid, organic, or inorganic NPs and have been used in humans to inhibit gene mutation and increase or correct gene expression (Hu et al., 2020).
Recently, United States Food and Drug Administration (USFDA) and European Commission (EC) have approved the first RNAi based therapy for clinical purposes called ONPATTRO (Patisiran), commercialized as a drug product to treat patients suffering from polyneuropathy, which is one of the symptoms associated with transthyretin amyloidosis (ATTR). A mutation in the gene coding for hereditary transthyretin (TTR), which is a protein synthesized mainly in the liver and responsible for carrying vitamin A and Thyroxine, causes protein misfolding and aggregation leading to the accumulation of formed amyloid at different locations and hence developing ATTR accompanied with several manifestations including polyneuropathy. ONPATTRO is produced using lipid NPs to encapsulate siRNA and enhance its delivery to the hepatocytes, thereby inhibiting the gene expression of both wild and mutant types (Huang, 2019). Similarly, Gillmore and colleagues investigated the effect of CRISPR-Cas9 as a potential therapeutic agent to knockdown TTR protein. Clinical and in-vivo results conducted on a small group of ATTR patients suffering from polyneuropathy showed a durable inhibition of TTR gene expression ranging from 80%–90% after 28 days of receiving a single dose (Gillmore et al., 2021). New advancement and alteration to CRISPER utilized novel base switchers known as base editing where Cas9 nickase is coupled to deaminase protein to allow single base conversions. Such advancements can potentially improve the use of gene editing technologies as treatment interventions for many alternative splicing defects. Chemello et al. (2021) reported combined two different strategies; namely, base and prime editing to developed gene editing tool to modify dystrophin gene where mutation in the splice donor leads to exon 51 deletion causing Duchenne muscular dystrophy (DMD). Their finding demonstrates a successful correction of the exon deletion of DMD gene tested on human cardiac iPSC models of DMD patients. Interestingly, have exploited a unique base editing approach to disrupt genes and minimize the unwanted double stranded breaks that Cas9 usually rely on to edit genes. They introduced their SpliceR tool to design base edited sgRNA to target splice site and achieved more reliable and efficient effect in primary human T cells (Kluesner et al., 2021). Similarly, investigators have employed CRISPR-cas9 base editing techniques targeting splice acceptor site to achieve a permanent exon skipping and improve the compatibility with adeno-associated viral packaging for in-vivo treatment (Winter et al., 2019).
Givosiran is another example of the developed RNAi based therapeutic agent loaded in lipid NPs to reduce the expression of delta aminolevulinic acid synthase 1 (ALAS1) gene and hence treating acute Intermittent porphyria (AIP). Overexpression of ALAS1 could lead to the deposition of neurotoxic heme compounds leading to painful neurovisceral attacks or causing chronic symptoms. Promising and effective reduction in the level of porphyria attacks was observed in clinical trials following the administration of Givosiran to AIP patients (Yasuda et al., 2014; Balwani et al., 2019; Sardh et al., 2019; Agarwal et al., 2020; Balwani et al., 2020; de Paula Brandão et al., 2020). Such findings emphasize the critical role of lipid NPs in accelerating the clinical use of RNA therapies. During the COVID-19 pandemic, the rapid response from Pfizer-BioNTech and Moderna by exploiting lipid NPs to encapsulate mRNA helped in developing the vaccine, which was granted the emergency authorization by the USFDA to combat against the emerging SARS-CoV-2 virus (Milane and Amiji, 2021).
Despite the great potency of using RNA therapies, a number of concerns need to be raised and tackled. For instance, RNAs degradation by endosomes and lysosomes must be avoided for the successful translocation to cytoplasm wherein selective targeting might occur. Wang et al. developed a novel endoplasmic reticulum membrane-modified hybrid nanoplexes (EhCv/siRNA NPs) encapsulating siRNA and protecting it from lysosomal degradation for efficient siRNA transportation to cytoplasm in order to improve siRNA silencing ability (Qiu et al., 2019). Nanocarriers of lipids and lipidoids require specific structural design criteria including selected phospholipids exhibiting two or more hydrophobic tails, tertiary amines, lipidoid O13 tail, and a pKa value
AA picked the topic and designed the outlines. BK and AA performed the literature search. BK, SA, and AA wrote the manuscript. SA and AA edited the manuscript. All authors contributed to the article and approved the submitted version.
The authors declare that the research was conducted in the absence of any commercial or financial relationships that could be construed as a potential conflict of interest.
All claims expressed in this article are solely those of the authors and do not necessarily represent those of their affiliated organizations, or those of the publisher, the editors and the reviewers. Any product that may be evaluated in this article, or claim that may be made by its manufacturer, is not guaranteed or endorsed by the publisher.
Afonin, K. A., Bindewald, E., Yaghoubian, A. J., Voss, N., Jacovetty, E., Shapiro, B. A., et al. (2010). In vitro assembly of cubic RNA-based scaffolds designed in silico. Nat. Nanotechnol. 5, 676–682. doi:10.1038/nnano.2010.160
Afonin, K. A., Kasprzak, W., Bindewald, E., Puppala, P. S., Diehl, A. R., Hall, K. T., et al. (2014). Computational and experimental characterization of RNA cubic nanoscaffolds. Methods 67, 256–265. doi:10.1016/j.ymeth.2013.10.013
Afonin, K. A., Kireeva, M., Grabow, W. W., Kashlev, M., Jaeger, L., and Shapiro, B. A. (2012). Co-transcriptional assembly of chemically modified RNA nanoparticles functionalized with siRNAs. Nano Lett. 12, 5192–5195. doi:10.1021/nl302302e
Agarwal, S., Simon, A. R., Goel, V., Habtemariam, B. A., Clausen, V. A., Kim, J. B., et al. (2020). Pharmacokinetics and pharmacodynamics of the small interfering ribonucleic acid, givosiran, in patients with acute hepatic porphyria. Clin. Pharmacol. Ther. 108, 63–72. doi:10.1002/cpt.1802
Alsudir, S. A., Almalik, A., and Alhasan, A. H. (2021). Catalogue of self-targeting nano-medical inventions to accelerate clinical trials. Biomaterials Sci. 9, 3898–3910. doi:10.1039/d1bm00235j
Amini, Z. N., and Müller, U. F. (2015). Increased efficiency of evolved group i intron spliceozymes by decreased side product formation. RNA 21, 1480–1489. doi:10.1261/rna.051888.115
Amini, Z. N., Olson, K. E., and Müller, U. F. (2014). Spliceozymes: ribozymes that remove introns from pre-mRNAs in trans. PLoS ONE 9, e101932. doi:10.1371/journal.pone.0101932
An, C. I., Trinh, V. B., and Yokobayashi, Y. (2006). Artificial control of gene expression in mammalian cells by modulating RNA interference through aptamer-small molecule interaction. RNA 12, 710–716. doi:10.1261/rna.2299306
Anna, A., and Monika, G. (2018). Splicing mutations in human genetic disorders: examples, detection, and confirmation. J. Appl. Genet. 59, 253–268. doi:10.1007/s13353-018-0444-7
Bai, Y., Ji, S., and Wang, Y. (2015). IRcall and IRclassifier: two methods for flexible detection of intron retention events from RNA-Seq data. BMC Genomics 16, S9. doi:10.1186/1471-2164-16-S2-S9
Balwani, M., Gouya, L., Rees, D., Stein, P., Stölzel, U., Aguilera, P., et al. (2019). GS-14-ENVISION, a phase 3 study to evaluate efficacy and safety of givosiran, an investigational RNAi therapeutic targeting aminolevulinic acid synthase 1, in acute hepatic porphyria patients. J. Hepatology 70, e81–e82. doi:10.1016/s0618-8278(19)30142-2
Balwani, M., Sardh, E., Ventura, P., Peiró, P. A., Rees, D. C., Stölzel, U., et al. (2020). Phase 3 trial of RNAi therapeutic givosiran for acute intermittent porphyria. N. Engl. J. Med. 382, 2289–2301. doi:10.1056/nejmoa1913147
Beilstein, K., Wittmann, A., Grez, M., and Suess, B. (2015). Conditional control of mammalian gene expression by tetracycline-dependent hammerhead ribozymes. ACS Synth. Biol. 4, 526–534. doi:10.1021/sb500270h
Berens, C., Groher, F., and Suess, B. (2015). RNA aptamers as genetic control devices: the potential of riboswitches as synthetic elements for regulating gene expression. Biotechnol. J. 10, 246–257. doi:10.1002/biot.201300498
Bonnal, S. C., López-Oreja, I., and Valcárcel, J. (2020). Roles and mechanisms of alternative splicing in cancer—implications for care. Nat. Rev. Clin. Oncol. 17 (8), 457–474. doi:10.1038/s41571-020-0350-x
Boutz, P. L., Bhutkar, A., and Sharp, P. A. (2015). Detained introns are a novel, widespread class of post-transcriptionally spliced introns. Genes. Dev. 29, 63–80. doi:10.1101/gad.247361.114
Brady, L. K., Wang, H., Radens, C. M., Bi, Y., Radovich, M., Maity, A., et al. (2017). Transcriptome analysis of hypoxic cancer cells uncovers intron retention in EIF2B5 as a mechanism to inhibit translation. PLoS Biol. 15, e2002623. doi:10.1371/journal.pbio.2002623
Braunschweig, U., Barbosa-Morais, N. L., Pan, Q., Nachman, E. N., Alipanahi, B., Gonatopoulos-Pournatzis, T., et al. (2014). Widespread intron retention in mammals functionally tunes transcriptomes. Genome Res. 24, 1774–1786. doi:10.1101/gr.177790.114
Bruno, I. G., Karam, R., Huang, L., Bhardwaj, A., Lou, C., Shum, E., et al. (2011). Identification of a MicroRNA that activates gene expression by repressing nonsense-mediated RNA decay. Mol. Cell. 42, 500–510. doi:10.1016/j.molcel.2011.04.018
Buckley, P. T., Khaladkar, M., Kim, J., and Eberwine, J. (2014). Cytoplasmic intron retention, function, splicing, and the sentinel RNA hypothesis. Wiley Interdiscip. Rev. RNA 5, 223–230. doi:10.1002/wrna.1203
Bui, M. N., Brittany Johnson, M., Viard, M., Satterwhite, E., Martins, A. N., Li, Z., et al. (2017). Versatile RNA tetra-U helix linking motif as a toolkit for nucleic acid nanotechnology. Nanomedicine Nanotechnol. Biol. Med. 13, 1137–1146. doi:10.1016/j.nano.2016.12.018
Charoenphol, P., and Bermudez, H. (2014). Design and application of multifunctional DNA nanocarriers for therapeutic delivery. Acta Biomater. 10, 1683–1691. doi:10.1016/j.actbio.2013.07.021
Chemello, F., Chai, A. C., Li, H., Rodriguez-Caycedo, C., Sanchez-Ortiz, E., Atmanli, A., et al. (2021). Precise correction of Duchenne muscular dystrophy exon deletion mutations by base and prime editing. Sci. Adv. 7 (18), eabg4910. doi:10.1126/sciadv.abg4910
Chen, R., Zhang, H., Yan, J., and Bryers, J. D. (2018). Scaffold-mediated delivery for non-viral mRNA vaccines. Gene Ther. 25, 556–567. doi:10.1038/s41434-018-0040-9
Childs-Disney, J. L., Yang, X., Gibaut, Q. M. R., Tong, Y., Batey, R. T., and Disney, M. D. (2022). Targeting RNA structures with small molecules. Nat. Rev. Drug Discov. 21 (10), 736–762. doi:10.1038/s41573-022-00521-4
Conde, J., Ambrosone, A., Sanz, V., Hernandez, Y., Marchesano, V., Tian, F., et al. (2012). Design of multifunctional gold nanoparticles for in vitro and in vivo gene silencing. ACS Nano 6, 8316–8324. doi:10.1021/nn3030223
de Paula Brandão, P. R., Titze-de-Almeida, S. S., and Titze-de-Almeida, R. (2020). Leading RNA interference therapeutics Part 2: silencing delta-aminolevulinic acid synthase 1, with a focus on givosiran. Mol. Diagnosis Ther. 24, 61–68. doi:10.1007/s40291-019-00438-6
Desterro, J., Bak-Gordon, P., and Carmo-Fonseca, M. (2020). Targeting mRNA processing as an anticancer strategy. Nat. Rev. Drug Discov. 19 (2), 112–129. doi:10.1038/s41573-019-0042-3
Dong, Y., Siegwart, D. J., and Anderson, D. G. (2019). Strategies, design, and chemistry in siRNA delivery systems. Adv. Drug Deliv. Rev. 144, 133–147. doi:10.1016/j.addr.2019.05.004
Dvinge, H., and Bradley, R. K. (2015). Widespread intron retention diversifies most cancer transcriptomes. Genome Med. 7, 45. doi:10.1186/s13073-015-0168-9
Edwards, C. R., Ritchie, W., Wong, J. J. L., Schmitz, U., Middleton, R., An, X., et al. (2016). A dynamic intron retention program in the mammalian megakaryocyte and erythrocyte lineages. Blood 127, e24–e34. doi:10.1182/blood-2016-01-692764
Eymin, B. (2020). Targeting the spliceosome machinery: a new therapeutic axis in cancer? Biochem. Pharmacol. 189, 114039. doi:10.1016/j.bcp.2020.114039
Farina, A. R., Cappabianca, L., Sebastiano, M., Zelli, V., Guadagni, S., and Mackay, A. R. (2020). Hypoxia-induced alternative splicing: the 11th hallmark of cancer. J. Exp. Clin. Cancer Res. 39, 110. doi:10.1186/s13046-020-01616-9
Galante, P. A. F., Sakabe, N. J., Kirschbaum-Slager, N., and De Souza, S. J. (2004). Detection and evaluation of intron retention events in the human transcriptome. RNA 10, 757–765. doi:10.1261/rna.5123504
Garcia-Blanco, M. A. (2003). Messenger RNA reprogramming by spliceosome-mediated RNA trans-splicing. J. Clin. Investigation 112, 474–480. doi:10.1172/JCI200319462
Gillmore, J. D., Gane, E., Taubel, J., Kao, J., Fontana, M., Maitland, M. L., et al. (2021). CRISPR-Cas9 in vivo gene editing for transthyretin amyloidosis. N. Engl. J. Med. 385, 493–502. doi:10.1056/nejmoa2107454
Gontijo, A. M., Miguela, V., Whiting, M. F., Woodruff, R., and Dominguez, M. (2011). Intron retention in the Drosophila melanogaster Rieske iron sulphur protein gene generated a new protein. Nat. Commun. 2, 323. doi:10.1038/ncomms1328
Grabski, D. F., Broseus, L., Kumari, B., Rekosh, D., Hammarskjold, M., and Ritchie, W. (2021). Intron retention and its impact on gene expression and protein diversity: a review and a practical guide. Wiley Interdiscip. Rev. RNA 12, e1631. doi:10.1002/wrna.1631
Graczyk, A., Pawlowska, R., Jedrzejczyk, D., and Chworos, A. (2020). Gold nanoparticles in conjunction with nucleic acids as a modern molecular system for cellular delivery. Molecules 25, 204. doi:10.3390/molecules25010204
Graveley, B. R. (2001). Alternative splicing: increasing diversity in the proteomic world. Trends Genet. 17, 100–107. doi:10.1016/S0168-9525(00)02176-4
Gudipati, R. K., Xu, Z., Lebreton, A., Séraphin, B., Steinmetz, L., Jacquier, A., et al. (2012). Extensive degradation of RNA precursors by the exosome in wild-type cells. Mol. Cell. 48, 409–421. doi:10.1016/j.molcel.2012.08.018
Guo, J., O’Driscoll, C. M., Holmes, J. D., and Rahme, K. (2016). Bioconjugated gold nanoparticles enhance cellular uptake: a proof of concept study for siRNA delivery in prostate cancer cells. Int. J. Pharm. 509, 16–27. doi:10.1016/j.ijpharm.2016.05.027
Guo, J., Rahme, K., Fitzgerald, K. A., Holmes, J. D., and O’Driscoll, C. M. (2015). Biomimetic gold nanocomplexes for gene knockdown: will gold deliver dividends for small interfering RNA nanomedicines? Nano Res. 8, 3111–3140. doi:10.1007/s12274-015-0829-4
Havens, M. A., Duelli, D. M., and Hastings, M. L. (2013). Targeting RNA splicing for disease therapy. Wiley Interdiscip. Rev. RNA 4, 247–266. doi:10.1002/wrna.1158
Havens, M. A., and Hastings, M. L. (2016). Splice-switching antisense oligonucleotides as therapeutic drugs. Nucleic Acids Res. 44, 6549–6563. doi:10.1093/nar/gkw533
He, C., Lu, K., Liu, D., and Lin, W. (2014). Nanoscale metal-organic frameworks for the co-delivery of cisplatin and pooled siRNAs to enhance therapeutic efficacy in drug-resistant ovarian cancer cells. J. Am. Chem. Soc. 136, 5181–5184. doi:10.1021/ja4098862
Høiberg, H. C., Sparvath, S. M., Andersen, V. L., Kjems, J., and Andersen, E. S. (2019). An RNA origami octahedron with intrinsic siRNAs for potent gene knockdown. Biotechnol. J. 14, e1700634. doi:10.1002/biot.201700634
Horowitz, D. S., and Abelson, J. (1993). Stages in the second reaction of pre-mRNA splicing: the final step is ATP independent. Genes. and Dev. 7 (2), 320–329. doi:10.1101/gad.7.2.320
Hu, B., Zhong, L., Weng, Y., Peng, L., Huang, Y., Zhao, Y., et al. (2020). Therapeutic siRNA: state of the art. Signal Transduct. Target. Ther. 5, 101. doi:10.1038/s41392-020-0207-x
Hu, Q., Wang, S., Wang, L., Gu, H., and Fan, C. (2018). DNA nanostructure-based systems for intelligent delivery of therapeutic oligonucleotides. Adv. Healthc. Mater. 7, e1701153. doi:10.1002/adhm.201701153
Huang, Y. Y., Zhao, L., and Ding, Y. Q. (2019). Progress in the research of LASP-1: progress in the research of LASP-1. Prog. Biochem. Biophysics 37, 1182–1187. doi:10.3724/sp.j.1206.2010.00061
Hug, N., Longman, D., and Cáceres, J. F. (2015). Mechanism and regulation of the nonsense-mediated decay pathway. Nucleic Acids Res. 44, 1483–1495. doi:10.1093/nar/gkw010
Inoh, Y., Furuno, T., Hirashima, N., Kitamoto, D., and Nakanishi, M. (2011). Rapid delivery of small interfering RNA by biosurfactant MEL-A-containing liposomes. Biochem. Biophysical Res. Commun. 414, 635–640. doi:10.1016/j.bbrc.2011.09.147
Jacob, A. G., and Smith, C. W. J. (2017). Intron retention as a component of regulated gene expression programs. Hum. Genet. 136, 1043–1057. doi:10.1007/s00439-017-1791-x
Jaillon, O., Bouhouche, K., Gout, J. F., Aury, J. M., Noel, B., Saudemont, B., et al. (2008). Translational control of intron splicing in eukaryotes. Nature 451, 359–362. doi:10.1038/nature06495
Karam, R., Wengrod, J., Gardner, L. B., and Wilkinson, M. F. (2013). Regulation of nonsense-mediated mRNA decay: implications for physiology and disease. Biochimica Biophysica Acta - Gene Regul. Mech. 1829, 624–633. doi:10.1016/j.bbagrm.2013.03.002
Kawasaki, S., Ono, H., Hirosawa, M., and Saito, H. (2020). RNA and protein-based nanodevices for mammalian post-transcriptional circuits. Curr. Opin. Biotechnol. 63, 99–110. doi:10.1016/j.copbio.2019.11.019
Kelly, D. C., Raftery, R. M., Curtin, C. M., O’Driscoll, C. M., and O’Brien, F. J. (2019). Scaffold-based delivery of nucleic acid therapeutics for enhanced bone and cartilage repair. J. Orthop. Res. 37, 1671–1680. doi:10.1002/jor.24321
Kluesner, M. G., Lahr, W. S., Lonetree, C. l., Smeester, B. A., Qiu, X., Slipek, N. J., et al. (2021). CRISPR-Cas9 cytidine and adenosine base editing of splice-sites mediates highly-efficient disruption of proteins in primary and immortalized cells. Nat. Commun. 12 (1), 2437. doi:10.1038/s41467-021-22009-2
Kumari, A., Sedehizadeh, S., Brook, J. D., Kozlowski, P., and Wojciechowska, M. (2022). Differential fates of introns in gene expression due to global alternative splicing. Hum. Genet. 141, 31–47. doi:10.1007/s00439-021-02409-6
Lee, H., Lytton-Jean, A. K. R., Chen, Y., Love, K. T., Park, A. I., Karagiannis, E. D., et al. (2012). Molecularly self-assembled nucleic acid nanoparticles for targeted in vivo siRNA delivery. Nat. Nanotechnol. 7, 389–393. doi:10.1038/nnano.2012.73
Lejeune, F., and Maquat, L. E. (2005). Mechanistic links between nonsense-mediated mRNA decay and pre-mRNA splicing in mammalian cells. Curr. Opin. Cell. Biol. 17, 309–315. doi:10.1016/j.ceb.2005.03.002
Li, H. D., Funk, C. C., and Price, N. D. (2020). IREAD: a tool for intron retention detection from RNA-seq data. BMC Genomics 21, 128. doi:10.1186/s12864-020-6541-0
Li, Z., Vuong, J. K., Zhang, M., Stork, C., and Zheng, S. (2017). Inhibition of nonsense-mediated RNA decay by ER stress. RNA 23, 378–394. doi:10.1261/rna.058040.116
Lin, Y. X., Wang, Y., Blake, S., Yu, M., Mei, L., Wang, H., et al. (2020). RNA nanotechnology-mediated cancer immunotherapy. Theranostics 10, 281–299. doi:10.7150/thno.35568
Llorian, M., Gooding, C., Bellora, N., Hallegger, M., Buckroyd, A., Wang, X., et al. (2016). The alternative splicing program of differentiated smooth muscle cells involves concerted non-productive splicing of post-transcriptional regulators. Nucleic Acids Res. 44, 8933–8950. doi:10.1093/nar/gkw560
Lukashev, A. N., and Zamyatnin, A. A. (2016). Viral vectors for gene therapy: current state and clinical perspectives. Biochem. Mosc. 81, 700–708. doi:10.1134/S0006297916070063
Massonneau, J., Lacombe-Burgoyne, C., and Boissonneault, G. (2020). pH-induced variations in the TK1 gene model. Mutat. Res. - Genet. Toxicol. Environ. Mutagen. 849, 503128. doi:10.1016/j.mrgentox.2019.503128
Mathur, M., Xiang, J. S., and Smolke, C. D. (2017). Mammalian synthetic biology for studying the cell. J. Cell. Biol. 216, 73–82. doi:10.1083/jcb.201611002
Mauger, O., Lemoine, F., and Scheiffele, P. (2016). Targeted intron retention and excision for rapid gene regulation in response to neuronal activity. Neuron 92, 1266–1278. doi:10.1016/j.neuron.2016.11.032
Middleton, R., Gao, D., Thomas, A., Singh, B., Au, A., Wong, J. J. L., et al. (2017). IRFinder: assessing the impact of intron retention on mammalian gene expression. Genome Biol. 18, 51. doi:10.1186/s13059-017-1184-4
Milane, L., and Amiji, M. (2021). Clinical approval of nanotechnology-based SARS-CoV-2 mRNA vaccines: impact on translational nanomedicine. Drug Deliv. Transl. Res. 11, 1309–1315. doi:10.1007/s13346-021-00911-y
Modrek, B., and Lee, C. (2002). A genomic view of alternative splicing. Nat. Genet. 30, 13–19. doi:10.1038/ng0102-13
Mohammadinejad, R., Dehshahri, A., Sagar Madamsetty, V., Zahmatkeshan, M., Tavakol, S., Makvandi, P., et al. (2020). In vivo gene delivery mediated by non-viral vectors for cancer therapy. J. Control. Release 325, 249–275. doi:10.1016/j.jconrel.2020.06.038
Mol, A. A., Groher, F., Schreiber, B., Rühmkorff, C., and Suess, B. (2019). Robust gene expression control in human cells with a novel universal TetR aptamer splicing module. Nucleic acids Res. 47, e132. doi:10.1093/nar/gkz753
Monteuuis, G., Wong, J. J. L., Bailey, C. G., Schmitz, U., and Rasko, J. E. J. (2019). The changing paradigm of intron retention: regulation, ramifications and recipes. Nucleic Acids Res. 47, 11497–11513. doi:10.1093/nar/gkz1068
Murphy, A. J., Li, A. H., and Sun, H. (2022). Therapeutic targeting of alternative splicing: a new frontier in cancer treatment. Front. Oncol. 12, 868664. doi:10.3389/fonc.2022.868664
Myhrvold, C., and Silver, P. A. (2015). Using synthetic RNAs as scaffolds and regulators. Nat. Struct. Mol. Biol. 22, 8–10. doi:10.1038/nsmb.2944
Naro, C., Jolly, A., Di Persio, S., Bielli, P., Setterblad, N., Alberdi, A. J., et al. (2017). An orchestrated intron retention program in meiosis controls timely usage of transcripts during germ cell differentiation. Dev. Cell. 41, 82–93.e4. doi:10.1016/j.devcel.2017.03.003
Nasif, S., Contu, L., and Mühlemann, O. (2018). Beyond quality control: the role of nonsense-mediated mRNA decay (NMD) in regulating gene expression. Seminars Cell. Dev. Biol. 75, 78–87. doi:10.1016/j.semcdb.2017.08.053
Ohno, H., Akamine, S., and Saito, H. (2019). RNA nanostructures and scaffolds for biotechnology applications. Curr. Opin. Biotechnol. 58, 53–61. doi:10.1016/j.copbio.2018.11.006
Parmar, R. G., Brown, C. R., Matsuda, S., Willoughby, J. L. S., Theile, C. S., Charissé, K., et al. (2018). Facile synthesis, geometry, and 2′-substituent-dependent in vivo activity of 5′-(E)- and 5′-(Z)-Vinylphosphonate-Modified siRNA conjugates. J. Med. Chem. 61, 734–744. doi:10.1021/acs.jmedchem.7b01147
Passos, D. O., Doma, M. K., Shoemaker, C. J., Muhlrad, D., Green, R., Weissman, J., et al. (2009). Analysis of Dom34 and its function in No-Go decay. Mol. Biol. Cell. 20, 3025–3032. doi:10.1091/mbc.E09-01-0028
Petree, J. R., Yehl, K., Galior, K., Glazier, R., Deal, B., and Salaita, K. (2018). Site-selective RNA splicing nanozyme: DNAzyme and RtcB conjugates on a gold nanoparticle. ACS Chem. Biol. 13, 215–224. doi:10.1021/acschembio.7b00437
Pimentel, H., Conboy, J. G., and Pachter, L. (2015). Keep me around: intron retention detection and analysis. arXiv preprint arXiv:1510.00696.
Pissuwan, D., Niidome, T., and Cortie, M. B. (2011). The forthcoming applications of gold nanoparticles in drug and gene delivery systems. J. Control. Release 149, 65–71. doi:10.1016/j.jconrel.2009.12.006
Powers, K. T., Szeto, J. Y. A., and Schaffitzel, C. (2020). New insights into no-go, non-stop and nonsense-mediated mRNA decay complexes. Curr. Opin. Struct. Biol. 65, 110–118. doi:10.1016/j.sbi.2020.06.011
Qin, S., Tang, X., Chen, Y., Chen, K., Fan, N., Xiao, W., et al. (2022). mRNA-based therapeutics: powerful and versatile tools to combat diseases. Signal Transduct. Target. Ther. 7 (1), 166. doi:10.1038/s41392-022-01007-w
Qiu, C., Han, H. H., Sun, J., Zhang, H. T., Wei, W., Cui, S. H., et al. (2019). Regulating intracellular fate of siRNA by endoplasmic reticulum membrane-decorated hybrid nanoplexes. Nat. Commun. 10, 2702. doi:10.1038/s41467-019-10562-w
Rappsilber, J., Ryder, U., Lamond, A. I., and Mann, M. (2002). Large-scale proteomic analysis of the human spliceosome. Genome Res. 12, 1231–1245. doi:10.1101/gr.473902
Reischl, D., and Zimmer, A. (2009). Drug delivery of siRNA therapeutics: potentials and limits of nanosystems. Nanomedicine Nanotechnol. Biol. Med. 5, 8–20. doi:10.1016/j.nano.2008.06.001
Rekosh, D., and Hammarskjold, M. L. (2018). Intron retention in viruses and cellular genes: detention, border controls and passports. Wiley Interdiscip. Rev. RNA 9, e1470. doi:10.1002/wrna.1470
Rupaimoole, R., and Slack, F. J. (2017). MicroRNA therapeutics: towards a new era for the management of cancer and other diseases. Nat. Rev. Drug Discov. 16 (3), 203–222. doi:10.1038/nrd.2016.246
Ryu, K. J., Kim, J. H., and Lee, S. W. (2003). Ribozyme-mediated selective induction of new gene activity in hepatitis C virus internal ribosome entry site-expressing cells by targeted trans-splicing. Mol. Ther. 7, 386–395. doi:10.1016/S1525-0016(02)00063-1
Sachdeva, G., Garg, A., Godding, D., Way, J. C., and Silver, P. A. (2014). In vivo co-localization of enzymes on RNA scaffolds increases metabolic production in a geometrically dependent manner. Nucleic Acids Res. 42, 9493–9503. doi:10.1093/nar/gku617
Sardh, E., Harper, P., Balwani, M., Stein, P., Rees, D., Bissell, D. M., et al. (2019). Phase 1 trial of an RNA interference therapy for acute intermittent porphyria. N. Engl. J. Med. 380, 549–558. doi:10.1056/nejmoa1807838
Scotti, M. M., and Swanson, M. S. (2016). RNA mis-splicing in disease. Nat. Rev. Genet. 17 (1), 19–32. doi:10.1038/nrg.2015.3
Shi, Y., Liu, W., Zheng, H., Li, Z., Shi, X., Cai, S., et al. (2018). Imaging of pre-mRNA splicing in living subjects using a genetically encoded luciferase reporter. Biomed. Opt. Express 9, 518. doi:10.1364/boe.9.000518
Song, M. S., and Lee, S. W. (2006). Cancer-selective induction of cytotoxicity by tissue-specific expression of targeted trans-splicing ribozyme. FEBS Lett. 580, 5033–5043. doi:10.1016/j.febslet.2006.08.021
Spöring, M., Finke, M., and Hartig, J. S. (2020). Aptamers in RNA-based switches of gene expression. Curr. Opin. Biotechnol. 63, 34–40. doi:10.1016/j.copbio.2019.11.008
Steensma, D. P., Wermke, M., Klimek, V. M., Greenberg, P. L., Font, P., Komrokji, R. S., et al. (2021). Phase I first-in-human dose escalation study of the oral SF3B1 modulator H3B-8800 in myeloid neoplasms. Leukemia 35 (12), 3542–3550. doi:10.1038/s41375-021-01328-9
Sugnet, C. W., Kent, W. J., Ares, M., and Haussler, D. (2003). Transcriptome and genome conservation of alternative splicing events in humans and mice. Pac Symp. Biocomput 2003, 66–77. doi:10.1142/9789812704856_0007
Sullenger, B. A., and Cech, T. R. (1994). Ribozyme-mediated repair of defective mRNA by targeted trans-splicing. Nature 371, 619–622. doi:10.1038/371619a0
Sun, X., Liu, Z., Li, Z., Zeng, Z., Peng, W., Zhu, J., et al. (2023). Abnormalities in intron retention characterize patients with systemic lupus erythematosus. Sci. Rep. 13 (1), 5141. doi:10.1038/s41598-023-31890-4
Suñé-Pou, M., Limeres, M. J., Moreno-Castro, C., Hernández-Munain, C., Suñé-Negre, J. M., Cuestas, M. L., et al. (2020). Innovative therapeutic and delivery approaches using nanotechnology to correct splicing defects underlying disease. Front. Genet. 11, 731. doi:10.3389/fgene.2020.00731
Suñé-Pou, M., Prieto-Sánchez, S., Boyero-Corral, S., Moreno-Castro, C., El Yousfi, Y., Suñé-Negre, J., et al. (2017). Targeting splicing in the treatment of human disease. Genes. 8, 87. doi:10.3390/genes8030087
Supek, F., Miñana, B., Valcárcel, J., Gabaldón, T., and Lehner, B. (2014). Synonymous mutations frequently act as driver mutations in human cancers. Cell. 156, 1324–1335. doi:10.1016/j.cell.2014.01.051
Suresh, B. M., Akahori, Y., Taghavi, A., Crynen, G., Gibaut, Q. M. R., Li, Y., et al. (2022). Low-molecular weight small molecules can potently bind RNA and affect oncogenic pathways in cells. J. Am. Chem. Soc. 144 (45), 20815–20824. doi:10.1021/jacs.2c08770
Sznajder, Ł. J., Thomas, J. D., Carrell, E. M., Reid, T., McFarland, K. N., Cleary, J. D., et al. (2018). Intron retention induced by microsatellite expansions as a disease biomarker. Proc. Natl. Acad. Sci. U. S. A. 115, 4234–4239. doi:10.1073/pnas.1716617115
Tagami, T., Uehara, Y., Moriyoshi, N., Ishida, T., and Kiwada, H. (2011). Anti-PEG IgM production by siRNA encapsulated in a PEGylated lipid nanocarrier is dependent on the sequence of the siRNA. J. Control. Release 151, 149–154. doi:10.1016/j.jconrel.2010.12.013
Tan, D. J., Mitra, M., Chiu, A. M., and Coller, H. A. (2020). Intron retention is a robust marker of intertumoral heterogeneity in pancreatic ductal adenocarcinoma. npj Genomic Med. 5, 55. doi:10.1038/s41525-020-00159-4
Tanackovic, G., Ransijn, A., Thibault, P., Abou Elela, S., Klinck, R., Berson, E. L., et al. (2011). PRPF mutations are associated with generalized defects in spliceosome formation and pre-mRNA splicing in patients with retinitis pigmentosa. Hum. Mol. Genet. 20 (11), 2116–2130. doi:10.1093/hmg/ddr094
Tsuiji, H., Iguchi, Y., Furuya, A., Kataoka, A., Hatsuta, H., Atsuta, N., et al. (2013). Spliceosome integrity is defective in the motor neuron diseases ALS and SMA. EMBO Mol. Med. 5 (2), 221–234. doi:10.1002/emmm.201202303
Ulkoski, D., Bak, A., Wilson, J. T., and Krishnamurthy, V. R. (2019). Recent advances in polymeric materials for the delivery of RNA therapeutics. Expert Opin. Drug Deliv. 16, 1149–1167. doi:10.1080/17425247.2019.1663822
Valadkhan, S., and Manley, J. L. (2001). Splicing-related catalysis by protein-free snRNAS. Nature 413, 701–707. doi:10.1038/35099500
Valadkhan, S., Mohammadi, A., Jaladat, Y., and Geisler, S. (2009). Protein-free small nuclear RNAs catalyze a two-step splicing reaction. Proc. Natl. Acad. Sci. U. S. A. 106, 11901–11906. doi:10.1073/pnas.0902020106
Van Hoof, A., Frischmeyer, P. A., Dietz, H. C., and Parker, R. (2002). Exosome-mediated recognition and degradation of mRNAs lacking a termination codon. Science 295, 2262–2264. doi:10.1126/science.1067272
Vanichkina, D. P., Schmitz, U., Wong, J. J. L., and Rasko, J. E. (2018). Challenges in defining the role of intron retention in normal biology and disease. Seminars Cell. Dev. Biol. 75, 40–49. doi:10.1016/j.semcdb.2017.07.030
Vasudevan, S., Peltz, S. W., and Wilusz, C. J. (2002). Non-stop decay - a new mRNA surveillance pathway. BioEssays 24, 785–788. doi:10.1002/bies.10153
Velema, W. A., and Lu, Z. (2023). Chemical RNA cross-linking: mechanisms, computational analysis, and biological applications. JACS Au 3 (2), 316–332. doi:10.1021/jacsau.2c00625
Vogel, M., Weigand, J. E., Kluge, B., Grez, M., and Suess, B. (2018). A small, portable RNA device for the control of exon skipping in mammalian cells. Nucleic acids Res. 46, e48. doi:10.1093/nar/gky062
Wally, V., Murauer, E. M., and Bauer, J. W. (2012). Spliceosome-mediated trans-splicing: the therapeutic cut and paste. J. Investigative Dermatology 132, 1959–1966. doi:10.1038/jid.2012.101
Watanabe, T., and Sullenger, B. A. (2000). Induction of wild-type p53 activity in human cancer cells by ribozymes that repair mutant p53 transcripts. Proc. Natl. Acad. Sci. U. S. A. 97, 8490–8494. doi:10.1073/pnas.150104097
Whitehead, K. A., Dorkin, J. R., Vegas, A. J., Chang, P. H., Veiseh, O., Matthews, J., et al. (2014). Degradable lipid nanoparticles with predictable in vivo siRNA delivery activity. Nat. Commun. 5, 4277. doi:10.1038/ncomms5277
Winter, J., Luu, A., Gapinske, M., Manandhar, S., Shirguppe, S., Woods, W. S., et al. (2019). Targeted exon skipping with AAV-mediated split adenine base editors. Cell. Discov. 5 (1), 41. doi:10.1038/s41421-019-0109-7
Wong, J. J. L., Ritchie, W., Ebner, O., Selbach, M., and Huang, Y. (2013). Orchestrated intron retention regulates normal granulocyte differentiation. Cell. 154, 583–595. doi:10.1016/j.cell.2013.06.052
Wu, Z., Zheng, J., Liu, J., Lin, C., and Li, H. D. (2023). DeepRetention: a deep learning approach for intron retention detection. Big Data Min. Anal. 6 (2), 115–126. doi:10.26599/bdma.2022.9020023
Xie, J., Zheng, H., Chen, S., Shi, X., Mao, W., and Wang, F. (2020). Rational design of an activatable reporter for quantitative imaging of RNA aberrant splicing in vivo. Mol. Ther. - Methods Clin. Dev. 17, 904–911. doi:10.1016/j.omtm.2020.04.007
Xue, H., Guo, P., Wen, W. C., and Wong, H. (2015). Lipid-based nanocarriers for RNA delivery. Curr. Pharm. Des. 21, 3140–3147. doi:10.2174/1381612821666150531164540
Yap, K., Lim, Z. Q., Khandelia, P., Friedman, B., and Makeyev, E. V. (2012). Coordinated regulation of neuronal mRNA steady-state levels through developmentally controlled intron retention. Genes. Dev. 26, 1209–1223. doi:10.1101/gad.188037.112
Yasuda, M., Gan, L., Chen, B., Kadirvel, S., Yu, C., Phillips, J. D., et al. (2014). RNAi-mediated silencing of hepatic Alas1 effectively prevents and treats the induced acute attacks in acute intermittent porphyria mice. Proc. Natl. Acad. Sci. U. S. A. 111, 7777–7782. doi:10.1073/pnas.1406228111
Yeo, G. W., Van Nostrand, E., Holste, D., Poggio, T., and Burge, C. B. (2005). Identification and analysis of alternative splicing events conserved in human and mouse. Proc. Natl. Acad. Sci. U. S. A. 102, 2850–2855. doi:10.1073/pnas.0409742102
Yoshida, K., Sanada, M., Shiraishi, Y., Nowak, D., Nagata, Y., Yamamoto, R., et al. (2011). Frequent pathway mutations of splicing machinery in myelodysplasia. Nature 478 (7367), 458–469. doi:10.1182/blood.v118.21.458.458
Yuan, J., Ma, Y., Huang, T., Chen, Y., Peng, Y., Li, B., et al. (2018). Genetic modulation of RNA splicing with a CRISPR-guided cytidine deaminase. Mol. Cell. 72, 380–394.e7. doi:10.1016/j.molcel.2018.09.002
Zheng, H., Chen, S., Wang, X., Xie, J., Tian, J., and Wang, F. (2019). Intron retained bioluminescence reporter for real-time imaging of pre-mRNA splicing in living subjects. Anal. Chem. 91, 12392–12398. doi:10.1021/acs.analchem.9b02935
Zheng, J. T., Lin, C. X., Fang, Z. Y., and Li, H. D. (2020). Intron retention as a mode for RNA-seq data analysis. Front. Genet. 11, 586. doi:10.3389/fgene.2020.00586
Zhou, J., Shao, Z., Liu, J., Duan, Q., Wang, X., Li, J., et al. (2020). From endocytosis to nonendocytosis: the emerging era of gene delivery. ACS Appl. Bio Mater. 3, 2686–2701. doi:10.1021/acsabm.9b01131
Keywords: intron retention, synthetic biology, spliceosome, spliceopathies, catalytic RNAs
Citation: Khalifah BA, Alghamdi SA and Alhasan AH (2023) Unleashing the potential of catalytic RNAs to combat mis-spliced transcripts. Front. Bioeng. Biotechnol. 11:1244377. doi: 10.3389/fbioe.2023.1244377
Received: 22 June 2023; Accepted: 23 October 2023;
Published: 16 November 2023.
Edited by:
Nikolay Shirokikh, Australian National University, AustraliaReviewed by:
Gazi Sakir Hossain, National University of Singapore, SingaporeCopyright © 2023 Khalifah, Alghamdi and Alhasan. This is an open-access article distributed under the terms of the Creative Commons Attribution License (CC BY). The use, distribution or reproduction in other forums is permitted, provided the original author(s) and the copyright owner(s) are credited and that the original publication in this journal is cited, in accordance with accepted academic practice. No use, distribution or reproduction is permitted which does not comply with these terms.
*Correspondence: Ali H. Alhasan, YWFsaGFzYW5Aa2Fjc3QuZWR1LnNh; Shareefa A. Alghamdi, c2FhYWxnaGFtZGkxQGthdS5lZHUuc2E=
Disclaimer: All claims expressed in this article are solely those of the authors and do not necessarily represent those of their affiliated organizations, or those of the publisher, the editors and the reviewers. Any product that may be evaluated in this article or claim that may be made by its manufacturer is not guaranteed or endorsed by the publisher.
Research integrity at Frontiers
Learn more about the work of our research integrity team to safeguard the quality of each article we publish.