- Department of Biomedical Engineering, Shantou University, Shantou, China
Addressing critical bone defects necessitates innovative solutions beyond traditional methods, which are constrained by issues such as immune rejection and donor scarcity. Smart polymeric biomaterials that respond to external stimuli have emerged as a promising alternative, fostering endogenous bone regeneration. Light-responsive polymers, employed in 3D-printed scaffolds and photothermal therapies, enhance antibacterial efficiency and bone repair. Thermo-responsive biomaterials show promise in controlled bioactive agent release, stimulating osteocyte differentiation and bone regeneration. Further, the integration of conductive elements into polymers improves electrical signal transmission, influencing cellular behavior positively. Innovations include advanced 3D-printed poly (l-lactic acid) scaffolds, polyurethane foam scaffolds promoting cell differentiation, and responsive polymeric biomaterials for osteogenic and antibacterial drug delivery. Other developments focus on enzyme-responsive and redox-responsive polymers, which offer potential for bone regeneration and combat infection. Biomaterials responsive to mechanical, magnetic, and acoustic stimuli also show potential in bone regeneration, including mechanically-responsive polymers, magnetic-responsive biomaterials with superparamagnetic iron oxide nanoparticles, and acoustic-responsive biomaterials. In conclusion, smart biopolymers are reshaping scaffold design and bone regeneration strategies. However, understanding their advantages and limitations is vital, indicating the need for continued exploratory research.
1 Introduction
Human bone structure, vital for mobility, structural support, and organ protection, has remarkable self-healing abilities (Stevens, 2008). However, critical-sized bone defects (CSD), resulted from tumor, trauma, infection, or other severe bone damages, is challenging due to problems such as immune rejection and donor shortage (Amini et al., 2012; Agarwal and García, 2015). An ideal bone scaffold for CSD should mimic the natural bone composition of collagen, hydroxyapatite, and cells. Additionally, the scaffold should eventually degrade, after serving its function (Battafarano et al., 2021). For this reason, polymeric materials have been extensively studied for bone repair, offering innovative properties for bone implant optimization, bone tissue engineering and therapeutic agent delivery (Laurencin and Khan, 2012; Ogueri et al., 2019; Filippi et al., 2020; Khan et al., 2022a). In principle, the material should emulate the properties of the surrounding tissue, be it rigidity for bone or pliability for softer tissues. Such properties are also influenced by the cellular requirements for porosity (Kohane and Langer, 2008).
Biomaterial factors such as biocompatibility, mechanical properties, and surface properties affect cell attachment, osteointegration, and osteogenesis (Ogle, 2015). Regeneration efficacy can be enhanced by delivering bioactive agents that regulate bone metabolic signaling pathways and new bone formation (Wei et al., 2022). Bone Tissue Engineering (BTE), a multidisciplinary field with decades of accumulated data, holds promise for addressing bone defects. BTE employs cells, growth factors, dynamic stresses, and biomaterials to fabricate bespoke bioactive scaffolds - including metals, ceramics, or polymers - to enhance bone repair (Wang et al., 2021).
Smart polymeric biomaterials are instrumental in controlled drug delivery systems, detecting stimuli and releasing bioactive agents accordingly (Wei et al., 2017; Montoya et al., 2021). Their function relies on stimuli-sensitive moieties that, when exposed to stimuli, undergo changes triggering drug release (Bustamante-Torres et al., 2021; Zhang et al., 2021). Stimuli-responsive biopolymers have recently gained attention as valuable graft materials. External physical triggers or certain pathological microenvironments can alter these materials’ configuration, influencing cell destiny and bolstering bone tissue therapy and regeneration (Sobol et al., 2011; Lavanya et al., 2020; Cerqueni et al., 2021; Sivakumar et al., 2022; Heng et al., 2023). This mini-review will delve into the major types of smart polymers used in bone regeneration, outlining their functions, advantages, and limitations.
2 Light responsive polymeric biomaterials for bone regeneration
Significant research underscores the utility of light-responsive polymers in precision drug delivery, boasting excellent control over spatial and temporal parameters and intensity, relevant in various medical conditions (Municoy et al., 2020; Pokharel and Park, 2022). In bone regeneration research, such materials can function as multifunctional scaffolds supporting bone repair or as drug delivery systems targeting antibacterial and osteogenic needs (Tomatsu et al., 2011).
One study reported a 3D-printed scaffold made of shape-memory polyurethane (SMPU) and magnesium (Mg) for bone repair. The implanted scaffold can form a tight contact within bone structure by changing its shape between original conformation and compressed conformation, which was controlled by near-infrared (NIR) irradiation-induced photothermal effects. The scaffold demonstrated significant osteo-promotive functions with in vitro and in vivo studies as shown in Figure 1A (Zhang et al., 2022). Photothermal therapy can also be used directly to bone defects, because heat at around 40°C–43°C can enhance proliferation and osteoblastic differentiation of mesenchymal stem cells (MSCs) (Liao et al., 2021). Tong et al. designed a biodegradable bone implant with black phosphorous (BP) nanosheets incorporated in poly (lactic-co-glycolic acid) (PLGA). After exposed to low intensity and periodic NIR, the implant significantly enhanced expressions of heat shock proteins, and increased osteogenesis in both cell and animal models (Tong et al., 2019). Furthermore, Zeng et al. developed a novel polydopamine-IR820-daptomycin coating for titanium bone implant. Under NIR irradiation, the composite had anti-bacterial efficiency. Additionally, the coating changed surface properties of implants, resulting in better contact with bones. The coating also significantly increased proliferation and osteogenic differentiation of bone marrow stem cells (Zeng et al., 2020).
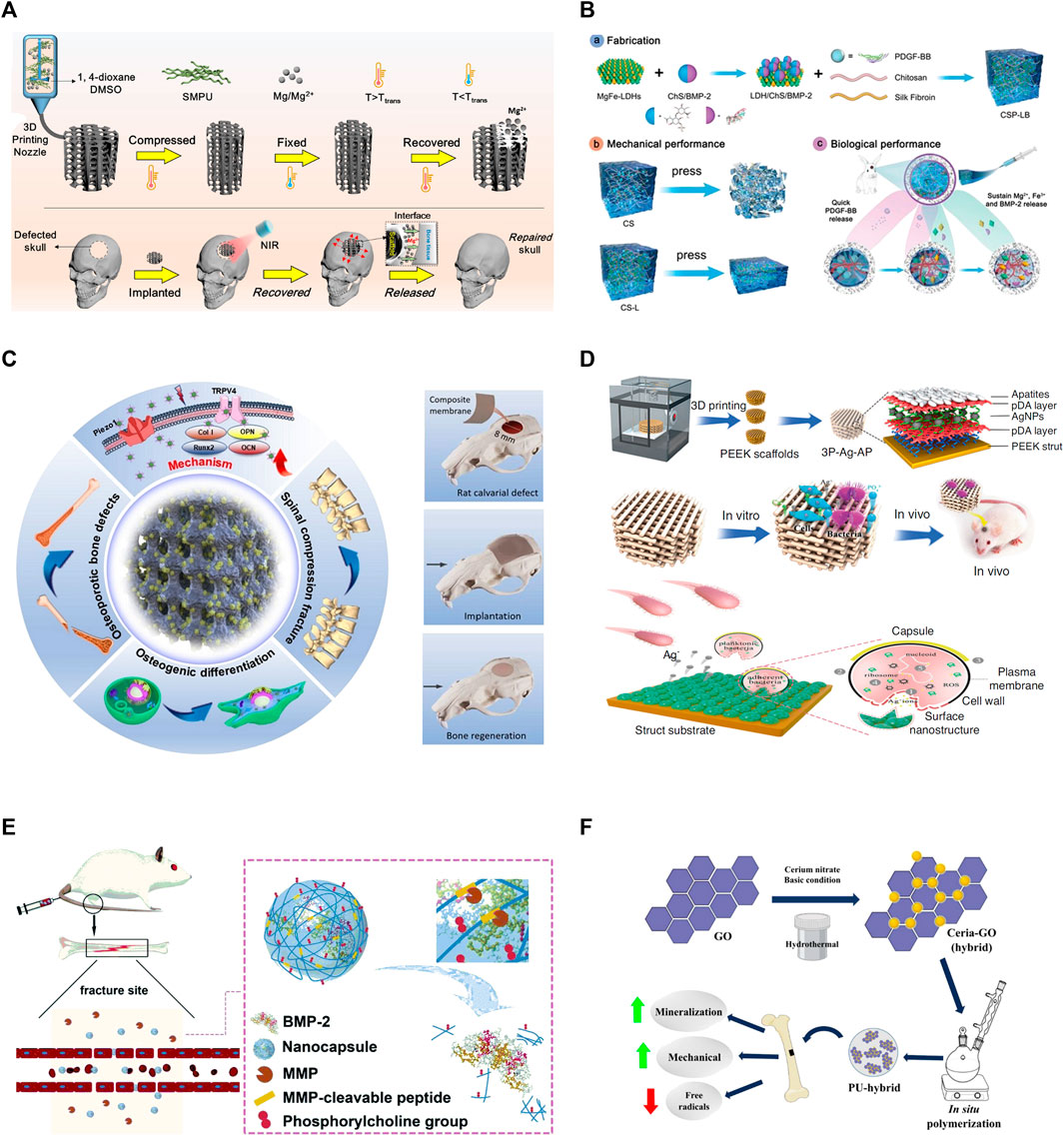
FIGURE 1. Representative mechanisms of smart polymeric biomaterials for bone regeneration. (A) Light-responsive polymers: NIR-induced tight contact of SMPU scaffold with bone structure and Mg+ release (Zhang et al., 2022). (B) Thermo-responsive polymers: Schematic illustration of the fabrication of CSP-LB hydrogel, enhanced mechanical and biological properties (Lv et al., 2023). (C) Electrically-responsive polymers: Porous poly (vinylidene fluoride-co-hexafluoropropylene) (PVDF-HFP) and calcium phosphate (CaP) coatings created the scaffold (left); Barium titanate (BTO)/polyvinylidene fluoride–trifluoroethylene (P(VDF-TrFE)) accelerate bone regeneration (right) (Ma Z. et al., 2023; Bai et al., 2023). (D) Enzyme-responsive polymers: MMP-induced BMP-2 release for fracture healing (Deng et al., 2020). (E) pH-responsive polymers: Bacteria-induced acid environments triggering drug release for anti-infection and osteogenesis (Qi et al., 2019). (F) Redox-responsive polymers: Ceria-Go-enhance radical-scavenging activity (Nilawar and Chatterjee, 2022).
Inflammatory response and low osteogenesis are two major issues that hinder bone regeneration. Kuang et al. developed a photo-responsive multicomponent hydrogel drug delivery system, which combined continuous drug release and NIR-controlled pulsatile drug release mechanisms together. In an osteoporosis animal model, the system can maintain parathyroid hormone (PTH) concentration in bone structure in a relatively stable manner, and thus promoted bone regeneration by achieving optimized osteoblast to osteoclast ratios (Kuang et al., 2021). In another study, osteo-inductive bone morphogenetic protein 2 (BMP-2) was attached to polydopamine-coated Mg-Ca carbonate microspheres which was incorporated into aspirin-containing hydroxybutyl chitosan (CS) hydrogel. The composite materials can release aspirin at early stage for anti-inflammatory effects, and then release BMP-2 to promote osteogenesis. Animal studies showed the presented composite promoted new bone formation (Wan et al., 2022). Furthermore, Wang et al. designed a NIR-triggered system with SrCl2-black phosphorous (BP)@PLGA microspheres, which can achieve on-demand release of Sr ions. After implanted into rat bone defects, the microspheres exhibit great biocompatibility and bone regeneration potential (Wang et al., 2018).
3 Thermo-responsive polymeric biomaterials for bone regeneration
Thermo-responsive biomaterials have piqued extensive interest, driven by their capacity for temperature-modulated bioactive agent release. Injectable systems enhance safety by transitioning phases without reliance on cross-linking agents, thereby avoiding denaturation. Moreover, the dynamic encapsulation process ensures therapeutic agents within the biomaterial. The rapid shift from a sol to a gel state at physiologic temperature eliminates the risk of premature burst release, optimizing the control of release kinetics (Duan et al., 2020).
Hydrogels’ therapeutic applications can be hampered by mechanical inadequacies and shrinkage during cell culture. To overcome this, Zhu et al. (2023) devised a thermo/photo dual-sensitive hydrogel, through physical and chemical cross-linking techniques, a thermo/photo dual-sensitive hydrogel was synthesized from methacrylated hydroxybutyl chitosan (MHBC) and chitin whisker (CHW). This M/C hydrogel exhibits a distinctive lamellar internal structure, and its mechanical properties and cellular compatibility can be tailored by modulating the M/C ratio.
A thermo-gel consisting of poly (ε-caprolactone-co-D,L-lactide)-poly (ethyleneglycol)-poly (ε-caprolactone-co-D,L-lactide) (PCLA-PEG-PCLA), simvastatin (SIM), strontium hydrogen phosphate (SrHPO4)/beta-tricalcium phosphate (beta-TCP) showcased superior osteocyte differentiation, facilitating bone tissue repair (Bian et al., 2023). Addressing periprosthetic wear debris-induced aseptic loosening, Lei et al. (2022) developed a thermosensitive PLGA-b-PEG-b-PLGA hydrogel. Infused with the TNF-alpha antagonist etanercept (ETN), the hydrogel mitigates debris-induced osteolysis through sustained ETN release, thereby reducing aseptic inflammation. For anterior cruciate ligament (ACL) repair, a thermos-responsive BP-FHE BP, primarily F127, oxidized hyaluronic acid (OHA), poly-epsilon-L-lysine (epsilon-EPL)) hydrogel was proven to promote mineralization, skin, and bone regeneration while reducing cytotoxicity, optimizing ACLR clinical use and recovery (Cho et al., 2023). Sui et al. (2023) L-PRF-based chitosan (CS)-hydroxyapatite (HAP) composite scaffold provides mechanical stability, sustained release, and enhanced cytotoxicity for bone regeneration. Another thermo-responsive hydrogel, chitosan/silk fibroin with platelet-derived MgFe-layered growth factor-BB (CSP-LB), incorporated with dual growth factors, exhibited improved angiogenesis, osteogenesis, bone regeneration, and mineral density compared to its CS counterpart, thanks to sequential growth factor release and sustained bioactive Mg2+/Fe3+ ion release as shown in Figure 1B (Khan et al., 2022b; Lv et al., 2023).
4 Electrically-responsive polymeric biomaterials for bone regeneration
The impact of electrical currents on bone formation is well-documented (Bassett et al., 1964), fostering the incorporation of conductive elements such as carbon nanofibers (Stout et al., 2012) and gold nanowires (Dvir et al., 2011) into conducting polymers. These polymers, soluble in organic solvents, can be blended with other polymers and processed into porous scaffolds, for instance, via electrospinning. Such uniformity enhances electrical signal transmission across the composite, influencing the behavior of all included cells.
Substantial strides have been made in scaffold designs for bone tissue engineering. For instance, a 3D-printed poly (l-lactic acid) (PLLA) scaffold was designed, featuring a fiber diameter of 150 μm and an osteogenic pore size of 450 μm, both crucial for bone growth. The design parameters were set to achieve the desired scaffold size. With impressive cytocompatibility, elasticity resembling that of trabecular bone, and inherent piezoelectric properties encouraging the adhesion of fibrinogen-coated osteoblast-like cells, these scaffolds exhibit significant promise (Karanth et al., 2023). Other notable developments include a 3D polyurethane foam (PUF) scaffold coated with piezoelectric PVDF-HFP and mineralized calcium phosphate (CaP), which stimulated osteogenic cell differentiation and in vivo ectopic bone formation due to its components’ synergistic effects (Ma et al., 2023a). BaTiO3 nanofibers (BTNF) integrated into a poly (vinylidene fluoridetrifluoroethylene) (P(VDF-TrFE)) matrix created an anisotropic surface potential, bolstering mechanotransduction, in vitro osteogenesis, and in vivo bone regeneration as shown in Figure 1C (Bai et al., 2023). Wang et al. (2023) suggested a composite scaffold consisting of piezoelectric Whitlockite (WH) and polycaprolactone (PCL) that fostered neurovascularized bone tissue regeneration through sustained Mg2+ release. A bifunctional composite formed by incorporating activated carbon nanotubes (ACNTs) into a polymethyl methacrylate (PMMA) matrix improved cell survival under electrical and magnetic stimuli (Li et al., 2022). A SiO2/PDMS composite electroactive membrane with embedded silicon dioxide electrets enhanced osteogenic differentiation and bone regrowth (Qiao et al., 2022). A pioneering approach introduced porous polymeric Fe3O4/GO scaffolds developed using cellulose and a co-dispersed nanosystem, exhibiting enhanced mechanical strength and antibacterial activities, as well as increased viability and proliferation of pre-osteoblast cell lines (Khan et al., 2022c). Lastly, a poly (l-lactic acid)-block-aniline pentamer (PLA-AP) and poly (lactic-co-glycolic acid)/hydroxyapatite (PLGA/HA-based) electroactive tissue engineering scaffold, loaded with the pSTAR-hBMP-4 plasmid, improved osteogenesis differentiation and bone healing, underlining the potential applications of multi-functional materials in bone tissue engineering (Cui et al., 2020).
5 pH-responsive polymeric biomaterials for bone regeneration
Osteoporosis, a metabolic bone disorder, arises from excessive osteoclast activity which breaks down bone structure via the secretion of acid and proteinases. Consequently, the pH of osteoporotic bones is lower than that of healthy ones (Blair, 1998). Bacterial infection, another major impediment to bone regeneration, can also lead to an acidic microenvironment around infection sites. Accordingly, pH-responsive polymeric biomaterials are predominantly used for the delivery of osteogenic and antibacterial drugs.
Deng et al. (2020) designed a dual-layer polydopamine coating for bone implants, incorporating silver nanoparticles (NPs) in the first layer and apatite in the second (Figure 1D). In response to bacterial infection, the coating releases Ag+, Ca2+, and PO43- ions. 3D-printed polyetheretherketone scaffolds modified with this coating demonstrated superior antibacterial and osteogenic properties in vitro, and promoted bone ingrowth and osseointegration in vivo in an infected bone defect. Another study introduced a drug release system composed of Poly [2-(dimethylamino) ethyl methacrylate] (PDMAEMA), chitosan, and a minocycline drug reservoir. As bacteria induce a pH reduction around the system, the pH-responsive PDMAEMA hydrogel propels the drug from the reservoir on-demand for bacterial inhibition with remarkable efficacy (Chen et al., 2023).
Synergistic effects of BMP-2 and dexamethasone (Dex) are critical for osteoblastic differentiation and bone regeneration. Gan et al. engineered a pH-sensitive, chitosan-functionalized mesoporous silica nanoparticle (chi-MSN). The design involves covalently attaching BMP-2 to chitosan and encapsulating smaller Dex molecules within the mesopores. Once delivered into cells, a lower pH triggers the release of Dex following the initial release of BMP-2. This system resulted in a substantial increase in osteoblastic differentiation and new bone formation in vivo over a period of 4 weeks (Gan et al., 2015). Finally, George et al. developed an injectable Oligo [poly (ethylene glycol) fumarate]-dopamine (OPF-DOPA) hydrogel that forms crosslinks under low pH conditions, subsequently increasing its stiffness and slowing its degradation rate. Notably, the hydrogel adheres to bone structures, preventing displacement of bone implants (George et al., 2022).
6 Enzyme-responsive polymeric biomaterials for bone regeneration
Enzymes are integral to bone growth and remodeling, modulating various signaling pathways within bone tissue such as cell proliferation, adhesion, and osteogenesis. Within the context of bone regeneration, native enzymes present in bone tissue, like matrix metalloproteinases (MMPs), can initiate specific reactions crucial for drug delivery, diagnostics, and tissue repair. Enzyme-responsive polymers have great biocompatibility, selectivity, and efficiency, and have excellent potential for bone regeneration.
Materials responsive to enzymes have also been employed to counteract bone infections. Polyglutamic acid (PG) is a homogeneous polymer featuring amide crosslinkers cleavable by the V8 enzyme, which is secreted by Staphylococcus aureus. Ding et al. (2020) encapsulated AgNPs into Mesoporous silica nanoparticles (MSNs), which were then enveloped by PG and polyallylamine hydrochloride (PAH) layers using a layer-by-layer technique. These nanoparticles were ultimately placed onto a polydopamine-coated surface as a titanium bone implant coating. The modified implants exhibited exceptional antimicrobial effects and significantly enhanced new bone formation in a bacteria-infected rat model. In a similar study for periodontal treatment, Alkaline Phosphatase (ALP) -responsive polyphosphoester and minocycline hydrochloride (PPEM) was incorporated into a chitosan membrane, and the effects were evaluated in cell and animal models. The results confirmed release of antibiotic and osteogenic drugs from PPEM membrane and their effects (Li et al., 2019).
In a recent study, researchers engineered Matrix metalloproteinases (MMP)-responsive nanocapsules to deliver bone BMP-2 for bone fracture healing. These nanocapsules, formed via in situ 2-(methacryloyloxy) ethyl phosphorylcholine (MPC) polymerization, incorporated the isacryloylated VPLGVRTK peptide as MMP cleavable crosslinkers on the BMP-2 surface, maintaining the functionality of BMP-2 throughout the process. The nanocapsules were delivered to fracture site via malformed blood vessels and accumulated there. Once MMPs disrupted the capsule, BMP-2 was released, facilitating bone regeneration as demonstrated with in vivo studies as shown in Figure 1E (Qi et al., 2019). Various other enzymes, including tyrosinase, lysozyme, horseradish peroxidase, transglutaminase (TG), and alkaline phosphatase (AP), have been examined for their potential to induce beneficial reactions for bone regeneration (Yuan et al., 2018; Sood et al., 2022).
7 Redox-responsive polymeric biomaterials for bone regeneration
Redox signaling pathways, predicated on electron transfer and free radicals, underpin mammalian bone formation and regeneration, especially balancing reactive oxygen species (ROS) (Zhang et al., 2023). Recent discoveries underline the post-fracture influence of redox on cellular responses.
Well-known for their role in redox modulation, ceria nanoparticles have been incorporated into a polyurethane matrix alongside graphene, creating a multifunctional biomaterial. Ceria-graphene oxide hybrid nanoparticles were synthesized through a hydrothermal process that started with the sonication of graphene oxide in distilled water. Following this, cerium nitrate hexahydrate was incorporated into the mixture and stirred magnetically. The pH was elevated to 10 through the addition of an ammonia hydroxide solution, and stirring was continued. The composite was then placed into a Teflon-lined stainless steel hydrothermal reactor and kept in an oven. Particles were dried in a hot air oven. Ceria nanoparticles and reduced graphene oxide sheets were similarly synthesized, albeit without adding graphene oxide and the cerium precursor, respectively. The end products displayed enhanced properties, specifically in terms of radical scavenging and osteogenesis (Nilawar et al., 2023). Ceria-graphene oxide hybrid nanoparticles were synthesized via a hydrothermal process and demonstrated heightened radical-scavenging and osteogenic properties. The bioactivity of 3D-printed, porous PLA scaffolds can be augmented by ceria, fostering osteogenesis enhancement and antimicrobial properties as shown in Figure 1F (Nilawar and Chatterjee, 2022). Further, nanoceria-cellulose-gelatin scaffolds (CG-NCs) have been crafted to combat ROS-induced oxidative stress inhibiting bone repair, boasting superior mechanical properties, bio-mineralization capabilities, and promoting cell proliferation and differentiation (Singh et al., 2023).
Gelatin methacrylate (GelMA) hydrogels, enhanced with magnesium-seamed C-propylpyrogallol[4]arene (PgC (3) Mg), offering dual-release of bioactive Mg2+ and antioxidants, boosting bioactivity and resilience to oxidative stress. The modified hydrogels decreased intracellular ROS levels and improved bone repair in severe cranial defects (Tan et al., 2022). Moreover, scaffold combining radially aligned mineralized collagen (RA-MC) fibers and nanosilicon (nSi) exhibited osteoconductivity and osteoinductivity, guiding reparative cells and reducing inflammation, thus showing promise for major bone defect repair (Mac et al., 2022). Additionally, sodium alginate hydrogel, embedded with calcium peroxide nanoparticles and vitamin C, has shown promising results in alleviating bone defect hypoxia and promoting bone healing under hypoxic conditions (Zhao et al., 2021). YQ Chen’s research focuses on enhancing the biocompatibility, biosafety, and biodegradability of polysaccharide-based hydrogels, used as 3D scaffolds for bone healing. A photocrosslinked composite hydrogel was synthesized under UV irradiation, merging a novel, water-soluble phosphate-functionalized chitosan (CSMAP), prepared with methacrylic anhydride (MA) and phosphonopropionic acid (P), and strontium phosphosilicate (SPS) bioceramic nanoparticles. The CSMAP-SPS hydrogel’s porous network amplified mechanical strength and bioactive ion release. This hydrogel demonstrated superior biomineralization, cytocompatibility with preosteoblast MC3T3-E1 cells, and encouraged osteogenic differentiation and endothelial tube formation, suggesting potential utility in bone regeneration (Chen et al., 2021).
8 Others smart polymeric biomaterials for bone regeneration
Several stimuli-responsive biomaterials warrant further exploration, particularly in mechanical, magnetic, and acoustic domains. In the mechanical field, Wolff’s 1892 hypothesis proposed bone’s responsiveness to biophysical stimuli, shedding light on bone and tissue healing as well as the impact of workouts and machine-induced stress on bone and mesenchymal tissue development (Ma et al., 2023b). On the other hand, specific polymers are capable of responding to compression, shear and other mechanical stimuli with network structural change or polymeric degradation, which affect bone implant design and drug delivery. PLA scaffold reinforced with 20% magnesium demonstrated 2.4 times of degradation rate in the presence of 3 MP static compression during a 30-day period, while fluid shear stress greatly increased PLAG degradation (Chu et al., 2017; Chu et al., 2019). The results suggest that carefully chosen stress-responsive polymers may play important roles in bone regeneration. Magnetic-responsive biomaterials also show potential, particularly when pristine superparamagnetic iron oxide nanoparticles (pSPIONs) are incorporated into additively manufactured scaffolds. Such scaffolds, composed of chitosan (CS), poly (vinyl alcohol) (PVA), and hydroxyapatite (HA), exhibit enhanced magnetic properties useful for magnetic hyperthermia and bone regeneration. Notably, the presence of pSPIONs increases cell adherence, proliferation, and ALP expression in human osteosarcoma Saos-2 cells, making these scaffolds a promising choice for bone regenerative applications (Tavares et al., 2023). The synergy of low-intensity pulsed ultrasound and lipid microbubbles with 3D-printed PLGA/TCP scaffolds has also been demonstrated to enhance bone marrow stem cell growth and differentiation, representing a potential strategy for bone regeneration (Jin et al., 2023). Furthermore, titanium-hydroxyapatite and titanium-wollastonite composites exhibit physicochemical and biocompatible properties conducive to future bone implants, underscoring the potential of metal-ceramic composites in bone implant advancements (Shanmuganantha et al., 2022).
9 Discussion and conclusion
Smart biopolymers exhibit potential in bone regeneration via innovative scaffold construction, material enhancement, and tailored drug delivery, thereby providing diverse therapeutic avenues. As reviewed before, light-responsive polymers enhance antibacterial effectiveness and bone repair in 3D-printed scaffolds and photothermal treatments. Thermo-responsive materials, conductive polymers, and pH-responsive biomaterials have demonstrated potential in controlled drug release, improved cellular behavior, and combating osteoporosis and infections, respectively. Also, enzyme-responsive polymers and redox signaling pathways targeting materials have shown promise in bone regeneration and infection mitigation. Stimuli-responsive materials have made advances in the mechanical, magnetic, and acoustic domains. Notably, magnetic-responsive biomaterials enhance cell adherence and proliferation, while acoustic-responsive materials stimulate stem cell growth and bone differentiation. The advantages and limitations of smart biopolymers were summarized in Table 1.
Despite the considerable potential of smart biopolymers in bone regeneration, obstacles persist. Interactions of biomaterials depend on factors like size, charge, and shape, and the application of responsive polymers remains challenging due to deep tissue penetration limits, mechanical properties variability, and potential induction of oxidative stress. In conclusion, remarkable advances in bone regeneration have been made, but the ideal polymeric materials for this purpose remain to be developed. Their biophysical and biochemical properties should be further exploited to guide material design and fabrication. Additionally, more theoretical and experimental studies are needed to facilitate controllable manipulation to explore their potentials. Furthermore, interdisciplinary collaborations with artificial intelligence (AI) may also foster designs of polymeric biomaterials for bone repair. AI-assisted techniques such as multi stimuli-responsive methodologies and robocasting may enable biomaterial customization with potential breakthroughs in pore shape control and deep tissue penetration to unlock their full potential in bone regeneration.
Author contributions
CS and YX wrote the manuscript. SD, LQ, and DL provided supports of reference and suggestions. All authors contributed to the article and approved the submitted version.
Funding
This research was funded by Shantou University (STU Scientific Research Foundation for Talents: NTF21014 to YX, NTF21032 to CS) and the 2020 Li Ka Shing Foundation Cross-Disciplinary Research Grant (2020LKSFG02C).
Conflict of interest
The authors declare that the research was conducted in the absence of any commercial or financial relationships that could be construed as a potential conflict of interest.
Publisher’s note
All claims expressed in this article are solely those of the authors and do not necessarily represent those of their affiliated organizations, or those of the publisher, the editors and the reviewers. Any product that may be evaluated in this article, or claim that may be made by its manufacturer, is not guaranteed or endorsed by the publisher.
Supplementary material
The Supplementary Material for this article can be found online at: https://www.frontiersin.org/articles/10.3389/fbioe.2023.1240861/full#supplementary-material
References
Agarwal, R., and García, A. J. (2015). Biomaterial strategies for engineering implants for enhanced osseointegration and bone repair. Adv. Drug Deliv. Rev. 94, 53–62. doi:10.1016/j.addr.2015.03.013
Amini, A. R., Laurencin, C. T., and Nukavarapu, S. P. (2012). Bone tissue engineering: recent advances and challenges. Crit. Rev. Biomed. Eng. 40 (5), 363–408. doi:10.1615/critrevbiomedeng.v40.i5.10
Bai, Y., Zheng, X., Zhong, X., Cui, Q., Zhang, S., Wen, X., et al. (2023). Manipulation of heterogeneous surface electric potential promotes osteogenesis by strengthening RGD peptide binding and cellular mechanosensing. Adv. Mater 35, e2209769. doi:10.1002/adma.202209769
Bassett, C. A., Pawluk, R. J., and Becker, R. O. (1964). Effects of electric currents on bone in vivo. Nature 204, 652–654. doi:10.1038/204652a0
Battafarano, G., Rossi, M., De Martino, V., Marampon, F., Borro, L., Secinaro, A., et al. (2021). Strategies for bone regeneration: from graft to tissue engineering. Int. J. Mol. Sci. 22 (3), 1128. doi:10.3390/ijms22031128
Bian, Q., Guo, C., Cui, S., Liu, J., Xu, G., and Feng, W. (2023). Accelerating bone regeneration in cranial defects using an injectable organic-inorganic composite hydrogel. J. Mater Chem. B 11 (16), 3713–3726. doi:10.1039/d3tb00332a
Blair, H. C. (1998). How the osteoclast degrades bone. Bioessays 20 (10), 837–846. doi:10.1002/(sici)1521-1878(199810)20:10<837::aid-bies9>3.0.co;2-d
Bustamante-Torres, M., Romero-Fierro, D., Arcentales-Vera, B., Palomino, K., Magaña, H., and Bucio, E. (2021). Hydrogels classification according to the physical or chemical interactions and as stimuli-sensitive materials. Gels 7 (4), 182. doi:10.3390/gels7040182
Cerqueni, G., Scalzone, A., Licini, C., Gentile, P., and Mattioli-Belmonte, M. (2021). Insights into oxidative stress in bone tissue and novel challenges for biomaterials. Mater Sci. Eng. C Mater Biol. Appl. 130, 112433. doi:10.1016/j.msec.2021.112433
Chen, Y., Udduttula, A., Xie, X., Zhou, M., Sheng, W., Yu, F., et al. (2021). A novel photocrosslinked phosphate functionalized Chitosan-Sr5(PO4)2SiO4 composite hydrogels and in vitro biomineralization, osteogenesis, angiogenesis for bone regeneration application. Compos. Part B Eng. 222, 109057. doi:10.1016/j.compositesb.2021.109057
Chen, H., Tan, W., Tong, T., Shi, X., Ma, S., and Zhu, G. (2023). A pH-responsive asymmetric microfluidic/chitosan device for drug release in infective bone defect treatment. Int. J. Mol. Sci. 24 (5), 4616. doi:10.3390/ijms24054616
Cho, E., Qiao, Y., Chen, C., Xu, J., Cai, J., Li, Y., et al. (2023). Injectable FHE+BP composites hydrogel with enhanced regenerative capacity of tendon-bone interface for anterior cruciate ligament reconstruction. Front. Bioeng. Biotechnol. 11, 1117090. doi:10.3389/fbioe.2023.1117090
Chu, Z., Li, X., Li, Y., Zheng, Q., Feng, C., Guo, M., et al. (2017). Effects of different fluid shear stress patterns on the in vitro degradation of poly(lactide-co-glycolide) acid membranes. J. Biomed. Mater Res. A 105 (1), 23–30. doi:10.1002/jbm.a.35860
Chu, C. L., Li, X., Yu, W., Han, L., Bai, J., and Xue, F. (2019). Degradation behaviors of PLA-matrix composite with 20vol% magnesium alloy wires under static loading conditions. J. Mater. Sci. 54 (6), 4701–4709. doi:10.1007/s10853-018-03199-5
Cui, L., Zhang, J., Zou, J., Yang, X., Guo, H., Tian, H., et al. (2020). Electroactive composite scaffold with locally expressed osteoinductive factor for synergistic bone repair upon electrical stimulation. Biomaterials 230, 119617. doi:10.1016/j.biomaterials.2019.119617
Deng, Y., Shi, X., Chen, Y., Yang, W., Ma, Y., Shi, X. L., et al. (2020). Bacteria-triggered pH-responsive osteopotentiating coating on 3D-printed polyetheretherketone scaffolds for infective bone defect repair. Industrial Eng. Chem. Res. 59, 12123–12135. doi:10.1021/acs.iecr.0c02107
Ding, Y., Hao, Y., Yuan, Z., Tao, B., Chen, M., Lin, C., et al. (2020). A dual-functional implant with an enzyme-responsive effect for bacterial infection therapy and tissue regeneration. Biomater. Sci. 8 (7), 1840–1854. doi:10.1039/c9bm01924c
Duan, J., Huang, Y., Zong, S., and Jiang, J. (2020). Preparation and drug release properties of a thermo sensitive ga hydrogel. Polym. (Basel) 13 (1), 119. doi:10.3390/polym13010119
Dvir, T., Timko, B. P., Brigham, M. D., Naik, S. R., Karajanagi, S. S., Levy, O., et al. (2011). Nanowired three-dimensional cardiac patches. Nat. Nanotechnol. 6 (11), 720–725. doi:10.1038/nnano.2011.160
Filippi, M., Born, G., Chaaban, M., and Scherberich, A. (2020). Natural polymeric scaffolds in bone regeneration. Front. Bioeng. Biotechnol. 8, 474. doi:10.3389/fbioe.2020.00474
Gan, Q., Zhu, J., Yuan, Y., Liu, H., Qian, J., Li, Y., et al. (2015). A dual-delivery system of pH-responsive chitosan-functionalized mesoporous silica nanoparticles bearing BMP-2 and dexamethasone for enhanced bone regeneration. J. Mater Chem. B 3 (10), 2056–2066. doi:10.1039/c4tb01897d
George, M. N., Liu, X., Miller, A. L., Zuiker, E., Xu, H., and Lu, L. (2022). Injectable pH-responsive adhesive hydrogels for bone tissue engineering inspired by the underwater attachment strategy of marine mussels. Biomater. Adv. 133, 112606. doi:10.1016/j.msec.2021.112606
Heng, B. C., Bai, Y., Li, X., Meng, Y., Lu, Y., Zhang, X., et al. (2023). The bioelectrical properties of bone tissue. Anim. Model. Exp. Med. 6 (2), 120–130. doi:10.1002/ame2.12300
Jin, L., Shan, J., Hao, Y., Wang, Y., and Liu, L. (2023). Enhanced bone regeneration by low-intensity pulsed ultrasound and lipid microbubbles on PLGA/TCP 3D-printed scaffolds. BMC Biotechnol. 23 (1), 13. doi:10.1186/s12896-023-00783-9
Karanth, D., Puleo, D., Dawson, D., Holliday, L. S., and Sharab, L. (2023). Characterization of 3D printed biodegradable piezoelectric scaffolds for bone regeneration. Clin. Exp. Dent. Res. 9 (2), 398–408. doi:10.1002/cre2.712
Khan, M. U. A., Razak, S. I. A., Rehman, S., Hasan, A., Qureshi, S., and Stojanović, G. M. (2022a). Bioactive scaffold (sodium alginate)-g-(nHAp@SiO(2)@GO) for bone tissue engineering. Int. J. Biol. Macromol. 222, 462–472. doi:10.1016/j.ijbiomac.2022.09.153
Khan, M. U. A., Al-Arjan, W. S., Ashammakhi, N., Haider, S., Amin, R., and Hasan, A. (2022b). Multifunctional bioactive scaffolds from ARX-g-(Zn@rGO)-HAp for bone tissue engineering: in vitro antibacterial, antitumor, and biocompatibility evaluations. ACS Appl. Bio Mater 5 (11), 5445–5456. doi:10.1021/acsabm.2c00777
Khan, M. U. A., Rizwan, M., Razak, S. I. A., Hassan, A., Rasheed, T., and Bilal, M. (2022c). Electroactive polymeric nanocomposite BC-g-(Fe(3)O(4)/GO) materials for bone tissue engineering: in vitro evaluations. J. Biomater. Sci. Polym. Ed. 33 (11), 1349–1368. doi:10.1080/09205063.2022.2054544
Kohane, D. S., and Langer, R. (2008). Polymeric biomaterials in tissue engineering. Pediatr. Res. 63 (5), 487–491. doi:10.1203/01.pdr.0000305937.26105.e7
Kuang, L., Huang, J., Liu, Y., Li, X., Yuan, Y., and Liu, C. (2021). Injectable hydrogel with NIR light-responsive, dual-mode PTH release for osteoregeneration in osteoporosis. Adv. Funct. Mater. 31 (47), 2105383. doi:10.1002/adfm.202105383
Laurencin, C. T., and Khan, Y. (2012). Regenerative engineering. Sci. Transl. Med. 4 (160), 160ed9. doi:10.1126/scitranslmed.3004467
Lavanya, K., Chandran, S. V., Balagangadharan, K., and Selvamurugan, N. (2020). Temperature- and pH-responsive chitosan-based injectable hydrogels for bone tissue engineering. Mater Sci. Eng. C Mater Biol. Appl. 111, 110862. doi:10.1016/j.msec.2020.110862
Lei, K., Wang, Y., Peng, X., Yu, L., and Ding, J. (2022). Long-term delivery of etanercept mediated via a thermosensitive hydrogel for efficient inhibition of wear debris-induced inflammatory osteolysis. J. Polym. Sci. 60 (20), 2875–2888. doi:10.1002/pol.20220337
Li, N., Jiang, L., Jin, H., Wu, Y., Liu, Y., Huang, W., et al. (2019). An enzyme-responsive membrane for antibiotic drug release and local periodontal treatment. Colloids Surf. B Biointerfaces 183, 110454. doi:10.1016/j.colsurfb.2019.110454
Li, M., Zhou, Q., Song, H., Lu, Y., Lu, M., Lu, X., et al. (2022). Electrically-magnetically bifunctional PMMA composite with improved physicochemical properties and promoting BMSC growth under electrical and magnetic stimulation. Mater. Lett. 314, 131780. doi:10.1016/j.matlet.2022.131780
Liao, J., Han, R., Wu, Y., and Qian, Z. (2021). Review of a new bone tumor therapy strategy based on bifunctional biomaterials. Bone Res. 9 (1), 18. doi:10.1038/s41413-021-00139-z
Lv, Z., Hu, T., Bian, Y., Wang, G., Wu, Z., Li, H., et al. (2023). A MgFe-ldh nanosheet-incorporated smart thermo-responsive hydrogel with controllable growth factor releasing capability for bone regeneration. Adv. Mater 35 (5), e2206545. doi:10.1002/adma.202206545
Ma, Z., Hu, X., Zhang, Y., Li, X., Chen, B., An, Q., et al. (2023a). Biomineralized piezoelectrically active scaffolds for inducing osteogenic differentiation. Chemistry 29 (15), e202203166. doi:10.1002/chem.202203166
Ma, Q., Miri, Z., Haugen, H. J., Moghanian, A., and Loca, D. (2023b). Significance of mechanical loading in bone fracture healing, bone regeneration, and vascularization. J. Tissue Eng. 14, 204173142311725. doi:10.1177/20417314231172573
Mac, C. H., Chan, H. Y., Lin, Y. H., Sharma, A. K., Song, H. L., Chan, Y. S., et al. (2022). Engineering a biomimetic bone scaffold that can regulate redox homeostasis and promote osteogenesis to repair large bone defects. Biomaterials 286, 121574. doi:10.1016/j.biomaterials.2022.121574
Montoya, C., Du, Y., Gianforcaro, A. L., Orrego, S., Yang, M., and Lelkes, P. I. (2021). On the road to smart biomaterials for bone research: definitions, concepts, advances, and outlook. Bone Res. 9 (1), 12. doi:10.1038/s41413-020-00131-z
Municoy, S., Álvarez Echazú, M. I., Antezana, P. E., Galdopórpora, J. M., Olivetti, C., Mebert, A. M., et al. (2020). Stimuli-responsive materials for tissue engineering and drug delivery. Int. J. Mol. Sci. 21 (13), 4724. doi:10.3390/ijms21134724
Nilawar, S., and Chatterjee, K. (2022). Surface decoration of redox-modulating nanoceria on 3D-printed tissue scaffolds promotes stem cell osteogenesis and attenuates bacterial colonization. Biomacromolecules 23 (1), 226–239. doi:10.1021/acs.biomac.1c01235
Nilawar, S., Bs, M., and Chatterjee, K. (2023). Nanoceria-decorated graphene nanosheets enhance mechanical properties and bioactivity of a degradable polyurethane for biomedical applications. J. Polym. Environ. 31, 2941–2955. doi:10.1007/s10924-023-02786-1
Ogle, O. E. (2015). Implant surface material, design, and osseointegration. Dent. Clin. North Am. 59 (2), 505–520. doi:10.1016/j.cden.2014.12.003
Ogueri, K. S., Jafari, T., Escobar Ivirico, J. L., and Laurencin, C. T. (2019). Polymeric biomaterials for scaffold-based bone regenerative engineering. Regen. Eng. Transl. Med. 5 (2), 128–154. doi:10.1007/s40883-018-0072-0
Pokharel, M., and Park, K. (2022). Light mediated drug delivery systems: A review. J. Drug Target 30 (4), 368–380. doi:10.1080/1061186x.2021.2005610
Qi, H., Yang, L., Li, X., Sun, X., Zhao, J., Hou, X., et al. (2019). Systemic administration of enzyme-responsive growth factor nanocapsules for promoting bone repair. Biomater. Sci. 7 (4), 1675–1685. doi:10.1039/c8bm01632a
Qiao, Z., Lian, M., Liu, X., Zhang, X., Han, Y., Ni, B., et al. (2022). Electreted sandwich membranes with persistent electrical stimulation for enhanced bone regeneration. ACS Appl. Mater Interfaces 14 (28), 31655–31666. doi:10.1021/acsami.2c06665
Shanmuganantha, L., Aslam Khan, M. U., Sulong, A. B., Ramli, M. I., Baharudin, A., Ariffin, H. M., et al. (2022). Characterization of titanium ceramic composite for bone implants applications. Ceram. Int. 48 (16), 22808–22819. doi:10.1016/j.ceramint.2022.04.140
Singh, S., Bhushan, S., Das, A., Barui, A., and Dutt, D. (2023). Surgical cotton microfibers loaded with nanoceria: A new platform for bone tissue engineering. Ceram. Int. 49 (1), 1114–1127. doi:10.1016/j.ceramint.2022.09.087
Sivakumar, P. M., Yetisgin, A. A., Sahin, S. B., Demir, E., and Cetinel, S. (2022). Bone tissue engineering: anionic polysaccharides as promising scaffolds. Carbohydr. Polym. 283, 119142. doi:10.1016/j.carbpol.2022.119142
Sobol, E., Shekhter, A., Guller, A., Baum, O., and Baskov, A. (2011). Laser-induced regeneration of cartilage. J. Biomed. Opt. 16 (8), 080902. doi:10.1117/1.3614565
Sood, A., Ji, S. M., Kumar, A., and Han, S. S. (2022). Enzyme-triggered crosslinked hybrid hydrogels for bone tissue engineering. Mater. (Basel) 15 (18), 6383. doi:10.3390/ma15186383
Stevens, M. M. (2008). Biomaterials for bone tissue engineering. Mater. Today 11 (5), 18–25. doi:10.1016/s1369-7021(08)70086-5
Stout, D. A., Yoo, J., Santiago-Miranda, A. N., Noemi Santiago-Miranda, A., and Webster, T. J. (2012). Mechanisms of greater cardiomyocyte functions on conductive nanoengineered composites for cardiovascular application. Int. J. Nanomedicine 7, 5653–5669. doi:10.2147/IJN.S34574
Sui, X., Zhang, H., Yao, J., Yang, L., Zhang, X., Li, L., et al. (2023). 3D printing of 'green' thermo-sensitive chitosan-hydroxyapatite bone scaffold based on lyophilized platelet-rich fibrin. Biomed. Mater 18 (2), 025022. doi:10.1088/1748-605x/acbad5
Tan, X., Wu, J., Wang, R., Wang, C., Sun, Y., Wang, Z., et al. (2022). PgC(3)Mg metal-organic cages functionalized hydrogels with enhanced bioactive and ROS scavenging capabilities for accelerated bone regeneration. J. Mater Chem. B 10 (28), 5375–5387. doi:10.1039/d2tb00907b
Tavares, F., Soares, P. I. P., Silva, J. C., and Borges, J. P. (2023). Preparation and in vitro characterization of magnetic CS/PVA/HA/pSPIONs scaffolds for magnetic hyperthermia and bone regeneration. Int. J. Mol. Sci. 24 (2), 1128. doi:10.3390/ijms24021128
Tomatsu, I., Peng, K., and Kros, A. (2011). Photoresponsive hydrogels for biomedical applications. Adv. Drug Deliv. Rev. 63 (14-15), 1257–1266. doi:10.1016/j.addr.2011.06.009
Tong, L., Liao, Q., Zhao, Y., Huang, H., Gao, A., Zhang, W., et al. (2019). Near-infrared light control of bone regeneration with biodegradable photothermal osteoimplant. Biomaterials 193, 1–11. doi:10.1016/j.biomaterials.2018.12.008
Wan, Z., Dong, Q., Guo, X., Bai, X., Zhang, X., Zhang, P., et al. (2022). A dual-responsive polydopamine-modified hydroxybutyl chitosan hydrogel for sequential regulation of bone regeneration. Carbohydr. Polym. 297, 120027. doi:10.1016/j.carbpol.2022.120027
Wang, X., Shao, J., Abd El Raouf, M., Xie, H., Huang, H., Wang, H., et al. (2018). Near-infrared light-triggered drug delivery system based on black phosphorus for in vivo bone regeneration. Biomaterials 179, 164–174. doi:10.1016/j.biomaterials.2018.06.039
Wang, Z., Wang, Y., Yan, J., Zhang, K., Lin, F., Xiang, L., et al. (2021). Pharmaceutical electrospinning and 3D printing scaffold design for bone regeneration. Adv. Drug Deliv. Rev. 174, 504–534. doi:10.1016/j.addr.2021.05.007
Wang, L., Pang, Y., Tang, Y., Wang, X., Zhang, D., Zhang, X., et al. (2023). A biomimetic piezoelectric scaffold with sustained Mg(2+) release promotes neurogenic and angiogenic differentiation for enhanced bone regeneration. Bioact. Mater 25, 399–414. doi:10.1016/j.bioactmat.2022.11.004
Wei, M., Gao, Y., Li, X., and Serpe, M. J. (2017). Stimuli-responsive polymers and their applications. Polym. Chem. 8 (1), 127–143. doi:10.1039/c6py01585a
Wei, H., Cui, J., Lin, K., Xie, J., and Wang, X. (2022). Recent advances in smart stimuli-responsive biomaterials for bone therapeutics and regeneration. Bone Res. 10 (1), 17. doi:10.1038/s41413-021-00180-y
Yuan, Z., Huang, S., Lan, S., Xiong, H., Tao, B., Ding, Y., et al. (2018). Surface engineering of titanium implants with enzyme-triggered antibacterial properties and enhanced osseointegration in vivo. J. Mater Chem. B 6 (48), 8090–8104. doi:10.1039/c8tb01918e
Zeng, J., Wang, Y., Sun, Z., Chang, H., Cao, M., Zhao, J., et al. (2020). A novel biocompatible PDA/IR820/DAP coating for antibiotic/photodynamic/photothermal triple therapy to inhibit and eliminate Staphylococcus aureus biofilm. Chem. Eng. J. 394, 125017. doi:10.1016/j.cej.2020.125017
Zhang, P., Xiao, C., and Chen, X. (2021). Stimuli-responsive polypeptides for controlled drug delivery. Chem. Commun. (Camb) 57 (75), 9489–9503. doi:10.1039/d1cc04053g
Zhang, Y., Li, C., Zhang, W., Deng, J., Nie, Y., Du, X., et al. (2022). 3D-printed NIR-responsive shape memory polyurethane/magnesium scaffolds with tight-contact for robust bone regeneration. Bioact. Mater 16, 218–231. doi:10.1016/j.bioactmat.2021.12.032
Zhang, H., Hao, J., Hong, H., Gu, W., Li, Z., Sun, J., et al. (2023). Redox signaling regulates the skeletal tissue development and regeneration. Biotechnol. Genet. Eng. Rev. Epub ahead of print 12, 1–24. doi:10.1080/02648725.2023.2199244
Zhao, B., He, J., Wang, F., Xing, R., Sun, B., and Zhou, Y. (2021). Polyacrylamide-sodium alginate hydrogel releasing oxygen and vitamin C promotes bone regeneration in rat skull defects. Front. Mater. 8. doi:10.3389/fmats.2021.758599
Zhu, Y., Qin, D., Liu, J., Wu, G., Wang, H., Wu, F., et al. (2023). Chitin whiskers enhanced methacrylated hydroxybutyl chitosan hydrogels as anti-deformation scaffold for 3D cell culture. Carbohydr. Polym. 304, 120483. doi:10.1016/j.carbpol.2022.120483
Glossary
Keywords: smart biomaterials, bone regeneration, stimuli, polymer, osteogenic
Citation: Xing Y, Qiu L, Liu D, Dai S and Sheu C-L (2023) The role of smart polymeric biomaterials in bone regeneration: a review. Front. Bioeng. Biotechnol. 11:1240861. doi: 10.3389/fbioe.2023.1240861
Received: 15 June 2023; Accepted: 02 August 2023;
Published: 17 August 2023.
Edited by:
Muhammad Umar Aslam Khan, Qatar University, QatarReviewed by:
Muhammad Yasir, University of Lahore, PakistanRana Manzoor Ahmad, Government College University, Lahore, Pakistan
Copyright © 2023 Xing, Qiu, Liu, Dai and Sheu. This is an open-access article distributed under the terms of the Creative Commons Attribution License (CC BY). The use, distribution or reproduction in other forums is permitted, provided the original author(s) and the copyright owner(s) are credited and that the original publication in this journal is cited, in accordance with accepted academic practice. No use, distribution or reproduction is permitted which does not comply with these terms.
*Correspondence: Chia-Lin Sheu, eHVqaWFsaW5Ac3R1LmVkdS5jbg==